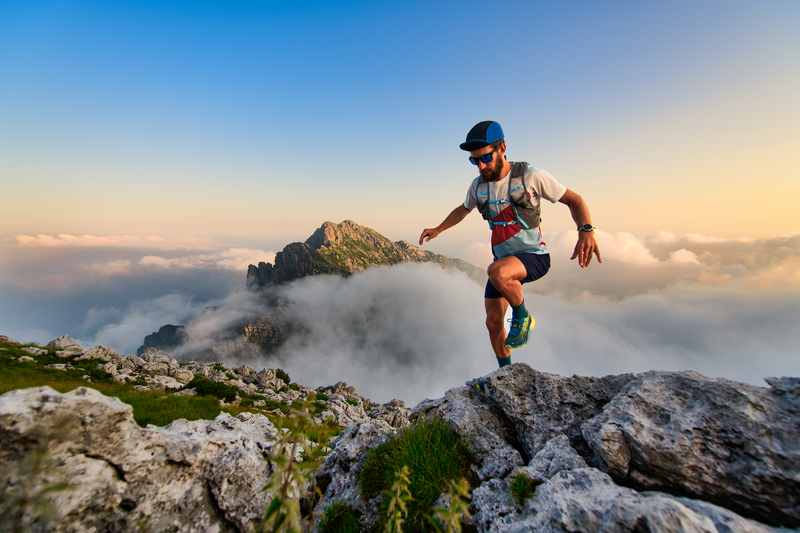
95% of researchers rate our articles as excellent or good
Learn more about the work of our research integrity team to safeguard the quality of each article we publish.
Find out more
ORIGINAL RESEARCH article
Front. Earth Sci. , 27 February 2023
Sec. Volcanology
Volume 11 - 2023 | https://doi.org/10.3389/feart.2023.1116157
This article is part of the Research Topic Linking Subaqueous, Subglacial, and Subaerial Volcanism: How Water Influences Eruption Dynamics and Creation of Volcanic Products View all 10 articles
Many Quaternary stratovolcanoes host (or hosted) glacial ice with volumes that have fluctuated in response to long-term global climate cycles. The repeated advance and retreat of ice in valleys on the flanks of volcanoes throughout their eruptive histories has impacted how and where lava flows are emplaced and preserved. Understanding the dynamics of lava-ice interaction is a vital part of reconstructing the growth histories of many stratovolcanoes and can provide valuable clues about the evolution of Earth’s climate. We have constructed a basic experiment, using common kitchen ingredients and utensils, to replicate the interaction between lava flows and glaciers on stratovolcanoes. This article outlines the ingredients and recipes for soda bread (stratovolcano analogy), ice cream (glacier analogies), and sauce (lava flow analogies), and describes exercises that provide qualitative lessons about the morphology of volcanoes, natural hazards, and paleoclimate. As such, the experiment can be used in geoscience outreach demonstrations for students and will assist non-specialist scientists with undertaking field identification of ice-bounded lava flows.
Many volcanoes are sites of interaction between the hottest (magma) and coldest (ice) natural materials on Earth’s surface. Magmatic and volcanic activity in the presence of ice or snow gives rise to eruptive activity defined under the term of glaciovolcanism (Kelman et al., 2002; Smellie, 2006). In addition to the obvious candidates of Antarctica and Iceland, glaciovolcanism has also occurred extensively at volcanoes that line the edges of continents around the Pacific Ocean (e.g., Mee et al., 2009; Cole et al., 2018; Wilson et al., 2019; Kataoka et al., 2021; Loewen et al., 2021; Figure 1). Subduction zone volcanism throughout Japan, Kamchatka, north-western United States, Central and South America, New Zealand, Papua New Guinea, Indonesia, and the Philippines has typically produced tall composite cones that form as a result of frequent eruptions over long time periods (Davidson and de Silva, 2000). The lofty heights of these volcanic features mean that many of them experience seasonal snowfall under current climate conditions, and ones that are located in the high-latitudes host permanent ice caps and glaciers (e.g., Mount Veniaminof, Alaska). In this “Pacific ring of fire and ice”, even volcanoes in tropical regions such as Papua New Guinea (e.g., Mount Giluwe; Barrows et al., 2011) and Mexico (e.g., Nevado de Toluca; Capra et al., 2013) have experienced glaciation and therefore may have been affected by glaciovolcanism.
FIGURE 1. Locations and examples of arc stratovolcanoes where glaciovolcanism has occurred during the last ∼250,000 years. (A) Map of volcanic arcs (grey lines) around the Pacific Ocean that have been affected by glaciers and heavy snow. Locations are noted for Rainier and Ruapehu volcanoes, and for representative study sites mentioned in the text. (B) Photograph of a glacial valley (Wahianoa River) on the southeast flank of snow-covered Mount Ruapehu in New Zealand. (C) Photograph of the southeast flank of Mount Rainier in Washington, United States, which comprises glaciers and steep ridges of lava. Photographs were provided by Dougal Townsend.
The abundance and extent of glaciers on volcanoes have fluctuated in response to 100,000 years-long global climate cycles over the last ∼700,000 years (Goñi et al., 2019; Coombs and Jicha, 2020). During the Last Glacial Maximum (LGM), around 25,000–20,000 years ago, when the average global temperature was 6°C colder than today (Tierney et al., 2020), large glaciers were present on many volcanoes, including ones that are currently free of permanent ice (Eaves et al., 2016). Signs of past glaciation exist as u-shaped valleys, moraines, and striated lavas (Figure 1B). Within the traditional view of the evolution of stratovolcanoes affected by glaciers (e.g., Hobden et al., 1996), these features record periods of erosion (during relatively cold climate stages) that were distinct in timing from periods of volcanic growth (during relatively warm climate stages). Under this paradigm, ridges of lava on the sides of valleys are inferred to have been carved out by glaciers. This has certainly occurred at numerous volcanoes (e.g., Singer et al., 1997); however, 40Ar/39Ar and K-Ar dating of lavas has also shown that eruptions have occurred during periods when glaciers were extensive in many cases (Fiertsein et al., 2011; Conway et al., 2016; Calvert et al., 2018; Pure et al., 2020). These examples challenge the dichotomy between volcanic growth and glacial erosion and present a key question: what happens when lava is erupted at a stratovolcano that is covered in ice?
Building on pioneering work by Mathews (1952) in British Columbia, a landmark publication by Lescinsky and Sisson (1998) showed that thick lavas located on the tops and sides of ridges at Mount Rainier in Washington, United States (Figure 1C), formed when they flowed down the flanks of the volcano and were confined along the margins of valley-filling glaciers during past cold periods of Earth’s history. The ice deflected the lavas from flowing into valleys, forcing them to pond into thick units along high-elevation parts of the volcano. The glaciers retreated after thousands of years, and left the lavas “perched” on the tops and sides of ridges. Similar landforms have been described at Mount Ruapehu in New Zealand (Figures 1, 2; Spörli and Rowland, 2006; Conway et al., 2015). The steep sides of such outcrops display glassy textures and characteristic fractures that indicate they cooled quickly against water and steam produced by melting of the ice (Figure 2C). Rapid cooling against vertical walls of ice has produced horizontal or curved “columns” on many ice-bounded lavas (Figure 2D), which are formed by the propagation of fractures away from (often complex) cooling surfaces (Lodge and Lescinsky, 2009). The intersection of the fractures at angles of ∼60° forms the characteristic cross-sectional polygons with diameters of ∼10–20 cm (Figure 2C).
FIGURE 2. Examples of ice-bounded lava flows at Mount Ruapehu, New Zealand. (A) Thick lavas on the northern ridge of the Mangaturuturu valley on the southwestern flank, which were emplaced next to glacial ice between ∼45–20 ka. Red arrows point to “knuckles” of lava. Photograph provided by Dougal Townsend. (B) Column-forming joints on the margin of an ice-bounded lava flow on the western flank. Photograph provided by Dougal Townsend. (C) Cross-section view of “columns” with diameters of ca. 10 cm on an ice-bounded lava flow on the southeastern flank. (D) Fanning column-forming joints in an ice-bounded lava flow on the northwest flank. People are standing 15 m apart.
As forecast by Lescinsky and Sisson (1998), ice-bounded lavas have now been identified at several stratovolcanoes around the Pacific (e.g., Mee et al., 2009; Conway et al., 2015; Lachowycz et al., 2015; Coombs and Jicha, 2020; Mixon et al., 2021). Although the mechanism of their formation is well-defined in theory, it remains difficult to grasp the dynamics of lava-ice interaction without direct observation of such an eruption. Emplacement of lava flows onto snow (Edwards et al., 2015) and ice caps (Loewen et al., 2021) has been documented, and large-scale experiments using basaltic melt have been undertaken in the last decade (Edwards et al., 2013). Even with these reference points, identification of ice-bounded lava flows during field surveys requires a certain level of intuitive assessment via real-time reconstruction of past environments. There are many circum-Pacific stratovolcanoes with overlapping eruption and glacial histories where lava-ice interaction has not been documented. The experiment described in this contribution is designed to convey the stratigraphic and morphological characteristics of ice-bounded lavas, which will assist field geologists with identifying these features in such settings.
Active learning methods have been shown to be a successful teaching tool for secondary and tertiary school science subjects (Hake, 1998). Food-based experiments have been particularly popular within this approach, likely because they use inexpensive items and kitchens can be portrayed as accurate representations of scientific laboratories, where hypotheses are tested by carrying out reproducible methods. In Japan, the recipes of “Volcano Kitchen” have been a popular source for outreach activities that utilize common ingredients to teach lessons in volcanology (Hayashi, 2006). A recently published children’s book based on the August 2021 eruption of Fukutoku-Okanoba volcano has also incorporated cooking to introduce primary school students to the petrology of volcanic rocks (Maruya, 2022). Baked goods and candies that look like rocks and minerals are commonly found at geopark visitor centres throughout Japan, and have been successful for introducing domestic and foreign tourists to the textures of rocks in the geologically diverse country. Other examples from around the world include the M&M® magma chamber for simulating fractional crystallization (Wirth, 2003) and the use of fudge and syrup for explaining lava flow rheology (Rust et al., 2008). Whereas precise scaling of natural phenomena is required for analogue modelling (Galland et al., 2006), analogy experiments (after Ichihara, 2023), including the one presented in this contribution, can still convey valuable information with approximate scaling of physical parameters.
Providing a pathway for students to see geoscience as an option for further study and work is indeed a worthwhile activity. Building sustainable and resilient societies will rely on future generations tackling the major issues of climate change mitigation, renewable energy generation, mineral extraction, and natural hazard assessment. Introducing geoscience research to primary and secondary school students (ages 5–18 years) may encourage them to pursue further study of science, technology, engineering, and mathematics (STEM) subjects at university and a career aimed at tackling these challenges. The aim of this contribution is to showcase an analogy experiment of a common glaciovolcanic process (i.e., lava-ice interaction), that can be utilized to introduce the topics of climate change and volcanic hazards to students and non-specialist audiences. The common ingredients and basic kitchen tools make this exercise widely applicable, and the hands-on format is designed to encourage laboratory research via active participation in school classrooms and outreach demonstrations for all ages.
The ingredients and equipment required to make the analogy experiment materials are listed in Table 1, and described herein. Instructions for the experiment are outlined in this section and are also provided in the Supplementary Material.
A basic soda bread is used as the stratovolcano analogy (hereafter, Volcano) in the experiment. Sift 3 cups (360 g) of wheat flour and 3 teaspoons (12 g) of baking powder into a large bowl and mix. Pour 100 mL of soda water (also known as sparkling water) into the bowl at first, and stir into the dry ingredients. Add more soda water by increments of 10 mL until the mixture becomes flaky. Push the flaky parts together with your hands to form a dough, and knead by pressing and folding it over repeatedly. If the dough is not sticking together when you fold it, add some more soda water until it becomes a cohesive ball. If the dough is sticking to your hands, add some more flour until you can handle it easily. Spread the dough out into a broad cone, by pushing down the edges. Place the dough on a lightly greased oven-proof plate. Score the top of the dough by making four ∼1 cm-deep incisions from near the edges to within 2 cm of the centre, at 90° to each other. Melt the butter and rub it over the top of the dough. The oven or microwave (using the oven setting) should be pre-heated to 200°C, prior to putting in the plate with the dough to bake for 30 min at 200°C. Take out the bread and leave to cool for approximately 10 min. Cut around the score marks to form “valleys” that are 2–3 cm-wide and ∼5 cm-long.
Two gelatinous chocolate sauces are used as lava flow analogies in the experiment. To make the first lava flow analogy (hereafter, Lava 1), place 5 g of thickener (powdered collagen gelatine) in a cup and add ∼10 mL of hot water, plus 5 g of dark chocolate and 15 g of white chocolate. Heat in a microwave for 40 s (∼700 W power), then stir thoroughly to form a thick sauce with even consistency. Repeat the steps using 20 mg of dark chocolate, 5 g of thickener and 10–15 mL of hot water to make Lava 2 in a separate cup. Lava 2 should be slightly less viscous than Lava 1 for the purposes of the experiment.
Buy a small container of light-colored plain milk-based ice cream (e.g., vanilla) to use as the glacier analogies (hereafter, Glaciers) in the experiment.
Any carbonated drink can be used as an alternative to soda water. The authors have successfully made the bread volcano using ginger ale, cola, and beer (including non-alcoholic beer). To modify the taste of the bread, add 1–2 teaspoons of salt, and/or 1–2 tablespoons of sugar to the dry ingredients. Milk can be used as an alternative to water when making the sauces.
The ingredients contain gluten, dairy, and sugar. Please check the dietary restrictions of participants if you choose to share the bread, ice cream and chocolate as snacks to eat after the experiment. We do not recommend eating the sauce or ice cream used during the experiment. Instead, consume the remaining chocolate and ice cream ingredients.
The experiment can be undertaken after the analogy materials have been constructed (Volcano, Lava 1 and Lava 2, and Glaciers). There are 3 main steps within the experiment, each representing a key stage in the evolution of a glaciated stratovolcano: 1) Syn-glacial; 2) deglacial; and 3) post-glacial.
The aim of syn-glacial stage of the experiment is to reproduce lava flow eruptions that occurred during glacial stages when ice extent was advanced on stratovolcanoes (e.g., during the LGM). Add Glaciers to the valleys on the Volcano. The Glaciers should be thickest in the middle of the valleys, and sit just below the height of the flanks at the heads and sides of the valleys. Leave an area with no Glaciers on the top of the Volcano to represent the summit vent area. Pour Lava 1 onto this summit area at an approximate rate of 2–4 mL s−1, from a height of 2 cm. Vary the starting point of these lava flows on the summit so that Lava 1 moves down onto different sides of the Volcano. Lava 1 should flow down along the ice-free parts of the Volcano, and into the gap between the sides of the valleys and their Glaciers (Figures 3A, B). Leave the Lava 1 flows to cool down and harden.
FIGURE 3. The experiment. (A,B) Syn-glacial lava flows (s) were deflected by glaciers (g) and emplaced along ridges during the glacial stage. (C,D) Post-glacial lavas (p) flowed over older lavas and into ice-free valleys after deglaciation.
Whilst making Lava 2, leave the Glaciers to melt and then scoop away the remainder carefully from the valleys. This represents the deglacial stage of the experient. Try not to disturb the Lava 1 flows that have set along the tops and sides of the valleys.
The post-glacial stage of the experiment is designed to replicate the effusion of lava flows on an ice-free stratovolcano following deglaciation. Pour Lava 2 at the same rate onto the top of the volcano in increments and from different starting points again. This time, Lava 2 flows should travel over the syn-glacial stage Lava 1 flows and move into the bottom of the valleys (Figures 3C, D).
Herein, we highlight the similarities between the experimental products and real examples of ice-bounded lava flows, and note aspects of lava-ice interaction at volcanoes that are not captured by the experiment, and vice versa. Eruptions that have produced ice-bounded lava flows at Ruapehu and Rainier volcanoes started from vents that were covered by ice at their summits. It is envisioned that such vents eventually became ice-free as eruptive products piled up during the onset of an eruption, allowing lavas to travel through or beside glaciers via meltwater channels created by lava-ice interaction (Lescinsky and Fink, 2000). In the experiment, Lava 1 was poured onto the ice-free summit part of the Volcano for simplicity during the syn-glacial stage, rather than being pushed up from beneath the Glaciers. Similarly, Lava 2 was poured onto the top of the Volcano during the post-glacial stage, after the Glaciers had been removed.
Lava 1, which flowed along the margins of the Glaciers, remained perched on the sides of valleys after the Glaciers were removed during the deglacial stage (Figure 3). These features are close replicants of ice-bounded lava flows at Mount Ruapehu, New Zealand (Figures 2, 4). At Ruapehu, and other stratovolcanoes around the margins of the Pacific Ocean (Figure 1A), ice-bounded lavas stand out as thick (>20 m-thick) and steep-sided units on the tops and sides of ridges adjacent to glacial valleys (Figures 4A, C; Lescinsky and Sisson, 1998), and as broad ice-dammed lavas within valleys (Figures 4B, D; Conway et al., 2015). They can show local variations in thickness along ridges, as their morphology was governed by the shape of the ice wall against which they were impounded. This can be seen in some examples at Ruapehu where lavas are relatively thin along the crest of ridges and thicken considerably into “knuckles” toward the valley (Figure 2A). These intricacies are not reproduced by the small-scale experiment, nor are the fine-scale cooling fractures, such as decimetre-scale column-forming joints, that are produced by rapid quenching of lava against meltwater and steam at the edges of glaciers (Figure 2C; Lescinsky and Fink, 2000; Mee et al., 2009; Lodge and Lescinsky, 2009; Conway et al., 2015). While fracturing and fragmentation of Lava 1 will not occur in the experiment, one might see mixing of melted ice cream and the lavas. Such features resemble ice-melt lahars generated by lava-ice interaction, which can be fatal phenomena produced at ice-capped stratovolcanoes (Pierson et al., 1990; Smellie, 2022). Ice-bounded lavas on volcanoes may undergo erosion through rockfall, because their steep and finely fractured margins are vulnerable to collapse. They may also be affected by subsequent re-advance of glaciers. These aspects are not captured by the experiment; following the removal of the Glaciers, Lava 1 flows will remain in-place where they hardened along the sides of the valleys.
FIGURE 4. Comparison of real lava flows at Mount Ruapehu and experimental products. (A,C) Post-glacial lavas (p) flowed over and around older syn-glacial lavas (s). (B,D) Thick and wide syn-glacial lavas (s) were impounded by glaciers within valleys. Photograph (A) was provided by Dougal Townsend.
After removal of the Glaciers, Lava 2 flowed over and around the syn-glacial Lava 1 to build up the top of the Volcano and moved into the valleys during the post-glacial stage of the experiment (Figures 3C, D). These characteristics are commonly observed for Holocene lavas on volcanoes that erupted during the current interglacial period. Such flows often appear thin and rubbly on the tops of ridges because they were not contained or impounded by obstacles, as opposed to ice-bounded flows (Figures 4A, C). This feature was emphasized in the experiment by using a greater amount of water to ensure that Lava 2 was less viscous than Lava 1.
There are 138 active arc stratovolcanoes with glaciers on Earth (Edwards et al., 2020). Many more stratovolcanoes hosted glaciers during past cold periods such as the LGM, but are currently free of permanent ice (e.g., Eaves et al., 2016). Glaciers have therefore been a key part of the life cycles of many volcanoes, and will continue to impact eruptions in the future. As fluctuations in the volumes of glaciers on human timescales become apparent (Vargo et al., 2020), it is pertinent to be aware of how glacier extents have changed over longer timescales in the past. Examining the links between volcanism and the cryosphere that arose from these past changes is important for forecasting future eruptive activity.
Stratovolcanoes grow into large constructional structures over time periods of several 102 to 105 years due to the repetitive emplacement of lava and tephra onto their flanks (Davidson and de Silva, 2000; Yamamoto et al., 2018; Christiansen et al., 2020). Whereas symmetrical cones such as Mount Fuji are often presented as icons of arc stratovolcanoes, many are in fact asymmetrical and complex structures (Figures 1B, C). The latter types exist because volcanoes also undergo destruction throughout their lifetimes, via sector collapse and erosion. Ice is a key agent for both collapse and erosion at volcanoes that have been affected by glaciers. Retreating glaciers promote edifice collapse by destabilising steep and weak units of volcanic rock (Capra, 2006), whereas advancing glaciers scour out valleys and push eroded material onto the ring plains that surround volcanoes (Eaves et al., 2016). Ice also acts to modify the preservation and distribution of volcanic rocks on stratovolcanoes during eruptions, as shown by the experiment (Figure 3). Understanding how glaciers have affected the preservation of volcanic deposits is vital, because these deposits provide a necessary record of past volcanic activity and, therefore, a framework for the possible timing and types of future eruptions at active volcanoes.
The most important message conveyed by the experiment is that many of the lavas observed on the sides of valleys exist because glaciers deflected them onto those locations during eruptions, rather than having exposed them via erosion after they were emplaced onto ice-free flanks. Syn-glacial lavas exhibit an increase in thickness towards the valley and characteristic cooling fractures on their margins that indicate they were impounded and chilled by valley-filling ice (Figure 2; Figure 5A). Post-glacial lavas generally exhibit more uniform thicknesses, with no evidence for impedance of their flow towards lower elevations by a morphological barrier, and will lack evidence for rapid cooling (Figure 5A). A major implication of identifying ice-bounded lavas is that stratovolcanoes can be continuously active during their lifetimes. This differs from the traditional model of stratovolcano evolution that states that eruptions and edifice growth occur during interglacial stages and relative inactivity and erosion occur during glacial stages.
FIGURE 5. Stratigraphic features of pre-, syn-, and post-glacial lava lavas (pre, s, p) at Mount Ruapehu, New Zealand. (A) The syn-glacial lava exhibits a thick and uneven morphology, and is younger than the lower pre-glacial lava, which is a uniformly thin unit that has been eroded by glacial (and fluvial) action. (B) This post-glacial lava flowed into the valley and was not ponded along the ridge. (C) The post-glacial lava is younger than the syn-glacial lava in this photo. Although it looks like (p) is outcropping from beneath (s) at the location marked by the arrow, the post-glacial lava flowed into the valley after retreat of the ice that buttressed the syn-glacial lava.
Age data for ice-bounded lava flows record more than just the timing of an eruption; they provide in situ constraints of the past presence and extent of ice in alpine settings (Lescinsky and Fink, 2000). Conversely, ages for post-glacial lavas that flowed into valleys provide constraints for the timing of glacier retreat (Figure 5B; Conway et al., 2015). These constraints are valuable for reconstructing the past climate and environments of Earth. Despite these useful products, the influence of ice on the distribution of lava flows during eruptions also produces complex stratigraphic relationships that can be difficult to unravel. Post-glacial lava flows may appear to be stratigraphically lower than syn-glacial ice-bounded lavas in the field, although they are younger in age (Figure 5C). This apparent inversion of stratigraphy is important to recognize when documenting the eruptive history of a stratovolcano, and using geochronology to measure absolute ages for volcanic units is useful for testing such stratigraphic relationships in the field.
Studying the differences in volumes and compositions of syn-versus post-glacial lavas and tephras (explosive eruption deposits) also offers the opportunity to investigate whether the addition/removal of ice masses on volcanoes affect their underlying magmatic systems. Geological and geochemical evidence from Iceland indicates that deglaciation after the LGM resulted in an increase in rates of volcanism because the removal of ice on the crust resulted in increased production and eruption of new magma (Maclennan et al., 2002). It remains unclear whether deglaciation leads to increased eruption rates at arc stratovolcanoes (Watt et al., 2013; Conway et al., 2016; Pure et al., 2020), although a few studies have interpreted that eruption dynamics at some volcanoes were influenced by the retreat of ice following the LGM in Chile (Rawson et al., 2016). With glacier volumes in many locations currently decreasing in response to anthropogenic climate change (Vargo et al., 2020), it is critical to understand the links between deglaciation and volcanism in more detail so that we can forecast how the melting of glaciers and ice sheets will affect eruptive activity in the future (Tuffen, 2010).
Additional hazards related to volcano-ice interaction include sector collapses and lahars. A large landslide in 2010 at Mount Meager in southwest Canada was prompted by glacial retreat and melting of snow (Roberti et al., 2018). Meltwater lahars are an extremely hazardous phenomenon produced at snow- and ice-clad stratovolcanoes (Pierson et al., 1990; Uesawa, 2014; Smellie, 2022). Snowy volcanoes are popular tourist locations for winter activities, so constraining rates of meltwater production (Cole et al., 2021) and modelling the travel pathways for lahars (Kataoka et al., 2021) represent vital research objectives and hazard mitigation tools in volcanology.
The coexistence of magma and ice at glaciated stratovolcanoes make them hotspots to learn about cool geological ideas. Lava-ice interaction has produced stunning outcrops of volcanic rock (Figure 2), which record valuable information about the evolution of volcanoes and Earth’s climate. These visually striking features can serve as icons for geoscience education, to increase future generations’ interest in mitigating climate change and natural hazards. Background information and the key lessons about volcanoes and glaciers that the experiment highlights are summarized below.
1 Global climate variability has produced repetitive 100,000 years-long cycles of glacial advance and retreat over the last ∼700,000 years. Ice sheets and glaciers grew during the last glacial period, and had well-advanced extents during the LGM around 20 ka, when the average global temperature was 6°C lower than today.
2 As glaciers have advanced and retreated due to these long-term climate cycles, they have repeatedly carved out valleys on many long-lived arc stratovolcanoes with high summit elevations, such as many of those located around the margins of the Pacific Ocean.
3 If effusive eruptions occur when valleys are filled with ice, lava is deflected toward the sides of the valleys and becomes ponded and chilled along ridges adjacent to glaciers. This process creates thick lava flows that remain perched on the tops and sides of ridges after the glaciers retreat. Ice-bounded lava flows often exhibit horizontally oriented column-forming joints that indicate that the lava cooled quickly next to the vertical margins of glaciers.
4 If eruptions occur when valleys are not filled with ice, lava will flow into valleys and become emplaced at lower elevations on volcanoes. These lavas will be thinner along ridges than ice-bounded lavas.
5 Measuring the ages, volumes, and compositions of syn- and post-glacial lava flows and tephras (explosive eruption products) has several major implications for understanding the evolution of our planet. Ages for ice-bounded and valley-filling lavas provide valuable constraints on the timing of past glacial advance and retreat, respectively. Comparing the volumes and composition of syn-glacial lavas and tephras with those of post-glacial lavas and tephras allows investigation of whether the addition and removal of ice masses on top of volcanoes affects the behaviour of the underlying magma systems.
6 Interactions between volcanoes and ice/snow pose major risks for nearby populations. Explosive eruptions, landslides, and lahars are examples of hazardous phenomena produced at ice- and snow-clad volcanoes. Further research is required to understand how eruptive activity will be affected by ice sheet and glacier decay associated with climate change in the future, and how society can build resilience to volcanic hazards at ice- and snow-clad volcanoes.
This article has described a classroom exercise that conveys lessons about the morphology of volcanoes, natural hazards, and paleoclimate by using a small-scale kitchen experiment with easily accessible ingredients and apparatus. Simple recipes for soda bread (stratovolcano analogy), ice cream (glacier analogies), and sauce (lava analogies) are outlined along with instructions for how to use them to replicate the interaction between lava flows and ice on stratovolcanoes. The experiment conveys the process of lava-ice interaction, whereby effusive eruptions during glacial periods lead to the deflection and ponding of lava flows on the ridges adjacent to ice-filled valleys. Recognition of the products of this process is vital for understanding the eruption histories of glaciated stratovolcanoes, and can inform paleoclimate reconstructions by providing valuable constraints on the past extent and timing of glacial advances. This is a qualitative experiment designed to equip students and non-specialist scientists with the understanding to undertake field identification of ice-bounded lava flows. Moreover, we hope that this exercise generates interest in the related themes of climate change and volcanic hazards, and encourages students to consider careers in science.
The datasets presented in this study can be found in online repositories. The names of the repository/repositories and accession numbers can be found in the article/Supplementary Material.
CC conceptualized the experiment. It was first trialled by CC, KT, and TS at the National Museum of Nature and Science in Tsukuba, Japan, and first utilized in a classroom experiment conducted by CC at Utsunomiya Girls’ High School in 2017. CC and KM carried out the recorded version of the experiment. All authors contributed to trialling and refining the recipes and experiment, and to the writing of the manuscript.
CC and OI were supported by JSPS-RSNZ bilateral research grant JPJSBP120211003. National Geographic Society Explorer Grant CP-114R-17 helped CC, KT, and OI to pursue glaciovolcanism research activities in Japan.
The bread recipe described in this article was modified from one provided to CC by Patsy Sziranyi and Esther Conway, with additional baking advice from Patrick Conway. We are very grateful to Dougal Townsend (GNS Science) for providing photographs. CC thanks Andrew Calvert, Rosie Cole, Carolyn Driedger, Shaun Eaves, John Gamble, Graham Leonard, Leo Pure, Thomas Sisson, Dougal Townsend, James Vallance, James White, and Colin Wilson for valuable discussions during field surveys at Ruapehu and Rainier volcanoes. Thank you to John Smellie, Valerio Acocella, and the two reviewers for very helpful comments.
The authors declare that the research was conducted in the absence of any commercial or financial relationships that could be construed as a potential conflict of interest.
All claims expressed in this article are solely those of the authors and do not necessarily represent those of their affiliated organizations, or those of the publisher, the editors and the reviewers. Any product that may be evaluated in this article, or claim that may be made by its manufacturer, is not guaranteed or endorsed by the publisher.
The Supplementary Material for this article can be found online at: https://www.frontiersin.org/articles/10.3389/feart.2023.1116157/full#supplementary-material
Barrows, T. T., Hope, G. S., Prentice, M. L., Fifield, L. K., and Tims, S. G. (2011). Late pleistocene glaciation of the Mt Giluwe volcano, Papua New Guinea. Quat. Sci. Rev. 30, 2676–2689. doi:10.1016/j.quascirev.2011.05.022
Calvert, A. T., Fierstein, J., and Hildreth, W. (2018). Eruptive history of middle sister, Oregon cascades, USA–product of a late pleistocene eruptive episode. Geosphere 14, 2118–2139. doi:10.1130/GES01638.1
Capra, L. (2006). Abrupt climatic changes as triggering mechanisms of massive volcanic collapses. J. Volcanol. Geotherm. Res. 155, 329–333. doi:10.1016/j.jvolgeores.2006.04.009
Capra, L., Bernal, J. P., Carrasco-Núñez, G., and Roverato, M. (2013). Climatic fluctuations as a significant contributing factor for volcanic collapses. Evidence from Mexico during the Late Pleistocene. Glob. Planet. Change. 100, 194–203. doi:10.1016/j.gloplacha.2012.10.017
Christiansen, R. L., Calvert, A. T., Champion, D. E., Gardner, C. A., Fierstein, J. E., and Vazquez, J. A. (2020). The remarkable volcanism of Shastina, a stratocone segment of Mount Shasta, California. Geosphere 16, 1153–1178. doi:10.1130/GES02080.1
Cole, R. P., White, J. D. L., Conway, C. E., Leonard, G. S., Townsend, D. B., and Pure, L. R. (2018). The glaciovolcanic evolution of an andesitic edifice, South Crater, Tongariro volcano, New Zealand. J. Volcanol. Geotherm. Res. 352, 55–77. doi:10.1016/j.jvolgeores.2017.12.003
Cole, R. P., White, J. D. L., Dürig, T., Büttner, R., Zimanowski, B., Bowman, M. H., et al. (2021). Controls on andesitic glaciovolcanism at ice-capped volcanoes from field and experimental studies. Geology 49, 1069–1073. doi:10.1130/G48735.1
Conway, C. E., Townsend, D. B., Leonard, G. S., Wilson, C. J. N., Calvert, A. T., and Gamble, J. A. (2015). Lava-ice interaction on a large composite volcano: A case study from Ruapehu, New Zealand. Bull. Volcanol. 77 (21), 21. doi:10.1007/s00445-015-0906-2
Conway, C. E., Leonard, G. S., Townsend, D. B., Calvert, A. T., Wilson, C. J. N., Gamble, J. A., et al. (2016). A high-resolution 40Ar/39Ar lava chronology and edifice construction history for Ruapehu volcano, New Zealand. J. Volcanol. Geotherm. Res. 327, 152–179. doi:10.1016/j.jvolgeores.2016.07.006
Coombs, M. L., and Jicha, B. R. (2020). The eruptive history, magmatic evolution, and influence of glacial ice at long-lived Akutan volcano, eastern Aleutian Islands, Alaska, USA. Geol. Soc. Amer. Bull. 133, 963–991. doi:10.1130/B35667.1
Davidson, J., and de Silva, S. (2000). “Composite volcanoes,” in Encyclopedia of volcanoes. Editor H. Sigurdsson (London: Academic Press), 663–682.
Eaves, S. R., Mackintosh, A. N., Anderson, B. M., Doughty, A. M., Townsend, D. B., Conway, C. E., et al. (2016). The last glacial Maximum in the central north island, New Zealand: Palaeoclimate inferences from glacier modelling. Clim. Past. 12, 943–960. doi:10.5194/cp-12-943-2016
Edwards, B. R., Karson, J., Wysocki, R., Lev, E., Bindeman, I., and Kueppers, U. (2013). Insights on lava–ice/snow interactions from large-scale basaltic melt experiments. Geology 41, 851–854. doi:10.1130/G34305.1
Edwards, B. R., Belousov, A., Belousova, M., and Melniko, D. (2015). Observations on lava, snowpack and their interactions during the 2012–13 Tolbachik eruption, Klyuchevskoy Group, Kamchatka, Russia. J. Volcanol. Geotherm. Res. 307, 107–119. doi:10.1016/j.jvolgeores.2015.08.010
Edwards, B. R., Kochtitzky, W., and Battersby, S. (2020). Global mapping of future glaciovolcanism. Glob. Planet. Change 195, 103356. doi:10.1016/j.gloplacha.2020.103356
Fiertsein, J., Hildreth, W., and Calvert, A. T. (2011). Eruptive history of South sister, Oregon cascades. J. Volcanol. Geotherm. Res. 207, 145–179. doi:10.1016/j.jvolgeores.2011.06.003
Galland, O., Cobbold, P. R., Hallot, E., de Bremond d’Ars, J., and Delavaud, G. (2006). Use of vegetable oil and silica powder for scale modelling of magmatic intrusion in a deforming brittle crust. Earth Planet. Sci. Lett. 3, 786–804. doi:10.1016/j.epsl.2006.01.014
Goñi, M. F. S., Ferretti, P., Polanco-Martínez, J. M., Rodrigues, T., Alonso-García, M., Rodríguez-Tovar, F. J., et al. (2019). Pronounced northward shift of the westerlies during MIS 17 leading to the strong 100-kyr ice age cycles. Earth Plan. Sci. Lett. 511, 117–129. doi:10.1016/j.epsl.2019.01.032
Hake, R. R. (1998). Interactive-engagement versus traditional methods: A six-thousand-student survey of mechanics test data for introductory physics courses. Amer. J. Phys. 66, 64–74. doi:10.1119/1.18809
Hayashi, S. (2006). The world’s most delicious book on volcanoes: Eruption experiments using chocolate and cocoa powder. Komine Shoten 127. (in Japanese). ISBN-13:978-4338186087.
Hobden, B. J., Houghton, B. F., Lanphere, M. A., and Nairn, I. A. (1996). Growth of the tongariro volcanic complex: New evidence from K-Ar age determinations. New zeal. J. Geol. geophys. 39, 151–154. doi:10.1080/00288306.1996.9514701
Ichihara, M. (2023). Understanding the flow-to-fracture transition of volcanic fluids through analogy experiments. Sci. Assembly Int. Assoc. Volcanol. Chem. Earth’s Interior. Abstract number 1489.
Kataoka, K. S., Tsunematsu, K., Matsumoto, T., Urabe, A., and Kawashima, K. (2021). Crisis hazard assessment for snow-related lahars from an unforeseen new vent eruption: The 2018 eruption of kusatsu-shirane volcano, Japan. Earth Planets Space 73 (220), 220. doi:10.1186/s40623-021-01522-0
Kelman, M. C., Russell, J. K., and Hickson, C. J. (2002). Effusive intermediate glaciovolcanism in the garibaldi volcanic belt, southwestern British Columbia, Canada. Chapman. Geol. Soc. Lond. Spec. Pub 202, 195–211. Volcano-ice interaction on Earth and Mars. Edited by J. L. Smellie and M. G. doi:10.1144/GSL.SP.2002.202.01.10
Lachowycz, S. M., Pyle, D. M., Gilbert, J. S., Mather, T. A., Mee, K., Naranjo, J. A., et al. (2015). Glaciovolcanism at volcán sollipulli, southern Chile: Lithofacies analysis and interpretation. J. Volcanol. Geotherm. Res. 303, 59–78. doi:10.1016/j.jvolgeores.2015.06.021
Lescinsky, D. T., and Fink, J. H. (2000). Lava and ice interaction at stratovolcanoes: Use of characteristic features to determine past glacial extents and future volcanic hazards. J. Geophys. Res. Sol. Earth 105, 23711–23726. doi:10.1029/2000JB900214
Lescinsky, D. T., and Sisson, T. W. (1998)., 26. Washington, 351–354. doi:10.1130/0091-7613(1998)026<0351:RFIBLF>2.3.CO;2Ridge-forming, ice-bounded lava flows at Mount Rainier, WashingtonGeology
Lodge, R. W. D., and Lescinsky, D. T. (2009). Fracture patterns at lava–ice contacts on Kokostick Butte, OR, and Mazama Ridge, Mount Rainier, WA: Implications for flow emplacement and cooling histories. J. Volcanol. Geotherm. Res. 185, 298–310. doi:10.1016/j.jvolgeores.2008.10.010
Loewen, M. W., Dietterich, H. R., Graham, N., and Izbekov, P. (2021). Evolution in eruptive style of the 2018 eruption of Veniaminof volcano, Alaska, reflected in groundmass textures and remote sensing. Bull. Volcanol. 83 (72), 72. doi:10.1007/s00445-021-01489-6
Maclennan, J., Jull, M., McKenzie, D., Slater, L., and Grönvold, K. (2002). The link between volcanism and deglaciation in Iceland. Geochem. Geophys. Geosyst. 3, 1–25. doi:10.1029/2001GC000282
Mathews, W. H. (1952). Ice-dammed lavas from clinker mountain, southwestern British Columbia. Amer. J. Sci. 250, 553–565. doi:10.2475/ajs.250.8.553
Mee, K., Gilbert, J. S., McGarvie, D. W., Naranjo, J. A., and Pringle, M. S. (2009). Palaeoenvironment reconstruction, volcanic evolution and geochronology of the Cerro Blanco subcomplex, Nevados de Chillán volcanic complex, central Chile. Bull. Volcanol. 71, 933–952. doi:10.1007/s00445-009-0277-7
Mixon, E. E., Singer, B. S., Jicha, B. R., and Ramirez, A. (2021). Calbuco, a monotonous andesitic high-flux volcano in the Southern Andes, Chile. J. Volcanol. Geotherm. Res. 416, 107279. doi:10.1016/j.jvolgeores.2021.107279
Pierson, T. C., Janda, R. J., Thouret, J.-C., and Borrero, C. A. (1990). Perturbation and melting of snow and ice by the 13 November 1985 eruption of Nevado del Ruiz, Colombia, and consequent mobilization, flow and deposition of lahars. J. Volcanol. Geotherm. Res. 41, 17–66. doi:10.1016/0377-0273(90)90082-Q
Pure, L. R., Leonard, G. S., Townsend, D. B., Wilson, C. J. N., Calvert, A. T., Cole, R. P., et al. (2020). A high resolution 40Ar/39Ar lava chronology and edifice construction history for Tongariro volcano, New Zealand. J. Volcanol. Geotherm. Res. 403, 106993. doi:10.1016/j.jvolgeores.2020.106993
Rawson, H., Pyle, D. M., Mather, T. A., Smith, V. C., Fontijn, K., Lachowycz, S. M., et al. (2016). The magmatic and eruptive response of arc volcanoes to deglaciation: Insights from southern Chile. Geology 44, 251–254. doi:10.1130/G37504.1
Roberti, G., Ward, B., van Wyk de Vries, B., Friele, P., Perotti, L., Clague, J. J., et al. (2018). Precursory slope distress prior to the 2010 Mount Meager landslide, British Columbia. Landslides 15, 637–647. doi:10.1007/s10346-017-0901-0
Rust, A., Cashman, K., and Wright, H. (2008). Fudge factors in lessons on crystallization, rheology and morphology of basalt lava flows. J. Geosci. Educ. 56, 73–80. doi:10.5408/1089-9995-56.1.73
Singer, B. S., Thompson, R. A., Dungan, M. A., Feeley, T. C., Nelson, S. T., Pickens, J. C., et al. (1997). Volcanism and erosion during the past 930 k.y. at the Tatara–San Pedro complex, Chilean Andes. Geol. Soc. Bull. Amer. 109, 127–142. doi:10.1130/0016-7606(1997)109<0127:VAEDTP>2.3.CO;2
Smellie, J. L. (2022). “Sedimentation associated with glaciovolcanism: A review. Volcanic processes in the sedimentary record: When volcanoes meet the environment, Geol. Soc. Lond. Spec. Pub, 520, 1–43. doi:10.1144/SP520-2021-135
Smellie, J. L. (2006). The relative importance of supraglacial versus subglacial meltwater escape in basaltic subglacial tuya eruptions: An important unresolved conundrum. Earth-Sci. Rev. 74, 241–268. doi:10.1016/j.earscirev.2005.09.004
Spörli, K. B., and Rowland, J. V. (2006). ‘Column on column’ structures as indicators of lava/ice interaction, Ruapehu andesite volcano, New Zealand. J. Volcanol. Geotherm. Res. 157, 294–310. doi:10.1016/j.jvolgeores.2006.04.004
Tierney, J. E., Zhu, J., King, J., Malevich, S. B., Hakim, G. J., and Poulsen, C. J. (2020). Glacial cooling and climate sensitivity revisited. Nature 584, 569–573. doi:10.1038/s41586-020-2617-x
Tuffen, H. (2010). How will melting of ice affect volcanic hazards in the twenty-first century? Phil. Trans. Roy. Soc. A 368, 2535–2558. doi:10.1098/rsta.2010.0063
Uesawa, S. (2014). A study of the Taisho lahar generated by the 1926 eruption of Tokachidake Volcano, central Hokkaido, Japan, and implications for the generation of cohesive lahars. J. Volcanol. Geotherm. Res. 270, 23–34. doi:10.1016/j.jvolgeores.2013.11.002
Vargo, L. J., Anderson, B. M., Dadíc, R., Horgan, H. J., Mackintosh, A. N., King, A. D., et al. (2020). Anthropogenic warming forces extreme annual glacier mass loss. Nat. Clim. Change 10, 856–861. doi:10.1038/s41558-020-0849-2
Watt, S. F. L., Pyle, D. M., and Mather, T. A. (2013). The volcanic response to deglaciation: Evidence from glaciated arcs and a reassessment of global eruption records. Earth-Sci. Rev. 122, 77–102. doi:10.1016/j.earscirev.2013.03.007
Wilson, A. M., Russell, J. K., and Ward, B. C. (2019). Paleo-glacier reconstruction in southwestern British Columbia, Canada: A glaciovolcanic model. Quat. Sci. Rev. 218, 178–188. doi:10.1016/j.quascirev.2019.06.024
Wirth, K. R. (2003). Using an M&M® magma chamber to illustrate magmatic differentiation. Geol. Soc. Amer. Ann. Meet., 120–218.
Keywords: glaciovolcanism, stratovolcano, lava-ice interaction, climate change, geoscience outreach
Citation: Conway CE, Tani K, Sano T, Matsumoto K and Ishizuka O (2023) A kitchen experiment for replicating lava-ice interaction on stratovolcanoes. Front. Earth Sci. 11:1116157. doi: 10.3389/feart.2023.1116157
Received: 05 December 2022; Accepted: 15 February 2023;
Published: 27 February 2023.
Edited by:
John Smellie, University of Leicester, United KingdomReviewed by:
Adelina Geyer, Institute of Earth Sciences Jaume Almera (CSIC), SpainCopyright © 2023 Conway, Tani, Sano, Matsumoto and Ishizuka. This is an open-access article distributed under the terms of the Creative Commons Attribution License (CC BY). The use, distribution or reproduction in other forums is permitted, provided the original author(s) and the copyright owner(s) are credited and that the original publication in this journal is cited, in accordance with accepted academic practice. No use, distribution or reproduction is permitted which does not comply with these terms.
*Correspondence: Chris E. Conway, Yy5jb253YXlAYWlzdC5nby5qcA==
Disclaimer: All claims expressed in this article are solely those of the authors and do not necessarily represent those of their affiliated organizations, or those of the publisher, the editors and the reviewers. Any product that may be evaluated in this article or claim that may be made by its manufacturer is not guaranteed or endorsed by the publisher.
Research integrity at Frontiers
Learn more about the work of our research integrity team to safeguard the quality of each article we publish.