- 1EXPEC ARC (Exploration and Petroleum Engineering Center—Advanced Research Center), Dhahran, Saudi Arabia
- 2Geochemical and Environmental Research Group, Texas A&M University, College Station, TX, United States
The Mississippian mudrocks of central Oklahoma, USA, encompass hydrocarbon-rich unconventional reservoirs. Many factors control the success of hydrocarbon producibility from the Sooner Trend oilfield, Anadarko Basin, and Canadian and Kingfisher Counties (“STACK”) play in Oklahoma, including rock mineralogy, thermal maturity, and organic richness. Petroleum sourcing in these Mississippian reservoirs is complex, encompassing multiple hydrocarbon charges from different source rocks in addition to the Woodford Shale. Therefore, determining petroleum generation kinetic parameters for Mississippian mudrocks is crucial to understand the thermal maturation and associated hydrocarbon fluid types, which can ultimately aid in identifying the “sweet spot”. This investigation presents the first experimental evidence of petroleum generation kinetics for the Mississippian mudrocks in central Oklahoma. Here, core samples collected from Lincoln County, central Oklahoma, were examined for petroleum generation kinetics using a hydrous pyrolysis reactor. Generated pyrolysate products were then examined for key molecular parameters in comparison with produced crude oils. The results from the hydrous pyrolysis experiments and corresponding Arrhenius plots suggest slightly higher reaction rates for hydrocarbons generated from the Mississippian mudrocks compared to the Woodford Shale. These reaction rates result in lower activation energy and frequency factor values for the Mississippian mudrocks when compared to the Woodford Shale. Molecular signatures from pyrolysate oils match with Mississippian sourced crude oils with a predominance of lower and extended tricyclic terpane biomarkers. The geological implications of the determined kinetics suggest that Mississippian petroleum source rocks can generate oil and gas at lower temperatures compared to the Woodford Shale. The low kinetic parameters of Mississippian rocks are postulated due to organic-matter structure co-occurring as infused amorphinite and bituminite within the mineral matrix. The large surface area between the macerals and the mineral matrix could increase the reactivity, with clays acting as catalysts for petroleum generation.
1 Introduction
In Oklahoma, United States, petroleum production from the Mississippian strata has occurred since 1897 (Franks, 1980). To date, the majority of the produced hydrocarbons are from the Mississippian reservoirs in northern Oklahoma and Southern Kansas. In particular, new discoveries of hydrocarbons contained within a number of Mississippian unconventional tight mudrock reservoirs, encompassing the Sooner Trend oilfield, Anadarko Basin, and Canadian and Kingfisher Counties (collectively known as “STACK”) and the South Central Oklahoma Oil Province (SCOOP) (Figure 1) (Milam, 2013; Atwah et al., 2019).
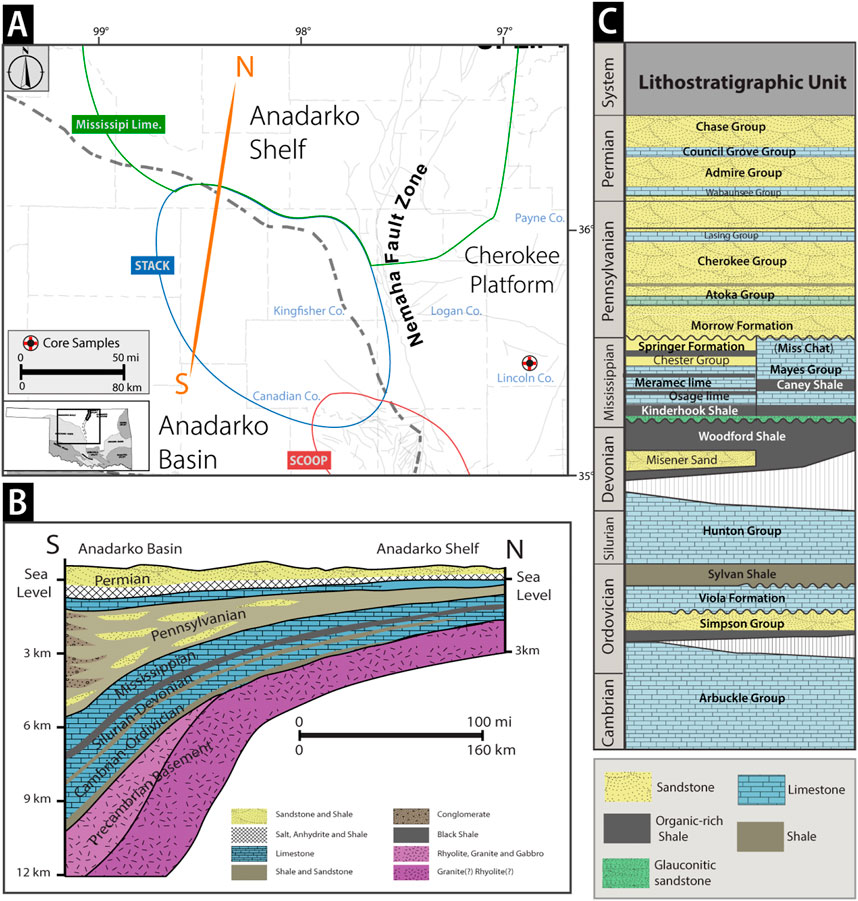
FIGURE 1. (A) Study area map showing boundaries of major Devonian-Mississippian hydrocarbon plays in Oklahoma. The location of the study core is indicated in the southern part of Lincoln County; (B) Generalized north to south cross-section of the Anadarko Basin (C) Generalized stratigraphic column of the Anadarko Basin formations denoting major source rocks and reservoir intervals, according to extracted data from previously published study (Atwah et al., 2021).
Petroleum source rocks in the Anadarko Basin range from Ordovician to Pennsylvanian in age, with the primary source of hydrocarbons being contained within the Devonian-Mississippian rocks (Wang and Philp, 1997; Al Atwah et al., 2019). The Woodford Shale is comprised of organic-rich radioactive shales that are regionally present across Oklahoma (Kirkland et al., 1992; Miceli Romero and Philp, 2012; Rivera et al., 2015; Curiale and Curtis, 2016). In addition to the Woodford Shale, a number of Mississippian organic-rich carbonate rocks exhibit source rock potential (Atwah et al., 2019). These complex molecular fingerprints observed in Mississippian-reservoir fluids are attributed, in part, to variations in the type and quantity of organic matter and lithology resulting from the presence of multiple source rocks (Atwah et al., 2019). Therefore, the regional distribution of Mississippian petroleum accumulations shows variable compositional and bulk physical properties across the Anadarko Basin (Wang and Philp, 1997; Atwah et al., 2019). This variability strongly influences oil and gas wells performance in producing from unconventional tight reservoirs (Curiale and Curtis, 2016).
Understanding petroleum generation kinetics of source rocks is crucial for defining oil and gas windows and the timing of hydrocarbon generation. In Oklahoma, the Woodford Shale has gained significant attention due to the regional-scale presence and the widespread availability of core samples (Cardott, 2012; Quan et al., 2013; Abrams and Thomas, 2020; Atwah et al., 2020). Conversely, the Mississippian mudrocks and associated organic-rich facies are heterogeneously complex, and only a few organic geochemical evaluations have shown in some locations the presence of good source rock potential (Wang and Philp, 1997; Atwah et al., 2020). Kinetic parameters within the Woodford Shale have been previously investigated using hydrous pyrolysis isothermal experiments (Lewan and Ruble, 2002); however, petroleum generation kinetics remain poorly constrained for the Mississippian mudrocks, largely due to the assumption that the Mississippian rocks function as a reservoir rather than a source of hydrocarbons. (Dolton and Finn, 1989; Ball et al., 1991). This study aims to define the petroleum generation kinetics of the organic-rich Mississippian rocks by carrying out series of hydrous pyrolysis experiments combined with molecular geochemistry of generated fluids. The results of this research can provide a better understanding of the petroleum generation kinetics of unconventional Mississippian Mudrocks in Central Oklahoma, and contribute to the development of effective exploration and production strategies.
2 Geological settings
The studied core is situated within the Cherokee Platform province in central Oklahoma, United States (Figure 1). The Cherokee platform is bounded to the east by the Ozark Uplift and to the west by the Nemaha Uplift. The Cherokee Platform was part of the regional Oklahoma Basin during early to middle Paleozoic time and prior to the development of present-day Oklahoma’s geological provinces (Johnson, 1989). During this period, the Oklahoma Basin was part of passive-margin settings with marine sediments forming cratonic basin settings, that resulted in thick deposits of marine carbonate formation interbedded with sandstone and shales, including the deposition of the Mississippian carbonate formations (Garner and Turcotte, 1984; Donovan, 1986).
The transition from the passive margin setting across the Oklahoma Basin to form present-day geological features occurred during an orogenic episode during Late Mississippian to Pennsylvanian time (Johnson, 1989; Perry, 1989. This phase marks the shift of a passive margin and cratonic basin to foreland basin settings. This period of foreland deformation was additionally accompanied by intense crustal shortening, folding, uplifts, and downwarping, ultimately disjointing the Oklahoma Basin into tectonic provinces comparable to present-day structural features, including the formation of the Cherokee platform (Ham et al., 1964; Johnson, 1989).
The Mississippian-aged formations are primarily carbonates interbedded micritic facies across the Anadarko Basin and Cherokee Platform (Curtis and Champlin, 1959). A recent investigation of the Mississippian mudrocks across STAK play documented mixed fine-grained lithology of clay, carbonate grains, and quartz silts, with clay content in the argillaceous quartz siltstone facies ranging between 25% and 50% within the Mississippian mudrocks (Price et al., 2020). The organic geochemistry of Mississippian mudrocks is characterized by more than 2% of total organic carbon by weight percent and enrichment in hydrogen index based on pyrolysis analysis (Atwah et al., 2020). Moreover, Mississippian mudrocks, associated oils, and oil-bearing fluid inclusion are well-known to have unique biomarker signatures such as the enrichment of extended tricyclic terpane relative to hopane in the terpane biomarker class and enrichment of cholestane relative to stigmastane and ergostane in the sterane biomarker class (Atwah et al., 2021).
3 Materials and methods
3.1 Core and crude oil samples
Rock samples were obtained from a core located in Lincoln County, central Oklahoma (Figure 1). Since the STACK play is situated at the Anadarko Basin’s northeastern edge at which both the Mississippian and Woodford Shale have been subjected to substantial thermal stress (Ro>0.9%), which disqualify any rocks within the STACK play for hydrous pyrolysis to derive kinetic parameters (Cardott, 2012). The selected core is used as an immature analog for the Mississippian mudrocks with great source rock potential. Measurements of the maturity of solid bitumen and vitrinite show that the core is within 0.49% Ro (Figure 2). For hydrous pyrolysis, a composite sample of the most organic-rich Mississippian samples was prepared by combining two organic-rich rock samples from depths of 4,983 ft and 5,050 ft to achieve the sufficient rock mass required for the experiment. The composite Mississippian source rock was crushed into 2–3-mm sized rock chips. The composite source rock was then subdivided into nine rock aliquots for hydrous pyrolysis experiments.
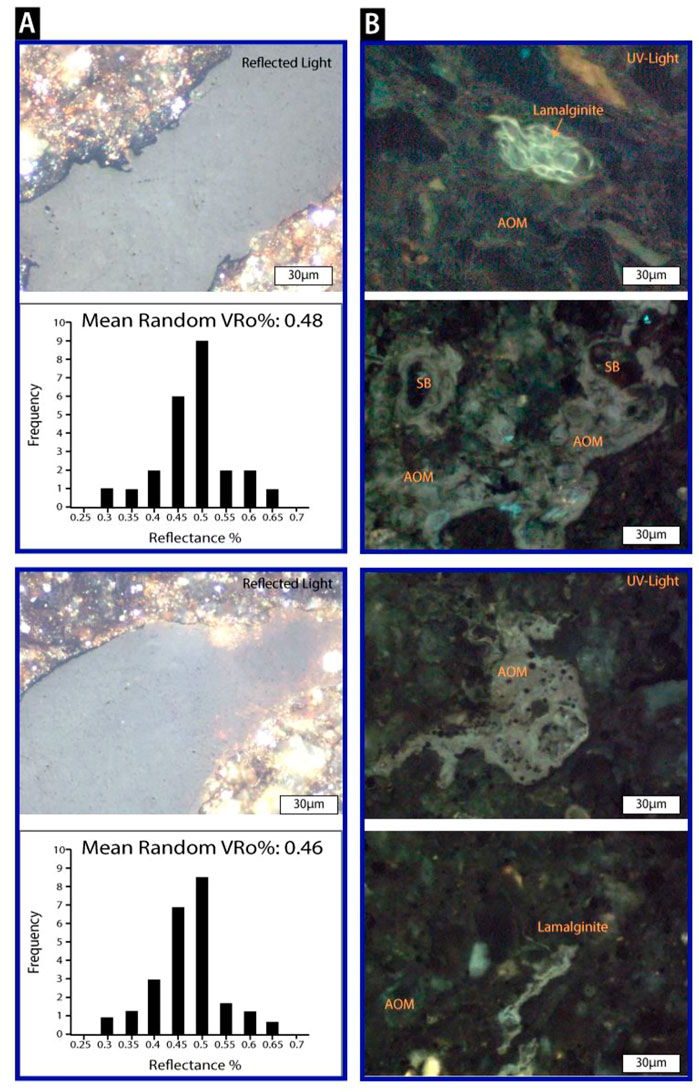
FIGURE 2. (A) Example microphotograph of vitrinite clasts and histogram reflecting the distribution of measured random VRo%; (B) Microphotographs under UV light reflecting major maceral structures in the Mississippian samples including lamalginite, amorphous organic matter (AOM), and solid bitumen (SB).
Two selected crude-oil end-members were included in this study. The two end-members represent typical Woodford-sourced and Mississippian-sourced oils. Both oils showed distinctive geochemical signatures and exhibited a thermal maturity within the peak-oil window (0.7%–0.9% Ro equivalent) based on a previous study (Atwah et al., 2019). The crude oil end-members were included for comparison purposes with the hydrous pyrolysis-generated fluids.
3.2 Hydrous pyrolysis
For each sample, organic-rich rock chips (30 g) were placed in a 250 mL T316 Parr reactor vessel and submerged in distilled water at various volumes. The exact amount of distilled water used is calculated to ensure rocks were completely submerged in water during the entire hydrous pyrolysis temperature conditions (Meyer et al., 1983). The vessel was then tightly sealed and purged with nitrogen followed by argon gas. The vessel then was placed into the heating module and subjected to an isothermal range from 280°C to 360°C. The warm-up time of the reaction vessel ranged from 0.5 to 1 h, after which the reaction vessel was kept at a single temperature for 72 h. After the reaction, the vessel was cooled and expelled oil was recovered. The inner walls of each vessel were washed using dichloromethane and then dewatered using sodium sulfate. The generated oil in each experiment was weighed to derive the kinetics based on Arrhenius plots. This approach assumes a first-order reaction for petroleum generation, as proven through time-series experiments. Thus, the kinetic parameters are determined based on the oil expelled from the hydrous pyrolysis experiments using the following Arrhenius expression:
where
For petroleum generation, the Arrhenius equation can be rearranged as a function of the oil generation extent [f (Ɛ)], as follows:
where
were
Using this relationship, the rate constant (kT) for each experimental temperature can be expressed as:
where
Combining Eqs (2, 4) gives the following expression:
Kinetic parameters including the activation energy (Ea) and frequency factor (A) can be determined by plotting the natural logarithm of the rate constant (Ln k) against the reciprocal of temperature (1/K). Ea is then defined as the slope and the frequency factor is calculated from the intercept.
3.3 Rock-Eval pyrolysis
Both pre and post hydrous pyrolysis rock samples were analyzed on Rock-Eval 6. Approximately 60 mg of rock powder was placed in pyrolysis crucibles and loaded on the autosampler. The Rock-Eval instrument was set on the classic source rock and total organic matter (TOC) evaluation parameters as described by previous studies (Espitalie and Bordenave, 1993). In brief, the pyrolysis oven programmed was set at initial temperature of 300°C for 4 min, which then increased to 650°C at a heating rate of 25°C/min, whereby all pyrolyzed carbon is detected by the flame ionization detector. For the oxidation oven program, the sample was heated from 300°C to 850°C at a heating rate of 25°C/min, whereby all mineral and residual carbon is detected by the sensitive infrared detector.
3.4 Organic petrography
Organic petrography and vitrinite reflectance analysis were undertaken for a total of eight samples, including initial samples (before hydrous pyrolysis) and pyrolyzed samples (after pyrolysis). Whole-rock pellets were prepared for the characterization of organic macerals and reflectance measurements. Whole-rock chips ranging in size from 1 to 2 cm were mounted in one-inch epoxy resin pellets. For the pyrolyzed rocks, samples were kept in a desiccator overnight to dry the samples before the preparation of the epoxy pellets. The pellets were then ground and polished using silicon carbide paper (grit sizes from P220 to P1,200) in the presence of deionized water, and further polished using 0.3 μm and 0.05 μm alumina polishing suspensions.
The prepared rock pellets were examined using a Leica microscope equipped with a CRAIC 508 PV™ Microscope Spectrophotometer to characterize fluorescence and petrography. Macerals were counted (at approximately 500 points) and classified. Reflectance analyses were performed on vitrinite (VRo%) and solid bitumen (BRo%) by taking an average of approximately 25 reflectance measurements per sample. Vitrinite was identified, and reflectance measurements were made on a random-mean basis. Very limited clasts of vitrinite were observed that couldn’t be conclusively distinguished from solid bitumen solely from morphology; however, in such instances, vitrinite is identified based on slightly elevated reflectance, and unlike solid bitumen, vitrinite clasts did not fluorescence under UV light. Solid bitumens were identified based on morphology, and it’s weak fluorescence, solid bitumen reflectance was also measured and corrected to the vitrinite equivalent reflectance values (Landis and Castaño, 1995). Glass standards (i.e., spinel, strontium-titanate, and yttrium-aluminum-garnet) were used to calibrate the reflected light microscope.
3.5 SARA and gas chromatography-mass spectrometry
The oil samples generated from each experiment were spiked with surrogate standards (β-cholane and d10-fluorene) and then analyzed using a gas chromatograph equipped with a triple quadrupole mass spectrometer (GC-MS-QQQ). Prior to the analysis, an aliquot of pyrolysate oil was fractionated into saturate, aromatic, resins, and asphaltenes (SARA) based gravimetric quantification of each fraction. First, pyrolysate oil was extracted with n-pentane into maltenes and precipitated asphaltenes. Maltenes were further fractionated using silica-gel open-column to saturate, aromatic, and resins. In the saturate fraction, linear alkanes were subsequently removed using a molecular sieve column. Both saturate, and aromatic fractions were then analyzed using an Agilent 7890-7010B triple quadrupole system with a DB-1 fused-silica column (60 m × 0.25 mm × 0.25 μm) for separations. The samples were then heated in an oven at an initial temperature of 80°C for 1 min rising to 320°C at a rate of 2°C/min and held at the final temperature for 5 min. Helium was used as a carrier gas with a flow rate of 1.3 mL/min, and in the second quadrupole, nitrogen was used as the collision gas. The GC-MS-QQQ was run in precursor-product ion mode for multiple reaction monitoring (MRM) and single ion monitoring (SIM).
4 Results
4.1 Organic petrography
Organic petrography microphotographs are shown in Figure 2. Macerals contained in the examined Mississippian rocks are predominantly composed of amorphous organic matter (AOM). These AOM structures are infused within the mineral matrix, as shown in Figures 2A, B, with AOM exhibiting light-yellow fluorescence under UV light and appearing dark-brown-to-gray under reflected light. Structured liptinite macerals were observed in very few cases, which were classified as telalginites. Some vitrinite and solid bitumen clasts were also observed, and their reflectance was measured. The initial samples exhibited a random mean reflectance value of 0.48 Ro%, which strongly suggest, that most of the observed solid bitumen is pre-oil solid based on Curiale (1986) classification, which resulted from the conversion of solid organic matter (kerogen) at lower thermal maturity to semi-solid bitumen in the source rock. Additionally, the post-pyrolysis rock samples showed a linear increase in reflectance values (from 0.54% to 1.27%) at higher hydrous pyrolysis temperatures. A further detailed comparison between macerals in Mississippian mudrocks and Woodford Shale can be found in previously published studies (Cardott, 2012; Atwah et al., 2020).
4.2 Rock-Eval and hydrous pyrolysis
Table 1 lists the measured pyrolysis parameters of the initial samples, and a comparison of Rock-Eval parameters for the initial and pyrolyzed rock samples is provided in Figure 3. The composite (before hydrous pyrolysis) Mississippian rocks exhibit a total organic carbon (TOC) content of 7.61% consisting of 2.47 mg/g of free hydrocarbons and 40.21 mg/g of thermally cracked hydrocarbons. Organic content showed a decreasing trend with increasing temperature for the post-pyrolysis samples, as reflected by the positive correlation between Tmax values and production index (PI) values (Figure 3A). Similarly, this trend was observed in the Pseudo–Van Krevelen diagram, with hydrogen index (HI) and oxygen index (OI) values showing a decreasing trend (Figure 2B). While Rock-Eval pyrolysis parameters are crucial for evaluating retained hydrocarbons for unconventional shale oil plays, however, all the listed Rock-Eval pyrolysis data are intended for evaluating hydrocarbon generation potential and will be further examined in detail in another study for evaluating hydrocarbon expulsion and retention phenomena in Mississippian mudrocks.
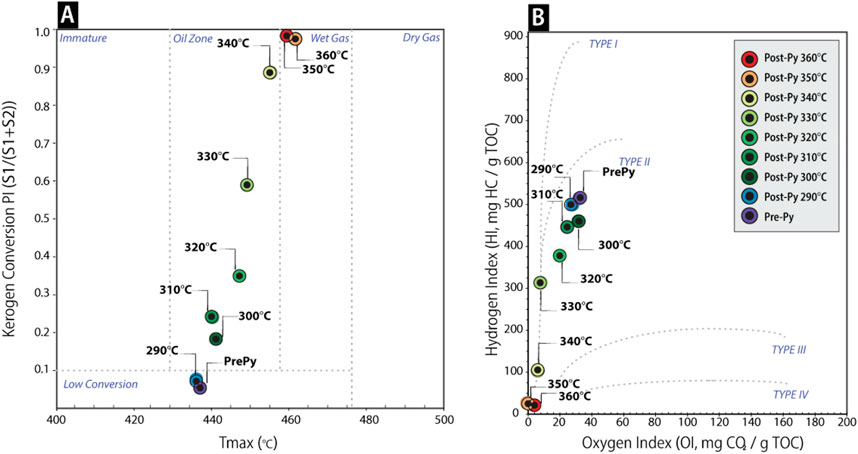
FIGURE 3. Rock-Eval pyrolysis results: (A) Cross plot comparing kerogen conversion (PI) and Tmax; (B) Pseudo–Van Krevelen diagram for the examined samples comparing (HI) with oxygen index (OI). Note the similarities between the two diagrams showing a linear trend with increasing hydrous pyrolysis temperature as a function of thermal maturation.
The results of the hydrous pyrolysis experiments are summarized in Table 2; Figure 4. Expelled oil yields of the examined Mississippian samples were compared to the Woodford Shale based on previous work (Lewan and Ruble, 2002). The maximum yields of expelled oil from the Mississippian samples were observed at 330°C, with a total of 307 mg of expelled oil per gram of TOC (mg/gTOC) (Table 2). Minimum yields were observed at 280°C, at 25 mg/gTOC. A dramatic increase in yield was observed at the transition from 300°C to 310°C, from 78.10 mg/gTOC to 262.77 mg/gTOC (Table 2). Above these temperatures, oil yields slightly increased to their maximum (at 330°C) and then decreased between 340°C and 360°C (Figure 4). The activation energy (Ea) and frequency factor (A0) for the Mississippian samples were determined to be 41.87 kcal/mol and 4.16 × 1013 h-1, respectively, which are lower than those of the Woodford Shale (52.16 kcal/mol and 6.51 × 1016 h-1, respectively).
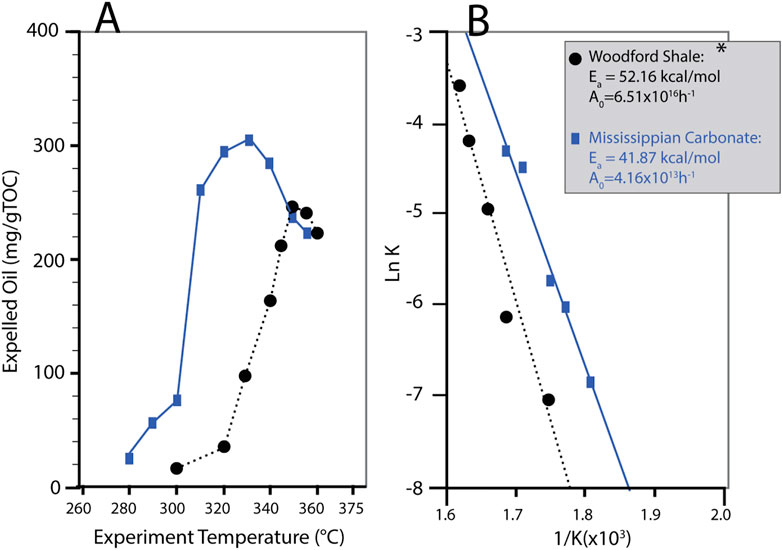
FIGURE 4. (A) Cross plot comparing hydrous pyrolysis temperature and the weight of C15+ hydrocarbons generated after the reaction was complete; (B) Arrhenius plot assuming a first-order reaction rate with Woodford Shale data extracted from previous study (Lewan and Ruble, 2002).
4.3 Geochemical composition
4.3.1 Saturate hydrocarbons
Figure 5 shows the distribution of C10 and higher normal alkanes and isoprenoids for some selected samples. A noticeable change was observed in the abundance of n-alkanes relative to isoprenoids before and after pyrolysis. The initial rock extracts showed a unique distribution of n-alkanes favoring odd-numbered carbons, with a high abundance of isoprenoids (Figure 5). The distribution of n-alkanes was significantly different in the expelled oils from the hydrous pyrolysis experiments. Overall, n-alkanes increased with a unimodal distribution peaking at C11. A subtle ‘hump’ was observed in the chromatogram baselines at lower temperatures (<320°C) while at temperatures of 330°C and above, the baseline was more stable with a similar overall distribution of n-alkanes (Figure 5).
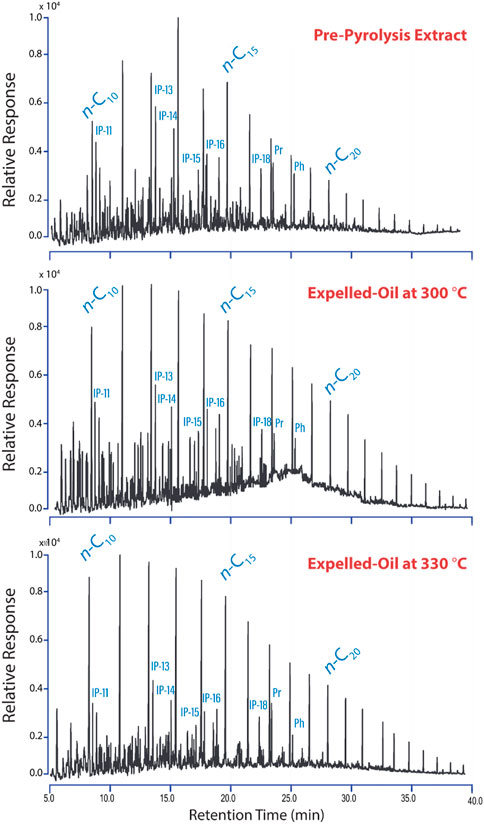
FIGURE 5. Gas chromatograms for Mississippian rock extracts before pyrolysis (top) and immiscible oil generated at 300°C (middle) and 330°C (bottom). Note the relative abundance of n-alkane to isoprenoids (IP). Pr = pristane and Ph = phytane.
Terpane biomarkers of some selected samples are shown in Figure 6 and their ratios are listed in Table 3. Mass chromatograms for the Mississippian samples are also shown in Figure 6 along with those of crude oils produced from the Mississippian and Woodford Shale sources. Generally, samples showed a varying abundance of extended tricyclic terpanes, with an extended tricyclic terpane to homohopane ratio (ETT/HH) ranging from 1.56 to 7.25 and a high abundance of tricyclic terpanes in the Mississippian pyrolysate and crude oils (Figure 6). In contrast, Woodford crude oil exhibited a low ETT/HH ratio and a predominance of pentacyclic terpanes, with high abundance of C30 hopane relative to C29 nor-hopane. The hopane to moretane ratio (Hop/Mor) for all of the examined samples ranged between 0.02 and 1.60 while the Ts/Tm ratio ranged between 0.24 and 0.66. For the sterane biomarkers, the C27/C29 ratio of the regular steranes (ααα 20R) varied across the examined samples, ranging between 0.62 and 0.95 (Table 3).
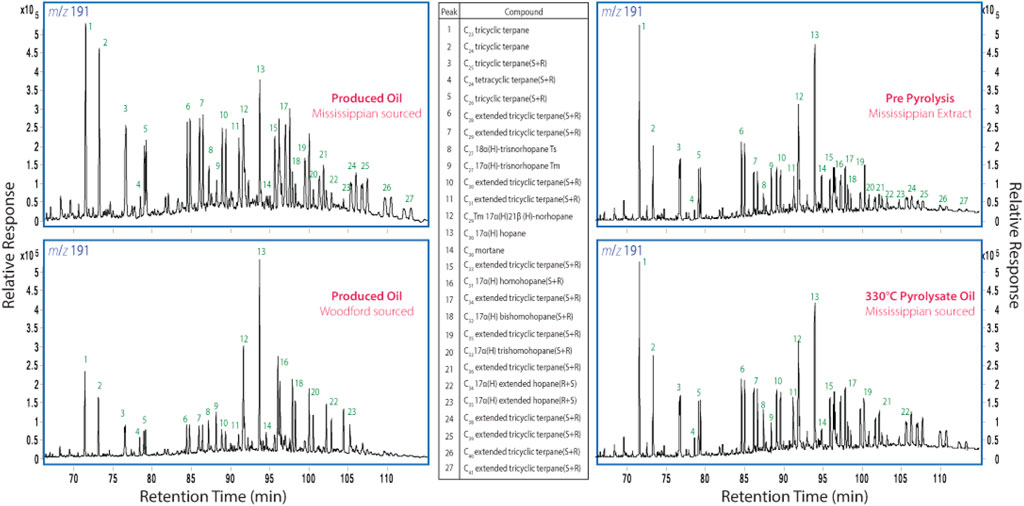
FIGURE 6. Mass chromatograms for crude oils sourced from the Mississippian mudrocks (top left) and Woodford Shale (bottom left) and in central Oklahoma and for pre-pyrolysis bitumen (top right) and pyrolysate generated at 330°C (bottom right). Note the overall abundance of extended tricyclic terpanes at different maturities in Mississippian sourced fluids.
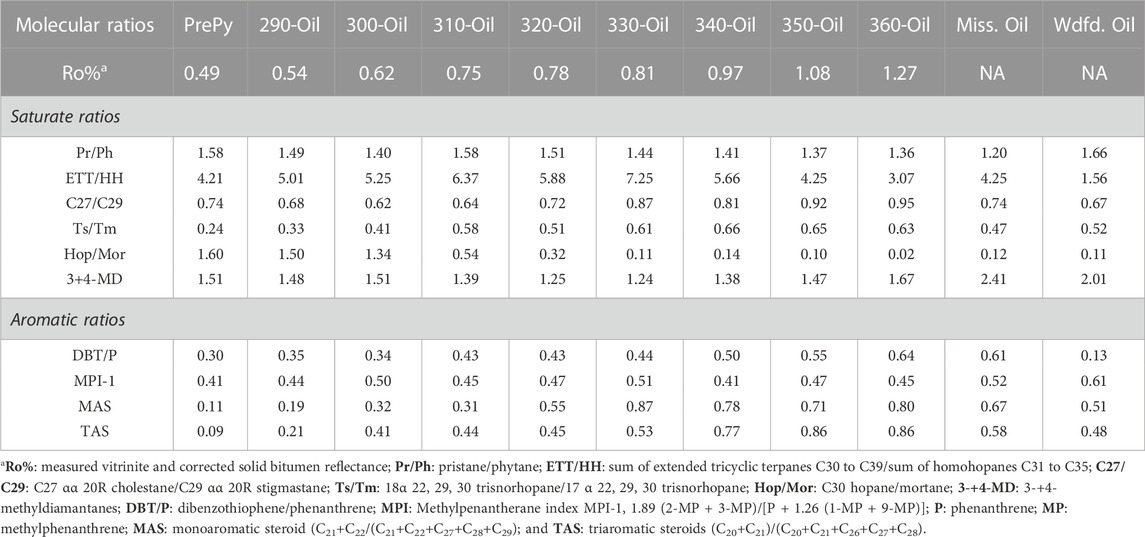
TABLE 3. Key molecular ratios as a function of the maturity of saturate and aromatic hydrocarbons obtained from pre-pyrolysis bitumen and pyrolysate oils.
4.3.2 Aromatic hydrocarbons
Selected aromatic ratios for maturity and source are listed in Table 3. For source rock facies, Figure 7 provides a comparison of dibenzothiophene/phenanthrene (DBT/P) and pristane/phytane ratios, with all samples plotting within the “marine shale” source. Figure 8 compares multiple aromatic maturity ratios of pyrolysate oils with the associated measured vitrinite reflectance values of the rocks. The triaromatic steroid (TAS) ratios showed the strongest linear relationship with measured vitrinite reflectance, while the monoaromatic steroid (MAS) ratios showed less consistency with the reflectance values (Figure 8). Methyl phenanthrene index values varied and did not exhibit any systematic changes with an increase in maturity (Figure 8).
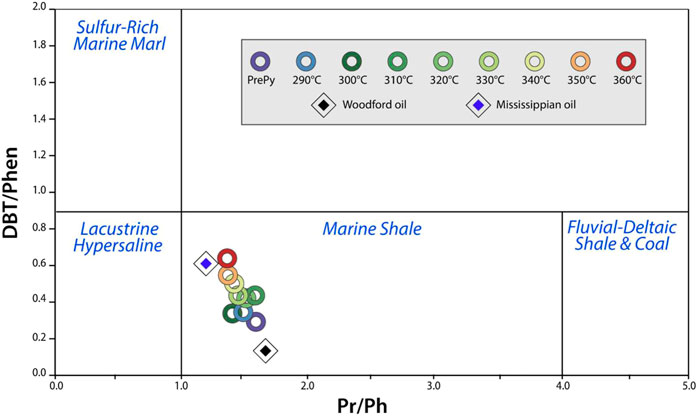
FIGURE 7. Cross-plot used to estimate the depositional settings of source rocks and generated oils. Note all examined samples plot within the “Marine Shale” setting. DBT = dibenzothiophene; Phen = Phenanthrene; Pr = pristane; and Ph = phytane. Modified from Hughes, et al. (1995).
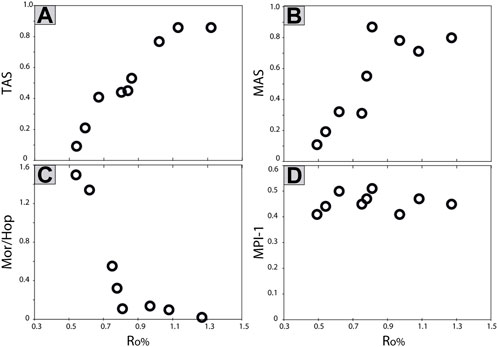
FIGURE 8. Cross-plot comparing changes in the maturity parameters of pyrolysate oils and the measured vitrinite reflectance of post-pyrolysis rocks: (A) triaromatic steroid ratios against Ro%; (B) monoaromatic steroid ratio against Ro%; (C) moretane/C30 hopane against Ro%; and (D) methylphenanthrene index against Ro%. The formulas used for calculating the ratios are provided in Table 3.
5 Discussion
5.1 Petroleum generation in Mississippian rocks
Petroleum generation in Mississippian source rocks in Oklahoma is confirmed through multiple lines of evidence in this study and previous studies. First, petroleum was successfully generated from Mississippian rocks through hydrous pyrolysis experiments. Second, high total organic matter content in the Mississippian rocks (7.61%) and the S2 peak and hydrogen index (Table 1) indicate the high hydrocarbon generation potential of the examined core. Organic petrography evidence is also consistent with the generation potential from pyrolysis, through the observed occurrences of enriched fluorescing amorphous organic matter (Figure 2). Furthermore, the observations reported here are consistent with previous studies reporting elevated organic content in Mississippian carbonates and mudrocks in Oklahoma (Al Atwah et al., 2019; Atwah et al., 2019). Despite this, the regional distribution and presence of organic-rich facies within the Mississippian strata across the Anadarko Basin and adjacent shelves remain largely unknown, this in part because of the difficulty of establishing regional chronostratigraphic based stratigraphic framework because of the lack of diagnostic biostratigraphic markers that can define the Mississippian sets of prograding clinoforms (Wilson et al., 2019). Nevertheless, muddier Mississippian facies are reported across a large area within the STACK play and it is likely that these facies extend south towards deeper water settings according to the regional paleo-depositional context (Price et al., 2020).
Petroleum generation kinetics of the Mississippian source rocks are relatively low compared to other marine source rocks, and particularly the Woodford Shale (Figure 4). Typically, low activation energy and frequency factor values are associated with the presence of organo-sulfur compounds or sulfur-rich kerogen (Lewan, 1985; Baskin and Peters, 1992). Ideally, measuring organic sulfur content in kerogen isolate of Mississippian mudrocks would provide an accurate assessment of organo-sulfur presence. However, a molecular approach of Mississippian pyrolysate oils can be used to deduce that the examined Mississippian mudrocks are unlikely to contain type II-S kerogen. First, the ratio of dibenzothiophene (DBT) relative to phenanthrenes (Phen) was low in the generated pyrolysates and crude oils (Figure 7). According to Hughes et al. (1995), sulfur-rich source rock and oils typically show a DBT/Phen ratio higher than 1, which positively correlated with the measured total sulfur content in crude oils. The DBT/Phen ratio has been shown to remain unaffected by thermal maturity as determined by pyrolysis and molecular geochemistry approaches (Srinivasan et al., 2022). Second, oils produced from the Woodford and Mississippian rocks are, broadly speaking, sweet crudes with minimal hydrogen sulfide content.
The low activation energy observed in Mississippian mudrocks could be attributed to the organic matter type and associated morphological structure. As shown in Figure 2, Mississippian macerals predominantly consist of amorphinite and bituminite, which are characterized by soft, thinly-layered, and wavey amorphous morphology infused with the rock mineral matrix. Such structureless morphologies increase the surface area of macerals, allowing for more reactivity within the mineral matrix, especially where clays are present as clay catalytic reactions can lower the kinetic parameters of petroleum generation (Johns, 1979). A similar observation has been reported before within the Mississippian mudrocks in Oklahoma and several Mississippian-aged organic matter across different sedimentary basins (Stasiuk and Fowler, 2004; Gross et al., 2015; Atwah et al., 2020). The mineralogy of the Mississippian mudrocks in central Oklahoma indicates a considerable clay content. Moreover, organic-rich beds within the Mississippian limestone formation vary between clay-rich siltstones and mudstones and wackstones, with a clay content of up to 20%–25% (Atwah et al., 2020; Price et al., 2020). A more recent study examined uXRF and the elemental composition of Mississippian mudrocks in central Oklahoma, showing abundance of illite with mixed mineralogy of calcite and quartz, with a notable occurrence of potassium-feldspar (Atwah et al., 2023).
5.2 Molecular signatures
Expelled oils from the temperature experiment series showed SARA compositions similar to crude oil produced from Mississippian reservoirs. In general, the pyrolysate oils were highest in saturate hydrocarbons, followed by aromatics and then polar compounds (Table 2), which is consistent with previous hydrous pyrolysis experiments (Lewan and Ruble, 2002). This further supports the value of hydrous pyrolysis closed experiments, as the fluids they generate have a similar composition to naturally-generated hydrocarbons over geological timescales.
The generated pyrolysate oils from the Mississippian mudrocks exhibit geochemical fingerprints similar to some crude oils produced from Mississippian reservoirs across the Anadarko Basin. In general, the presence and enrichment of tricyclic terpanes is a typical characteristic of Mississippian-sourced crudes in Oklahoma (De Grande et al., 1993; Al Atwah et al., 2019; Atwah et al., 2020). The generation of extended tricyclic terpanes was observed to increase with increasing hydrous pyrolysis temperatures, mirroring the amounts of expelled oils from the pyrolysis reactors (Table 3). This confirms that enrichment of lower and extended tricyclic terpanes in Mississippian sourced crude oils is related to the organic matter source and not to maturity. Although tricyclic terpanes exhibit higher thermal stability than pentacyclic terpanes, their enrichment even at low temperatures rules out the maturity origin and suggests their enrichment in the Mississippian samples is due to organic matter sources (Moldowan et al., 1983; De Grande et al., 1993). The abundance of extended tricyclic terpanes was also observed to increase at 330°C, which coincided with the highest yields of pyrolysate oils at 307 mg/gTOC (Figure 6).
The concentration of diamondoids, and particularly methyl diamantanes, in the Mississippian pyrolysate remained consistent throughout the temperature range of the experiments (Table 3). The diamondoid baseline as defined by Dahl et al. (1999) consists of the sum of 3- and 4-methyldiamantanes (3-4-MD). The diamondoid baseline for the Mississippian source rocks can be defined as 1.5 ppm (Table 3). While the overall concertation of diamantanes remained consistent across the different isothermal temperature regimes, a slight decrease in 3-4-MD was observed in pyrolysate oils generated at temperatures between 310°C and 340°C. This could be the result of hydrocarbon generation, representing a slight dilution effect. The observed diamondoid baseline for the Mississippian rocks also coincides with previously reported diamondoid concentrations in rock-extracts and crude oil produced from the Anadarko Basin (Atwah et al., 2019; Moldowan et al., 2015). Furthermore, the stability of the diamondoid baseline throughout the hydrous pyrolysis experiments suggests that the generated pyrolysate did not experience any further cracking. Such stability of pyrolysate during hydrous pyrolysis experiments is also reflected in the stable slope of normalized n-alkanes.
Molecular ratios indicative of the maturity of pyrolysate show some systematic changes under different degrees of thermal stress. For aromatic steroids, with increasing thermal maturity, the short-chain compounds increase relative to their higher homologs (Seifert and Moldowan, 1978; Mackenzie et al., 1982; Riolo et al., 1986). The triaromatic steroid ratio exhibits a better correlation with measured reflectance than the monoaromatic steroid ratio (Figure 8). This could be a function of the higher thermal stability of triaromatic steroids compared to their monoaromatic counterparts, as reflected by the presence of three benzene rings at the A, B, and C positions compared to the single C-ring (Seifert and Moldowan, 1978). Further aromatization of monoaromatic to triaromatic steroids is possible, which resulted in a slight scatter in the monoaromatic steroid ratios (Table 3). The moretane-to-hopane ratio was sensitive to maturity at the early-window stage and reached an equilibrium state at 0.9 Ro% (Figure 8). A similar trend was also observed in the Ts/Tm ratio, which reached equilibrium at an early-oil window stage (Table 3). This observation is consistent with previous studies of the monoaromatic steroid and Ts/Tm ratios (Seifert and Moldowan, 1980). Unlike most of the ratios, the methyl phenanthrene index varied the least with increasing hydrous pyrolysis temperature (Figure 8). This irregular distribution could be related to the lithology of the Mississippian rocks. Such an anomalous methyl phenanthrene response has also been reported in marine carbonate rock extracts from the La Luna Formation and its associated crude oils (Cassani et al., 1988).
5.3 Mississippian hydrocarbon charge
Hydrocarbon charge is a complex aspect of the Devonian-Mississippian petroleum systems in Oklahoma due to the presence of multiple source beds, i.e., the Woodford Shale and Mississippian mudrocks (Atwah et al., 2020). This is further compounded by episodic pulses of hydrocarbons from the same source but with different maturities, as evidenced by oil inclusions and molecular ratios (Atwah et al., 2021). Therefore, the petroleum generation kinetics of the Mississippian source rocks are a crucial piece of information for defining the episodes where these source rocks enter the oil and gas windows.
The geological implications of petroleum generation kinetics are typically integrated into basin modeling frameworks for the broad definition of rates of petroleum generation (Lewan and Ruble, 2002). In particular, temperature and time are the most crucial geological factors, which can be accounted for in geological heating rates. The heating rates of sedimentary basins typically range between 1°C/myr and 10°C/myr depending on tectonic and geothermal regimes (Gretener and Curtis, 1982; Allen and Allen, 2013; Higley, 2013). The heating rate within the Anadarko Basin is suggested to be 5°C/myr based on Bertha Rogers 1—one of the deepest drilled wells in Oklahoma (Higley, 2013). To highlight the impact of petroleum generation kinetics on hydrocarbon charge, Figure 9 shows source rock transformation over time by assigning a constant heating rate of 5°C/myr. A notable gap is apparent in petroleum generation at approximately 25 myr, with the Mississippian source rocks generating hydrocarbons before the Woodford Shale (Figure 9).
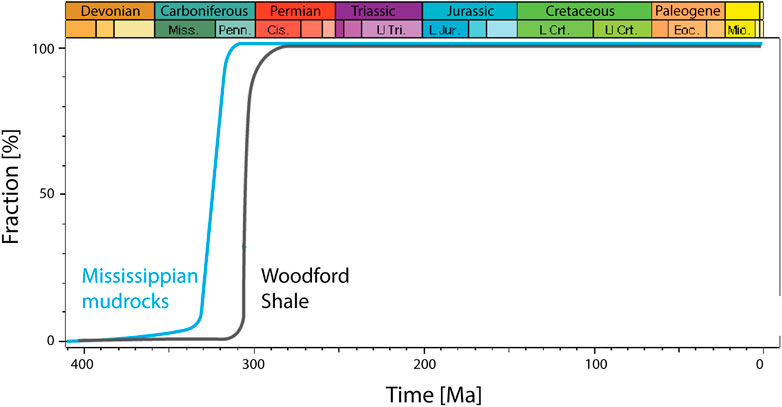
FIGURE 9. Petroleum generation curves for the burial history of the Woodford Shale and Mississippian mudrocks at the depocenter of the Anadarko Basin. The blue curve represents the transformation ratios of the Mississippian mudrocks determined using the hydrous pyrolysis experiments of this study. The black curve shows the Woodford Shale kinetics as determined based on data extracted from previous study (Lewan and Ruble, 2002).
The examination of petroleum generation kinetics from Mississippian carbonates in Oklahoma has yielded profound implications for the comprehension of the intricate process of hydrocarbon generation and its potential applications within the global geological community. The Mississippian carbonates in Oklahoma are deemed a critical hydrocarbon source rock, distinguished by their elevated organic content and thermal maturity. The kinetics of petroleum generation in these carbonates have been the subject of in-depth scrutiny, leading to invaluable insights that can be applied to analogous geological formations across the globe, such as those prevalent in the Middle East and North Africa. These discoveries have the potential to influence exploration strategies and the design of reservoir management practices, thereby enabling the sustainable development of hydrocarbon resources. The elucidation of petroleum generation kinetics from Mississippian carbonates in Oklahoma offers a sophisticated and scientifically rigorous framework that contributes significantly to the wider understanding of the energy industry.
6 Conclusion
• Mississippian source rocks in central Oklahoma recovered from an immature core showed evidence for hydrocarbon generation. Mississippian mudrocks are primarily composed of marine organic matter with amorphous macerals that lack a coherent structure and with a TOC of up to 7%.
• Hydrous pyrolysis experiments using these rocks generated petroleum at relatively lower kinetic parameter values, with an activation energy of 41.87 kcal/mol and a frequency factor of 4.16 × 1,013 h-1. Such low values are due to the amorphous nature of the macerals, with higher surface area contact with clay minerals that facilitate petroleum generation. Type II-S kerogen and associated organo-sulfur compounds are not present in large amounts in the Mississippian source rocks and did not, therefore, affect the kinetic analysis.
• The molecular ratios indicative of source and thermal maturity in Mississippian pyrolysate oils showed unique characteristics. Pyrolysate oils exhibit an abundance of extended tricyclic terpanes similar to naturally recovered crude oils within the Mississippian reservoir in Oklahoma. While thermal maturity ratios of particularly aromatic steroids showed systematic change with increasing hydrous pyrolysis temperature.
• Petroleum generation kinetics of Mississippian source rock in comparison with the Woodford Shale indicate hydrocarbon generation at a lower temperature. At a heating rate of 5°C/my, petroleum generation in Mississippian rocks would precede generation from the Woodford Shale by approximately 25 MY.
Data availability statement
The original contributions presented in the study are included in the article, further inquiries can be directed to the corresponding author.
Author contributions
IA: lab analysis, data interpretation, and drafted main manuscript. SS: conducted experiments, data interpretation and manuscript review.
Conflict of interest
Author IA was employed by the company Exploration and Petroleum Engineering Center—Advanced Research Center (Saudi Aramco).
The remaining author declares that the research was conducted in the absence of any commercial or financial relationships that could be construed as a potential conflict of interest.
Publisher’s note
All claims expressed in this article are solely those of the authors and do not necessarily represent those of their affiliated organizations, or those of the publisher, the editors and the reviewers. Any product that may be evaluated in this article, or claim that may be made by its manufacturer, is not guaranteed or endorsed by the publisher.
References
Abrams, M. A., and Thomas, D. (2020). Geochemical evaluation of oil and gas samples from the upper devonian and mississippian reservoirs southern Anadarko Basinbasin Oklahoma and its implication for the Woodford shale unconventional play. Mar. Petroleum Geol. 112, 104043. doi:10.1016/j.marpetgeo.2019.104043
Al Atwah, I., Puckette, J., Pantano, J., Arouri, K., and Moldowan, J. M. (2019). “Organic geochemistry and crude oil source rock correlation of devonian-mississippian petroleum systems in northern Oklahoma,” in Mississippian Reservoirs of the midcontinent. Editors J. M. G. G. M. Grammer, J. O. Puckette, P. Jaiswal, S. J. Mazzullo, M. J. Pranter, and R. H. Goldstein (Tulsa, Oklahoma, USA: The American Association of Petroleum Geologists), 301–321. Memoir v. 122.
Allen, P. A., and Allen, J. R. (2013). Basin analysis: Principles and application to petroleum play assessment. Chichester: John Wiley & Sons.
Atwah, I., Adeboye, O. O., Zhang, J., Wilcoxson, R., and Marcantonio, F. (2023). Linking biomarkers with elemental geochemistry to reveal controls on organic richness in Devonian-Mississippian mudrocks of Oklahoma. Palaeogeogr. Palaeoclimatol. Palaeoecol. 611, 111355. doi:10.1016/j.palaeo.2022.111355
Atwah, I., Mohammadi, S., Moldowan, J. M., and Dahl, J. (2021). Episodic hydrocarbon charge in tight Mississippian reservoirs of Central Oklahoma, USA: Insights from oil inclusion geochemistry. Mar. Petroleum Geol. 123, 104742. doi:10.1016/j.marpetgeo.2020.104742
Atwah, I., Puckette, J., Becker, M., and Moldowan, J. M. (2020). Source-rock reservoirs geochemistry of Devonian–Mississippian mudrocks in central Oklahoma. AAPG Bull. 104 (3), 657–680. doi:10.1306/06121918136
Atwah, I., Sweet, S., Pantano, J., and Knap, A. (2019). Light hydrocarbon geochemistry: Insight into mississippian crude oil sources from the Anadarko Basin, Oklahoma, USA. Geofluids 2019, 1–15. doi:10.1155/2019/2795017
Ball, M. M., Henry, M. E., and Frezon, S. E. (1991). Petroleum geology of the Anadarko Basin region, province (115), Kansas, Oklahoma, and Texas. Open-file report. Denver, Colorado, USA: U.S. Geological Survey, Denver Federal Center.
Baskin, D. K., and Peters, K. E. (1992). Early generation characteristics of a sulfur-rich Monterey kerogen. AAPG Bull. 76 (1), 1–13. doi:10.1306/BDFF874A-1718-11D7-8645000102C1865D
Cardott, B. J. (2012). Thermal maturity of Woodford Shale gas and oil plays, Oklahoma, USA. Int. J. Coal Geol. 103, 109–119. doi:10.1016/j.coal.2012.06.004
Cassani, F., Gallango, O., Talukdar, S., Vallejos, C., and Ehrmann, U. (1988). Methylphenanthrene maturity index of marine source rock extracts and crude oils from the Maracaibo Basin. Org. Geochem. 13, 73–80. doi:10.1016/0146-6380(88)90027-7
Curiale, J. A., and Curtis, J. B. (2016). Organic geochemical applications to the exploration for source-rock reservoirs – a review. J. Unconv. Oil Gas Resour. 13, 1–31. doi:10.1016/j.juogr.2015.10.001
Curiale, J. A. (1986). Origin of solid bitumens, with emphasis on biological marker results. Org. Geochem. 10 (1-3), 559–580. doi:10.1016/0146-6380(86)90054-9
Curtis, D. M., and Champlin, S. C. (1959). Depositional environments of Mississippian limestones Oklahoma. Tulsa Geol. Soc. Dig. 27, 90–103.
Dahl, J. E., Moldowan, J. M., Peters, K. E., Claypool, G. E., Rooney, M. A., Michael, G. E., et al. (1999). Diamondoid hydrocarbons as indicators of natural oil cracking. Nature 399 (6731), 54–57. doi:10.1038/19953
De Grande, S. M. B., Aquino Neto, F. R., and Mello, M. R. (1993). Extended tricyclic terpanes in sediments and petroleums. Org. Geochem. 20, 1039–1047. doi:10.1016/0146-6380(93)90112-o
Dolton, G. L., and Finn, T. F. (1989). Petroleum geology of the Nemaha uplift, central Midcontinent. Denver, Colorado, USA: U.S. Geological Survey, Denver Federal Center.
Donovan, R. N. (1986). Geology of the slick hills: Oklahoma geological survey guidebook. Norman, Oklahoma, USA: Oklahoma Geological Survey, 1–12.
Espitalié, J., and Bordenave, M. L. (1993). “Screening techniques for source rocks evaluation; tools for source rocks routine analysis; rock-eval pyrolysis,” in Applied petroleum geochemistry (Paris: Editions Technip), 261–273.
Garner, D. L., and Turcotte, D. L. (1984). The thermal and mechanical evolution of the Anadarko basin. Tectonophysics 107 (1-2), 1–24. doi:10.1016/0040-1951(84)90026-x
Gretener, P., and Curtis, C. (1982). Role of temperature and time on organic metamorphism: Geologic notes. AAPG Bull. 66, 1124–1129. doi:10.1306/03B5A651-16D1-11D7-8645000102C1865D
Gross, D., Sachsenhofer, R. F., Bechtel, A., Pytlak, L., Rupprecht, B., and Wegerer, E. (2015). Organic geochemistry of Mississippian shales (Bowland Shale Formation) in central Britain: Implications for depositional environment, source rock and gas shale potential. Mar. Petroleum Geol. 59, 1–21. doi:10.1016/j.marpetgeo.2014.07.022
Ham, W. E., Denison, R. E., and Merritt, C. A. (1964). Basement rocks and structural evolution of southern Oklahoma. AAPG Bull. 49, 927–934. doi:10.1306/A6633682-16C0-11D7-8645000102C1865D
Higley, D. K. (2013). 4D petroleum system model of the Mississippian System in the Anadarko Basin Province, Oklahoma, Kansas, Texas, and Colorado, U.S.A. Mt. Geol. 50, 81–98.
Hughes, W. B., Holba, A. G., and Dzou, L. I. (1995). The ratios of dibenzothiophene to phenanthrene and pristane to phytane as indicators of depositional environment and lithology of petroleum source rocks. Geochimica Cosmochimica Acta 59 (17), 3581–3598. doi:10.1016/0016-7037(95)00225-o
Johns, W. D. (1979). Clay mineral catalysis and petroleum generation. Annu. Rev. Earth Planet. Sci. 7, 183–198. doi:10.1146/annurev.ea.07.050179.001151
Johnson, K. S. (1989). “Geologic evolution of the Anadarko Basin,” in Anadarko Basin symposium. Editor K. S. Johnson (Oklahoma: Department of the Interior, US Geological Survey), 3–12. Oklahoma Geological Survey, Norman, Oklahoma.
Kirkland, D., Denison, R., Summers, D., Gormly, J., Johnson, K., and Cardott, B. (1992). Geology and organic geochemistry of the Woodford Shale in the Criner Hills and Western Arbuckle Mountains, Oklahoma. Okla. Geol. Surv. Circ. 93, 38–69.
Landis, C. R., and Castaño, J. R. (1995). Maturation and bulk chemical properties of a suite of solid hydrocarbons. Org. Geochem. 22, 137–149. doi:10.1016/0146-6380(95)90013-6
Lewan, M. D., and Ruble, T. E. (2002). Comparison of petroleum generation kinetics by isothermal hydrous and nonisothermal open-system pyrolysis. Org. Geochem. 33, 1457–1475. doi:10.1016/s0146-6380(02)00182-1
Lewan, M. (1985). Evaluation of petroleum generation by hydrous pyrolysis experimentation. Philosophical Trans. R. Soc. Lond. A 315, 123–134.
Mackenzie, A. S., Lamb, N. A., and Maxwell, J. R. (1982). Steroid hydrocarbons and the thermal history of sediments. Nature 259, 223–226. doi:10.1038/295223a0
Meyer, C. A., Silvestri, G. J., and Spencer, R. C. (1983). ASME steam tables: Thermodynamic and transport properties of steam: Comprising tables and charts for steam and water. 5th ed. American Society of Mechanical Engineers, 332.
Miceli Romero, A., and Philp, R. P. (2012). Organic geochemistry of the Woodford Shale, southeastern Oklahoma: How variable can shales be? AAPG Bull. 96, 493–517. doi:10.1306/08101110194
Moldowan, J. M., Dahl, J., Zinniker, D., and Barbanti, S. M. (2015). Underutilized advanced geochemical technologies for oil and gas exploration and production-1. The diamondoids. J. Petroleum Sci. Eng. 126, 87–96. doi:10.1016/j.petrol.2014.11.010
Moldowan, J. M., Seifert, W. K., and Gallegos, E. J. (1983). Identification of an extended series of tricyclic terpanes in petroleum. Geochimica Cosmochimica Acta 47, 1531–1534. doi:10.1016/0016-7037(83)90313-7
Perry, W. J. (1989). Tectonic evolution of the Anadarko Basin region, Oklahoma (No. 1866). Department of the Interior, US Geological Survey. Available at: https://pubs.usgs.gov/bul/1866a/report.pdf
Price, B. J., Pollack, A. C., Lamb, A. P., Peryam, T. C., and Anderson, J. R. (2020). Depositional interpretation and sequence stratigraphic control on reservoir quality and distribution in the Meramecian Sooner trend Anadarko Basin, Canadian, and Kingfisher Counties (STACK) play, Anadarko Basin, Oklahoma, United States. AAPG Bull. 104 (2), 357–386. doi:10.1306/04301917411
Quan, T. M., Adigwe, E. N., Riedinger, N., and Puckette, J. (2013). Evaluating nitrogen isotopes as proxies for depositional environmental conditions in shales: Comparing Caney and Woodford Shales in the Arkoma Basin, Oklahoma. Chem. Geol. 360, 231–240. doi:10.1016/j.chemgeo.2013.10.017
Riolo, J., Hussler, G., Albrecht, P., and Connan, J. (1986). Distribution of aromatic steroids in geological samples: their evaluation as geochemical parameters. Org. Geochem. 10, 981–990. doi:10.1016/s0146-6380(86)80036-5
Rivera, K. T., Puckette, J., and Quan, T. M. (2015). Evaluation of redox versus thermal maturity controls on δ15N in organic rich shales: A case study of the Woodford Shale, Anadarko Basin, Oklahoma, USA. Org. Geochem. 83-84, 127–139. doi:10.1016/j.orggeochem.2015.03.005
Seifert, W. K., and Moldowan, J. M. (1978). Applications of steranes, terpanes and monoaromatics to the maturation, migration and source of crude oils. Geochimica Cosmochimica Acta 42, 77–95. doi:10.1016/0016-7037(78)90219-3
Seifert, W. K., and Moldowan, J. M. (1980). The effect of thermal stress on source-rock quality as measured by hopane stereochemistry. Phys. Chem. Earth 12, 229–237. doi:10.1016/0079-1946(79)90107-1
Srinivasan, P., Jacobi, D., Atwah, I., Karg, H., and Azzouni, A. (2022). Integration of methyldibenzothiophene and pyrolysis techniques to determine thermal maturity in sulfur-rich Type II-S source rocks and oils. Org. Geochem. 163, 104333. doi:10.1016/j.orggeochem.2021.104333
Stasiuk, L. D., and Fowler, M. G. (2004). Organic facies in Devonian and Mississippian strata of Western Canada Sedimentary Basin: relation to kerogen type, paleoenvironment, and paleogeography. Bull. Can. Petroleum Geol. 52 (3), 234–255. doi:10.2113/52.3.234
Wang, H. D., and Philp, R. P. (1997). Geochemical study of potential source rocks and crude oils in the Anadarko Basin, Oklahoma. AAPG Bull. 81, 249–275. doi:10.1306/522B42FD-1727-11D7-8645000102C1865D
Wilson, E. N., Watney, W. L., and Grammer, G. M. (2019). “An overview of the giant heterogeneous Mississippian carbonate system of the midcontinent: Ancient structure, complex stratigraphy, conventional traps, and unconventional technology in a high fluid volume world,” in Mississippian Reservoirs of the midcontinent (Tulsa: AAPG), 1–23.
Keywords: STACK, mudrocks, Woodford Shale, Mississippian carbonate, hydrous pyrolysis, petroleum generation kinetics
Citation: Atwah I and Sweet S (2023) Petroleum generation kinetics of unconventional Mississippian mudrocks in central Oklahoma, United States. Front. Earth Sci. 11:1146251. doi: 10.3389/feart.2023.1146251
Received: 17 January 2023; Accepted: 05 April 2023;
Published: 05 May 2023.
Edited by:
Yingfang Zhou, University of Aberdeen, United KingdomReviewed by:
Omid Haeri-Ardakani, Department of Natural Resources, CanadaSaipeng Huang, Northeast Petroleum University, China
Copyright © 2023 Atwah and Sweet. This is an open-access article distributed under the terms of the Creative Commons Attribution License (CC BY). The use, distribution or reproduction in other forums is permitted, provided the original author(s) and the copyright owner(s) are credited and that the original publication in this journal is cited, in accordance with accepted academic practice. No use, distribution or reproduction is permitted which does not comply with these terms.
*Correspondence: Ibrahim Atwah, SWJyYWhpbS5hdHdhaEBhcmFtY28uY29t