- 1Research Center for Oceanography-National Research and Innovation Agency (BRIN), Jakarta Utara, Indonesia
- 2Department of Marine Science, Faculty of Fisheries and Marine Science, Sam Ratulangi University, Manado, Indonesia
- 3Graduate School of Agricultural and Life Sciences, The University of Tokyo, Bunkyo, Japan
- 4Assistant Deputy for Basic, Urban, and Water Resources Infrastructure, Deputy for Infrastructure and Transportation Coordination, Coordinating Ministry for Maritime Affairs and Investment, Jakarta, Indonesia
- 5Research Center of Hydrodynamics Technology- National Research and Innovation Agency (BRIN), Yogyakarta, Indonesia
- 6Department of Marine Science, Faculty of Marine Science and Fisheries, Hasanuddin University, Makassar, Indonesia
- 7Centre for Tropical Water and Aquatic Ecosystem Research (TropWATER), James Cook University, Cairns, QLD, Australia
- 8School of Environment and Society, Tokyo Institute of Technology, Tokyo, Japan
Seagrass canopies are important components of the world’s coastal environments providing critical ecological services. Nearshore hydrodynamics, i.e., waves and currents, are essential in controlling the ecological processes across coastal environments. Seagrass meadows can impose more complex hydrodynamics processes by attenuating sea-swell waves and decreasing the impact of nearshore mean water level rise due to wave setup and Infragravity (IG) waves. Consequently, the seagrasses dissipate waves and reduce flows allowing sediments to settle and accrete the shorelines. However, despite their significant roles, knowledge of hydrodynamics in the Indonesian seagrass ecosystems is relatively limited compared to other coastal ecosystems such as sandy beaches, mangroves, and coral reefs. This review highlights the dynamics of waves and currents, and their interaction with sediment transport and ecological processes, including biogeochemical and dispersal processes on the seagrass ecosystem contributing to the existing seagrass research in Indonesia. The associated literature is collected from scientific databases such as Scopus and Google Scholar that range between 1965 and 2021. The result showed that most of the research on hydrodynamic in seagrass ecosystems was carried out in temperate zones. Until recently, there have been limited publications discussing the interaction between the Indonesian (tropical) seagrass ecosystem and hydrodynamics parameters, even though the region has abundant seagrass species. Moreover, Indonesia is strongly influenced by various atmospheric-oceanic forcing, including the Asian monsoon affecting the dynamic of the coastal area with seagrass ecosystems. At a canopy scale, the correlation between the nearshore (tropical) hydrodynamics and ecological processes in the system is yet to be explored. Considering the potential benefit of seagrasses to coastal ecosystems, developing future research in hydrodynamics across the ecosystem is critical to overcoming the knowledge gaps in Indonesia. The knowledge gained could support the Indonesian seagrass ecosystem services and their resilience to potential hazards and climate change.
1 Introduction
Seagrasses are marine flowering plants that form extensive meadows in sheltered coastal areas with sufficient sunlight that occupy 10% of nearshore regions globally (Duarte 1991a; Green and Short 2003). The meadows, termed canopies (a term describing very rough surfaces, see Dalrymple et al., (1984)), are one of the most productive ecosystems on Earth (Thomson et al., 2015), providing various ecosystem goods and benefits. For the environment, seagrass ecosystems act as blue carbon storage (Ricart et al., 2020), nutrient fixation (Presley and Caffrey 2021), as well as offering shelters and food for invertebrates, fishes, and species of conservation interest, such as dugongs and green sea turtles (Olson et al., 2019). Moreover, for human wellbeing, seagrass ecosystems significantly contribute to material and non-material benefits of the community, including shoreline protection (Donatelli et al., 2019), coastal fisheries (Unsworth and Cullen 2010) or recreation and cultural activities (de la Torre-Castro and Rönnbäck 2004).
1.1 General hydrodynamics processes
Most seagrass species live in shallow nearshore areas with sufficient sunlight for photosynthesis (Hayes et al., 2020; Short et al., 2007). Although seagrass can be found in water deeper than 30 m (e.g., Duarte 1991b; Esteban et al., 2018; Zubak et al., 2020), these are predominantly species of Halophila where the shoot heights would not be sufficient to modify the hydrodynamics mechanism within the deeper regions. Therefore, it could be assumed that the ecosystem of the deeper seagrass areas would be comparable to typical oceanic environments. Similar to other coastal environments, the physical mechanism underlying the generation of hydrodynamics, i.e., waves and currents, forcing in seagrass environments is also significantly influenced by atmospheric-oceanic interactions. Ocean waves are a superposition of regular waves at wide bands of wave periods in the order of milliseconds to more than 24 h. Offshore waves that contribute most of the total wave energy have short periods (5–12 s), termed as wind-sea waves, are generated by winds (Zhao et al., 2016; Holthuijsen 2010; Young 1999). The sea-air interaction also generates longer periods of surface modulations, including swell waves with periods of 12–25 s, or Infragravity (IG) waves with periods of 25 s to a couple of minutes (Cheriton et al., 2016). Meanwhile, offshore currents are driven by complex forces; amongst them are tides (e.g., Timko et al., 2013), winds (e.g., Bressan and Constantin 2019), water density differences due to temperatures, and salinity variation (e.g., Whitehead 2018). Moreover, the patterns of ocean currents are also influenced by their geographical locations (Fu 2009) and regional topography (Saenko and Merryfield 2005).
Even though the offshore hydrodynamics processes are relatively similar across many environments, the characteristic of waves and currents in coastal areas varies depending on the local environments. Across nearshore regions, including seagrass ecosystems, wave energy dissipation is mostly influenced by wave-seabed interaction. Waves will break and dissipate their energy when the proportion between the wave height and water depth reaches a critical limit (Watanabe et al., 2020). While (short) wave energy is mainly dissipated due to breaking, there is a portion of longer period waves (periods of 25–300 s, the IG waves) that do not break and freely propagate onshore (Sheremet et al., 2002). These typical waves may also be generated from a time-varying breakpoint on relatively steep beach profiles (Battjes and Janssen 1978; Symonds et al., 1982). Some research identifies the IG energy spectrum to be dominant near the shoreline, especially during extreme wave events (Péquignet et al., 2014; e.g.; Becker et al., 2016). The breaking waves impose cross-shore and along-shore radiation stress gradients that drive nearshore water mass movement in onshore directions inducing currents and setup (Longuet-Higgins and Stewart 1964). The wave-induced currents can be significant near the breaking points (compared to the oceanic currents) and become the main driving factor of coastal sediment transport. Alternatively, the residual wave energy reaches the shorelines as bores and induces swash fluctuations around the shorelines. Wave setup and swash excursion are the main components of wave runup that significantly impact coastal inundation and erosion above the shorelines (Suanez 2015; Palmsten and Splinter 2016).
1.2 The interaction between seagrass canopy and hydrodynamics
The existence of seagrass meadows within nearshore regions can impose more complex hydrodynamics processes than bare sand environments (Nepf 2012; Schaefer and Nepf 2020). Seagrasses attenuate sea-swell waves providing a sheltered area across the meadows (Oprandi et al., 2020). Seagrass meadows decrease the impact of nearshore mean water level rise due to wave setup and IG waves (van Rooijen et al., 2015; van Rooijen et al., 2016a), which subsequently reduce the wave runup (John et al., 2016; Passarella et al., 2020). Seagrasses obstruct flows (resulting from waves, tides, and other forcings), allowing sediments to settle and accrete along shorelines (Gacia et al., 1999; Bos et al., 2007). Hence, the ability of seagrasses to reduce hydrodynamics pressure and stabilize sediments is an important element for natural coastal protection against hazards (aka a Nature-based Solution) (Paul 2018).
There are several intercorrelated parameters affecting the behavior of hydrodynamics in seagrass environments. At a canopy scale, drag is a fundamental parameter for wave-current attenuation across seagrass meadows (Fonseca et al., 1982). On sandy (bare) beaches, the drag substantially comes from the interaction between hydrodynamics and the seafloor (e.g., Feddersen et al., 2003). On more complex beaches like seagrass environments, seagrass meadows increase the roughness of the bottom (e.g., Monismith et al., 2019). Consequently, the drag exerted by the canopies imposes dissipation on wave-driven (orbital) and current-driven (unidirectional) velocities (Koch and Gust 1999; Luhar et al., 2013; van Rooijen et al., 2018).
The drag on the seagrass canopy is substantially influenced by the geometric attributes of seagrass meadows (Nepf 2012). The height of a canopy relative to water depth proportionally affects the amount of dissipated wave energy (Chen et al., 2007) and flows (Lowe et al., 2005). Fonseca and Cahalan (1992) evaluated four North American seagrass species, i.e., Halodule wrightii, Syringodium filiforme, Thalassia testudinum, and Zostera marina, using a wave flume test. They found the substantial influence of the shoot/blade length on the wave reduction (−40%) when the ratio between the shoot length to the water depth is equal. These findings were supported by similar flume experiments on other seagrass species, e.g., Manca et al. (2012) on Posidonia oceanica. Similar results were reported from a study in the Indonesian coastal region where a low seagrass canopy could still provide shelter for coastlines by reducing excessive wave energy (Christianen et al., 2013). Assuming the canopy height is lower than the total depth, the velocity within the canopy layer is significantly reduced compared to above the canopy up to the water surface. The velocity within the canopy is also lower than that of within the water column of bare sand environments (Finnigan 2000; Abdolahpour et al., 2017). Moreover, the canopy also deflects a portion of the incoming flows upward, resulting in higher flow velocity above the canopy (Morris et al., 2008). These conditions result in vertical gradients of orbital and unidirectional velocities across the water column of the seagrass canopy, as illustrated in Figure 1. Some studies in temperate regions (e.g., Carr et al., 2012; Hansen and Reidenbach 2013) associated the correlations between seasonal wave heights and the variation of seagrass morphology. The studies showed the length of seagrass shoots tends to be shorter in winter when wave heights are higher. In contrast, the length of seagrass shoots reaches a peak during summer when waves are calm, and the length may reach two times longer than that in winter. These behaviors were identified in the northern (e.g., Reidenbach and Thomas 2018) and southern hemispheres (e.g., Bulthuis and Woelkerling 1983). Abdelrhman (2007) indicated that the longer length of seagrass shoots during summer is influenced by the increased light intensity and temperature, inducing photosynthetic productivity.
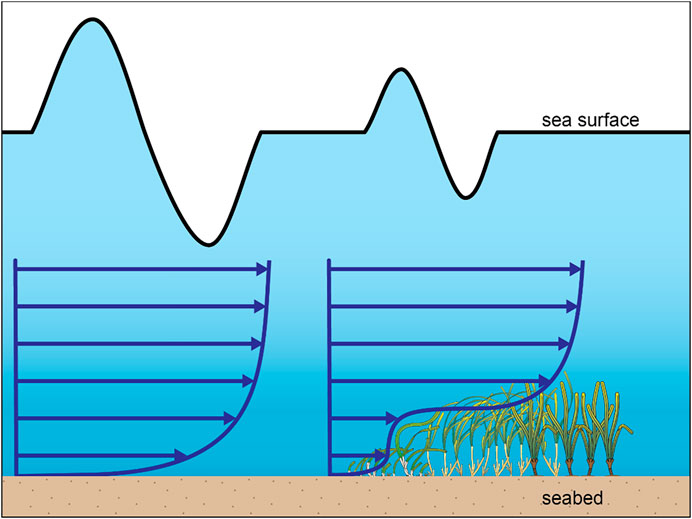
FIGURE 1. Schematics of time average vertical velocity profile within a bare sand environment (left) and a seagrass meadow (right). The sinusoidal waves on top of the velocity profiles illustrate the significant wave height evolution where seagrasses induce dissipation.
The length of seagrass meadows, i.e., the distance of the meadows from the offshore edge to the shoreward edge, is beneficial to impose waves and currents attenuations (Fonseca et al., 1983). The increase in meadow length is generally proportional to waves and currents attenuation, which is attributed to the increase of drag across the canopy (Bradley and Houser 2009). The finding was confirmed by Twomey et al. (2020) through a numerical analysis identifying the impact of various meadow lengths on the reduction of wave height. In a low wave energy condition (Hs < 1 m, T 6 s) with a shoot density of 1200/m2, canopy height of 0.5 m, and water depth of 2 m; A 100 m canopy could attenuate 80% of wave energy. Meanwhile, a 200 m canopy tested in the numerical model with similar wave and canopy settings could attenuate almost 90% of wave energy. Other studies showed that wave heights increase around the offshore edge of the meadows before dissipation due to sudden changes in bottom topography before being dissipated due to friction (Bradley and Houser 2009; Stratigaki et al., 2011; Manca et al., 2012). Interestingly, Villanueva et al. (2021) found that the magnitude of wave attenuation due to the meadows would not increase after certain lengths.
Laboratory (e.g., Manca et al., 2012; Koftis et al., 2013) and field (e.g., Reidenbach and Thomas 2018; Donatelli et al., 2019) experiments identified the importance of seagrass density per unit area to wave-currents attenuation. A flume study by Weitzman et al. (2015) identified the shoot density of seagrass canopies could be the most important parameter in wave attenuation. A similar result was reported from the Mediterranean Sea during high wave events suggesting the bottom roughness induced by seagrass meadow depends on the shoot density (Infantes et al., 2012). A higher seagrass shoot density per unit area generally increases the drag to impede the perpendicular (orbital and unidirectional) flows. On top of dense canopies, the vertical velocity profile forms an inflection point that induces Kelvin-Helmholtz flow instability and the generation of turbulence (Ghisalberti and Nepf 2002). Twomey et al. (2020) performed numerical analyses showing that an increase in seagrass density per square meter (600–1800 shoots) was proportional to the increase in wave attenuation (68%–84%). Meanwhile, the results of field studies are more variable than the laboratories. A field experiment in South Bay, Virginia Coasts Reserve (United States) exhibited −20% of wave attenuation on −500 shoots/m2 across Zostera marina dominated bay (Zhu et al., 2021). However, another field experiment by Paul and Amos (2011) on Ryde Sand Beach (UK), exhibited comparable wave reduction, i.e., −20%, was obtained at a much higher density (−4000 shoots/m2) of Zostera noltii meadows. Various hydrodynamic behavior among different seagrass species suggested the influence of vegetation characteristics on shoots level to the wave-current attenuation (Pinsky et al., 2013).
At a smaller scale, i.e., seagrass shoots, the mechanism of seagrasses to modify the behavior of waves and currents depends on their physical features forming the canopies. The drag reaches its maximum when the shoots are in their natural upright postures. However, as waves propagate nearshore, the flexible seagrass shoots deform (bend) and move due to wave-orbital flow inducing drag and in turn causing wave decay across the seagrass environment (Abdolahpour et al., 2018). The effectiveness of shoot motion to dissipate wave energy is primarily determined by the effective shoot length parameter, in which shoot length is a combined leaf length (including sheath) and vertical stem length (if present). This parameter is governed by the physical characteristics of individual shoots, i.e., width, length, thickness, and elastic modulus, representing the stiffness of the shoot. The effective shoot length parameter is also determined by the ratio between the shoot length and fluid particle elliptical orbits due to wave actions (Lei and Nepf 2019). Meanwhile, the parameters describing physical features are defined by the Cauchy number, i.e., the ratio between the wave-induced drag and the restoring force due to the shoot stiffness and the Buoyancy parameter. Wider shoots are known to have a higher drag imposing higher wave dissipation and impediment to flow (Fonseca et al., 2007; Luhar and Nepf 2011; 2016; Luhar et al., 2017; Twomey et al., 2020). Moreover, the drag is also correlated with the flow regime, expressed in the vegetation Reynolds number (Re), which is a function of the shoot width, water kinematic viscosity, and the wave-induced characteristic velocity acting on the vegetation (Bradley and Houser, 2009; Paul and Amos, 2011).
Seagrass shoots modify the flow structure across the environments. Under the flow regimes, stiffer shoots generate less turbulence than flexible shoots (Houseago et al., 2022). Meanwhile, more flexible shoots may overbend and synchronously oscillate, creating wavy motions termed “monami” (Ackerman and Okubo 1993; Ghisalberti and Nepf 2006). This phenomenon is one of the essential characteristics of seagrass canopies since it may reduce canopy drag by 40%. The monami occurs when the flows above the meadow suppress the critical value increasing the frictional resistance to particle movement. Accordingly, vortices are generated through Kelvin-Helmholtz instability (Grizzle et al., 1996; Bryan et al., 2007; Ghisalberti and Nepf 2009). The characteristic of monami is influenced by the coherence between the natural frequency of seagrass shoots and the flow motion induced by mixing layer instability. Stiffer shoots indicate a higher natural frequency (O’Connor and Revell 2019). Later, Taphorn et al. (2021) identified the natural frequency of seagrass shoots is determined by the shoot’s stiffness which is represented by the modulus of elasticity and the moment of inertia parameters. Moreover, the characteristic of monami is also considered to be affected by the non-linear interactions between the two phenomena. First is Kelvin-Helmnhotz turbulence, and second is complicated 3D flows that loop and wrap around near the canopy edge, termed as “hairpin vortex” (Tschisgale et al., 2021).
The dynamic of nearshore waves and currents across seagrass-dominated environments is determined by the wide variability of offshore forcings. While most literature discusses the dynamics of seagrass meadows under short wave onset that become the largest portion of the offshore wave spectrum, some research has also identified the interaction between the meadows and the lower wave frequencies. Manca et al. (2010), through a flume study, found the presence of wind waves at lower frequencies (higher periods than 6.4 s in the study) seemed to impose the extra bending of the top of the canopy reducing wave attenuation. The result is expected since the height of the canopy reduces to allow more waves to pass through. Interestingly, A −13 months field observation in the UK by Paul and Amos (2011) exhibited low-density seagrass canopy could effectively dissipate low-frequency wind waves. At Infragravity bands with periods of 25–600 s, a numerical study by Chen et al. (2022) identified seagrass canopies could dissipate more than 50% of the incident IG wave height. Accordingly, the significant reduction of IG wave energy implies reduced wave runup (John et al., 2016) and erosion at the lee side of the canopies (Chen et al., 2022).
1.3 The morphodynamics of seagrass-associated beaches
At various temporal scales, the short time scales, i.e., hours to days, the dynamic of beach morphology on seagrass-dominated environments are influenced mainly by extreme wave events that lead to excessive erosion and deposition. Sometimes, the impacts of storms are devastating for seagrass ecosystems. Not only causing the change of nearshore bottom profiles, but episodic wave events may degrade seagrass meadows due to the grass uprooting (e.g., Gera et al., 2014) and/or sediment burial (e.g., Cabaço et al., 2008). For example, a severe storm hit the Ligurian coast of the Mediterranean Sea in October 2018, resulting in the loss of around 50% coverage of the P. oceanica dominated canopies. The eroded sediment mostly buried the canopies, and the loss due to 1 day storm was estimated to be equal to that of −160 years of anthropogenic influences (Oprandi et al., 2020). Similar extreme events resulting in massive losses have been documented in other areas across the globe, such as the United States (e.g., Carlson et al., 2010) or Africa (e.g., Côté-Laurin et al., 2017). Interestingly, while shorelines and seagrass meadows may suffer significant physical damage after high wave events, which is a consequence of the flexible shoot structures, many reports show seagrass meadows could recover quickly. For example, a study after Hurricane Katrina in 2005 reported the seagrass density and biomass conditions had returned to their initial state within less than a year (Anton et al., 2009). Similar conditions were also reported in China (e.g., Yang and Huang 2011) and Central America (e.g., Michot et al., 2002). Above all, even though the seagrass meadows could quickly recover, the recovery phase of eroded shorelines on the lee side of the meadows could be much slower. This indicates that seagrass meadows could be less suitable for protecting shorelines in high-energy beach systems.
On the other hand, at longer time scales, i.e., months to years, the morphodynamics of seagrass-associated beaches are more related to the geographical locations inducing the fluctuations of seagrass biomass as well as atmospheric and oceanic forcings. In temperate regions, the winter could include a period where the coastlines are highly dynamic. During this season, the biomass and density of seagrass are low due to low temperatures and low light availability reducing photosynthesis (Duarte 1989; Alcoverro et al., 2001). At the same time (not limited to seagrass ecosystems), the winter waves, currents, and mean water levels are significantly higher than the summer’s (e.g., Risandi et al., 2020). Therefore, these conditions increase the vulnerability of shorelines to erosion (Carr et al., 2018). In contrast, seagrass density normally peaks in the summer, considerably reducing unidirectional and orbital velocities. Moreover, the atmospheric and oceanic forcings are relatively calmer, inducing additional sediment suspension and shoreline deposition (Hansen and Reidenbach 2013; Ganthy et al., 2015; Zhu et al., 2021; Contti Neto et al., 2022). In tropical regions, although seagrass density and canopy heights can similarly change (Erftemeijer and Herman 1994; McKenzie 1994; McKenzie et al., 2016), studies on temporal variations of seagrass-dominated beach morphology across tropical regions are poorly documented. Nevertheless, some reviews (Potouroglou et al., 2017; e.g.; James et al., 2019) exhibited a net gain/equilibrium state of sedimentation on tropical beaches (Christianen et al., 2013), indicating the contribution of seagrass to beach protection.
1.4 Hydrodynamics impact on seagrass ecological processes
Similar to other coastal environments, nearshore hydrodynamics play a significant role in controlling biogeochemical processes within seagrass ecosystems, yet the knowledge still needs further exploration. For instance, an ecosystem with low waves and currents is associated with the increase of organic carbon (Corg) stock within seagrass soil. This is because of the reduced sediment erosion and seagrass detritus washout within the ecosystem, resulting in seagrass soil enrichment (Röhr et al., 2016; Novak et al., 2020; Mazarrasa et al., 2021). Interestingly, high hydrodynamic exposure is also found to increase the density of bottom sediment near the surface (at 0–25 cm depth), increasing the accumulation of carbon and nitrogen in the sediment (Dahl et al., 2020). Moreover, the dynamic of seagrass primary productivity and nutrient concentration is also significantly affected by the fluctuation of waves and currents. The hydrodynamics may impose nutrient overload that is considered to negatively affect the existence of seagrass meadows due to ammonium toxicity (Burkholder et al., 2007; El-Hacen et al., 2019). In high wave energy environments, waves can stir nearshore sediment, causing a decrease in water quality that affects seagrass growth (Greve and Binzer 2004). A study in Australian coastal lakes exhibited low seagrass biomass in an area exposed to high wave energy, suggesting the impact of bottom sediment resuspension on the reduced seagrass photosynthesis efficiency (Ferguson et al., 2016). In contrast, Egea et al. (2018) reported the increase of current velocity under low seawater pH condition, referred to as ocean acidification, positively affect the primary productivity of seagrass (Z. noltei).
Ocean currents and waves act as abiotic dispersal vectors that interact with key traits of seagrass life-history stages, including pollens, reproductive propagules, vegetative fragments, and clonal growth (Orth et al., 2007; Pereda-Briones et al., 2018). In addition, the dispersal of vegetation fragments, pollen, and seed is mainly affected by tidal currents and wind-generated waves (Koch et al., 2006; Lai et al., 2018). The dispersal mechanism of both tropical and subtropical seagrass species is mostly by waterborne transport of viable propagules (Berković et al., 2014). Propagule movements on the sea surface and within the water column are influenced by currents produced by tides, waves, wind, seas, and swells (Micheli et al., 2010; McMahon et al., 2014). Moreover, seagrass propagules’ response to current and wave actions is strongly affected by the buoyancy (floating) of their propagules. The response could be positive, neutral, or negative (Kendrick, 2012). The floating fruits of Enhalus and Thalassia could be dispersed by ocean currents to distances over 350 km from the originating plant (van Dijk et al., 2009; Kendrick, 2012). However, the drag forces from currents and waves are also the major factors that cause the uprooting of seagrass propagules, resulting in the failure of their recruitment and establishment (Zenone et al., 2022).
1.5 Seagrass ecosystem in Indonesia
Indonesia is a global hotspot for seagrass ecosystems, with nearly 3,000 square kilometers of meadows have been mapped and possibly as much as ten times that amount remaining undocumented (Esteban et al., 2018; McKenzie et al., 2020). Of the 72 world’s seagrass species (Short et al., 2011), 14 occur in Indonesia’s coastal waters, including, Cymodocea rotundata, Cymodocea serrulata, Enhalus acoroides, Halophila decipiens, Halophila major, Halophila minor, Halophila ovalis, Halophila spinulosa, Halophila sulawesii, Halodule pinifolia, Halodule uninervis, Syringodium isoetifolium, Thalassia hemprichii, and Thalassodendron ciliatum (Kuo 2007; Kurniawan et al., 2020).
Based on seagrasses’ ability to resist environmental pressures and their capacity to recover from physical degradation (Kilminster et al., 2015), Indonesia’s seagrasses can be divided into three main species groups (Figure 2). 1. Persistent species, e.g., Thalassia hemprichii, Thalassodendron ciliatum, and Enhalus acoroides. These species are characterized by taller shoots with large biomass and high durability to environmental pressures, but they need a long period to recover. T. hemprichii and E. acoroides can be found in shallow waters (<3 m depth) near the shoreline, but T. ciliatum typically occurs in deeper water (around 10 m depth); 2. Colonizing species, e.g., Halophila ovalis, Halophila minor, Halophila spinulosa, Halophila decipiens, Halophila sulawesii, and Halophila major. These species are characterized by small morphology and shorter shoots, with a low resistance to environmental pressures, but can recover from physical degradation rapidly. All these colonizing species usually live in shallow waters (<3 m depth) near the shoreline, but H. sulawesii can be found in the deeper water of 25 m (Kuo 2007); and 3. Opportunistic species, e.g., Cymodocea rotundata, Cymodocea serrulata, Halodule pinifolia, Halodule uninervis and Syringodium isoetifolium, which are medium-sized and possess transitory characteristics between persistent and colonizing. These transitory species usually can be found in shallow water near the beach (<3 m depth).
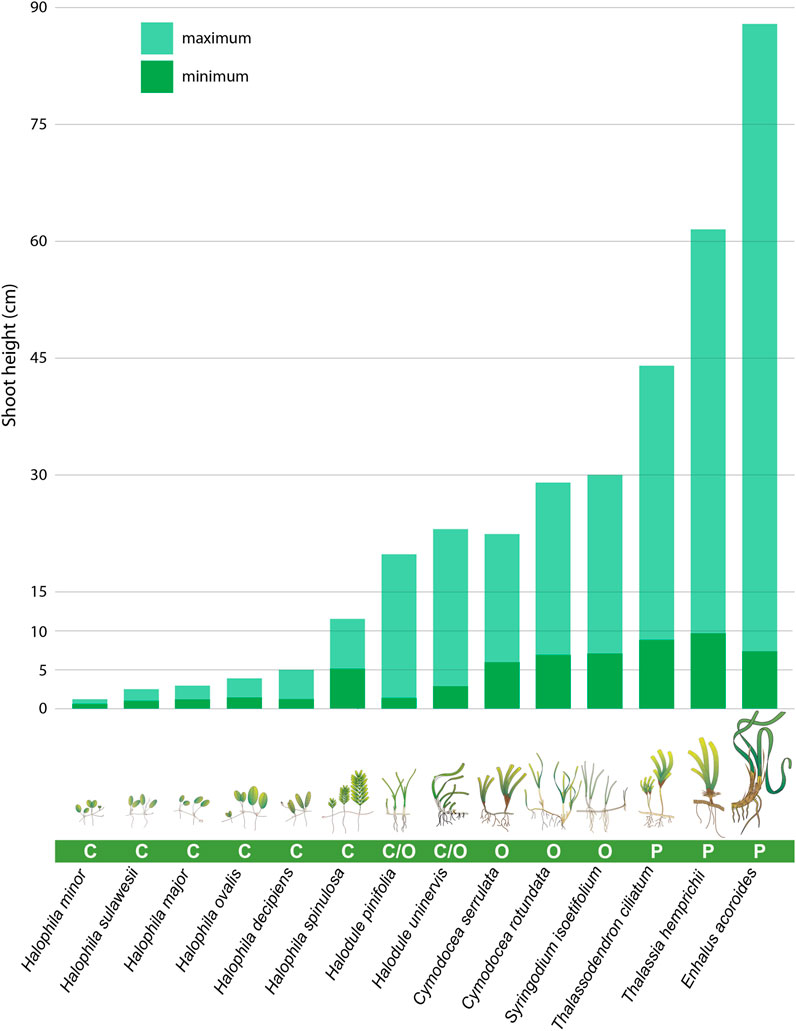
FIGURE 2. Shoot height variability of different Indonesian seagrass species, grouped by dominant traits, i.e., colonizing (C), opportunistic (O), and persistent (P) seagrasses, as per Kilminster et al. (2015). Shoot heights sourced from data portals, including http://www.wildsingapore.com and https://www.seagrasswatch.org/indonesia/; and herbarium specimens, Seagrass-Watch Virtual Herbarium (SWVH) [not an official acronym], Cairns, Australia; Nationaal Herbarium Nederland—Rijksherbarium, Leiden (L), Naturalis Biodiversity Center, Netherlands.
In terms of seagrass species distribution, a nationwide seagrass monitoring program conducted between 2015 and 2017 at 366 locations across Indonesia coastal (from intertidal to subtidal zones) found that persistent and large-size species, such as E. acoroides and T. hemprichii are the most common species. Moreover, T. hemprichii had the largest distribution as it was found at 310 locations, followed by E. acoroides which occurred at 301 sites (Sjafrie et al., 2018). This finding highlights the need for a proper management strategy to sustain the life of these slow recover species.
Considering the importance of seagrass meadows to support coastal ecosystems, understanding the dynamics of waves and currents within the ecosystem is beneficial. To our knowledge, a few of the existing scientific works reviewed and described the hydrodynamics and related topics across seagrass environments. For example, the theoretical explanation of wave-seagrass interactions, the stages of conducting field wave observations on seagrass ecosystems, and the numerical-physical modelings were reviewed by Koch et al. (2006). An in-depth review of flow behavior across seagrass meadows that is influenced by the variety of canopy density inducing drag was described by Nepf (2012). Moreover, a review on the role of the seagrass shoot flexibility and density, as well as the heights of the seagrass meadows on sediment transport affecting the shoreline changes, was presented by Ondiviela et al. (2014). Despite the existing literature that mostly covers studies on temperate seagrasses, more studies are still needed to provide an improved understanding of the nearshore dynamics across tropical seagrass ecosystems. Emphasis needs to be given to the Indonesian archipelago, which is considered a region with abundant seagrass resources and strongly influenced by the monsoon inducing the seasonal atmospheric-oceanic variability.
Until now, the research in Indonesia has been dominated by studying the biological and ecological aspects of seagrasses. Meanwhile, appropriate investigations on the interaction between Indonesian seagrass ecosystems and hydrodynamics have been limited, even though most related research identified the importance of these physical parameters to seagrasses. For example, Ambo-Rappe (2022) indicated the successful seagrass restoration using seeds in Spermonde Island, Indonesia, was determined by the area’s exposure to wave actions. Other research in northern Papua (Nugraha et al., 2021) and Talaud Islands (Kawaroe et al., 2016) suggested the correlation between low coverage of seagrass meadows in the study areas with high wave exposure. However, most seagrass studies in Indonesia did not provide proper observations of the hydrodynamics, causing the lack of evidence and scientific explanations underlying the phenomena. This review highlights the knowledge gaps in the dynamics of waves and currents to the associated shoreline changes, biogeochemical, and propagules’ dispersal in the Indonesian seagrass ecosystem. By doing so, this review would guide future strategies related to seagrass research that benefit Indonesian coastal management.
2 Methodology
The review utilized two datasets. The primary data was retrieved using a literature search in Scopus and Google Scholar databases, covering the periods between 1965 and 2021. To identify the associated scientific works discussing the hydrodynamics in seagrass meadows in Indonesia, we used the following string of command: [“seagrass” AND “Indonesia” AND “hydrodynamics” AND “waves” AND “currents”]. An additional search was conducted to gather peer-reviewed papers and grey literature related to Indonesian seagrass ecological processes due to hydrodynamics using the following list of keywords: “monsoon”, “morphology”, “shoreline”, “coastal protection”, “ecology”, “eutrophication”, “carbon”, “tropical”, “dispersal”, and “modeling.”
To exhibit the influence of the Asian monsoon on Indonesian regional currents and waves, we analyzed the spatial hydrodynamic data obtained from the secondary data source. The Indonesian ocean surface currents dataset was retrieved from the daily average surface currents of the Ocean Surface Current Analyses Real-time (OSCAR) of The National Aeronautics and Space Administration (NASA), dated December 2020 to November 2021. Meanwhile, the wave height data was downloaded from the hourly ERA5, the fifth generation of European Centre for Medium-Range Weather Forecasts (ECMWF) atmospheric reanalysis data with a similar timeframe to that of the ocean currents data. The currents (0.25° grid resolution) and waves (0.5° grid resolution) data were averaged over the period representing the Asian monsoonal cycles, i.e., December 2020 to February 2021 for the winter monsoon and June to August 2021 for the summer monsoon.
3 Results
Most studies on seagrass hydrodynamics were focused on temperate seagrasses and a limited representation of tropical species or species occurring within the Indonesian coastal region. The literature search identified some publications related to Indonesian seagrass and hydrodynamics, including waves and currents. However, most of the publications only reported visual conditions of the hydrodynamics without proper observations (using wave gauges or current meters), models, or secondary data analyses. Hence, we excluded those publications from this review. Based on screening and eligibility checks, we found only five relevant publications that were carried out in Indonesia with appropriate quantitative explanations of the interaction between hydrodynamics and the seagrass ecosystem. Among them, the following: a wave observation in Derawan Island by Christianen et al. (2013); a wave flume study on seagrass leaf-trapping capacity by Gillis et al. (2014); the impact of Indonesian regional currents on seagrass dispersal studied by Hernawan et al. (2017); A wave flume observation to study the hydrodynamics of Indonesian seagrass species conducted by Lanuru et al. (2018); and a hydrodynamic numerical study on a seagrass dominated ecosystem in East Kalimantan produced by Tarya et al. (2018). The limited number of studies available in Indonesia indicates that there are knowledge gaps on nearshore waves and currents within the country’s seagrass environments and their implications for Indonesian coastal dynamics. These knowledge gaps are discussed and elaborated in the succeeding subsections.
3.1 The identification of Indonesian seagrass physical characteristics
Information on seagrass in Indonesia is mainly related to the ecological aspects of seagrass species. The additional aspects to be investigated could include the physical characteristics of Indonesian seagrass shoots to modify hydrodynamic regimes. To our understanding, the knowledge of Indonesian seagrass morphologies is still limited to the heights of seagrass shoots (Section 1.5; Figure 2). Meanwhile, complete descriptions of the shoot attributes, i.e., width, length, thickness, and elastic modulus, determining the effectiveness of seagrasses to dissipate waves and currents energy do not exist. Moreover, studying the characteristics of various seagrass rhizoid systems in Indonesia would be advantageous. The below substrate rooting system is essential to keep the seagrass firmly on the sea floor. Deeply anchored rhizomes and flexible shoots could endure more against extreme hydrodynamics (Cruz-Palacios and van Tussenbroek 2005). Additionally, information is still lacking on the physical attributes of Indonesian (tropical) seagrasses that are likely to differ from that of temperate seagrasses.
Knowing Indonesian seagrass morphology is essential to further examine the interaction between vegetation and hydrodynamics. However, performing full-scale seagrass simulations in wave flumes would be very challenging in Indonesia due to limited Indonesian wave flume/basin facilities. Knowledge of the seagrass morphology would be useful for mimicking the full-scale natural seagrass shoots into the down-scale artificial ones. Accordingly, the similitude of the inertia, the gravitation, and the viscosity forces, between the real and the model could still be retained (Zeller et al., 2014). Likewise, the characteristics of seagrasses are essential for predicting the wave dissipation parameter in numerical models (van Rooijen et al., 2016b; James et al., 2021; Yin et al., 2021). However, the literature related to Indonesian seagrasses remains limited or non-existence.
3.2 The interaction between (future) atmospheric-oceanic forcing and seagrass ecosystem across the Indonesian region
The Indonesian archipelago is situated on the equator with wide-ranging ocean and climate variability. For example, tidal currents are relatively strong due to the vicinity of narrow straits (Ray et al., 2005), as indicated by the reddish color in Figure 3. Moreover, Indonesian seas are characterized by regional oceanic forcings that are the parts of global circulation, e.g., the Indonesian Through Flow (Gordon 2005). Some features of the region with abundant seagrass meadows are prone to extreme wave events. For example, in Manado, Northern Sulawesi facing the Philippines, the area is frequently influenced by typhoons inducing high waves. In the Southern part of Indonesia facing the Indian Ocean, e.g., southern Java and Bali, the wave energy is much higher (Figure 4). This is caused by the interactions among atmospheric and oceanic forcing, such as the Asian monsoon, the Indian Ocean Dipole Mode, and El Nino Southern Oscillation (Rachmayani et al., 2018).
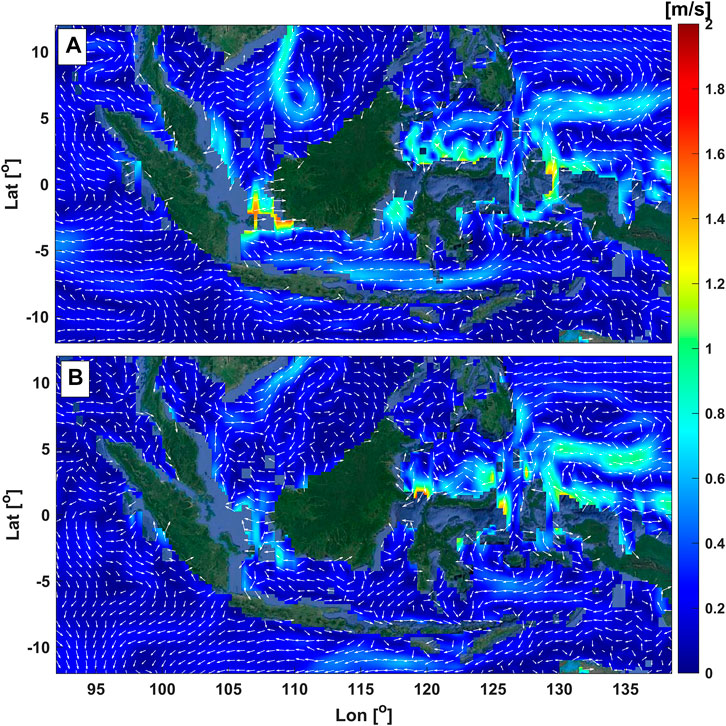
FIGURE 3. Average current speed and direction across the Indonesian region during (A) the winter and (B) the summer monsoons. The current vectors are on a logarithmic scale. The dataset’s source is the OSCAR’s daily average surface currents from December 2020 to November 2021. The Source of the aerial image is Google Earth satellite imagery.
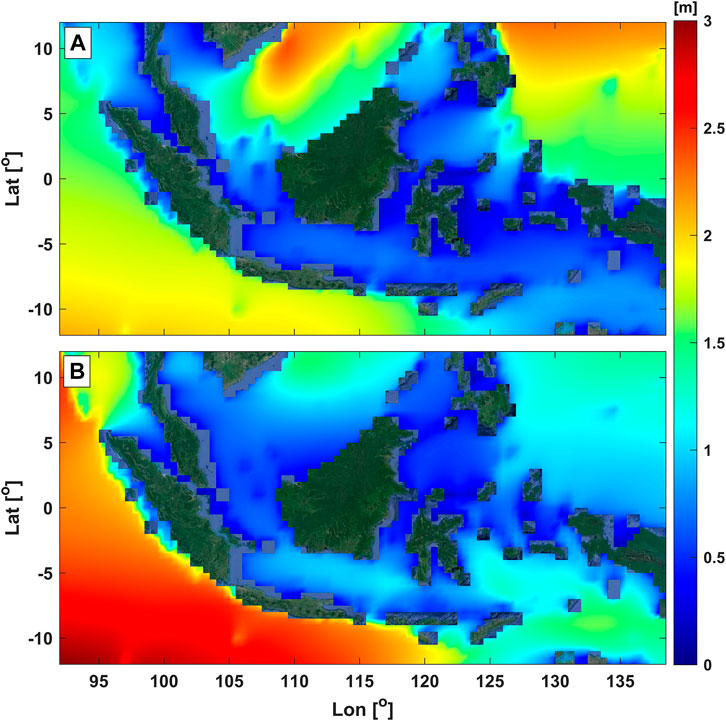
FIGURE 4. Average wave heights across the Indonesian region during (A) the winter and (B) the summer monsoons. The source of the dataset is the hourly wave heights of the ERA5 from December 2020 to November 2021. The Source of the aerial image is Google Earth satellite imagery.
Among the atmospheric forcing, Indonesia is significantly influenced by the periodic Asian monsoon winds with different characteristics throughout the years that affect the variability of hydrodynamics within the region. Between December to February, the northwest (Asian winter) monsoonal wind blows from Eurasia toward Australia, inducing eastward dominated currents (see Figure 3A), with low salinity water passing through the Indonesian seas. In contrast, the southeast (Asian summer) monsoonal wind drives reversal currents (see Figure 3B) with more-saline water during June-August (Gordon et al., 2003; Qian et al., 2010; Basit et al., 2021). The wave characteristics in Indonesia are also affected by the monsoonal cycles, in which the exceedance of high wave across the region are associated with the peak of the summer and winter monsoon. During the peak of the Asian winter monsoon, higher waves mainly occur in the northern parts of Indonesia, i.e., the area around the South China Sea and the Pacific Ocean, see Figure 4A. Meanwhile, the peak of the Asian summer monsoon would impose higher waves around the southern region, i.e., the Indian Ocean and its adjacent regions like the Banda Sea and the Arafura Sea (Figure 4B). This phenomenon was identified by Rachmayani et al. (2018), Purwanto et al. (2021), and Kurniawan and Khotimah (2016). Considering Indonesia is an archipelago, the influence of the Asian monsoon on the seagrass hydrodynamics could be variable among the islands/regions that need further study. For example, the correlation between seasonal monsoonal waves and the morphology of local seagrass remains unknown but could be essential information for conservation purposes. A study in the North Indian Ocean suggested the impact of weakening Indian monsoon on the reducing heat transfer that accelerates the increase of mean sea level over decades within the region affecting coastal areas (Swapna et al., 2017). However, to our knowledge, studies on the impact of variable Asian monsoon to SLR affecting the Indonesian nearshores, especially the seagrass ecosystem, are lacking despite the importance to Indonesian coastal management.
In shallower regions, extreme wave events usually induce the generation of IG waves (e.g., Anarde et al., 2020) and setup (e.g., Kim et al., 2010) that increase the mean water levels associated with coastal flooding and erosion. While some publications reported the dissipation of sea-swell waves in seagrass environments (see Section 2), the knowledge on the influence of seagrasses on IG waves is less known. However, some research (e.g., van Rooijen et al., 2016a) exhibited the reduction of IG wave energy over the meadows. In Indonesia, the knowledge gaps are even wider despite the frequent coastal flooding and the abundance of seagrass within the country.
Furthermore, the substantial impacts of climate change on coastal areas have been identified and widely accepted (Toimil et al., 2020; e.g.; Athanasiou et al., 2020; Bruun 1962). Not only increasing low-lying coastal inundation and extreme atmospheric-oceanic forcing, but the rise of mean sea level due to climate change also increases the vulnerability of coastal regions to erosion as wave energy dissipation further onshore. A recent Intergovernmental Panel on Climate Change (IPCC) publication (2021) estimates Sea Level Rise (SLR) around 10–25 cm and more frequent extreme waves by 2050 that would significantly modify the overall processes across coastal regions, including seagrass environments. Under SLR, the response of seagrasses is variable, i.e., they would adapt to new conditions or migrate into suitable regions or survive on their positions relative to the water level through sediment accretion, and if the conditions become unsuitable, the vegetation would decline (Short and Neckles 1999; Duarte 2002; Waycott et al., 2007). A study by Saunders et al. (2013) predicted that 17% of seagrass would decline under 1.1 m of SLR, which would further affect the sedimentation rate across the ecosystem. In contrast, a model by Dumbauld et al. (2022) predicted the increase of seagrass coverage under 0.4–0.74 m of SLR due to the suitable environmental variables, i.e., temperatures and CO2 enrichment.
Until now, little research has been conducted globally on the correlation between SLR and seagrasses. In Indonesia, developing knowledge on the impacts of SLR on seagrass meadows is critical since the ecosystems are among the largest in the world, providing beneficial ecosystem services to the wellbeing of the Indonesian people. However, the threats to Indonesian seagrass ecosystems are exacerbated by anthropogenic activities, causing a decline of 30%–40% of the meadows coverage since the 60s (Alongi et al., 2016; Unsworth et al., 2018). The rate of decline of the seagrass ecosystem was estimated to be around two-fold the global average (Duarte et al., 2013), which in the future will worsen as a consequence of global climate change. Hence, the need for long-term and detailed investigation on the impact of SLR (mainly due to the Asian monsoon) and anthropogenic pressures on eutrophication within the Indonesian seagrass ecosystem is critical to mitigating the ongoing seagrass degradation.
3.3 Long-term investigations on the impact of seagrass on Indonesian shoreline variability
Despite the abundance of seagrasses in tropical regions, including Indonesia, long-term studies on the morphodynamic changes and the governing processes within such locations are very limited compared to temperate regions. The longest study recorded across the Indonesia region was by Christianen et al. (2013) that investigated the behavior of a seagrass-dominated beach in Derawan Island through a −3-month measuring campaign (December 2011-February 2012). They found that the seagrass canopy dissipated the offshore wave energy and subsequently stabilized the nearshore sedimentation. However, longer-term records (more than a year) on the interactions among the hydrodynamics, beach response, and seagrass meadows variability to comprehensively assess the dynamics of Indonesian (tropical) seagrass ecosystems are greatly lacking or do not exist.
Until recently, seagrass restoration projects have been promoted within the country due to the reported decline of Indonesia’s seagrass conditions. However, until now, the impacts of replanting seagrass on the behavior of hydrodynamics and the resulting shoreline changes in tropical regions, mainly in Indonesia, are poorly documented. The Spermonde restoration project in South Sulawesi, regarded as the longest recorded period of the restoration project in Indonesia, did not cover the hydrodynamic and nearshore morphology observations (Ambo-Rappe 2022).
3.4 Ecological process across Indonesian seagrass ecosystems
The monsoon variation plays an important role in influencing the rainfall intensities across the Indonesian area and further affects many ocean parameters. For instance, the variability of seawater salinity (e.g., Chen et al., 2019), upwelling (e.g., Wirasatriya et al., 2021), and downstream flooding (e.g., Narulita and Ningrum 2018) depend on the monsoonal cycle, potentially affecting the sustainability of seagrass ecosystems. A study on seagrass physical characteristics showed the decrease of carbohydrates within seagrass shoots due to the increase of seawater salinity affecting the elasticity of the shoots (Touchette 2007). As a result, the ability of seagrasses to withstand hydrodynamics pressures decreases (Houseago et al., 2022). In a tropical region like Indonesia, high rainfall intensity potentially imposes coastal upwelling to modify the local biogeochemistry regime of seagrass environments (Hessing-Lewis et al., 2015). The rainfall intensity also induces flooding that generates excessive sedimentation in coastal areas (Saunders et al., 2017), potentially harming seagrasses. Yet, the impacts of those atmospheric-oceanic interactions on seagrass ecosystems in Indonesia are still less known.
The research on the impact of hydrodynamics on Corg stocks within Indonesian seagrass meadows is limited. A study conducted in Spermonde Archipelago, considered the first reported Corg observation in the Indonesian region, indicated the influence of waves and tides to spatially control the distribution and accumulation of organic matter. Some areas exposed to wave energy had a smaller fraction of seagrass detritus than the sheltered ones (Rahayu, 2019). However, the study did not measure the hydrodynamics parameters, and how it can influence the carbon stocks. Considering the Indonesian region has a wide-ranging ocean and climate variability with various seagrass species, more studies are needed to accurately estimate the carbon capacity of the seagrass ecosystems and their role in mitigating climate change.
The focus of the research could be expanded to improve the understanding of the interactions among biological, chemical, and physical (including hydrodynamics) affecting the production, dispersion, trapping, and preservation of Corg (e.g., Kim et al., 2022) within Indonesian coastal region. Carbon can be transported elsewhere beyond ecosystems due to ocean currents and storms (Krause-Jensen and Duarte 2016; Duarte and Krause-Jensen 2017). Some evidence proved that C export from seagrass meadows could travel far beyond hundreds to thousands of kilometers from initial sources (Hyndes et al., 2014; Duarte and Krause-Jensen 2017). In Indonesia, the Asian monsoonal cycle significantly influences the regional Indonesian hydrodynamics, see Figures 3, 4 as well as references like Najamuddin et al. (2021), which in turn would affect the variability of Corg stocks within the archipelago. Additionally, there were studies (e.g., Gordon and McClean 1999; Zhao et al., 2017) that suggested Asian monsoon winds also affect the fluctuation of ocean temperatures and salinity within the southeast Asia region affecting the structures of thermohaline circulation. Studies on this topic are necessary since the carbon may be transported across the globe, passing through the tropical areas due to the difference of salinity generating thermohaline (aka “conveyor belt”) circulation (Kawada et al., 2006; Riebeek 2008). This is another focus that should be addressed to enhance seagrass Corg sequestration by modifying hydrodynamic parameters across seagrass-dominated beaches (e.g., Serrano et al., 2020) to mitigate climate change in Indonesia.
The increase in sea level and its impact on eutrophication need to be considered for future studies of Indonesian seagrass. As for eutrophication, water quality degradation is the main reason for seagrass loss and the loss of resilience against nutrient pollution (Thomsen et al., 2020). Both eutrophication and sea level rise are relevant challenges in the context of Indonesian seagrass, mainly for coastal developments. Understanding land-use change is imperative in managing Indonesian coastal ecosystems with numerous environmental impacts from land-use conversion (Lukman, 2021). In seagrass ecosystems, anthropogenic activities such as coastal urbanization, tourism, and aquaculture can also be linked with eutrophication, which leads to seagrass degradation (e.g., Thomsen et al., 2020), which a few those anthropogenic activities have been occurring in Indonesia for decades.
At a regional level, the pattern of seagrass dispersal in Indonesia was observed to be partly influenced by contemporary ocean currents. Asymmetrical connectivity among seagrass populations follows a pattern of westward and southward migration, with seagrass in eastern Indonesia as the main source of migration. The flowing pattern is congruent to major ocean currents across the Indonesian region, e.g., Indonesian Throughflow and South Java Current (Hernawan et al., 2017). However, this flowing pattern was based only on one species, i.e., Thalassia hemprichii. Yet, there are 16 species recorded in Indonesia’s waters (Fortes, 2018) that might exhibit different dispersal patterns as these species have various traits of their life history stages. For example, Thalassia hemprichii has fruits and seeds that can float for a particular time, enabling long-distance dispersal. In contrast, the fruits and seeds of other species, like Halophila ovalis do not float (Wu et al., 2016). Under similar environmental conditions, the different dispersal strategies might result in different patterns of seagrass movement. Hence, the influence of various atmospheric-oceanic forcings, like the Asian monsoon system, on the behavior of hydrodynamic regimes within the Indonesian region needs to be considered to enhance the accuracy of seagrass dispersal. Future research should investigate the interaction between ocean hydrodynamics and various key traits of seagrass life-history stages inducing the movement of seagrass and the consequences to seagrass ecology.
4 Discussion
Seagrasses in Indonesia are among the most diverse in the world, with various benefits and goods for ecosystems and human well-being. However, the condition and spatial extent of seagrass ecosystems in the country are declining due to natural and anthropogenic stressors (Unsworth et al., 2018). The current conditions have stimulated conservation and restoration projects across the country with the support of various stakeholders, including the Indonesian government, non-government organizations, and the seagrass scientific community (e.g., Ambo-Rappe 2022). Unfortunately, seagrass research in Indonesia to support conservation and restoration projects is dominated by biological and ecological aspects. The interactions between hydrodynamics and seagrass ecosystems are less investigated. Moreover, based on our document search, there were no publications about Indonesian seagrass site suitability evaluation that includes hydrodynamics assessment in supporting the effectiveness of conservation and restoration projects. This finding suggests the lack of community awareness of the impacts of these parameters on the success rates of conservation and restoration measures.
We identified several constraints that limit the knowledge of seagrass hydrodynamics and related themes in Indonesia. The biggest hindrance is that Indonesian communities have low awareness of the important benefits of seagrass ecosystems (Rifai et al., 2022a). There are even areas in the country where people are not aware of seagrasses’ benefits or what they are (Nadiarti et al., 2012). Consequently, this led to limited public support for conservation and restoration projects of seagrasses in Indonesia. Another issue is the limited budget allotted for seagrass research topics. In Indonesia, seagrass studies are still considered non-profit projects with relatively small budgets compared to those for-profit ones like oil and gas or port development-related studies. The limited budget hinders the progress of seagrass research in the country because the equipment needed for investigating the hydrodynamic parameters is costly. For instance, the price of wave gauges or current meters is thousands of USD. Meanwhile, the rental price of such devices in Indonesia is more than 100 USD per day. The stated prices do not yet include the expenses for installation and surveillance against vandalism, which cost more than a thousand USD per day. Similarly, numerical and/or physical models are not cheap methods to be carried out in Indonesia, where facilities are lacking or unequipped to do so. Numerical models in complex beach profiles like seagrass ecosystems are more challenging due to large bottom roughness than sandy beaches (e.g., Ruiz-Montoya et al., 2015). More comprehensive processes of hydrodynamic parameters, e.g., IG waves and runup, could be included in the simulations using phase-resolving wave models. This type of model offers greater detail of the physical processes across the model domains that phase-averaged models cannot solve (e.g., Passarella et al., 2020). Yet, applying phase-resolving simulations requires significant computational resources that imply an increase in the overall research budget (David et al., 2022). Likewise, the application of physical models in wave flumes for flexible vegetation like seagrass costs a bundle consisting of operational and material expenditures (Mattis et al., 2019).
Other constraints included the lack of oceanographic equipment, wave flume/basin facilities, and human resources to research seagrass hydrodynamic-related topics in Indonesia. Besides the high price (to buy or rent), the availability of oceanographic devices in Indonesia to measure long-duration and accurate waves and/or currents behaviors is limited relative to the vast Indonesian coastal region. Due to the presence of seagrasses in nearshore zones that causes disturbance in the waves and flows, more accurate devices should be used. Therefore, the use of current meters with the ability to measure flows across whole water columns like ADCP (Acoustic Doppler Current Profiler) is not recommended. Instead, ADV (Acoustic Doppler Velocimeter), which produces higher resolution at a specific point within water columns and, thus, could give more accurate results, should be deployed (Horstman et al., 2011). Yet, based on our experience, this device is scarce in Indonesia. Moreover, Indonesia’s wave flume or basin facilities are limited in size and capacity to model small and flexible structures like seagrasses. Hence, the lack of facilities for physical models may cause issues related to the required similitudes, such as geometric, kinematic, and dynamic, affecting the accuracy of the models (e.g., Pranesh and Mani 1988; Zhang and Geng 2015). The lack of awareness, budget, and proper devices contributed to the limited number of local experts doing seagrass research. Consequently, this resulted in a limited number of literature on the interaction between seagrasses and hydrodynamics in Indonesia.
The most critical step to overcome these constraints is increasing stakeholders’ awareness of the significance of seagrasses. Additionally, there is a need to improve their knowledge of the interaction between hydrodynamics and seagrass ecosystems. By doing so, it can potentially encourage stakeholders to allocate more funds for seagrass hydrodynamics research and its related topics. Another pathway is to capacitate local scholars on the topic, including developing low-budget alternatives to hydrodynamic investigation and modeling and constructing low-cost parallel computers with high-performance computation capabilities (e.g., Costa et al., 2009; Jin et al., 2011). An example is the Arduino-based devices to record wave information (Lyman et al., 2020; Perales and Herman 2020; Eidam et al., 2022). Overall, if these constraints are addressed, they could further improve predictions on nearshore hydrodynamics impacts on seagrass ecosystems as well as progress on implementation strategies for seagrass restoration projects and natural protection in Indonesia.
5 Conclusion
Seagrasses are greatly beneficial to coastal ecosystems and play a multi-functional role in supporting human wellbeing. Besides their provisioning services, such as food and livelihood sources, seagrasses can also dissipate wave energy and modify the mean flow field. Thus, the conservation and protection of seagrass meadows is also a potential Nature-based Solution to protect shorelines from excessive erosion in addition to addressing societal challenges (Rifai et al., 2022b). Moreover, the hydrodynamics within seagrass meadows play significant roles in controlling ecological processes as documented in this review. Most of the existing publications discussed seagrasses in temperate zones, while those from tropical zones were limited. This gap is critical since the characteristics of seagrass meadows and hydrodynamics could be different between these zones such as those found in the Indonesian region. Nevertheless, the methodologies developed in studying the interaction between seagrasses and hydrodynamics of temperate zones could be applicable in Indonesian areas. Overall, there is a knowledge gap in assessing the interaction of Indonesian seagrasses’ physical characteristics with nearshore hydrodynamics. The correlation between the seagrasses and the atmospheric-oceanic forcing (particularly the Asian monsoon system) within the region and the inducing impacts on the shoreline variability, biogeochemical, and dispersal processes remain undocumented.
Indonesia, the largest archipelago in the world located in a tropical region, has a variety of parameters (physical, biological, chemical, and anthropogenic) that could potentially affect the ecology of seagrasses that is different from temperate regions. Hence, there is an opportunity for the region to be a site for tropical seagrass investigations. Considering the potential benefits of seagrasses and their widespread abundance in Indonesia, understanding the interaction between seagrasses and hydrodynamics within the region is critical to managing the ecosystem better. Yet, many limitations (e.g., lack of knowledge on seagrass importance) prevent this from occurring. Therefore, relevant stakeholders in Indonesia need to strengthen their collaborations to improve the overall understanding and knowledge of seagrass ecosystems.
Author contributions
JR, HR, CS, and KL Conceptualized the idea, designed the images, and wrote the draft and the revised manuscript. LM Designed the images and wrote the draft of the manuscript. UH, JQ, RH, RA-R, ML, RK, and KN Wrote the draft of the manuscript.
Funding
BRIN Research Scheme DIPA OR KM BRIN. No: SP DIPA-124.01.1.690501/2022 dated 17 November 2021; Rumah Program Kebencanaan-OR Kebumian & Maritim BRIN-2022; Riset Pengembangan Kapasitas (RPK) COREMAP CTI-LIPI [5942. SDA.001]-2021; Peserta Program Pengembangan Kapasitas Sumber Daya Manusia Melalui Mobilitas Periset BRIN, Program Post-Doctoral, No: 64/II/HK/2022; Japan Science and Technology Agency (JST) and Japan International Cooperation Agency (JICA) through the Science and Technology Research Partnership for Sustainable Development Program (SATREPS)—Comprehensive Assessment and Conservation of Blue Carbon Ecosystems and Their Services in the Coral Triangle (BlueCARES) project; Asia-Pacific Network for Global Change Research Grant Number CBA 2020-05SY-RK; and JSPS KAKENHI Grant Numbers JP22H03852; JP21K18456; JP20K12398; JP17K02105.
Acknowledgments
The authors are grateful to the Research Center for Oceanography and Marine Research Center for the support.
Conflict of interest
The authors declare that the research was conducted in the absence of any commercial or financial relationships that could be construed as a potential conflict of interest.
Publisher’s note
All claims expressed in this article are solely those of the authors and do not necessarily represent those of their affiliated organizations, or those of the publisher, the editors and the reviewers. Any product that may be evaluated in this article, or claim that may be made by its manufacturer, is not guaranteed or endorsed by the publisher.
References
Abdelrhman, M. A. (2007). Modeling coupling between eelgrass Zostera marina and water flow. Mar. Ecol. Prog. Ser. 338, 81–96. doi:10.3354/meps338081
Abdolahpour, M., Ghisalberti, M., McMahon, K., and Lavery, P. S. (2018). The impact of flexibility on flow, turbulence, and vertical mixing in coastal canopies. Limnol. Oceanogr. 63 (6), 2777–2792. doi:10.1002/lno.11008
Abdolahpour, M., Hambleton, M., and Ghisalberti, M. (2017). The wave-driven current in coastal canopies. J. Geophys. Res. Oceans 122 (5), 3660–3674. doi:10.1002/2016JC012446
Ackerman, J. D., and Okubo, A. (1993). Reduced mixing in a marine macrophyte canopy. Funct. Ecol. 9, 305. doi:10.2307/2390209
Alcoverro, T., Manzanera, M., and Romero, J. (2001). Annual metabolic carbon balance of the seagrass Posidonia oceanica: The importance of carbohydrate reserves. Mar. Ecol. Prog. Ser. 211, 105–116. doi:10.3354/meps211105
Alongi, D. M., Murdiyarso, D., Fourqurean, J. W., Kauffman, J. B., Hutahaean, A., Crooks, S., et al. (2016). Indonesia’s blue carbon: A globally significant and vulnerable sink for seagrass and mangrove carbon. Wetl. Ecol. Manag. 24 (1), 3–13. doi:10.1007/s11273-015-9446-y
Ambo-Rappe, R. (2022). The success of seagrass restoration using Enhalus acoroides seeds is correlated with substrate and hydrodynamic conditions. J. Environ. Manag. 310, 114692. doi:10.1016/j.jenvman.2022.114692
Anarde, K., Figlus, J., Sous, D., and Tissier, M. (2020). Transformation of infragravity waves during Hurricane overwash. J. Mar. Sci. Eng. 8 (8), 545. doi:10.3390/jmse8080545
Anton, A., Just, C., Duarte, C. M., Heck, K. L., and Goff, J. (2009). Low impact of Hurricane Katrina on seagrass community structure and functioning in the northern gulf of Mexico. Bull. Mar. Sci. 85 (1), 45–59.
Athanasiou, P., van Dongeren, A., Giardino, A., Vousdoukas, M. I., Ranasinghe, R., and Kwadijk, J. (2020). Uncertainties in projections of sandy beach erosion due to Sea Level rise: An analysis at the European scale. Sci. Rep. 10 (1), 11895–11914. doi:10.1038/s41598-020-68576-0
Basit, A., Mulyadi, H. A., Pitriana, P., Putri, M. R., Mayer, B., and Pohlmann, T. (2021). Hydrodynamic conditions of the Banda and northern Arafura seas in the northwest monsoon (Februay2014). Mar. Res. Indonesia 46 (1). doi:10.14203/mri.v46i1.599
Battjes, J. A., and Janssen, J. P. F. M. (1978). Energy loss and set-up due to breaking of random waves. Coast. Eng. 1978, 569–587.
Becker, J. M., Merrifield, M. A., and Yoon., H. (2016). Infragravity waves on fringing reefs in the tropical pacific: Dynamic setup. J. Geophys. Res. Oceans 121 (5), 3010–3028. doi:10.1002/2015jc011516
Berković, B., Cabaco, S., Barrio, J. M., Santos, R., Serrão, E. A., and Alberto, F. (2014). Extending the life history of a clonal aquatic plant: Dispersal potential of sexual and asexual propagules of Zostera noltii. Aquat. Bot. 113, 123–129. doi:10.1016/j.aquabot.2013.10.007
Bos, A. R., Bouma, T. J., de Kort, G. L. J., and van Katwijk, M. M. (2007). Ecosystem engineering by annual intertidal seagrass beds: Sediment accretion and modification. Estuar. Coast. Shelf Sci. 74 (1–2), 344–348. doi:10.1016/j.ecss.2007.04.006
Bradley, K., and Houser, C. (2009). Relative velocity of seagrass blades: Implications for wave attenuation in low-energy environments. J. Geophys. Res. Earth Surf. 114 (F1), F01004. doi:10.1029/2007jf000951
Bressan, A., and Constantin, A. (2019). The deflection angle of surface ocean currents from the wind direction. J. Geophys. Res. Oceans 124 (11), 7412–7420. doi:10.1029/2019jc015454
Bruun, P. (1962). Sea-level rise as a cause of shore erosion. J. Waterw. Harb. Div. 88 (1), 117–130. doi:10.1061/jwheau.0000252
Bryan, K. R., Tay, H. W., Pilditch, C. A., Lundquist, C. J., and Hunt, H. L. (2007). The effects of seagrass (Zostera muelleri) on boundary-layer hydrodynamics in whangapoua estuary, New Zealand. J. Coast. Res. 2007, 668–672.
Bulthuis, D. A., and Woelkerling, W. J. (1983). Seasonal variation in standing crop, density and leaf growth rate of the seagrass, heterozostera tasmanica, in western port and port phillip bay, victoria, Australia. Aquat. Bot. 16 (2), 111–136. doi:10.1016/0304-3770(83)90088-8
Burkholder, J. A. M., Tomasko, D. A., and Touchette, B. W. (2007). Seagrasses and eutrophication. J. Exp. Mar. Biol. Ecol. 350 (1–2), 46–72. doi:10.1016/j.jembe.2007.06.024
Cabaço, S., Santos, R., and Carlos, M. D. (2008). The impact of sediment burial and erosion on seagrasses: A review. Estuar. Coast. Shelf Sci. 79 (3), 354–366. doi:10.1016/j.ecss.2008.04.021
Carlson, P. R., Yarbro, L. A., Kaufman, K. A., and Mattson, R. A. (2010). Vulnerability and resilience of seagrasses to Hurricane and runoff impacts along Florida’s west coast. Hydrobiologia 649 (1), 39–53. doi:10.1007/s10750-010-0257-0
Carr, J. A., D’Odorico, P., McGlathery, K. J., and Wiberg, P. L. (2012). Stability and resilience of seagrass meadows to seasonal and interannual dynamics and environmental stress. J. Geophys. Res. Biogeosciences 117 (G1). doi:10.1029/2011JG001744
Carr, J., Mariotti, G., Fahgerazzi, S., McGlathery, K., and Wiberg, P. (2018). Exploring the impacts of seagrass on coupled marsh-tidal flat morphodynamics. Front. Environ. Sci. 6, 92. doi:10.3389/fenvs.2018.00092
Chen, B., Qin, H., Chen, G., and Xue, H. (2019). Ocean salinity as a precursor of summer rainfall over the East asian monsoon region. J. Clim. 32 (17), 5659–5676. doi:10.1175/jcli-d-18-0756.1
Chen, S. N., Sanford, L. P., Koch, E. W., Shi, F., and North, E. W. (2007). A nearshore model to investigate the effects of seagrass bed geometry on wave attenuation and suspended sediment transport. Estuaries Coasts 30 (2), 296–310. doi:10.1007/bf02700172
Chen, W. L., Muller, P., Grabowski, R. C., and Dodd, N. (2022). Green nourishment: An innovative nature-based solution for coastal erosion. Front. Mar. Sci. 8, 814589. doi:10.3389/fmars.2021.814589
Cheriton, O. M., Storlazzi, C. D., and Rosenberger, K. J. (2016). Observations of wave transformation over a fringing coral reef and the importance of low-frequency waves and offshore water levels to runup, overwash, and coastal flooding. J. Geophys. Res. Oceans 121 (5), 3121–3140. doi:10.1002/2015jc011231
Christianen, M. J. A., van Belzen, J., Herman, P. M. J., van Katwijk, M. M., Lamers, L. P. M., van Leent, P. J. M., et al. (2013). Low-canopy seagrass beds still provide important coastal protection services. PloS One 8 (5), e62413. doi:10.1371/journal.pone.0062413
Contti Neto, N., Pomeroy, A., Lowe, R., and Ghisalberti, M. (2022). Seagrass meadows reduce wind-wave driven sediment resuspension in a sheltered environment. Front. Mar. Sci. 8, 733542. doi:10.3389/fmars.2021.733542
Costa, M., Oliveira, A., Rodrigues, M., and Azevedo, A. (2009). Application of parallel, high-performance computing in coastal environmental modeling: Circulation and ecological dynamics in the Portuguese coast. Proc. Da 3rd Iber. Grid Infrastruture 2009, 375–386.
Côté-Laurin, M. C., Benbow, S., and Karim, E. (2017). The short-term impacts of a cyclone on seagrass communities in southwest Madagascar. Cont. Shelf Res. 138, 132–141. doi:10.1016/j.csr.2017.03.005
Cruz-Palacios, V., and van Tussenbroek, B. I. (2005). Simulation of hurricane-like disturbances on a caribbean seagrass bed. J. Exp. Mar. Biol. Ecol. 324 (1), 44–60. doi:10.1016/j.jembe.2005.04.002
Dahl, M., Asplund, M. E., Björk, M., Deyanova, D., Infantes, E., Isaeus, M., et al. (2020). The influence of hydrodynamic exposure on carbon storage and nutrient retention in eelgrass (Zostera marina L.) meadows on the Swedish skagerrak coast. Sci. Rep. 10 (1), 13666. doi:10.1038/s41598-020-70403-5
Dalrymple, R. A., Kirby, J. T., and Hwang, P. A. (1984). Wave diffraction due to areas of energy dissipation. J. Waterw. Port, Coast. Ocean Eng. 110 (1), 67–79. doi:10.1061/(asce)0733-950x(1984)110:1(67)
David, D. R., Rijnsdorp, D. P., Hansen, J. E., Lowe, R. J., and Buckley, M. L. (2022). Predicting coastal impacts by wave farms: A comparison of wave-averaged and wave-resolving models. Renew. Energy 183, 764–780. doi:10.1016/j.renene.2021.11.048
de la Torre-Castro, M., and Rönnbäck, P. (2004). Links between humans and seagrasses—An example from tropical East Africa. Ocean Coast. Manag. 47 (7–8), 361–387. doi:10.1016/j.ocecoaman.2004.07.005
Donatelli, C., Ganju, N. K., Singh Kalra, T., Fagherazzi, S., and Leonardi, N. (2019). Changes in hydrodynamics and wave energy as a result of seagrass decline along the shoreline of a microtidal back-barrier estuary. Adv. Water Resour. 128, 183–192. doi:10.1016/j.advwatres.2019.04.017
Duarte, C. M. (1991a). Allometric scaling of seagrass form and productivity. Mar. Ecol. Prog. Ser. Oldend. 77 (2), 289–300. doi:10.3354/meps077289
Duarte, C. M., Kennedy, H., Marbà, N., and Hendriks, I. (2013). Assessing the capacity of seagrass meadows for carbon burial: Current limitations and future strategies. Ocean Coast. Manag. 83, 32–38. doi:10.1016/j.ocecoaman.2011.09.001
Duarte, C. M., and Krause-Jensen., D. (2017). Export from seagrass meadows contributes to marine carbon sequestration. Front. Mar. Sci. 4, 13. doi:10.3389/fmars.2017.00013
Duarte, C. M. (1991b). Seagrass depth limits. Aquat. Bot. 40 (4), 363–377. doi:10.1016/0304-3770(91)90081-F
Duarte, C. M. (1989). Temporal biomass variability and production/biomass relationships of seagrass communities. Mar. Ecol. Prog. Ser. Oldend. 51 (3), 269–276. doi:10.3354/meps051269
Duarte, C. M. (2002). The future of seagrass meadows. Environ. Conserv. 29 (2), 192–206. doi:10.1017/s0376892902000127
Dumbauld, B. R., Graham, E. R., McCoy, L. M., and Lewis, N. S. (2022). Predicted changes in seagrass cover and distribution in the face of Sea Level rise: Implications for bivalve aquaculture in a US west coast estuary. Estuaries Coasts 45, 1823–1841. doi:10.1007/s12237-022-01060-2
Egea, L. G., Jiménez-Ramos, R., Hernández, I., Bouma, T. J., and Brun, F. G. (2018). Effects of ocean acidification and hydrodynamic conditions on carbon metabolism and dissolved organic carbon (DOC) fluxes in seagrass populations. PLOS ONE 13 (2), e0192402. doi:10.1371/journal.pone.0192402
Eidam, E. F., Langhorst, T., Goldstein, E. B., and McLean, M. (2022). OpenOBS: Open-source, low-cost optical backscatter sensors for water quality and sediment-transport research. Limnol. Oceanogr. Methods 20 (1), 46–59. doi:10.1002/lom3.10469
El-Hacen, E. H. M., Bouma, T. J., Govers, L. L., Piersma, T., and Han, O. (2019). Seagrass sensitivity to collapse along a hydrodynamic gradient: Evidence from a pristine subtropical intertidal ecosystem. Ecosystems 22 (5), 1007–1023. doi:10.1007/s10021-018-0319-0
Erftemeijer, P. L. A., and Herman, P. M. J. (1994). Seasonal changes in environmental variables, biomass, production and nutrient contents in two contrasting tropical intertidal seagrass beds in South Sulawesi, Indonesia. Oecologia 99 (1), 45–59. doi:10.1007/bf00317082
Esteban, N., Unsworth, R. K. F., Gourlay, J. B. Q., and Hays, G. C. (2018). The discovery of deep-water seagrass meadows in a pristine Indian ocean wilderness revealed by tracking green turtles. Mar. Pollut. Bull. 134, 99–105. doi:10.1016/j.marpolbul.2018.03.018
Feddersen, F., Gallagher, E. L., Guza, R. T., and Elgar, S. (2003). The drag coefficient, bottom roughness, and wave-breaking in the nearshore. Coast. Eng. 48 (3), 189–195. doi:10.1016/s0378-3839(03)00026-7
Ferguson, A. J. P., Gruber, R., Orr, M., and Scanes, P. (2016). Morphological plasticity in Zostera muelleri across light, sediment, and nutrient gradients in Australian temperate coastal lakes. Mar. Ecol. Prog. Ser. 556 (1), 91–104. doi:10.3354/meps11830
Finnigan, J. (2000). Turbulence in plant canopies. Annu. Rev. Fluid Mech. 32 (1), 519–571. doi:10.1146/annurev.fluid.32.1.519
Fonseca, M. S., Fisher, J. S., Zieman, J. C., and Thayer, G. W. (1982). Influence of the seagrass, Zostera marina L., on current flow. Estuar. Coast. Shelf Sci. 15 (4), 351–364. doi:10.1016/0272-7714(82)90046-4
Fonseca, M. S., and Cahalan, J. A. (1992). A preliminary evaluation of wave attenuation by four species of seagrass. Estuar. Coast. Shelf Sci. 35 (6), 565–576. doi:10.1016/s0272-7714(05)80039-3
Fonseca, M. S., Koehl, M. A. R., and Kopp, B. S. (2007). Biomechanical factors contributing to self-organization in seagrass landscapes. J. Exp. Mar. Biol. Ecol. 340 (2), 227–246. doi:10.1016/j.jembe.2006.09.015
Fonseca, M. S., Zieman, J. C., Thayer, G. W., and Fisher, J. S. (1983). The role of current velocity in structuring eelgrass (Zostera marina L.) meadows. Estuar. Coast. Shelf Sci. 17 (4), 367–380. doi:10.1016/0272-7714(83)90123-3
Fortes, M. D., Ooi, J. L. S., Tan, Y. M., Bujang, J. S., Yaakub, S. M., Ooi, J. L. S., et al. (2018). Seagrass in southeast Asia: A review of status and knowledge gaps, and a road map for conservation. Bot. Mar. 61 (3), 269–288. doi:10.1515/bot-2018-0008
Fu, L. L. (2009). Pattern and velocity of propagation of the global ocean eddy variability. J. Geophys. Res. Oceans 114 (C11), C11017. doi:10.1029/2009jc005349
Gacia, E., Granata, T. C., and Duarte, C. M. (1999). An approach to measurement of particle flux and sediment retention within seagrass (Posidonia oceanica) meadows. Aquat. Bot. 65 (1–4), 255–268. doi:10.1016/s0304-3770(99)00044-3
Ganthy, F., Soissons, L., Sauriau, P. G., Verney, R., and Sottolichio, A. (2015). Effects of short flexible seagrass Zostera noltei on flow, erosion and deposition processes determined using flume experiments. Sedimentology 62 (4), 997–1023. doi:10.1111/sed.12170
Gera, A., Pages, J. F., Arthur, R., Farina, S., Roca, G., Romero, J., et al. (2014). The effect of a centenary storm on the long-lived seagrass Posidonia oceanica. Limnol. Oceanogr. 59 (6), 1910–1918. doi:10.4319/lo.2014.59.6.1910
Ghisalberti, M., and Nepf, H. M. (2002). Mixing layers and coherent structures in vegetated aquatic flows. J. Geophys. Res. Oceans 107 (C2), 3011–3013. doi:10.1029/2001jc000871
Ghisalberti, M., and Nepf, H. (2009). Shallow flows over a permeable medium: The hydrodynamics of submerged aquatic canopies. Transp. Porous Media 78 (2), 385–402. doi:10.1007/s11242-009-9434-x
Ghisalberti, M., and Nepf, H. (2006). The structure of the shear layer in flows over rigid and flexible canopies. Environ. Fluid Mech. 6 (3), 277–301. doi:10.1007/s10652-006-0002-4
Gillis, L. G., Bouma, T. J., Kiswara, W., Ziegler, A. D., and Herman, P. M. J. (2014). Leaf transport in mimic mangrove forests and seagrass beds. Mar. Ecol. Prog. Ser. 498, 95–102. doi:10.3354/meps10615
Gordon, A. L., and McClean, J. L. (1999). Thermohaline stratification of the Indonesian seas: Model and observations. J. Phys. Oceanogr. 29 (2), 198–216. doi:10.1175/1520-0485(1999)029<0198:TSOTIS>2.0.CO;2
Gordon, A. L., Dwi Susanto, R., and Vranes, K. (2003). Cool Indonesian Throughflow as a consequence of restricted surface layer flow. Nature 425 (6960), 824–828. doi:10.1038/nature02038
Gordon, A. L. (2005). Oceanography of the Indonesian seas and their Throughflow. Oceanography 18 (4), 14–27. doi:10.5670/oceanog.2005.01
Green, E. P., and Short, F. T. (2003). World atlas of seagrasses. Berkeley, CA, United States: Univ of California Press.
Greve, T. M., and Binzer, T. (2004). Which factors regulate seagrass growth and distribution. Eur. Seagrasses Introd. Monit. Manag. 19.
Grizzle, R. E., Short, F. T., Newell, C. R., Hoven, H., Kindblom, L., Hoven, H., et al. (1996). Hydrodynamically induced synchronous waving of Seagrasses:‘Monami’and its possible effects on larval mussel settlement. J. Exp. Mar. Biol. Ecol. 206 (1–2), 165–177. doi:10.1016/s0022-0981(96)02616-0
Hansen, J. C. R., and Reidenbach., M. A. (2013). Seasonal growth and senescence of a Zostera marina seagrass meadow alters wave-dominated flow and sediment suspension within a coastal bay. Estuaries Coasts 36 (6), 1099–1114. doi:10.1007/s12237-013-9620-5
Hayes, M. A., McClure, E. C., York, P. H., Jinks, K. I., Rasheed, M. A., Sheaves, M., et al. (2020). The differential importance of deep and shallow seagrass to nekton assemblages of the great barrier reef. Diversity 12 (8), 292. doi:10.3390/d12080292
Hernawan, U. E., van Dijk, K. J., Kendrick, G. A., Ming, F., Biffin, E., Lavery, P. S., et al. (2017). Historical processes and contemporary ocean currents drive genetic structure in the seagrass T halassia hemprichii in the indo-Australian archipelago. Mol. Ecol. 26 (4), 1008–1021. doi:10.1111/mec.13966
Hessing-Lewis, M. L., Hacker, S. D., Menge, B. A., McConville, S. O., and Henderson, J. (2015). Sea-oh McConville, and jeremy HendersonAre large macroalgal blooms necessarily bad? Nutrient impacts on seagrass in upwelling-influenced estuaries. Ecol. Appl. 25 (5), 1330–1347. doi:10.1890/14-0548.1
Holthuijsen, L. H. (2010). Waves in oceanic and coastal waters. Cambridge, UK: Cambridge University Press.
Horstman, E., Balke, T., Bouma, T., Dohmen-Janssen, M., and Hulscher, S. (2011). Optimizing methods to measure hydrodynamics in coastal wetlands: Evaluating the use and positioning of ADV, ADCP and HR-ADCP. Coast. Eng. Proc. 32, 51. doi:10.9753/icce.v32.waves.51, no.
Houseago, R. C., Liu, H., Cheng, S., Best, J. L., Parsons, D. R., and Chamorro, L. P. (2022). On the turbulence dynamics induced by a surrogate seagrass canopy. J. Fluid Mech. 934, A17. doi:10.1017/jfm.2021.1142
Hyndes, G. A., Nagelkerken, I., McLeod, R. J., Connolly, R. M., Lavery, P. S., and Vanderklift, M. A. (2014). Mechanisms and ecological role of carbon transfer within coastal seascapes. Biol. Rev. 89 (1), 232–254. doi:10.1111/brv.12055
Infantes, E., Orfila, A., Simarro, G., Terrados, J., Luhar, M., and Nepf, H. (2012). Effect of a seagrass (Posidonia oceanica) meadow on wave propagation. Mar. Ecol. Prog. Ser. 456, 63–72. doi:10.3354/meps09754
James, R. K., Lynch, A., Herman, P. M. J., van Katwijk, M. M., van Tussenbroek, B. I., Dijkstra, H. A., et al. (2021). Tropical biogeomorphic seagrass landscapes for coastal protection: Persistence and wave attenuation during major storms events. Ecosystems 24 (2), 301–318. doi:10.1007/s10021-020-00519-2
James, R. K., Silva, R., van Tussenbroek, B. I., Escudero-Castillo, M., Mariño-Tapia, I., Dijkstra, H. A., et al. (2019). Maintaining tropical beaches with seagrass and algae: A promising alternative to engineering solutions. BioScience 69 (2), 136–142. doi:10.1093/biosci/biy154
Jin, H., Jespersen, D., Mehrotra, P., Biswas, R., Huang, L., and Chapman, B. (2011). High performance computing using MPI and OpenMP on multi-core parallel systems. Parallel Comput. 37 (9), 562–575. doi:10.1016/j.parco.2011.02.002
John, B. M., Shirlal, K. G., Rao, S., and Rajasekaran, C. (2016). Effect of artificial seagrass on wave attenuation and wave run-up. Int. J. Ocean Clim. Syst. 7 (1), 14–19. doi:10.1177/1759313115623163
Kawada, Y., Watanabe, S., and Yoshida, S. (2006). “The role of interactions between the thermohaline circulation and oceanic carbon cycle-A case study of nagoya simple climate model,” in AGU Fall Meeting Abstracts, PP23B–1757.
Kawaroe, M., Nugraha, A., Juraij, J., and Tasabaramo, I. (2016). Seagrass biodiversity at three marine ecoregions of Indonesia: Sunda shelf, Sulawesi sea, and Banda Sea. Biodiversitas, J. Biol. Divers. 17 (5), 585–591. doi:10.13057/biodiv/d170228
Kendrick, G. A., Waycott, M., Carruthers, T. J. B., Cambridge, M. L., Hovey, R., Krauss, S. L., et al. (2012). The central role of dispersal in the maintenance and persistence of seagrass populations. Bioscience 62 (1), 56–65. doi:10.1525/bio.2012.62.1.10
Kilminster, K., McMahon, K., Waycott, M., Kendrick, G. A., Scanes, P., McKenzie, L., et al. (2015). Unravelling complexity in seagrass systems for management: Australia as a microcosm. Sci. Total Environ. 534, 97–109. doi:10.1016/j.scitotenv.2015.04.061
Kim, S. H., Suonan, Z., Le-Zheng, Q., Kim, H., Park, J. I., Young Kim, K., et al. (2022). Variability in blue carbon storage related to biogeochemical factors in seagrass meadows off the coast of the Korean peninsula. Sci. Total Environ. 813, 152680. doi:10.1016/j.scitotenv.2021.152680
Kim, S. Y., Yasuda, T., and Mase, H. (2010). Wave set-up in the storm surge along open coasts during typhoon anita. Coast. Eng. 57 (7), 631–642. doi:10.1016/j.coastaleng.2010.02.004
Koch, E. W., and Gust, G. (1999). Water flow in tide-and wave-dominated beds of the seagrass Thalassia testudinum. Mar. Ecol. Prog. Ser. 184, 63–72. doi:10.3354/meps184063
Koch, E. W., Sanford, L. P., Chen, S. N., Shafer, D. J., and Smith, J. M. (2006). Waves in seagrass systems: Review and technical recommendations.
Koftis, T., Prinos, P., and Stratigaki, V. (2013). Wave damping over artificial Posidonia oceanica meadow: A large-scale experimental study. Coast. Eng. 73, 71–83. doi:10.1016/j.coastaleng.2012.10.007
Krause-Jensen, D., and Duarte, C. M. (2016). Substantial role of macroalgae in marine carbon sequestration. Nat. Geosci. 9 (10), 737–742. doi:10.1038/ngeo2790
Kuo, J. (2007). New monoecious seagrass of Halophila sulawesii (hydrocharitaceae) from Indonesia. Aquat. Bot. 87 (2), 171–175. doi:10.1016/j.aquabot.2007.04.006
Kurniawan, F., Imran, Z., Darus, R. F., Anggraeni, F., Damar, A., Sunuddin, A., et al. (2020). Rediscovering Halophila major (zollinger) miquel (1855) in Indonesia. Aquat. Bot. 161, 103171. doi:10.1016/j.aquabot.2019.103171
Kurniawan, R., and Khotimah., M. K. (2016). Ocean wave characteristics in Indonesian waters for sea transportation safety and planning. IPTEK J. Technol. Sci. 26 (1). doi:10.12962/j20882033.v26i1.767
Lai, S., Yaakub, S. M., Poh, T. S. M., Bouma, T. J., and Todd, P. A. (2018). Unlikely nomads: Settlement, establishment, and dislodgement processes of vegetative seagrass fragments. Front. Plant Sci. 9, 160. doi:10.3389/fpls.2018.00160
Lanuru, M., Ambo-Rappe, R., Amri, K., and Williams, S. L. (2018). Hydrodynamics in indo-pacific seagrasses with a focus on short canopies. Bot. Mar. 61 (1), 1–8. doi:10.1515/bot-2017-0037
Legg, S. (2021). IPCC, 2021: Climate change 2021-the physical science basis. Interaction 49 (4), 44–45.
Lei, J., and Nepf, H. (2019). Wave damping by flexible vegetation: Connecting individual blade dynamics to the meadow scale. Coast. Eng. 147, 138–148. doi:10.1016/j.coastaleng.2019.01.008
Longuet-Higgins, M. S., and Stewart, R. W. (1964). Radiation stresses in water waves; a physical discussion, with applications. Deep Sea Res. Oceanogr. Abstr. 11, 529–562. doi:10.1016/0011-7471(64)90001-4
Lowe, R. J., Koseff, J. R., and Monismith, S. G. (2005). Oscillatory flow through submerged canopies: 1. Velocity structure. J. Geophys. Res. Oceans 110 (C10), C10016. doi:10.1029/2004JC002788
Luhar, M., Infantes, E., and Nepf., H. (2017). Seagrass blade motion under waves and its impact on wave decay. J. Geophys. Res. Oceans 122 (5), 3736–3752. doi:10.1002/2017jc012731
Luhar, M., Infantes, E., Orfila, A., Terrados, J., and Nepf, H. M. (2013). Field observations of wave-induced streaming through a submerged seagrass (Posidonia oceanica) meadow. J. Geophys. Res. Oceans 118 (4), 1955–1968. doi:10.1002/jgrc.20162
Luhar, M., and Nepf, H. M. (2011). Flow-induced reconfiguration of buoyant and flexible aquatic vegetation. Limnol. Oceanogr. 56 (6), 2003–2017. doi:10.4319/lo.2011.56.6.2003
Luhar, M., and Nepf, H. M. (2016). Wave-induced dynamics of flexible blades. J. Fluids Struct. 61, 20–41. doi:10.1016/j.jfluidstructs.2015.11.007
Lukman, K. M., Uchiyama, Y., Quevedo, J. M. D., and Harding, D. (2021). Yuta uchiyama, jay mar D quevedo, diana harding, and ryo KohsakaLand use changes assessment using a triangulated framework: Perception interviews, land-use/land cover observation, and spatial planning analysis in tanjung batu and derawan island, Indonesia. Hum. Ecol. 49 (5), 551–564. doi:10.1007/s10745-021-00253-w
Lyman, T. P., Elsmore, K., Gaylord, B., Byrnes, J. E. K., and Miller, L. P. (2020). Open wave height logger: An open source pressure sensor data logger for wave measurement. Limnol. Oceanogr. Methods 18 (7), 335–345. doi:10.1002/lom3.10370
Manca, E., Cáceres, I. J. V. I., Alsina, J. M., Stratigaki, V., Townend, I., and Amos, C. L. (2012). Wave energy and wave-induced flow reduction by full-scale model Posidonia oceanica seagrass. Cont. Shelf Res. 50, 100–116. doi:10.1016/j.csr.2012.10.008
Manca, E., Stratigaki, V., and Prinos, P. (2010). Large scale experiments on spectral wave propagation over Posidonia oceanica seagrass. Proc. 6th Int. Symposium Environ. Hydraulics 1, 463–469.
Mattis, S. A., Kees, C. E., Wei, M. V., Dimakopoulos, A., and Dawson, C. N. (2019). Computational model for wave attenuation by flexible vegetation. J. Waterw. Port, Coast. Ocean Eng. 145 (1). doi:10.1061/(asce)ww.1943-5460.0000487
Mazarrasa, I., Paul, L., Duarte, C. M., Lafratta, A., Lovelock, C. E., Macreadie, P. I., et al. (2021). Factors determining seagrass blue carbon across bioregions and geomorphologies. Glob. Biogeochem. Cycles 35 (6), e2021GB006935. doi:10.1029/2021GB006935
McKenzie, L. J., Nordlund, L. M., Jones, B. L., Cullen-Unsworth, L. C., Roelfsema, C., and Unsworth, R. K. F. (2020). The global distribution of seagrass meadows. Environ. Res. Lett. 15 (7), 074041. doi:10.1088/1748-9326/ab7d06
McKenzie, L. J. (1994). Seasonal changes in biomass and shoot characteristics of a Zostera capricorni aschers. Dominant meadow in Cairns harbour, northern queensland. Mar. Freshw. Res. 45 (7), 1337–1352. doi:10.1071/mf9941337
McKenzie, L. J., Yaakub, S. M., Tan, R., Seymour, J., and Yoshida, R. L. (2016). Seagrass habitats of Singapore: Environmental drivers and key processes. Raffles Bull. Zoology 34, 60.
McMahon, K., van Dijk, K. J., Ruiz-Montoya, L., Kendrick, G. A., Krauss, S. L., Waycott, M., et al. (2014). The movement ecology of seagrasses. Proc. R. Soc. B Biol. Sci. 281 (1795), 20140878. doi:10.1098/rspb.2014.0878
Micheli, C., Spinosa, F., Aliani, S., Gasparini, G. P., Molcard, A., and Peirano, A. (2010). Genetic input by Posidonia oceanica (L.) delile fruits dispersed by currents in the ligurian sea. Plant Biosyst. 144 (2), 333–339. doi:10.1080/11263501003764798
Michot, T. C., Burch, J. N., Arrivillaga, A., Rafferty, S. P., Doyle, T. W., and Scott Kemmerer, R. (2002). Impacts of Hurricane mitch on seagrass beds and associated shallow reef communities along the caribbean coast of Honduras and Guatemala. Reston, Virginia: US Department of the Interior, US Geological Survey.
Monismith, S. G., Hirsh, H., Batista, N., Francis, H., Egan, G., and Dunbar, R. B. (2019). Flow and drag in a seagrass bed. J. Geophys. Res. Oceans 124 (3), 2153–2163. doi:10.1029/2018jc014862
Morris, E. P., Peralta, G., Brun, F. G., van Duren, L., Bouma, T. J., and Perez-Llorens, J. L. (2008). Interaction between hydrodynamics and seagrass canopy structure: Spatially explicit effects on ammonium uptake rates. Limnol. Oceanogr. 53 (4), 1531–1539. doi:10.4319/lo.2008.53.4.1531
Nadiarti, E. R., Djuwita, I., Budiharsono, S., Purbayanto, A., and Asmus, H. (2012). Challenging for seagrass management in Indonesia. J. Coast. Dev. 15 (3), 234–242.
Najamuddin, T. P., Sanusi, H. S., Nurjaya, I. W., Pembonan, R. E., Umasangaji, H., Mutmainnah, I. T., et al. (2021). Surface currents modeling based on tidal cycles and monsoon in tropical estuary, Indonesia. IOP Conf. Ser. Earth Environ. Sci. 890 (1), 012003. doi:10.1088/1755-1315/890/1/012003
Narulita, I., and Ningrum, W. (2018). Extreme flood event analysis in Indonesia based on rainfall intensity and recharge capacity. IOP Conf. Ser. Earth Environ. Sci. 118, 12045. doi:10.1088/1755-1315/118/1/012045
Nepf, H. M. (2012). Flow and transport in regions with aquatic vegetation. Annu. Rev. Fluid Mech. 44, 123–142. doi:10.1146/annurev-fluid-120710-101048
Novak, A. B., Pelletier, M. C., Colarusso, P., Simpson, J., Gutierrez, M. N., Arias-Ortiz, A., et al. (2020). Factors influencing carbon stocks and accumulation rates in eelgrass meadows across new england, United States. Estuaries Coasts 43 (8), 2076–2091. doi:10.1007/s12237-020-00754-9
Nugraha, A. H., Tasabaramo, I. A., Hernawan, U. E., Rahmawati, S., Dwirama Putra, R., and Darus, R. F. (2021). Diversity, coverage, distribution and ecosystem services of seagrass in three small islands of northern Papua, Indonesia: Liki island, meossu island and befondi island. Biodiversitas J. Biol. Divers. 22 (12). doi:10.13057/biodiv/d221238
O’Connor, J., and Revell, A. (2019). Dynamic interactions of multiple wall-mounted flexible flaps. J. Fluid Mech. 870, 189–216. doi:10.1017/jfm.2019.266
Olson, A. M., Hessing-Lewis, M., Dana, H., and Juanes, F. (2019). Nearshore seascape connectivity enhances seagrass meadow nursery function. Ecol. Appl. 29 (5), e01897. doi:10.1002/eap.1897
Ondiviela, B., Losada, I. J., Lara, J. L., Maza, M., Galván, C., Bouma, T. J., et al. (2014). The role of seagrasses in coastal protection in a changing climate. Coast. Eng. 87, 158–168. doi:10.1016/j.coastaleng.2013.11.005
Oprandi, A., Mucerino, L., De Leo, F., Bianchi, C. N., Morri, C., Azzola, A., et al. (2020). Effects of a severe storm on seagrass meadows. Sci. Total Environ. 748, 141373. doi:10.1016/j.scitotenv.2020.141373
Orth, R. J., Harwell, M. C., and Inglis, G. J. (2007). “Ecology of seagrass seeds and seagrass dispersal processes,” in Seagrasses: Biology, ecologyand conservation (Dordrecht: Springer), 111–133. doi:10.1007/978-1-4020-2983-7_5
Palmsten, M. L., and Splinter, K. D. (2016). Observations and simulations of wave runup during a laboratory dune erosion experiment. Coast. Eng. 115, 58–66. doi:10.1016/j.coastaleng.2016.01.007
Passarella, M., Ruju, A., De Muro, S., and Coco, G. (2020). Horizontal runup and seagrass beach cast-litters: Modelling and observations. J. Coast. Res. 95 (SI), 143–147. doi:10.2112/SI95-028.1
Paul, M., and Amos, C. L. (2011). Spatial and seasonal variation in wave attenuation over Zostera noltii. J. Geophys. Res. Oceans 116 (C8), C08019. doi:10.1029/2010jc006797
Paul, M. (2018). The protection of sandy shores – can we afford to ignore the contribution of seagrass? Mar. Pollut. Bull. 134, 152–159. doi:10.1016/j.marpolbul.2017.08.012
Péquignet, A. C. N., Becker, J. M., and Merrifield, M. A. (2014). Energy transfer between wind waves and low-frequency oscillations on a fringing reef, I Pan, G uam. J. Geophys. Res. Oceans 119 (10), 6709–6724. doi:10.1002/2014jc010179
Perales, D., and Herman, R. B. (2020). Water pressure variations under the arctic sea ice. AGU Fall Meet. Abstr. 2020, C045–C0001.
Pereda-Briones, L., Infantes, E., Orfila, A., Tomas, F., and Terrados, J. (2018). Dispersal of seagrass propagules: Interaction between hydrodynamics and substratum type. Mar. Ecol. Prog. Ser. 593, 47–59. doi:10.3354/meps12518
Pinsky, M. L., Guannel, G., and Arkema, K. K. (2013). Quantifying wave attenuation to inform coastal habitat conservation. Ecosphere 4 (8), art95–16. doi:10.1890/es13-00080.1
Potouroglou, M., Bull, J. C., Krauss, K. W., Kennedy, H. A., Fusi, M., Daffonchio, D., et al. (2017). Measuring the role of seagrasses in regulating sediment surface elevation. Sci. Rep. 7 (1), 11917. doi:10.1038/s41598-017-12354-y
Pranesh, M. R., and Mani, J. S. (1988). Similitude engineering—ocean structure interaction. Ocean. Eng. 15 (2), 189–200. doi:10.1016/0029-8018(88)90028-5
Presley, R., and Caffrey, J. M. (2021). Nitrogen fixation in subtropical seagrass sediments: Seasonal patterns in activity in santa rosa sound, Florida, United States. J. Mar. Sci. Eng. 9 (7), 766. doi:10.3390/jmse9070766
Purwanto, D. N. S., Zainuri, M., Permatasari, G., Atmodjo, W., Rochaddi, B., Ismanto, A., et al. (2021). Seasonal variability of waves within the Indonesian seas and its relation with the monsoon wind. Indonesian J. Mar. Sciences/Ilmu Kelautan 26 (3), 189–196. doi:10.14710/ik.ijms.26.3.189-196
Qian, J. H., Robertson, A. W., and Vincent, M. (2010). Interactions among ENSO, the monsoon, and diurnal cycle in rainfall variability over Java, Indonesia. J. Atmos. Sci. 67 (11), 3509–3524. doi:10.1175/2010jas3348.1
Rachmayani, R., Ningsih, N. S., Ramadhan, H., and Nurfitri, S. (2018). Analysis of ocean wave characteristic in western Indonesian seas using wave spectrum model. MATEC Web Conf. 147, 5001. doi:10.1051/matecconf/201814705001
Rahayu, Y. P., Solihuddin, T., Kusumaningtyas, M. A., Salim, H. L., Rixen, T., Hutahaean, A. A., et al. (2019). The sources of organic matter in seagrass sediments and their contribution to carbon stocks in the Spermonde islands, Indonesia. Aquat. Geochem. 25 (3), 161–178. doi:10.1007/s10498-019-09358-7
Reidenbach, M. A., and Thomas, E. L. (2018). Influence of the seagrass, Zostera marina, on wave attenuation and bed shear stress within a shallow coastal bay. Front. Mar. Sci. 397. doi:10.3389/fmars.2018.00397
Ricart, A. M., York, P. H., Bryant, C. V., Rasheed, M. A., Ierodiaconou, D., and Macreadie, P. I. (2020). High variability of blue carbon storage in seagrass meadows at the estuary scale. Sci. Rep. 10 (1), 5865. doi:10.1038/s41598-020-62639-y
Rifai, H., Hernawan, U. E., Zulpikar, F., Sondakh, C. F. A., Ambo-Rappe, R., Sjafrie, N. D. M., et al. (2022a). Strategies to improve management of Indonesia’s blue carbon seagrass habitats in marine protected areas. Coast. Manag. 50 (2), 93–105. doi:10.1080/08920753.2022.2022948
Rifai, H., Quevedo, J. M. D., Lukman, K. M., Sondak, C. F. A., Risandi, J., Hernawan, U. E., et al. (2022b). Potential of seagrass habitat restorations as nature-based solutions: Practical and scientific implications in Indonesia. Ambio 52, 546–555. doi:10.1007/s13280-022-01811-2
Risandi, J., Hansen, J. E., Lowe, R. J., and Rijnsdorp, D. P. (2020). Shoreline variability at a reef-fringed pocket beach. Front. Mar. Sci. 7, 445. doi:10.3389/fmars.2020.00445
Röhr, M. E., Boström, C., Canal-Vergés, P., and Holmer, M. (2016). Blue carbon stocks in Baltic Sea eelgrass (Zostera marina) meadows. Biogeosciences 13 (22), 6139–6153. doi:10.5194/bg-13-6139-2016
Ruiz-Montoya, L., Lowe, R. J., and Kendrick, G. A. (2015). Contemporary connectivity is sustained by wind- and current-driven seed dispersal among seagrass meadows. Mov. Ecol. 3 (1), 9. doi:10.1186/s40462-015-0034-9
Saenko, O. A., and Merryfield, W. J. (2005). On the effect of topographically enhanced mixing on the global ocean circulation. J. Phys. Oceanogr. 35 (5), 826–834. doi:10.1175/jpo2722.1
Saunders, M. I., Leon, J., Phinn, S. R., Callaghan, D. P., O’Brien, K. R., Roelfsema, C. M., et al. (2013). Coastal retreat and improved water quality mitigate losses of seagrass from Sea Level rise. Glob. Change Biol. 19 (8), 2569–2583. doi:10.1111/gcb.12218
Saunders, M. I., Scott, A., Klein, C. J., Weber, T., and Possingham, H. P. (2017). Increased sediment loads cause non-linear decreases in seagrass suitable habitat extent. PloS One 12 (11), e0187284. doi:10.1371/journal.pone.0187284
Schaefer, R. B., and Nepf, H. M. (2020). Investigating wave damping and hydrodynamics in seagrass meadows under combined wave-current conditions. AGU Fall Meet. Abstr. 2020, EP052–0014.
Serrano, O., Lavery, P. S., James, B., and Duarte, C. M. (2020). Impact of seagrass establishment, industrialization and coastal infrastructure on seagrass biogeochemical sinks. Mar. Environ. Res. 160, 104990. doi:10.1016/j.marenvres.2020.104990
Sheremet, A., Guza, R. T., Elgar, S., and Herbers, T. H. C. (2002). Observations of nearshore infragravity waves: Seaward and shoreward propagating components. J. Geophys. Res. Oceans 107 (C8), 3095. doi:10.1029/2001jc000970
Short, F., Carruthers, T., Dennison, W., and Waycott, M. (2007). Global seagrass distribution and diversity: A bioregional model. J. Exp. Mar. Biol. Ecol. 350 (1), 3–20. doi:10.1016/j.jembe.2007.06.012
Short, F. T., and Neckles, H. A. (1999). The effects of global climate change on seagrasses. Aquat. Bot. 63 (3–4), 169–196. doi:10.1016/s0304-3770(98)00117-x
Short, F. T., Polidoro, B., Livingstone, S. R., Carpenter, K. E., Bandeira, S., Bujang, J. S., et al. (2011). Extinction risk assessment of the world’s seagrass species. Biol. Conserv. 144 (7), 1961–1971. doi:10.1016/j.biocon.2011.04.010
Sjafrie, N. D. M., Hernawan, U. E., Prayuda, B., Supriyadi, I. H., Iswari, M. Y., Rahmat, K. A., et al. (2018). Status padang lamun Indonesia. Jakarta, Indonesia: Puslit Oseanografi - LIPI.
Stratigaki, V., Manca, E., Prinos, P., Losada, I. J., Lara, J. L., Sclavo, M., et al. (2011). Large-scale experiments on wave propagation over Posidonia oceanica. J. Hydraulic Res. 49 (1), 31–43. doi:10.1080/00221686.2011.583388
Suanez, S., Cancouet, R., Floc'h, F., Ardhuin, F., Filipot, J. F., Cariolet, J. M., et al. (2015). Observations and predictions of wave runup, extreme water levels, and medium-term dune erosion during storm conditions. J. Mar. Sci. Eng. 3 (3), 674–698. doi:10.3390/jmse3030674
Swapna, P., Jyoti, J., Krishnan, R., Sandeep, N., and Griffies, S. M. (2017). Multidecadal weakening of Indian summer monsoon circulation induces an increasing northern Indian ocean Sea Level. Geophys. Res. Lett. 44 (20), 10,560–10,572. doi:10.1002/2017GL074706
Symonds, G., Huntley, D. A., and Bowen, A. J. (1982). Two-dimensional surf beat: Long wave generation by a time-varying breakpoint. J. Geophys. Res. Oceans 87 (C1), 492–498. doi:10.1029/jc087ic01p00492
Taphorn, M., Villanueva, R., Paul, M., Visscher, J., and Schlurmann, T. (2021). Flow field and wake structure characteristics imposed by single seagrass blade surrogates. J. Ecohydraulics 7, 58–70. doi:10.1080/24705357.2021.1938253
Tarya, A., Hoitink, A. J. F., van der Vegt, M., van Katwijk, M. M., Hoeksema, B. W., Bouma, T. J., et al. (2018). Exposure of coastal ecosystems to river plume spreading across a near-equatorial continental shelf. Cont. Shelf Res. 153, 1–15. doi:10.1016/j.csr.2017.12.003
Thomsen, E., Herbeck, L. S., and Jennerjahn, T. C. (2020). The end of resilience: Surpassed nitrogen thresholds in coastal waters led to severe seagrass loss after decades of exposure to aquaculture effluents. Mar. Environ. Res. 160, 104986. doi:10.1016/j.marenvres.2020.104986
Thomson, J. A., Burkholder, D. A., Heithaus, M. R., Fourqurean, J. W., Fraser, M. W., Statton, J., et al. (2015). Extreme temperatures, foundation species, and abrupt ecosystem change: An example from an iconic seagrass ecosystem. Glob. Change Biol. 21 (4), 1463–1474. doi:10.1111/gcb.12694
Timko, P. G., Arbic, B. K., Richman, J. G., Scott, R. B., Joseph Metzger, E., and Wallcraft, A. J. (2013). Skill testing a three-dimensional global tide model to historical current meter records. J. Geophys. Res. Oceans 118 (12), 6914–6933. doi:10.1002/2013jc009071
Toimil, A., Camus, P., Losada, I. J., Cozannet, G. L., Nicholls, R. J., Idier, D., et al. (2020). Climate change-driven coastal erosion modelling in temperate sandy beaches: Methods and uncertainty treatment. Earth-Science Rev. 202, 103110. doi:10.1016/j.earscirev.2020.103110
Touchette, B. W. (2007). Seagrass-salinity interactions: Physiological mechanisms used by submersed marine angiosperms for a life at sea. J. Exp. Mar. Biol. Ecol. 350 (1), 194–215. doi:10.1016/j.jembe.2007.05.037
Tschisgale, S., Loehrer, B., Meller, R., and Froehlich, J. (2021). Large eddy simulation of the fluid-structure interaction in an abstracted aquatic canopy consisting of flexible blades. J. Fluid Mech. 916, A43. doi:10.1017/jfm.2020.858
Twomey, A. J., O’Brien, K. R., Callaghan, D. P., Saunders, M. I., and Saunders, M. I. (2020). Synthesising wave attenuation for seagrass: Drag coefficient as a unifying indicator. Mar. Pollut. Bull. 160, 111661. doi:10.1016/j.marpolbul.2020.111661
Unsworth, R. K. F., Ambo-Rappe, R., Benjamin, L., Jones, Y. A. L. N., Irawan, A., Hernawan, U. E., et al. (2018). Indonesia’s globally significant seagrass meadows are under widespread threat. Sci. Total Environ. 634, 279–286. doi:10.1016/j.scitotenv.2018.03.315
Unsworth, R. K. F., and Cullen, L. C. (2010). Recognising the necessity for indo-pacific seagrass conservation. Conserv. Lett. 3 (2), 63–73. doi:10.1111/j.1755-263X.2010.00101.x
van Dijk, J. K., van Tussenbroek, B. I., Jiménez-Durán, K., Judith, G., Márquez-Guzmán, O. J., and Ouborg, J. (2009). High levels of gene flow and low population genetic structure related to high dispersal potential of a tropical marine angiosperm. Mar. Ecol. Prog. Ser. 390, 67–77. doi:10.3354/meps08190
van Rooijen, A. A., Lowe, R., Ghisalberti, M., Conde-Frias, M., Tan, L., and Tan, L. (2018). Predicting current-induced drag in emergent and submerged aquatic vegetation canopies. Front. Mar. Sci. 449. doi:10.3389/fmars.2018.00449
van Rooijen, A. A., McCall, R. T., Van Thiel de Vries, J. S. M., Van Dongeren, A. R., Reniers, A. J. H. M., and Roelvink, J. A. (2016b). Modeling the effect of wave-vegetation interaction on wave setup. J. Geophys. Res. Oceans 121 (6), 4341–4359. doi:10.1002/2015jc011392
van Rooijen, A. A., van Dongeren, A., Roelvink, D., McCall, R., Reniers, A., and van Thiel de Vries, J. (2016a). “The effect of vegetation on sea-swell waves, infragravity waves and wave-induced set-up,” in 2016 Ocean Sciences Meeting. AGU.
van Rooijen, A. A., van Thiel de Vries, J. S. M., McCall, R. T., Van Dongeren, A. R., Roelvink, J. A., and Reniers, A. J. H. M. (2015). “Modeling of wave attenuation by vegetation with XBeach,” in E-Proceedings of the 36th IAHR World Congress, The Hague, the Netherlands, 28 June-3 July 2015. Citeseer.
Villanueva, R., Thom, M., Visscher, J., Paul, M., and Schlurmann, T. (2021). Wake length of an artificial seagrass meadow: A study of shelter and its feasibility for restoration. J. Ecohydraulics 7, 77–91. doi:10.1080/24705357.2021.1938256
Watanabe, Y., Tsuda, Y., and Saruwatari, A. (2020). Wave packet focusing in shallow water. Coast. Eng. J. 62 (2), 336–349. doi:10.1080/21664250.2020.1756033
Waycott, M., Collier, C., McMahon, K., Ralph, P., McKenzie, L., James, U., et al. (2007). Vulnerability of seagrasses in the great barrier reef to climate change. Townsville, QLD: Great Barrier Reef Marine Park Authority and Australian Greenhouse Office.
Weitzman, J. S., Zeller, R. B., Thomas, F. I. M., and Koseff, J. R. (2015). The attenuation of current-and wave-driven flow within submerged multispecific vegetative canopies. Limnol. Oceanogr. 60 (6), 1855–1874. doi:10.1002/lno.10121
Whitehead, J. A. (2018). Transitions and oscillations in a thermohaline deep ocean circulation model. Geophys. Astrophysical Fluid Dyn. 112 (5), 321–344. doi:10.1080/03091929.2018.1530351
Wirasatriya, A., Dwi Susanto, R., Kunarso, K., Jalil, A. R., Ramdani, F., and Puryajati, A. D. (2021). Northwest monsoon upwelling within the Indonesian seas. Int. J. Remote Sens. 42 (14), 5433–5454. doi:10.1080/01431161.2021.1918790
Wu, K., Chen, C. N. N., and Keryea, S. (2016). Long distance dispersal potential of two seagrasses Thalassia hemprichii and Halophila ovalis. PloS One 11 (6), e0156585. doi:10.1371/journal.pone.0156585
Yang, D., and Huang, D. (2011). Impacts of typhoons tianying and dawei on seagrass distribution in xincun bay, hainan province, China. Acta Oceanol. Sin. 30 (1), 32–39. doi:10.1007/s13131-011-0088-5
Yin, K., Xu, S., Huang, W., Liu, S., and Li, M. (2021). Numerical investigation of wave attenuation by coupled flexible vegetation dynamic model and XBeach wave model. Ocean. Eng. 235, 109357. doi:10.1016/j.oceaneng.2021.109357
Zeller, R. B., Weitzman, J. S., Abbett, M. E., Zarama, F. J., Fringer, O. B., and Koseff, J. R. (2014). Improved parameterization of seagrass blade dynamics and wave attenuation based on numerical and laboratory experiments. Limnol. Oceanogr. 59 (1), 251–266. doi:10.4319/lo.2014.59.1.0251
Zenone, A., Badalamenti, F., Alagna, A., Gorb, S. N., and Infantes, E. (2022). Assessing tolerance to the hydrodynamic exposure of Posidonia oceanica seedlings anchored to rocky substrates. Front. Mar. Sci. 8, 788448. doi:10.3389/fmars.2021.788448
Zhang, H., and Geng, B. (2015). Introduction of the world largest wave flume constructed by TIWTE. Procedia Eng. 116, 905–911. doi:10.1016/j.proeng.2015.08.380
Zhao, R., Zhu, X. H., and Guo, X. (2017). The impact of monsoon winds and mesoscale eddies on thermohaline structures and circulation patterns in the northern South China sea. Cont. Shelf Res. 143, 240–256. doi:10.1016/j.csr.2016.06.009
Zhao, X., Yan, Z., and Zhang, X. P. (2016). A wind-wave farm system with self-energy storage and smoothed power output. IEEE Access 4, 8634–8642. doi:10.1109/access.2016.2631505
Zhu, Q., Wiberg, P. L., and Reidenbach, M. A. (2021). Quantifying seasonal seagrass effects on flow and sediment dynamics in a back-barrier bay. J. Geophys. Res. Oceans 126 (2), e2020JC016547. doi:10.1029/2020JC016547
Keywords: seagrass, waves, currents, shoreline, ecology, nearshore, asian monsoon, Indonesia
Citation: Risandi J, Rifai H, Lukman KM, Sondak CFA, Hernawan UE, Quevedo JMD, Hidayat R, Ambo-Rappe R, Lanuru M, McKenzie L, Kohsaka R and Nadaoka K (2023) Hydrodynamics across seagrass meadows and its impacts on Indonesian coastal ecosystems: A review. Front. Earth Sci. 11:1034827. doi: 10.3389/feart.2023.1034827
Received: 02 September 2022; Accepted: 30 January 2023;
Published: 09 February 2023.
Edited by:
Alexandra V. Turchyn, University of Cambridge, United KingdomReviewed by:
Thangaradjou T., Science and Engineering Research Board, IndiaAmrit Kumar Mishra, The University of Hong Kong, Hong Kong SAR, China
Hacen Mohamed El-Hacen, University of Groningen, Netherlands
Copyright © 2023 Risandi, Rifai, Lukman, Sondak, Hernawan, Quevedo, Hidayat, Ambo-Rappe, Lanuru, McKenzie, Kohsaka and Nadaoka. This is an open-access article distributed under the terms of the Creative Commons Attribution License (CC BY). The use, distribution or reproduction in other forums is permitted, provided the original author(s) and the copyright owner(s) are credited and that the original publication in this journal is cited, in accordance with accepted academic practice. No use, distribution or reproduction is permitted which does not comply with these terms.
*Correspondence: Johan Risandi, am9oYW4ucmlzYW5kaUBicmluLmdvLmlk