- 1Géosciences Environnement Toulouse, Observatoire Midi-Pyrénées, Université Toulouse III/CNRS/IRD/CNES, Toulouse, France
- 2Department of Geology and Geological Engineering, Colorado School of Mines, Golden, CO, United States
Magma’s volatile budget depends on deep magmatic sources, the degree of differentiation and degassing conditions, and volatile input from the assimilated crust. It is, therefore, important to know the exact mechanism by which the crust is assimilated into magma to understand volatile budgets and eruption behavior. To explore reactions between carbonate, calc–silicate, or skarn xenoliths and basaltic andesite magma, we studied ten calc–silicate xenoliths from the 1994, 1998, 2006, and 2010 Merapi eruptions and four sediment samples of local Javanese carbonate crust. An in situ electron probe microanalysis of the 1994–2010 calc–silicate xenolith minerals and glasses suggests that calcite is a minor and metastable mineral phase in association with wollastonite. In addition, carbonate melts quenched to calcic glasses (32 ± 7 wt% SiO2; 38 ± 3 wt% CaO), similar to experimental glasses produced by crust–melt interaction experiments. Thermodynamic modeling using rhyolite MELTS (version 1.2.0) predicts the production of highly silicic (up to ∼84 wt% of SiO2) and CaO-rich (up to ∼25 wt%) melts during partial melting of calc–silicate material. The observed mechanism of calc–silicate xenolith assimilation is the generation of highly silicic (77 ± 4 wt% of SiO2) melts in association with idiomorphic diopside [Wo49En29; 57 ± 3 Mg# = Mg/(Mg+Fe2+)] and other calcic pyroxenes (Wo54-82 En2-21; 16–43 Mg#) due to partial melting of xenolith and incongruent dissolution reactions. We hypothesize that the rate-limiting process is the subsequent mixing of the produced crustal melts with representative resident andesitic melt (average 65 wt% SiO2) through chemical diffusion, which explains major and volatile (Cl) element contents in the Merapi glass products. In addition to high Sr contents and radiogenic 87Sr/86Sr and elevated CO2, Ba, Co, Cr, Cu, V, Zn, and Zr contents in the magmatic minerals and associated glasses, the recrystallized and residual metamorphic sphene, quartz, garnet, and apatite predicted by rhyolite-MELTS or Magma Chamber Simulator modeling during wallrock melting and residual metastable calcite and wollastonite are important tracers of calc–silicate crust assimilation. The disequilibrium process of calc–silicate crustal assimilation can, thus, be well predicted by dissolution experiments and thermodynamic modeling using rhyolite-MELTS or Magma Chamber Simulator. The rate of calc–silicate crustal assimilation is still unconstrained without adequate high-temperature kinetic time-series experiments. We predict that the crustal assimilation rate is controlled by the Si–Al diffusion and associated convection in the hydrous silicate magma.
Introduction
Decarbonation of the arc-crust beneath Merapi may magnify the volcanic CO2 output and potentially contribute to the volcano’s eruption behavior (Borisova et al., 2013, 2016; Erdmann et al., 2014, 2016; Deegan et al., 2023). Numerous experimental works devoted to pure carbonate solubility in mafic melts demonstrated the important role that the addition of carbonate material has on the magma liquidus phase stability field (Freda et al., 2008; Iacono-Marziano et al., 2008, 2009; Mollo et al., 2010). Deegan et al. (2010), Jolis et al. (2013), and Blythe et al. (2015) experimentally studied the interaction of carbonate materials with mafic and alkaline melts at conditions corresponding to the deep crust. They discovered that the direct interaction of carbonates with the silicate melt is rapid (minutes), with the outcome being the formation of Ca-rich carbonate melts. Chadwick et al. (2007) documented the formation of Ca-rich glasses in calc–silicate xenoliths that were assimilated by the 1994–1998 Merapi (Indonesia) magmas. Costa et al. (2013), Borisova et al. (2013; 2016) and Troll et al. (2013) studied the lava, ash, xenolith, and igneous samples of recent Merapi eruptions. Geochemical and detailed in situ data on the glasses and minerals confirm an important effect that the crustal level (∼200 MPa depths, Borisova et al., 2013; Erdmann et al., 2016) assimilation of crustal calc–silicate xenoliths has on the 2010 basaltic andesite magma. The pre-eruptive saturation of the basaltic andesite magma with aqueous carbonic NaCl–HCl-bearing fluid was suggested to be responsible for the high explosivity of the Merapi volcano in 2010. Both Nadeau et al. (2011; 2013a, b) and Troll et al. (2013) showed that glass inclusions in Merapi’s phenocrysts are typically CO2-rich. Alternatively, it was proposed that an unusually large batch of recharge magma could also have triggered the 2010 eruption (Costa et al., 2013; Jousset et al., 2013).
A mechanism of crustal assimilation has been suggested by Borisova et al. (2013): 1) contact metamorphism of Javanese sediments and formation of calc–silicate skarns like xenoliths in the 1998 and 2006 eruptions; 2) assimilation of the calc–silicate xenoliths through complete digestion, partial melting, or dissolution (partial melting involves reaction of a mineral to silicate melt, whereas mineral dissolution happens in the presence of silicate melt) and generation of calcic melts like those defined by Borisova et al. (2016) (referred to as the 2010 Crustal Assimilant); and 3) effective mixing between hydrous K-rich (resident) melt and the 2010 Crustal Assimilant (CaO up to 10.5 wt% and CaO/Al2O3 up to 1.2) melts that are characterized by crustal signatures, followed by crystallization. However, the kinetics (rates and mechanisms) of the assimilation mechanisms occurring in the Merapi pre-eruptive system have not been constrained yet. Mechanisms of xenolith dissolution and/or partial melting in the resident basaltic andesite magma may be recorded through detailed investigation of crustal calc–silicate xenoliths. Finally, Whitley et al. (2020) performed a detailed investigation of the Merapi calc–silicate xenolith production; however, no modeling of partial melting of potential calc–silicate wallrock has been applied. Our work here investigates mechanisms of the crustal assimilation affecting the Merapi basaltic andesite magmas based on detailed petrologic, mineralogical, and geochemical investigations coupled with rhyolite-MELTS thermodynamic modeling (Gualda et al., 2012; Ghiorso and Gualda, 2015). The results demonstrated in this work have implications for explosive volcanism related to crustal carbonate assimilation (e.g., volcanoes Merapi and Kelut, Indonesia).
Geological background
Merapi stratovolcano is located ∼30 km north of the city of Yogyakarta, Indonesia. The volcano is composed mainly of basaltic-andesite tephra, pyroclastic flows, lava, and lahar deposits. Since the 19th century, Merapi has erupted every 4–6 years, with most of these eruptions having explosivity indices ≤VEI 2 (Volcanic Explosivity Index), although moderate VEI 1–3 (1832, 1849, 1930, 1961, 2014, and 2018) and large VEI 4 (2010) eruptions have also occurred (https://volcano.si.edu/). The recent activity of Merapi is characterized by 1) recurrent effusive growth of viscous lava domes that collapse to form pyroclastic currents, referred to as the Merapi-type block-and-ash flows (BAFs) (VEI 2) and 2) more exceptional explosive eruptions of the sub-Plinian type (VEI 3–4) associated with columns collapsing into pumice-and-ash pyroclastic flows (Voight et al., 2000). The explosive eruption of 2010 (Costa et al., 2013) marks an important change in Merapi’s activity toward more explosive behavior. Today, Merapi has reverted to more typical behavior since, i.e., relatively abundant eruptions at VEI 1–3.
Merapi is located on the carbonate crust of 8–10 km thickness of the Kendeng basin, where Cretaceous to Cenozoic volcanoclastic sediments are overlain by shallow marine limestones, all of which overlie arc and ophiolite basement rocks (Smyth et al., 2005). Recent detailed micro-analytical and experimental data suggest the effects of shallow-level assimilation of the local Javanese sedimentary crust are significant (Chadwick et al., 2007; Deegan et al., 2010; Deegan et al., 2010; Borisova et al., 2013, 2016; Troll et al., 2013). Carbonate crust assimilation can play a key role in producing highly explosive fluid-saturated magmas (Iacono-Marziano et al., 2008, 2009; Deegan et al., 2010; Borisova et al., 2013; Troll et al., 2013). To evaluate the mechanisms and rates of crustal assimilation, we analyzed major, trace, and volatile elements and Sr–Nd–Pb isotopes in the Javanese crust and Merapi xenoliths and their minerals and glasses, using state-of-the-art analytical methods. Based on these data, we propose a mechanism of crustal assimilation preceding and triggering the Merapi eruptions.
Materials and methods
1994–2010 Merapi sample collection
The 1994, 1998, 2006, and 2010 xenolith sample list is shown in Table 1. M94-CS-104 and M94-CS-106 are from the 1994 small-volume Merapi-type BAFs. Samples MXCS-1, MXCS-2, MXCS-4, MXCS-7, and MXCS-9 are from the 1998 BAFs. MXCS-06-KAT2 is a calc–silicate xenolith from the 2006 eruption, and 014-1B is from the 2010 eruption. Samples of the 2010 Merapi xenoliths were collected during the 2015 French expedition (Borisova et al., 2016). LST-1, LST-2, LST-3, and LST-4 are carbonate sediment samples from Parangtritis beach (Java, Indonesia). The sampling locations are shown in the study of Deegan et al. (2010). More details on the samples can be found in the study of Chadwick (2008), Table 2, and Table 3.
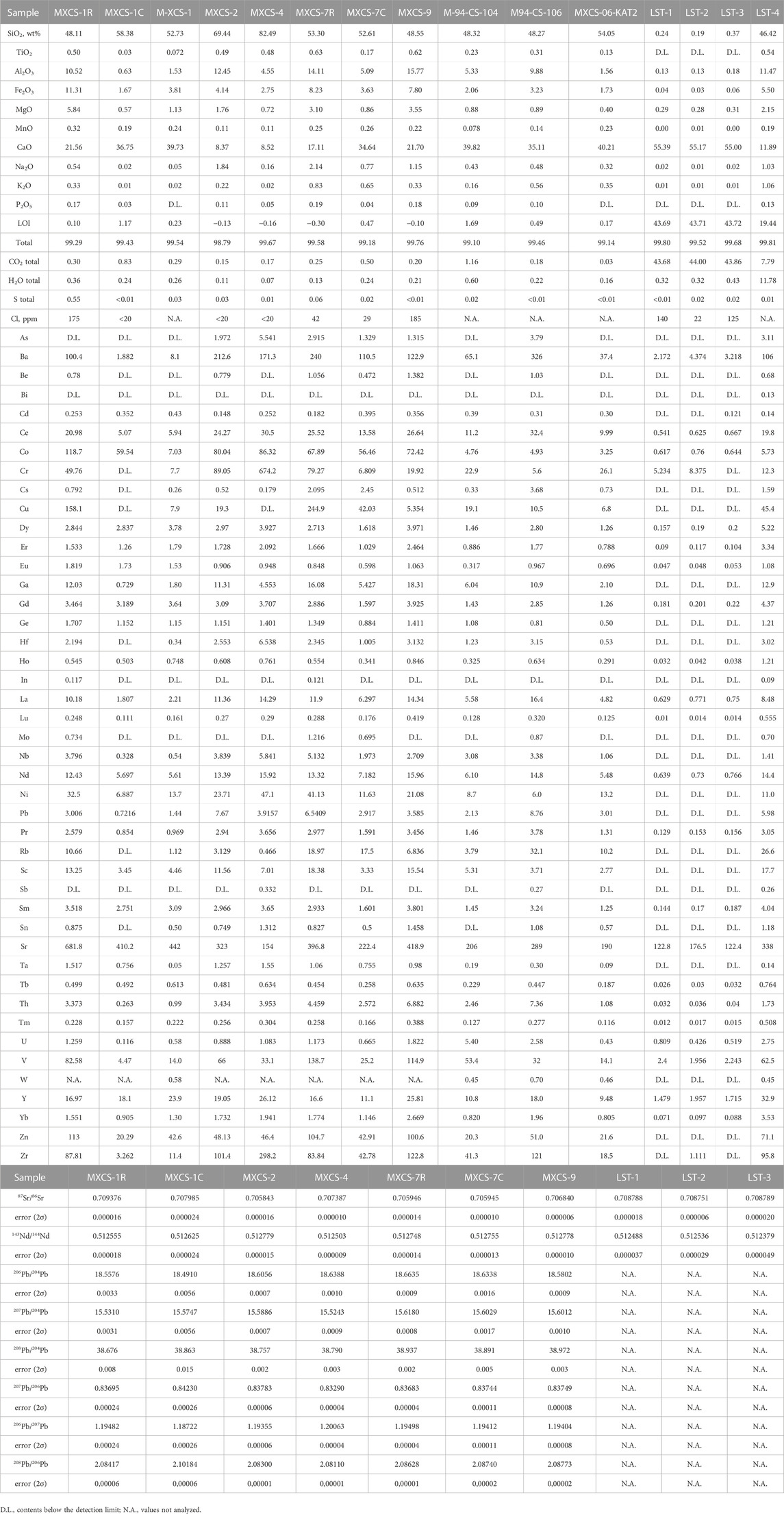
TABLE 2. Volatile, major, and trace element and isotopic composition of the bulk Merapi calc–silicate xenolith and Javanese limestone samples.
Volatile, major, and trace elements and Sr–Nd–Pb isotopic composition of the bulk xenolith and sediment samples
Volatile concentrations of CO2 and S were measured at SARM (Service d’Analyse des Roches et des Minéraux, Centre de Recherches Pétrographiques et Géochimiques, Vandoeuvre-ès-Nancy, France) using a Leco SC-144DR sulfur and carbon analyzer, by heating at 1400°C with oxygen flux and infrared (IR) detection. H2O concentrations were measured using Karl Fischer titration. Major and trace elements were measured by inductively coupled plasma optical emission spectroscopy (ICP–OES) and ICP–MS, using a method developed at SARM (Carignan et al., 2001) employing an ICP–OES IRIS Advantage ERS from Thermo Scientific and an ICP–MS x7 from Thermo Scientific without pre-concentration of rare earth elements (REEs) and U/Th.
For Sr–Nd–Pb isotope measurements at SARM, 100–200 mg of very fine rock powder (particle size <50 mm) was placed into a 15 mL Savillex beaker. A mixture of concentrated sub-boiled HNO3 (4 mL) and ultrapure HF (1 mL) was added to the powder samples. The solutions were kept at 115°C for 24–48 h, then evaporated, re-filled with concentrated HCl, and heated for 24 h to ensure complete digestion of the powder samples. The samples were then split into two parts and dried prior to purification of the elements.
For chromatographic separation of Pb from the matrix, 1 mL of HBr (0.8 M) was added to the aliquots. The chromatographic micro-column was filled with AG1X8 resin. The matrix was eluted with 0.8 M HBr, and Pb was recovered with 6 M HCl, following a method similar to that in the study of Manhès et al. (1980). Isotopic analysis was conducted using multicollector–inductively coupled plasma–mass spectrometry (MC–ICP–MS) (Micromass IsoProbe) using a Tl (NIST 997) spike to correct instrumental mass bias. Tl and Pb isotope values used for the NIST 981 and NIST 977 reference materials were those obtained by Thirlwall (2002). More details on the method are described by Cloquet et al. (2006).
For Sr and Nd isolation, the samples were diluted with 2 mL of 2 M HNO3, following the analytical protocol of Pin et al. (1994). An additional stage of further isolation of Nd from Sm was applied using Ln spec resin, following the procedure described by Pin et al. (1994). Strontium isotopes were measured using a thermal ionization mass spectrometer (TIMS; Triton Plus, from Thermo Electron) in the multicollection mode. In addition, five Faraday cups were used to monitor Rb. Sr was loaded onto a Re filament. Internal normalization using 86Sr/88Sr (0.1194) and the exponential law were used to correct the instrumental mass bias. NBS 987 was used to control methodological accuracy. The average blanks were negligible compared to the Sr concentration in the samples (average of 300 pg for Sr). Nd isotopes were measured using MC–ICP–MS (Micromass IsoProbe). To control instrumental mass bias, we applied a constant 146Nd/144Nd (0.72190) (Luais et al., 1997) and the exponential law. Reference JMC Nd was used to control methodological accuracy. The average blank corresponded to about 100 pg of Nd, which is negligible compared to the Nd concentration of the sample. Results of bulk rock Sr–Nd–Pb isotope analyses are summarized in Table 2 and Figure 1.
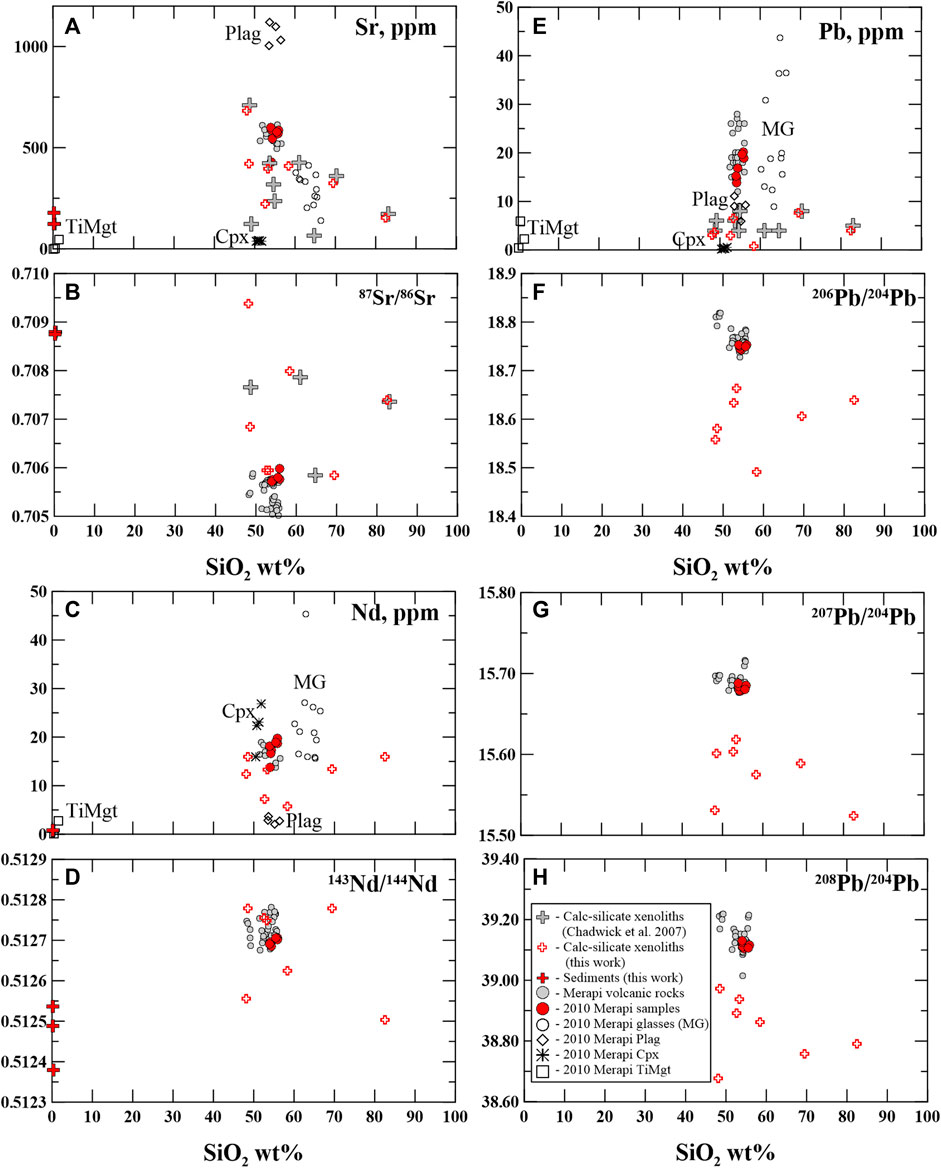
FIGURE 1. (A) Sr (ppm)–SiO2 (wt%), (B) 87Sr/86Sr–SiO2 (wt%), (C) Nd (ppm)–SiO2 (wt%), (D) 143Nd/144Nd–SiO2 (wt%), (E) Pb (ppm)–SiO2 (wt%), (F) 206Pb/204Pb–SiO2 (wt%), (G) 207Pb/204Pb–SiO2 (wt%), (H) 208Pb/204Pb–SiO2 (wt%) in the calc–silicate xenoliths and local Javanese carbonate sediments, the Merapi volcanic rocks, and the 2010 Merapi rocks and matrix glasses. The 2010 Merapi data are according to the study of Borisova et al. (2013), and the previous data on the calc–silicate xenoliths are from the study of Chadwick et al. (2007). Plag - plagioclase, Cpx- clinopyroxene, TiMgt - titanomagnetite. The Merapi volcanic rocks are from the MERAPIDATA database (Borisova et al., 2011).
Volatile, major, and minor element composition of the xenolith minerals and glasses
Major element analyses of minerals and glasses and backscattered electron images of the samples were performed at the Géosciences Environnement Toulouse (GET, Toulouse, France) laboratory and at the Centre de MicroCaractérisation Raimond Castaing (Toulouse, France). The main phases in the samples were identified using a scanning electron microscope (SEM) JEOL JSM-6360 LV coupled with energy-dispersive X-ray spectroscopy (EDS) (Borisova et al., 2013; Figure 2). The major, minor, and Cl contents of the crystals and glasses were analyzed using a CAMECA SX-Five microprobe. In general, beam conditions were 15 kV accelerating voltage and 10 or 20 nA probe current. For the analysis of glasses, the CAMECA SX-Five was used following the method of Borisova et al. (2010). The beam (15 kV and 10 nA) was set to a 10 μm beam diameter where possible. Sodium was analyzed first to ensure minimal loss during EPMA. These analytical conditions allowed an optimal signal for the major and minor elements, good internal precision, and minimal sodium loss during EPMA. The following synthetic and natural standards were used for calibration: albite (Na), corundum (Al), wollastonite (Si, Ca), sanidine (K), pyrophanite (Mn, Ti), hematite (Fe), periclase (Mg), and tugtupite (Cl). Element and background counting times for most analyzed elements were 10 s (except for 5 s for Na and K) and 5 s, respectively, whereas peak counting time was 120 s for Cl. The detection limit for Cl was 70 ppm. The silicate reference materials of MPI-DING covering ultramafic to felsic compositions (GOR132-G, GOR128-G, KL2-G, ML3B-G, and ATHO-G of the study of Jochum et al. (2006)) were analyzed as unknown samples to monitor analytical accuracy (Borisova et al., 2012, 2010). The estimated accuracy ranges from 0.5% to 3% (1σ RSD = relative standard deviation), depending on the element contents in the reference glasses. Additionally, the analysis of reference silicate material allowed us to estimate the analytical precision for the major and minor elements (e.g., Cl in glasses). The precision estimated in this way is compatible with the microprobe analytical uncertainty (derived from counting statistics).
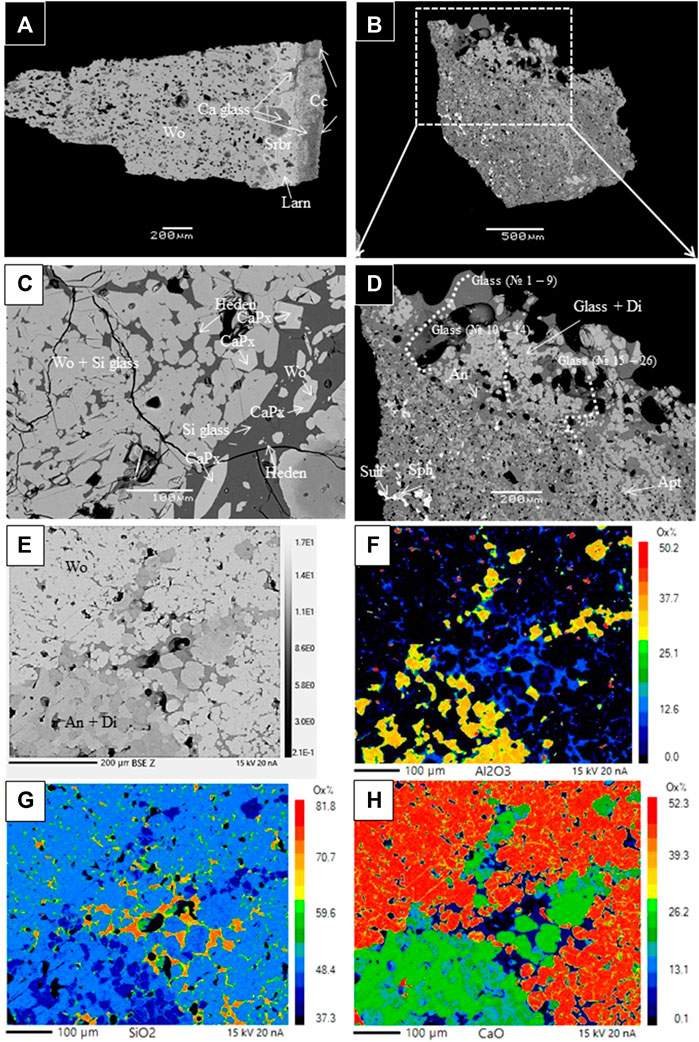
FIGURE 2. Representative backscattered electron images of the 1994–2006 Merapi calc–silicate xenoliths with mineral and glasses phases identified by EDS energy-dispersive X-ray spectroscopy and EPMA. Cc, calcite; Wo, wollastonite; Larn, larnite; Srbr, srebrodolskite; Ca glass, calcic glass; Si glass, silicic glass; Di, diopside, Heden, hedenbergite; An, anorthite; Sulf, sulfide; Sph, sphene; and Apt, apatite. (A) M94 CS104 (1994); (B, D) MXCS1 (1998); (C) MXC06KAT2 (2006); and (E–H) 014-1 B (2010).
Thermodynamic modeling by rhyolite-MELTS or Magma Chamber Simulator
Thermodynamic modeling using rhyolite-MELTS (Gualda et al., 2012; Ghiorso and Gualda, 2015) was performed to estimate the compositions of partial melts of representative calc–silicate crust. Rhyolite-MELTS is based on calibrations informed by experiments and constraints from natural silicic magma systems. For detailed model information, see the aforementioned references. Like all thermodynamic models, rhyolite-MELTS results must be carefully assessed to evaluate their applicability to natural systems. This includes comparing the resulting partial melts with compositions of observed products, such as the Si-rich glasses documented in this work.
In the thermodynamic modeling of the wallrock partial melting using rhyolite-MELTS (Gualda et al., 2012; Ghiorso and Gualda, 2015) or Magma Chamber Simulator MCS (Bohrson et al., 2014, 2020; Heinonen et al., 2020), we used two contrasting compositions (SiO2-rich and CaO-rich of the 1994–2010 calc–silicate xenoliths Figure 3) from the 1998–2006 Merapi collection (MXCS-1R; MXCS-4, Table 4) as potential crustal contaminants. The bulk compositions (Supplementary Dataset S1) of these two calc–silicate xenoliths underwent equilibrium crystallization (which is the equivalent of equilibrium melting) from the liquidus to the solidus or near solidus. Crystallization/melting occurred in five-degree increments. The resulting partial melts are shown in Figure 4, with the arrow showing the direction of increasing temperature (i.e., wallrock heating). Additional initial conditions include 200 MPa and Fe2O3/FeO ratio = 0.24, according to the results of studies of Costa et al. (2013) and Borisova et al. (2013; 2016).
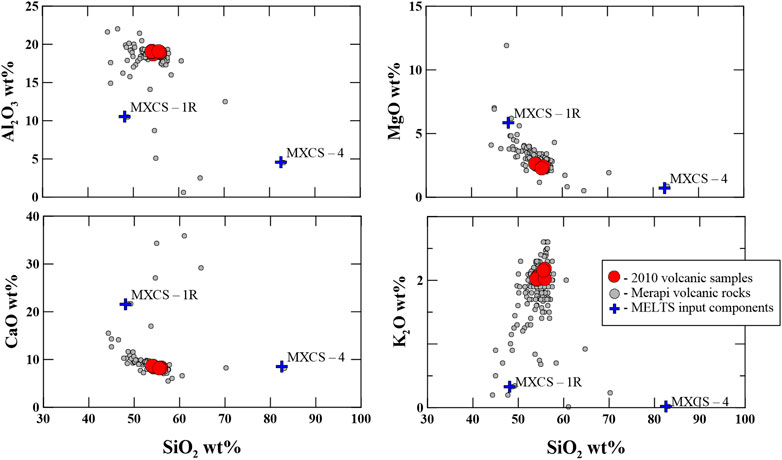
FIGURE 3. The 2010 and other Merapi volcanic rocks and the input component composition used for the thermodynamic modeling of the calc–silicate xenolith partial melting. All details of the thermodynamic modeling (MELTS) are summarized in Table 4 and Supplementary Dataset S1. The Merapi volcanic rocks are from the MERAPIDATA database (Borisova et al., 2011).
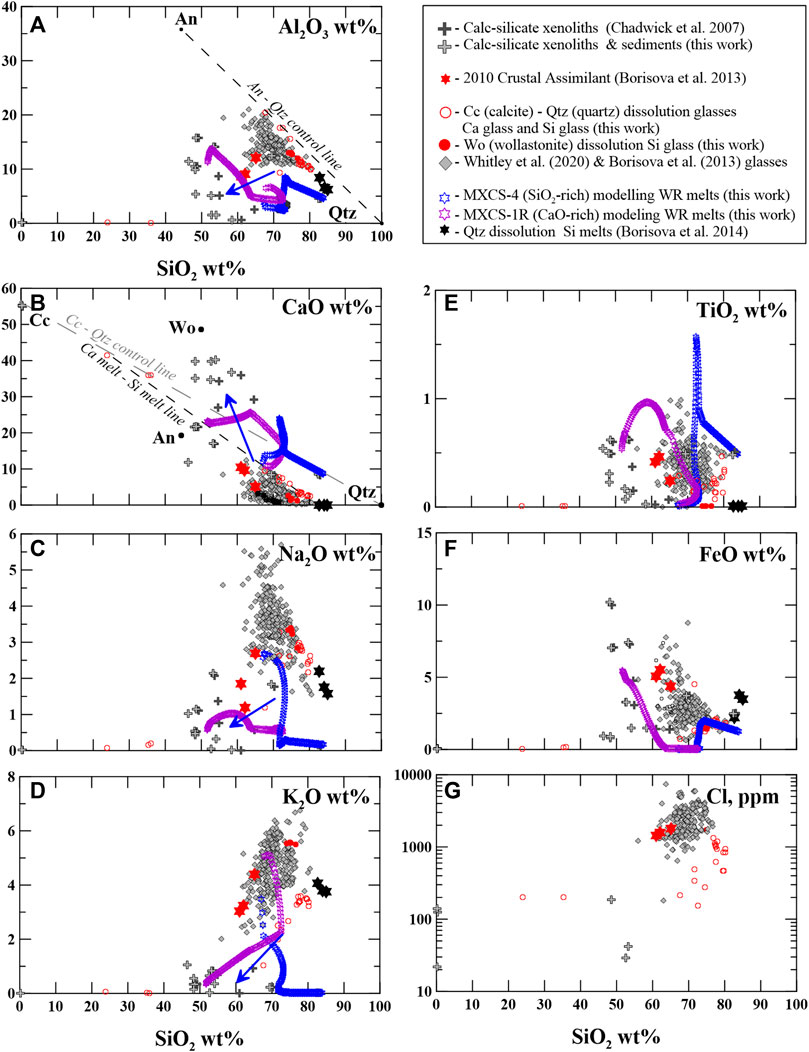
FIGURE 4. (A) Al2O3–SiO2, (B) CaO–SiO2, (C) Na2O–SiO2, (D) K2O–SiO2, (E) TiO2–SiO2, (F) FeO–SiO2, and (G) Cl (ppm–SiO2 (wt%) plotted for the bulk-rock calc–silicate xenoliths and local carbonate sediments, 2006 and 2010 glasses (groundmass), the 2010 Crustal Assimilant glasses compared to the Ca and Si glasses generated by dissolution of calcite, and quartz generated by Woll dissolution. Quartz dissolution glass compositions are from the study of Borisova et al. (2014). The 2006 data are from the study of Costa et al. (2013), and the 2010 data are from the study of Borisova et al. (2013). The MELTS (or MCS) modeling data (see Supplementary Dataset S1) are given for the wallrock melts. The blue arrows indicate the wallrock melt composition trajectories upon the xenolith heating and partial melting (WR). The blue arrows reflect the trends described by the 2010 Crustal Assimilant. An - anorthite; Qtz - quartz; Cc - calcite; Wo - wollastonite.
Results
Compositions of the calc–silicate xenoliths and Javanese sediment samples
Javanese carbonate sediments are strongly enriched in volatiles, mostly in CO2 (8–44 wt%) and H2O (0.3–11.8 wt%) contents, compared to calc–silicate xenoliths (0.03–1.2 wt% CO2total and 0.1–0.6 wt% H2Ototal contents). Both types of rock samples demonstrate similar low chlorine contents, 22–140 ppm for sediments and up to 185 ppm for xenoliths, whereas sulfur contents are extremely low in the sediments (up to 0.02 wt% S) and slightly more elevated in the xenoliths (up to 0.6 wt% S).
Among the major elements, carbonate sediment samples demonstrate an enrichment in calcium (11.9–55 wt% CaO), whereas these rocks are variably depleted in all other major elements: SiO2 (0.2–46.4 wt%), Al2O3 (0.1–12 wt%), MgO (0.3–2.2 wt%), and alkalis (up to 0.02–1.0 wt% Na2O). Compared to the sediment samples, the calc–silicate xenoliths have higher SiO2 (48–82 wt%, Figure 1); overlapping Al2O3 (0.6–16 wt%), Fe2O3 (1.7–11.3 wt%), MgO (0.4–5.8 wt%), alkalis (0.05–2.1 wt% Na2O and 0.02–0.8 wt% K2O), TiO2 (0.03–0.6 wt%), and P2O5 (up to 0.2 wt%); and lower CO2 (up to 1.2 wt%) contents. High SiO2 and Al2O3 contents in the xenoliths (MXCS-4: up to 82 wt% SiO2 and up to 12.45 wt% Al2O3) reflect strong enrichment in silicates due to skarn formation (Burt, 1982).
Javanese sediment samples have higher strontium (122–338 ppm) concentrations compared to other trace elements (2.0–63 ppm V) in these samples. The calc–silicate xenoliths are variably enriched in such trace elements as La (1.8–16.4 ppm), Ce (5–32.4 ppm), Co (up to 119 ppm), Cr (up to 674 ppm), Cu (up to 245 ppm), Ba (up to 326 ppm), Sr (154–682 ppm), Zn (20–113 ppm), and Zr (up to 298 ppm) compared to the sediments.
Figure 1 illustrates that sediments have radiogenic strontium isotope signatures (87Sr/86Sr = 0.70684–0.70879) and low 143Nd/144Nd isotope ratios (0.51238–0.51254). The xenolith samples are represented by extremely variable Sr isotope ratios (0.70595–0.70938), whereas the Nd isotope ratio range is more modest, from 0.51250 to 0.51278. Pb isotope ratios are variable: 206Pb/204Pb (18.491–18.664), 207Pb/204Pb (15.524–15.618), and 208Pb/204Pb (38.676–38.972).
Micro-textures, minerals, and glasses in the 1994–2010 calc–silicate xenolith samples
1994 xenolith M94-CS-104 (core)
Figure 2A demonstrates that the investigated sample fragment contains one predominant mineral phase: porous wollastonite. It is important to note that the sample also contains accessory calcite with ≤0.1 wt% MnO, larnite (Larn, Ca2SiO4), and srebrodolskite (Srbr, Ca2Fe3+2O5) (Table 3) in the localized zone of the xenolith edge. In the contact zone between the calcite and wollastonite, the sample contains micro-veins and micro-agglomerates (Figure 2A) of porous calcic glasses (31.7 ± 6.8 wt% SiO2; 37.8 ± 3.1 wt% CaO).
1998 xenolith MXCS1 (rim)
Figures 2B, D represent the edge zone of the xenolith fragment in contact with the lava. This contact zone contains idiomorphic diopside crystals (Table 3) in association with highly silicic glasses (76.5 ± 3.5 wt% SiO2) with variable low CaO (4.3 ± 2.2 wt%), low alkalis (2.6 ± 0.4 wt% Na2O and 3.1 ± 0.7 wt% K2O), and low MgO (up to 1.3 wt%), FeO (1.8 ± 0.8 wt%), and TiO2 (0.2 ± 0.1 wt%) contents. These natural silicic glasses are richer in SiO2 and poorer in Cl contents compared to the 2010 Crustal Assimilant and the groundmass 2006 and 2010 Merapi glasses (Figure 4). The notation of the 2010 Crustal Assimilant (natural high-Ca glasses in the 2010 Merapi pyroclastic lava products) has been introduced to describe a model assimilant composition that interacted with the 2010 Merapi pre-eruptive magma based on the work of Borisova et al. (2013), whereas these natural melts have compositions close to those of the partial melts modeled by MELTS. The natural silicic glasses highly enriched in SiO2 resemble not only those generated by MELTS during the calc–silicate wallrock partial melting (Supplementary Dataset S1) but also those generated by quartz dissolution in the 1991 Pinatubo basaltic xenoliths (Borisova et al., 2014).
1998 xenolith MXCS4 (core)
The investigated xenolith core contains an assemblage of re-crystallized coarse quartz, anorthite, clinopyroxene, and titanomagnetite identified by using a scanning electron microscope (EDS). The anorthite grains are polyhedral with ∼120 dihedral angles or porphyroblastic with abundant inclusions (i.e., typical metamorphic textures). Glass was not detected.
2006 xenolith MXCS06KAT2 (rim)
Another xenolith–lava contact zone is represented by the Merapi calc–silicate xenolith from the 2006 BAF (Figure 2C). It is noticed that the contact zone contains xenomorphic crystals of wollastonite (Wo83-97En0-3) with up to 0.3 wt% of Al2O3 in the groundmass and clusters of euhedral–subhedral individual crystals in highly silicic glass (SiO2 = 75.2 ± 1.1 wt%, Al2O3 = 12.7 ± 0.5 wt%, CaO = 1.7 ± 0.6 wt%, Figure 4). Supplementary Figures A1, A2 demonstrate idiomorphic rims of strongly zoned calcic pyroxenes (CaPx, Wo54-82 En2-21) with compositions (Al2O3 0.3–2.0 wt%, Mg# = 13–47) intermediate between hedenbergite and wollastonite at the rims. Moreover, at the xenolith–lava contact, the pyroxene cores are Al-poor augite (Wo43 En42, Al2O3 = 2.3 wt%) and diopside (Wo46 En46, Al2O3 = 2.1 wt%) that have high Mg# = 74–76.
2010 xenolith 014-1 B (rim)
Figures 2E–H show that the investigated 2010 Merapi xenolith contains wollastonite associated with silicic glasses and an assemblage of re-crystallized anorthite and diopside clinopyroxene as well as titanomagnetite identified by electron microprobe mapping (EPMA). The anorthite grains are polyhedral with ∼120 dihedral angles or porphyroblastic (i.e., typical metamorphic textures). The presence of silicic glass, detected in an assemblage with massive wollastonite at the rim of the calc–silicate xenolith, suggests production of silica-rich glasses at contact zones between the xenolith and magma.
Partial melt compositions predicted by rhyolite-MELTS modeling
Equilibrium partial melting results using rhyolite-MELTS (version 1.2.0) for two contrasting compositions (SiO2-rich and CaO-rich, Supplementary Dataset S1 and Table 4) of the calc–silicate xenoliths as the wallrocks from the 1998–2010 Merapi collection (MXCS-1R; MXCS-4, Table 2) are presented in Figure 3 (starting composition) and Figure 4 (modeled partial melt compositions). The SiO2-rich calc–silicate xenolith, MXCS-4, has a solidus assemblage (∼625°C at 200 MPa) of quartz >> garnet >> clinopyroxene > minor feldspar, sphene, and apatite. At its solidus, the assemblage is H2O-fluid saturated. During partial melting, melts range from ∼67 wt% SiO2 and 12 wt% CaO [at smaller degrees of melt (<1%)] to ∼84% SiO2 at the liquidus. Enriched CaO (>20 wt%) is produced during equilibrium partial melting between ∼790°C (∼3.5% partial melt) to ∼955°C (25% partial melt). Minerals are consumed in the following order: alkali feldspar (∼Or80) at ∼630°C, the fluid phase at ∼650°C, and apatite and garnet between ∼815°C and 845°C, followed by sphene (∼950°C), plagioclase (∼1055°C), and clinopyroxene (∼1100°C). Quartz remains the only solid phase above this temperature to the liquidus. See Figure 4 and Supplementary Dataset S1 for more details.
The CaO-rich xenolith, MXCS-1R, is at ∼5% melt at ∼656°C, where the rhyolite-MELTS run encountered a quadratic error. The conditions of partial melting are well represented by the rhyolite-MELTS results, despite this. The near-solidus assemblage includes clinopyroxene >> garnet > plagioclase > quartz > minor sphene, alkali feldspar, and apatite. This xenolith is not fluid saturated at any point at 200 MPa. During partial melting, SiO2 (wt%) values initiate at ∼67.9 (∼656°C), increase to a maximum of 72.6 (∼766°C), and then, decrease to ∼51.7 at the liquidus. CaO (wt%) starts at ∼11 at 656°C, increases to a maximum of 25.6 at 886°C (∼21% melting), and then, decreases to ∼23 wt% at the liquidus. Minerals are consumed in the following order: alkali feldspar (∼666°C), quartz (∼766°C), sphene and garnet between 856°C and 881°C, apatite (∼906°C), and plagioclase (1201°C). Clinopyroxene remains the only phase present to the liquidus. See Figure 4 and Supplementary Dataset S1 for more details.
Discussion
Reaction to produce the calc–silicate crust
Figure 2A demonstrates that the investigated sample fragment, 1994 xenolith M94-CS-104, contains one predominant mineral phase: porous wollastonite formed by regular skarn reaction:
where CaCO3S is a calcite molecule, SiO2F is SiO2 dissolved in a (an aqueous) fluid (F), CaSiO3S is the formed wollastonite mineral, and CO2F is the fluid liberated upon reaction. This reaction, also summarized in Figure 5, demonstrates an important flux of silica by shallow aqueous fluid to produce the calc–silicate crust that is enriched in silica relative to the sediments.
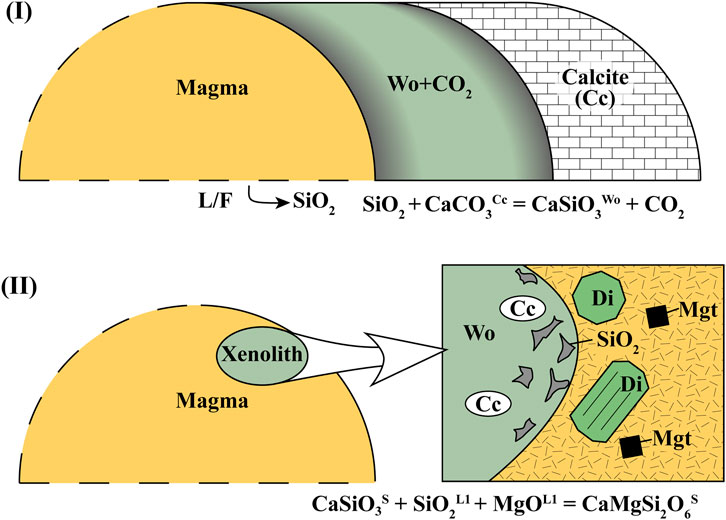
FIGURE 5. Principal schema of (I) the calc–silicate crust production and (II) calc–silicate crust assimilation by the Merapi magma (Merapi volcano, Indonesia). The elevated trace element (Ba, Co, Cr, Cu, V, Zn, and Zr) contents in the calc–silicate crust and the contaminated magma are related to the important flux of these elements from the magmatic source (in form of an aqueous-carbonic fluid) at stage I. Cc, calcite; Wo, wollastonite; Di, diopside; SiO2, silicic glasses; and Mgt, magnetite. Our hypothesis is that the rate-limiting process is the mixing of the produced crustal melts with andesitic melt (average 65 wt% SiO2) through chemical diffusion of Si–Al in hybrid aluminosilicate melts.
Geochemical and mineral tracers of calc–silicate xenolith assimilation
Figure 1 shows that Sr is a unique isotopic tracer of calc–silicate assimilation, whereas Pb isotopes are depleted in the calc–silicate xenoliths compared to the Merapi volcanic rocks. Nd behaves differently depending on the type of the crustal material. As to Sr–Pb isotope signatures, radiogenic Sr and unradiogenic Pb are characteristic features of the Merapi calc–silicate xenoliths (Figure 1). Table 2 also demonstrates that in addition to Sr (up to 682 ppm), trace elements such as Ba (up to 326 ppm), Co (up to 119 ppm), Cr (up to 674 ppm), Cu (up to 245 ppm), V (up to 139 ppm), Zn (up to 105 ppm), and Zr (up to 298 ppm) may be considered as geochemical tracers of calc–silicate crust assimilation. Moderate to strong enrichment of the calc–silicate xenoliths in the trace elements compared to the sediments is likely to be related to aqueous-carbonic fluid reaction at contact metamorphic conditions (Figure 1).
In addition to high Sr contents and radiogenic 87Sr/86Sr, elevated CO2, Ba, Co, Cr, Cu, V, Zn, and Zr contents in the magmatic minerals and associated glasses and re-crystallization/dissolution of wallrock sphene, quartz, garnet, apatite, and ilmenite are predicted by thermodynamic modeling (upon the wallrock melting) and are, thus, important tracers of calc–silicate crust assimilation.
Kinetics of reaction between calc–silicate crust and the Merapi magma
The 1994–2010 Merapi calc–silicate xenoliths are enriched in wollastonite in the core zones and frequently contain calcite as a relic mineral phase (Figure 2A; Deegan et al., 2010). In contrast, the xenoliths’ rims are depleted in wollastonite and are commonly enriched in anorthite and diopside. The xenolith samples also contain veins and spherical agglomerates of calcic glasses (31.7 ± 6.8 wt% SiO2, 37.8 ± 3.1 wt% CaO) (Table 3). This type of natural calcic glass strongly resembles “Ca-rich glasses” (28–38 wt% SiO2, 27–34 wt% CaO) experimentally produced by Jolis et al. (2013), Deegan et al. (2010), and Blythe et al. (2015) from calcite-enriched material heated with silicate melt at 500 MPa and 1200°C. Moreover, Deegan et al. (2010) reported even more Ca-rich glasses (containing up to 62 wt% CaO) associated with natural wollastonite–calcite in the Merapi xenoliths. We interpret the natural calcic glasses reported in Table 3 to be the result of the reaction between calcite CaCO3S and SiO2L/F (see Eq. 2; Blythe et al., 2015) rather than the “dissociation” proposed by Deegan et al. (2010). Figure 2A demonstrates the occurrence of calcic glasses in the M94 CS104 xenolith in an assemblage with larnite and wollastonite, whereas the major element composition of the calcic glasses coincides with a calcite–quartz control line, suggesting that the composition of the calcic melt is controlled by the following dissolution reaction:
where SiO2L/F (silicate melt (L) or fluid (F) form of SiO2) is derived from the magma, F is fluid, and L is the produced Ca–Si-melt.
In the xenolith rim of MXCS1, the observed assemblage of euhedral–subhedral individual crystals of diopside with highly silicic glasses (76.5 ± 3.5 wt% SiO2, 12.3 ± 3.2 wt% Al2O3, 4.3 ± 2.2 wt% CaO) in the 1998 calc–silicate xenoliths (Table 3; Figure 2B) resembles an association of idiomorphic clinopyroxene rims with silicic glasses around quartz xenocrysts produced by dissolution of quartz phenocrysts during the reaction with basaltic magma (Donaldson, 1986; Borisova et al., 2014). We infer, therefore, that the assemblage of silicic glasses and euhedral–subhedral individual crystals of diopside is the result of incongruent dissolution of metamorphic minerals associated with crystallization of calcic clinopyroxenes in the produced highly silicic melts. The incongruent dissolution reaction may be described as follows:
where CaSiO3S is residual wollastonite, SiO2L1 is SiO2 in the initial melt or solid SiO2S quartz, MgOL1 is MgO components in the initial melt (L1), and CaMgSi2O6S is diopside in the produced highly silicic melt. The mechanism of the crustal assimilation may be first marked by incongruent dissolution of crustal metamorphic minerals with generation of highly silicic (77 ± 4 wt% of SiO2, 12 ± 3 wt% of Al2O3) melts in association with idiomorphic diopside [Wo49En29; 57 ± 3 Mg# = Mg/(Mg+Fe2+)] or other calcic pyroxenes (Wo54-82 En2-21; 16–43 Mg#).
The highly silicic glasses (melts) may be directly predicted by thermodynamic equilibrium or modeling of partial melting of the calc–silicate xenoliths. The thermodynamic modeling results record silicic melt compositions with the highest SiO2 = 67–84 wt% and variable CaO = 12–26 wt% contents upon partial melting of the crustal xenoliths due to quartz, garnet, and feldspar consumption. Figure 4 demonstrates that all oxide contents in the modeled highly silicic melts produced at thermodynamic equilibrium during partial melting of calc–silicate xenoliths (at 630°C–1200°C) closely resemble those of the analyzed silicic glasses in the 1994–2010 xenoliths.
Consequently, SiO2, Al2O3, and CaO contents in the generated melts may be controlled by chemical mixing between the silicic melts and generated highly mobile carbonate (Ca-rich) melt. The capacity of carbonate melt rich in CO3 to be extremely mobile and to migrate from skarns to a magma chamber (Gaeta et al., 2009) indicates that CaO-SiO2-Al2O3 contents in the analyzed silicic glasses (Figure 4) can be modeled by mixing between the highly silicic and carbonate melts. In order to explain Al2O3 and other major element contents in the assimilating melts (e.g., the 2010 Crustal Assimilant), it is necessary to suggest involvement and chemical mixing with K–Cl-rich andesitic melts (average 65 wt% SiO2), proposed by Borisova et al. (2013; 2016) as a natural melt component of the resident magma. We hypothesize that the rate limiting process of the crustal assimilation is the mixing of the produced crustal melts with the andesitic to basaltic andesite melts through Si–Al diffusion that explains major and volatile (Cl) element contents in the Merapi glass products.
Physical–chemical disequilibrium versus thermodynamic modeling
We predict that because of rapid heat diffusion, the calc–silicate material has to reach the temperature of 900°C rapidly upon the magma–xenolith interaction, suggesting effective partial melting and dissolution of the crustal xenoliths at 900°C. Thermal stability of carbonates is limited to a temperature range of 820°C–870°C (Ar and Doǧu, 2001). Calcite starts to decompose at 620°C, and the calcination reaction is completed at 820°C at atmospheric pressure (Stern & Weise, 1966; Ar and Doǧu, 2001), suggesting complete consumption of calcite at the pre-eruptive temperatures of 925°C–1100°C. Given the chemical equilibrium during contact metamorphism created by heat flow from basaltic andesite magma, the xenolith (several cm in size) should attain the pre-eruptive temperature of 925°C–1100°C at 200–300 MPa. Nevertheless, the calcite inclusions (Deegan et al., 2010; Borisova et al., 2013) and calcite–larnite–wollastonite assemblage (Figure 2) are observed in the Merapi calc–silicate xenoliths as metastable mineral phases.
These observations suggest that the reaction between the magma and the 1994–2010 calc–silicate xenoliths was out of physical–chemical (or thermodynamic) equilibrium. The disequilibrium may be related to sluggish kinetics of the residual calcite consumption and Si–Al diffusion in the hybrid melt. In any case, it is likely that the crust–magma interaction is controlled by kinetics of reactions such as crustal partial melting, mineral dissolution and recrystallization, and melt mixing. Nevertheless, the mechanism of crustal calc–silicate material assimilation can be well predicted by thermodynamic modeling using rhyolite-MELTS, whereas more experiments are required to constrain the rate of the calc–silicate material-silicate magma interactions. Based on available experimental data (Zhang et al., 1989; Shaw, 2006), we predict that this crustal assimilation rate is controlled by Si–Al diffusion in the hydrous silicic melts produced during the melt–crust interaction. These experimental data suggest that silica activity in the melt defines the maximal dissolution rate, whereas the melt viscosity determines whether this maximum rate may be achieved in the case of diffusion control.
Conclusion
1) In situ electron probe microanalyses of the 1994–2010 calc–silicate xenolith minerals and glasses and the observed reactions and thermodynamic modeling using MELTS or MCS suggest that selective assimilation at ∼ 650°C–1100°C and 200–300 MPa prior to the 1994–2010 eruptions preserved wollastonite and carbonate melts quenched to natural calcic glasses (32 ± 7 wt% SiO2, 38 ± 3 wt% CaO) as well as minor calcite mineral as a thermodynamically metastable mineral phases in the calc–silicate xenoliths. The following reaction happened during the 1994–2010 calc–silicate xenolith interaction with the Merapi magma:
CaSiO3S + SiO2L1/S + MgOL1 = CaMgSi2O6S, where CaSiO3S is residual metastable wollastonite, SiO2L1/S are SiO2 and MgO in the initial melt (L1) or quartz (S), MgOL1 is MgO in the initial melt (L1), and CaMgSi2O6S is diopside in the produced highly silicic melt.
2) The mechanism of the 1994–2010 calc–silicate xenolith assimilation is generation of highly silicic (77 ± 4 wt% of SiO2, 12 ± 3 wt% of Al2O3) melts in association with idiomorphic diopside [Wo49En29; 57 ± 3 Mg# = Mg/(Mg+Fe2+)] or other calcic pyroxenes (Wo54-82 En2-21; 16–43 Mg#) due to mineral dissolution and recrystallization reactions. Modeling using rhyolite-MELTS predicts that the major element composition of the generated silicic glasses (up to 67–84 wt% SiO2) is controlled by the calc–silicate material partial melting.
3) In addition to elevated Sr contents and radiogenic 87Sr/86Sr ratio, important geochemical tracers of the calc–silicate assimilation are elevated CO2, Ba, Co, Cr, Cu, V, Zn, and Zr contents in the minerals and glasses. The re-crystallization/dissolution of wallrock-derived sphene, quartz, garnet, apatite, and ilmenite is predicted by thermodynamic modeling (upon the wallrock partial melting) and, thus, are important mineral tracers of crustal assimilation.
4) Although kinetics of the calc–silicate material–melt interaction likely control the calc–silicate assimilation process, the mechanism of the assimilation process can be well predicted by thermodynamic modeling (MELTS or MCS). Further application of kinetic experiments on the calc–silicate material interaction with aluminosilicate magma is useful to quantify the rates of crustal assimilation. We hypothesize that the rate-limiting process of crustal assimilation is chemical mixing of the produced crustal melts through the chemical diffusion of Si–Al in hybrid melts associated with convection.
Data availability statement
The original contributions presented in the study are included in the article/Supplementary Material; further inquiries can be directed to the corresponding author.
Author contributions
All authors listed have made a substantial, direct, and intellectual contribution to the work and approved it for publication.
Funding
This study was supported by the VELI national site “Volcans Explosifs Laboratoire Indonesien” and the mixed laboratory “Indonesian Subduction and associated risks” or “Subductions Indonésiennes et Risques associés” (SIR) in France and US National Science Foundation projects 1551052 and 2151038.
Acknowledgments
The authors thank the editor Patrizia Fiannacca, S. Erdmann, and another reviewer for the important suggestions on this work, Valentin R. Troll and Frances Deegan for providing the 1998—2006 Merapi xenolith and sediment samples, and Philippe de Parseval for electron probe mapping of the 2010 Merapi sample. The 2015 French expedition provided the 2006–2010 Merapi products for this work.
Conflict of interest
The authors declare that the research was conducted in the absence of any commercial or financial relationships that could be construed as a potential conflict of interest.
Publisher’s note
All claims expressed in this article are solely those of the authors and do not necessarily represent those of their affiliated organizations, or those of the publisher, the editors, and the reviewers. Any product that may be evaluated in this article, or claim that may be made by its manufacturer, is not guaranteed or endorsed by the publisher.
Supplementary material
The Supplementary Material for this article can be found online at: https://www.frontiersin.org/articles/10.3389/feart.2023.1186207/full#supplementary-material
References
Ar, I., and Doǧu, G. (2001). Calcination kinetics of high purity limestones. Chem. Engeneering J. 83, 131–137. doi:10.1016/s1385-8947(00)00258-8
Blythe, L. S., Deegan, F. M., Freda, C., Jolis, E. M., Masotta, M., Misiti, V., et al. (2015). CO2 bubble generation and migration during magma – carbonate interaction. Contrib. Mineral. Petrol. 169, 42. doi:10.1007/s00410-015-1137-4
Bohrson, W. A., Spera, F. J., Ghiorso, M. S., Brown, G. A., Creamer, J., and Mayfield, A. (2014). Thermodynamic model for energy-constrained open-system evolution of crustal magma bodies undergoing simultaneous recharge, assimilation and crystallization: the magma chamber simulator. J. Petrology 55, 1685–1717. doi:10.1093/petrology/egu036
Bohrson, W. A., Spera, F. J., Heinonen, J. S., Brown, G. A., Scruggs, M. A., Adams, J. V., et al. (2020). Diagnosing open-system magmatic processes using the magma chamber simulator (MCS): part I—major elements and phase equilibria. Contributions Mineralogy Petrology 175, 104–129. doi:10.1007/s00410-020-01722-z
Borisova, A. Y., Gurenko, A. A., Martel, C., Kouzmanov, K., Cathala, A., Bohrson, W. A., et al. (2016). Oxygen isotope heterogeneity of arc magma recorded in plagioclase from the 2010 Merapi eruption (Central Java, Indonesia). Geochimica Cosmochimica Acta 190, 13–34. doi:10.1016/j.gca.2016.06.020
Borisova, A. Y., Martel, C., Gouy, S., Pratomo, I., Sumarti, S., Toutain, J-P., et al. (2013). Highly explosive 2010 Merapi eruption: evidence for shallow-level crustal assimilation and hybrid fluid. J. Volcanol. Geotherm. Res. 261, 193–208. doi:10.1016/j.jvolgeores.2012.11.002
Borisova, A. Y., Martel, C., Pratomo, I., Toutain, J., Sumarti, S., and Surono, S. “Merapidata: new petrologic and geochemical database of the Merapi volcano, central java, Indonesia,” in Proceedings of the AGU Fall Meeting Abstracts, San Francisco, California, December 2011, V21E–V2536.
Borisova, A. Y., Pichavant, M., Bény, J.-M., Rouer, O., and Pronost, J. (2005). Constraints on dacite magma degassing and regime of the June 15, 1991, climactic eruption of Mount Pinatubo (Philippines): new data on melt and crystal inclusions in quartz. J. Volcanol. Geotherm. Res. 145 (1-2), 35–67. doi:10.1016/j.jvolgeores.2005.01.004
Borisova, A. Y., Pokrovski, G. S., Pichavant, M., Fréydier, R., and Candaudap, F. (2010). Amorphous Materials: properties, Structure, and Durability: arsenic enrichment in hydrous peraluminous melts: insights from femtosecond laser ablation-inductively coupled plasma-quadrupole mass spectrometry, and in situ X-ray absorption fine structure spectroscopy. Am. Mineralogist 95, 1095–1104. doi:10.2138/am.2010.3424
Borisova, A. Y., Toutain, J-P., Dubessy, J., Pallister, J., Zwick, A., and Salvi, S. (2014). H2O–CO2–S fluid triggering the 1991 Mount Pinatubo climactic eruption (Philippines). Bull. Volcanol. 76, 800. doi:10.1007/s00445-014-0800-3
Borisova, A. Y., Toutain, J-P., Stefansson, A., Gouy, S., and de Parseval, Ph. (2012). Processes controlling the 2010 Eyjafjallajökull explosive eruption. J. Geophys. Res. Solid Earth 117, B5. doi:10.1029/2012JB009213
Burt, D. M. (1982). Skarn deposits; historical bibliography through 1970. Econ. Geol. 77 (4), 755–763. doi:10.2113/gsecongeo.77.4.755
Carignan, J., Hild, P., Mevelle, G., Morel, J., and Yeghicheyan, D. (2001). Routine analyses of trace elements in geological samples using flow injection and low pressure on-line liquid chromatography coupled to icpms: A study of geochemical reference materials br, DR-N, UB-N, AN-G and gh. Geostand. Geoanal. Res. 25, 187–198. doi:10.1111/j.1751-908X.2001.tb00595.x
Chadwick, J. P. (2008). “Crustal processes in volcanic systems: case studies from northern Ireland, New Zealand and Indonesia,”. Ph.D. Thesis (Dublin, Ireland: University of Dublin), 274.
Chadwick, J. P., Troll, V. R., Ginibre, C., Morgan, D., Gertisser, R., Waight, T. E., et al. (2007). Carbonate assimilation at Merapi volcano, java, Indonesia: insights from crystal isotope stratigraphy. J. Petrology 48, 1793–1812. doi:10.1093/petrology/egm038
Cloquet, C., Carignan, J., and Libourel, G. (2006). Atmospheric pollutant dispersion around an urban area using trace metal concentrations and Pb isotopic compositions in epiphytic lichens. Atmos. Environ. 40, 574–587. doi:10.1016/j.atmosenv.2005.09.073
Costa, F., Andreastuti, S., Bouvet de Maisonneuve, C., and Pallister, J. (2013). Petrological insights into the storage conditions, and magmatic processes that yielded the centennial 2010 Merapi explosive eruption. J. Volcanol. Geotherm. Res. 261, 209–235. doi:10.1016/j.jvolgeores.2012.12.025
Deegan, F. M., Troll, V. R., Freda, C., Misiti, V., Chadwick, J. P., McLeod, C. L., et al. (2010). Magma–carbonate interaction processes and associated CO2 release atMerapi volcano, Indonesia: insights from experimental petrology. J. Petrology 51, 1027–1051. doi:10.1093/petrology/egq010
Deegan, F. M., Troll, V. R., Gertisser, R., and Freda, C. (2023). “Magma-carbonate interaction at Merapi volcano, Indonesia,” in Merapi Volcano. Active volcanoes of the world. Editors R. Gertisser, V. R. Troll, T. R. Walter, I. G. M. A. Nandaka, and A. Ratdomopurbo (Cham, Germany: Springer). doi:10.1007/978-3-031-15040-1_10
Erdmann, S., Martel, C., Pichavant, M., Bourdier, J. L., Champallier, R., Komorowski, J. C., et al. (2016). Constraints from phase equilibrium experiments on pre-eruptive storage conditions in mixed magma systems: A case study on crystal-rich basaltic andesites from mount Merapi, Indonesia. J. Petrology 57 (3), 535–560. doi:10.1093/petrology/egw019
Erdmann, S., Martel, C., Pichavant, M., and Kushnir, A. (2014). Amphibole as an archivist of magmatic crystallization conditions: problems, potential, and implications for inferring magma storage prior to the paroxysmal 2010 eruption of mount Merapi, Indonesia. Contributions Mineralogy Petrology 167, 1016–1023. doi:10.1007/s00410-014-1016-4
Freda, C., Gaeta, M., Misiti, V., Mollo, S., Dolfi, D., and Scarlato, P. (2008). Magma–carbonate interaction: an experimental study on ultrapotassic rocks from alban hills (central Italy). Lithos 101, 397–415. doi:10.1016/j.lithos.2007.08.008
Gaeta, M., Di Rocco, T., and Freda, C. (2009). Carbonate assimilation in open magmatic systems: the role of melt-bearing skarns and cumulate-forming processes. J. Petrology 50, 361–385. doi:10.1093/petrology/egp002
Ghiorso, M. S., and Gualda, G. A. R. (2015). An H2O-CO2 mixed fluid saturation model compatible with rhyolite-MELTS. Contributions Mineralogy Petrology 169, 53. doi:10.1007/s00410-015-1141-8
Gualda, G. A., Ghiorso, M. S., Lemons, R. V., and Carley, T. L. (2012). Rhyolite-MELTS: A modified calibration of MELTS optimized for silica-rich, fluid-bearing magmatic systems. J. Petrology 53 (5), 875–890. doi:10.1093/petrology/egr080
Heinonen, J. S., Bohrson, W. A., Spera, F. J., Brown, G. A., Scruggs, M. A., and Adams, J. V. (2020). Diagnosing open-system magmatic processes using the magma chamber simulator (MCS): part II—trace elements and isotopes. Contributions Mineralogy Petrology 175, 105–121. doi:10.1007/s00410-020-01718-9
Iacono-Marziano, G., Gaillard, F., and Pichavant, M. (2008). Limestone assimilation by basaltic magmas: an experimental re-assessment and application to Italian volcanoes. Contributions Mineralogy Petrology 155, 719–738. doi:10.1007/s00410-007-0267-8
Iacono-Marziano, G., Gaillard, F., Scaillet, B., Pichavant, M., and Chiodini, G. (2009). Role of non-mantle CO2 in the dynamics of volcano degassing: the mount vesuvius example. Geology 37, 319–322. doi:10.1130/g25446a.1
Jarosevich, E., Nelen, J. A., and Norberg, J. A. (1980). Reference samples for electron microprobe analysis. Geostand. Newsletters 4, 43–47. doi:10.1111/j.1751-908x.1980.tb00273.x
Jolis, E. M., Freda, C., Troll, V. R., Deegan, F. M., Blythe, L. S., McLeod, C. L., et al. (2013). Experimental simulation of magma-carbonate interaction beneath Mt.Vesuvius, Italy. Contributions Mineralogy Petrology 166, 1335–1353. doi:10.1007/s00410-013-0931-0
Jousset, P., Pallister, J., and Surono, (2013). The 2010 eruption of Merapi volcano. J. Volcanol. Geotherm. Res. 261, 1–6. doi:10.1016/j.jvolgeores.2013.05.008
Luais, B., Telouk, P., and Albarède, F. (1997). Precise and accurate neodymium isotopic measurements by plasma-source mass spectrometry. Geochim. Cosmochim. Acta 61, 4847–4854. doi:10.1016/S0016-7037(97).00293-7
Manhès, G., Allegre, C. J., Dupré, B., and Hamelin, B. (1980). Lead isotope study of basic-ultrabasic layered complexes–speculations about the age of the Earth and primitive mantle characteristics. Earth Planet. Sci. Lett. 47, 370–382. doi:10.1016/0012-821X(80)90024-2
Mollo, S., Gaeta, M., Freda, C., DiRocco, T., Misiti, V., and Scarlato, P. (2010). Carbonate assimilation in magmas: A reappraisal based on experimental petrology. Lithos 114, 503–514. doi:10.1016/j.lithos.2009.10.013
Pichavant, M., Martel, C., Bourdier, J.-L., and Scaillet, B. (2002). Physical conditions, structure, and dynamics of a zoned magma chamber: mount Pelée (Martinique, Lesser Antilles Arc). J. Geophys. Res. 107 (B5), 2093. doi:10.1029/2001JB000315
Pin, C., Briot, D., Bassin, C., and Poitrasson, F. (1994). Concomitant separation of strontium and samarium-neodymium for isotopic analysis in silicate samples, based on specific extraction chromatography. Anal. Chim. Acta 298, 209–217. doi:10.1016/0003-2670(94)00274-6
Pouchou, J. L., and Pichoir, F. (1984). A new model for quantitative X-ray-microanalysis. Application to the analysis of homogenous samples. La Rech. Aerosp. 3, 167–192.
Schwarzkopf, L. M., Schmincke, H. U., and Cronin, S. J. (2005). A conceptual model for block-and-ash flow basal avalanche transport and deposition, based on deposit architecture of 1998 and 1994 Merapi flows. J. Volcanol. Geotherm. Res. 139 (1-2), 117–134. doi:10.1016/j.jvolgeores.2004.06.012
Shaw, C. S. J. (2006). Effects of melt viscosity and silica activity on the rate and mechanism of quartz dissolution in melts of the CMAS and CAS systems. Contrib. Mineral. Petrol. 151, 665–680. doi:10.1007/s00410-006-0086-3
Smyth, H., Hall, R., Hamilton, J., and Kinny, P. “East Java: cenozoic basins, volcanoes and ancient basement,” in Proceedings of the Indonesian Petroleum Association, 30th Annual Convention, Jakarta, Indonesia, January 2005, 251–266.
Stern, K. H., and Weise, E. L. (1966). High temperature properties and decomposition of inorganic salts: Carbonates. Maryland, USA: US Department of Commerce, National Bureau of Standards.
Thirlwall, M. F. (2002). Multicollector ICP-MS analysis of Pb isotopes using a 207Pb-204Pb double spike demonstrates up to 400 ppm/amu systematic errors in Tl-normalization. Chem. Geol. 184, 255–279. doi:10.1016/S0009-2541(01)00365-5
Troll, V. R., Deegan, F. M., Jolis, E. M., Harris, C., Chadwick, J. P., Gertisser, R., et al. (2013). Magmatic differentiation processes at Merapi volcano: inclusion petrology and oxygen isotopes. J. Volcanol. Geotherm. Res. 261, 38–49. doi:10.1016/j.jvolgeores.2012.11.001
Voight, B., Constantine, E. K., Siswowidjoyo, S., and Torley, R. (2000). Historical eruptions of Merapi volcano, central Java, Indonesia, 1768–1998. J. Volcanol. Geotherm. Res. 100 (1-4), 69–138. doi:10.1016/s0377-0273(00)00134-7
Whitley, S., Halama, R., Gertisser, R., Preece, K., Deegan, F. M., and Troll, V. R. (2020). Magmatic and metasomatic effects of magma–carbonate interaction recorded in calc-silicate xenoliths from Merapi volcano (Indonesia). J. Petrology 61, 1–38. doi:10.1093/petrology/egaa048
Keywords: crustal assimilation, kinetics, mechanism, rate, carbonate crust, calc-silicate xenolith, mineral dissolution, silicic melts
Citation: Borisova AY and Bohrson WA (2023) How is carbonate crust digested by magma?. Front. Earth Sci. 11:1186207. doi: 10.3389/feart.2023.1186207
Received: 14 March 2023; Accepted: 28 June 2023;
Published: 10 August 2023.
Edited by:
Patrizia Fiannacca, University of Catania, ItalyReviewed by:
Rong Xu, Chinese Academy of Sciences (CAS), ChinaSaskia Erdmann, UMR7327 Institut des Sciences de la Terre d'Orléans (ISTO), France
Copyright © 2023 Borisova and Bohrson. This is an open-access article distributed under the terms of the Creative Commons Attribution License (CC BY). The use, distribution or reproduction in other forums is permitted, provided the original author(s) and the copyright owner(s) are credited and that the original publication in this journal is cited, in accordance with accepted academic practice. No use, distribution or reproduction is permitted which does not comply with these terms.
*Correspondence: Anastassia Y. Borisova, YW5hc3Rhc3NpYS5ib3Jpc292YUBnZXQub21wLmV1