- School of Earth Sciences and Engineering, Xi’an Shiyou University, Xi’an, China
As the last area with unknown oil exploration potential, Zealandia has attracted the attention of petroleum scientists. Under the constraint of the known rift distribution, the gravity and magnetic anomaly data are utilized to predict the distribution of rift basins, and the balanced cross-section technique and basin simulation technique are utilized to analyze the development of the rift basins and hydrocarbon generation history of source rocks for the hydrate prospect prediction of the rift basins of northwest Zealandia. The time of rifting in Zealandia varies greatly from place to place, and it has experienced extensive rifts, migrated to the east of the Lord Howe Ridge, migrated to the west of the Lord Howe Ridge, migrated to the west of the Dampier Ridge, and finally migrated to both sides of the Lord Howe Ridge and then experienced multiple stages of compression transformation and deep burial transformation. The Upper Cretaceous coal source rock in the rift Ⅱ stage has entered the wet gas generation stage in the deep part of the rift or deeper rift on the eastern side of the Lord Howe Ridge, and the coal-generated wet gas may migrate along the fault and form a gas hydrate in the shallow seabed stable zone.
1 Introduction
Zealandia is a hidden landmass in the southwestern Pacific Ocean, which is 94% submerged beneath the oceanic waters (Mortimer et al., 2016), as a result of widespread Late Cretaceous breakup, crustal thinning, and consequent isostatic balance. With the deepening of geological and geophysical investigation, especially with the discovery of the granites and metamorphic rocks in the pre-Cretaceous basement (Suggate et al., 1978; Beggs et al., 1990; Tulloch et al., 1991, 2009; Gamble et al., 1993; McDougall et al., 1994; Mortimer et al., 1997, 1998, 2006, 2008a, 2008b, 2014, 2015) and the discovery of synchronal volcanic records from the Late Cretaceous (Tulloch et al., 2009; Mortimer et al., 2014), a great deal of knowledge on the distribution, structure, and cracking process of the continental block has been obtained for the in-depth discussions (Corredorfo, 1996; Ringis, 1972; Shaw, 1979; Stock and Molnar, 1982; Luyendyk, 1995; Lafoy et al., 2005; Schellart et al., 2006; Mortimer et al., 2006). Zealandia is considered to be a consistent continent with an area of 4.9 Mkm2 (Mortimer et al., 2016), consisting of a series of grabens and horsts (Gaina et al., 1998). Also, the continental block is considered to be a product of the breakup of the continent Gondwana in the Late Cretaceous. Following the breakup of the continent Gondwana, the continental block experienced extensive rifting and plastic thinning and then sank under the ocean with the influence of gravity isostasy.
There is abundant evidence for gas hydrates in the Fairway Basin and Taranaki Basin, such as diapir structures and BSRs (Claypool and Kaplan, 1974; Kvenvolden, 1995; Exon et al., 1998; Collot et al., 2009; Kroeger et al., 2017). Owning to the limitation to data, a deep understanding of the distribution, the extensional process, the law of the later reformation, and the prospect of the exploration of the hydrate is still lacking. In recent years, with the development of seismic exploration and ocean drilling, it has shown that it has an important potential for gas hydrate exploration in view of the Upper Cretaceous coal-measure source rocks from drillings (Van de Beuque et al., 2003; Kroeger et al., 2017), especially the findings of BSR (bottom simulating reflection) and diapir and crater in the Fairway (Exon et al., 1998) and Taranaki basins (Auzende et al., 2000; Pierrick et al., 2015; Kroeger et al., 2017).
As the last area with unknown oil exploration potential, it has attracted the attention of energy scientists. In view of the fact that hydrocarbon gases can form hydrates in the shallow seabed, they migrated from the mature hydrocarbon generation of coal-measure source rocks. So this article is under the constraint of the known rift distribution, using gravity and magnetic anomaly data to predict the distribution of rift basins and combining with the results of seismic interpretation, the balanced cross-section technique, and basin simulation technique to analyze the development law of the lacunae and hydrocarbon generation history of source rocks for the hydrate prospect prediction.
2 Regional geological background
Zealandia is located between the Australian Plate in the west and the Pacific Plate in the east. Specifically, it is located in the sea area between the trench of New Britain–San Cristobal–New Hebrides and the Tasman Sea–Coral Sea on the eastern edge of Australia (Figure 1).
Bathymetric map of the southwestern Pacific region showing data used for this study. Countries (AUS: Australia, NZ: New Zealand, and NC: New Caledonia); rises (LHR: Lord Howe Rise, DR: Dampier Ridge, WNR: West Norfolk Ridge, NR: Norfolk Ridge, RR: Reinga Ridge, STR: South Tasman Ridge, and NHR, New Hebrides Ridge); seas (CS: Coral Sea and SS: Solomon Sea); back-arc basins (TB: Taranaki Basin, RB: Reinga Basin, NCB: New Caledonia Basin, NLB: North Loyalty Basin, FAB: Faust Basin, FB: Fairway Basin, CB: Capel Basin, MB: Middleton Basin, GB: Gower Basin, MOB: Moore Basin, MNB: Monowai Basin, and LB: Lord Howe Basin); troughs (PT: Pocklington Trough, NBT: New Britain Trough, and NHT: New Hebrides Trench). A-A′ and B-B′ are seismic profile positions (the same as follows).
There are a number of basins, basin-bounding plateaus, and elongated ridges in Zealandia, which were mainly rifted from the eastern margin of the Gondwana continent in the Late Cretaceous. The ridges and plateaus are underlain by extended continental crust (e.g., Dampier Ridge, Lord Howe Rise, Challenger Plateau, Norfolk Ridge, Campbell Plateau, and Chatham Rise). Most of the basins (Fairway Basin, Capel Basin, and Reinga Basin) are mainly located between the Lord Howe Rise and the Norfolk Ridge or on the east and west sides, which are mainly covered by mid to Late Cretaceous rift valley-related sediments and volcanic and Cenozoic pelagic sediments (Figure 2).
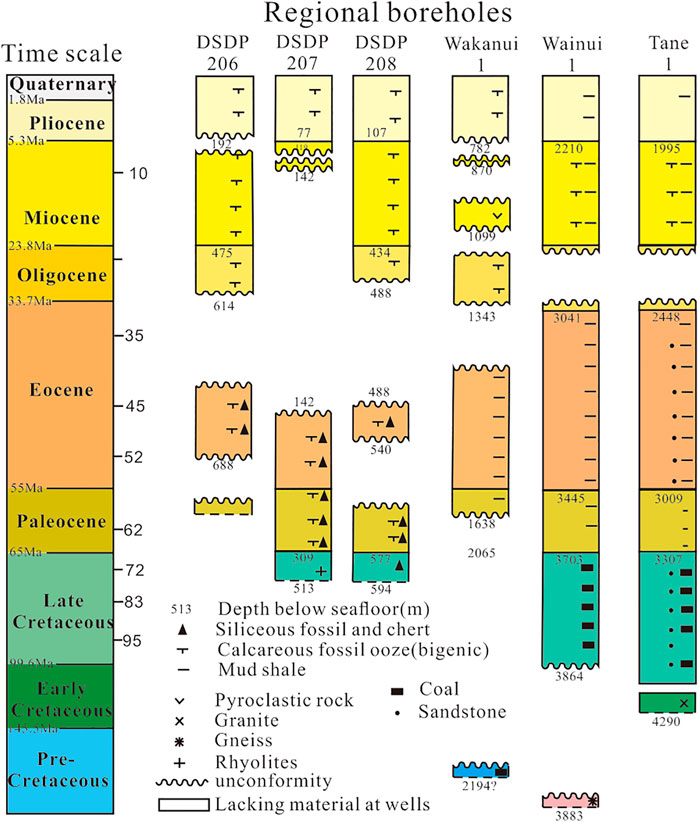
FIGURE 2. Comprehensive histogram of formation drilling (Willcox and Sayers, 2002; Stagg et al., 2002; Van de Beuque et al., 2003; Collot et al., 2009).
1) Initial continental rift stage (∼120–95 Ma)
This Zealandia extension and the formation of its internal rift basin groups were mainly followed by episodic opening from early to middle Cretaceous to present, as a direct consequence of hinge-retreat of the subducting Pacific Plate and collapse of the overriding Zealandia toward the retreating hinge of the Pacific Plate. It can be roughly divided into the following five stages.
From the early to middle Cretaceous, there is a strong, widespread east-west to northeast-southwest-directed extension in the eastern margin of Gondwana, resulting from east to northeast-directed rollback of west to southwest-dipping subduction of the Pacific Plate. A set of pyroclastic rocks deposited in these rift basins during this period overlain the pre-Cretaceous metamorphic or granite basement (Van de Beuque et al., 2003). In the Late Cretaceous Cenomanian (∼95 Ma), the overriding Zealandia extension ceased in relative plate motion from the collision between Zealandia and the Pacific Plate (Veevers, 2000a, b).
2) Pulsatile continental breakup and drift stage (95–52 Ma)
From the Late Cretaceous (∼95Ma) to Early Eocene (∼52Ma), the eastern margin of Gondwana continues to widespread rifting, primarily due to the west-directed subduction and east-directed rollback of the Pacific Plate (up to at least 1,200 km) (Schellart et al., 2006), accommodating opening of the New Caledonia, South Loyalty, Coral Sea, and Pocklington back-arc basins and partly accommodating spreading in the Tasman Sea. From 73 Ma to 68 Ma, the spreading rifting occurred between the Lord Howe Ridge and Dampier Ridge in the South Tasman Sea. Subsequently, the spreading rifting ridge then jumped to the west of the Dampier Ridge and continued to expand northward (Ringis, 1972), leading to the formation of the Coral Sea from 62 Ma to 52 Ma in the northern Tasman Sea (Gaina et al., 1999).
3) Pulsatile compressional tectonic stage (52–22.3 Ma)
In the Eocene, the convergent motion between the Pacific Plate and Zealandia increased gradually due to the northward migration of Australia and Zealandia. This convergence process resulted in the cessation of the expansion of the Coral Sea (∼52Ma) (Gaina et al., 1999), Tasman Sea (∼52 Ma) (Gaina et al., 1998), and the south Loyalty–Santa Cruz–Pocklington Basin (∼55Ma) (Schellart et al., 2006) and also caused the oceanic crust of the back-arc basin of the new back-arc basin subducting toward the New Caledonia island arc. Subsequently, New Caledonia–Pocklington subduction zone was formed in Paleocene to earliest Eocene (∼50–45 Ma) (Cluzel et al., 2001).
This convergence shortening event was represented by the formation of the high-pressure/low-temperature (HP/LT) rocks from the Pouebo metamorphic terrane in northern New Caledonia, the original rock of which was formed in ∼85–55 Ma (Spandler et al., 2005) and for which peak high-pressure metamorphism has been dated at ∼44Ma (Spandler et al., 2005). This subduction and convergence process continued until the earliest Miocene and eventually led to the obduction of ophiolite remnants and terranes in the South Loyalty back-arc basin along the newly formed New Caledonia–Pocklington subduction zone, which was formed in the Eocene to Early Miocene (∼38–33.7 Ma) (Cluzel et al., 2001).
This subduction–convergence event also has an obvious response in the sedimentary record, which caused the two angular unconformity contacts with the underlying Paleocene and between the Eocene and Oligocene. These angular unconformity contact relationships are revealed in drilling DSDP 206, 207, and 208 (Van de Beuque et al., 2003). With the weakening of subduction-compression, the rift basins entered the stage of stress relaxation depression and deposited a set of nannofossil ooze, chalk, and volcanic ash in Oligocene.
4) Shear extrusion tectonic stage (23.3–5 Ma)
Since 23 Ma, with the Southeast Indian Ocean Ridge extending eastward, the Resolution Ridge began to form due to seafloor expansion and mantle uplift, which led to the Alpine rupture of the North and South Islands of New Zealand. With the striking southwestward extension of the Campbell Plateau relative to the Challenger Plateau in New Zealand, the dextral lithospheric Alpine fault zone was gradually formed by left-lateral strike-slip (Kamp, 1986), which led to the multi-stage compression and denudation reformation in south Zealandia (Figure 2).
With the rapid southeastward expansion movement of the Southeast Indian Ocean Ridge, which merged with the Southwest Pacific Ridge, Zealandia was completely separated from the surrounding Antarctic at 10 Ma.
5) Thermogravimetric equilibrium subsidence stage (5–0 Ma)
From the latest Miocene or Pliocene to the present, especially owning to subducting westward and retreating eastward of the Pacific Plate (up to ∼400 km of rollback distance) (Schellart et al., 2006), Zealandia was strongly thermal and extensionally subsided and concealed under the sea, covered by thick deep-sea ooze deposits, accompanying with the extension of the Lau back-arc Basin.
3 Methods
3.1 Rift distribution identification method and process
3.1.1 Principle of rift identification and data source
The sedimentary strata in rift basins are characterized by large thickness variations, lateral discontinuity, and local distribution. Therefore, the gravity anomalies often caused by them should be local low-gravity anomalies. Since most of the rifted sedimentary layers in rift basins are non-magnetic or weakly magnetic, their magnetic anomalies should also be local low-magnetic anomalies. However, given that the magnetism of the metamorphic basement of the Zealandia plate may vary greatly when using the local anomalies of gravity and magnetism to identify the distribution of rifted layers in this area, gravity is the main force, and magnetism is the supplement.
Gravity satellite altimetry gravity anomaly data come from the latest satellite gravity data covering the world provided by the University of California, San Diego, and the Scripps Oceanographic Institute, version 23.1. Data sources and descriptions are available at http://topex.ucsd.edu/cgi-bin/get_data.cgi. The gravity potential field was processed in m or km. The magnetic data come from EMAG2 (the Earth Magnetic Anomaly Grid), which is the result of data splicing of different observation forms (aerial magnetic survey and marine ship survey) in different regions. The network degree of air magnetic survey and marine ship survey is different, and the average grid degree of data is 2′×2'. See https://www.ngdc.noaa.gov/docucomp/page?xml = NOAA/NESDIS/NGDC/MGG/Geophysical_Models/iso/xml/EMAG2.xml &view=getDataView&header=none for data source and description.
Modified from (Bache et al., 2013), the section location is shown in Figure 1.
3.1.2 Method and process of rift distribution identification
By comparing the locations of the rift basin in both the plane and the profile with those identified by the seismic profile, the local gravity and magnetic anomalies would be determined for the purpose of identifying the distribution of rift basins. The identification method and process of the Northwest Zealandia rift basin are as follows.
First, the extraction of the gravity anomaly of the sedimentary layer and the calculation of the pole magnetic anomaly were performed. The Bouguer gravity anomaly was calculated by subtracting the seawater gravity anomaly from the free space gravity anomaly, which is obtained by forward modeling from seafloor topography data. The density of seawater is 1.03 × 103 kg/m3, and the average density of the rift sedimentary layer is 2.30 × 103 kg/m3. The gravity anomaly of the sedimentary layer was obtained by subtracting the Moho gravity anomaly from the Bouguer gravity anomaly, and the fluctuation of the Moho was forwardly calculated by the Moho depth data in CRUST1.0, with the density difference between the crust and mantle being 0.5 × 103 kg/m3. Affected by the oblique magnetization, the position of the geological body does not correspond to that of the magnetic force ΔT anomaly. Therefore, the pole magnetic anomaly was calculated by the method of changing the magnetic dip angle. According to the comprehensive histogram of regional formation drilling, the stratigraphic unit age in the seismic profile (Figure 3 and Bache et al., 2013) is revised. Unit 1a: Oligocene to Pleistocene deposits (?); unit 2a: Paleocene to Eocene sedimentary rocks (?); unit 3: Late Cretaceous Rift seismic unit (inference from rift sequence characteristics); RU1 and RU2: regional unconformities.
Second, the extraction of rift weight and magnetic anomalies was performed. In order to determine the range of local gravity and magnetic anomalies, the vertical first-order derivative of the gravity anomaly and the vertical first-order derivative of the polarized magnetic force anomaly are obtained at different extension heights through, respectively, calculating the vertical first-order derivatives of the sedimentary layer gravity anomaly and the pole magnetic anomaly by the upward continuation method to suppress the high-frequency interference. By comparing the different calculation results with the positions of the rifts identified by the seismic data (Figure 3), the vertical first-order derivative of gravity and magnetic anomalies is determined, which is most consistent with the seismic rift distribution results (Figure 4).
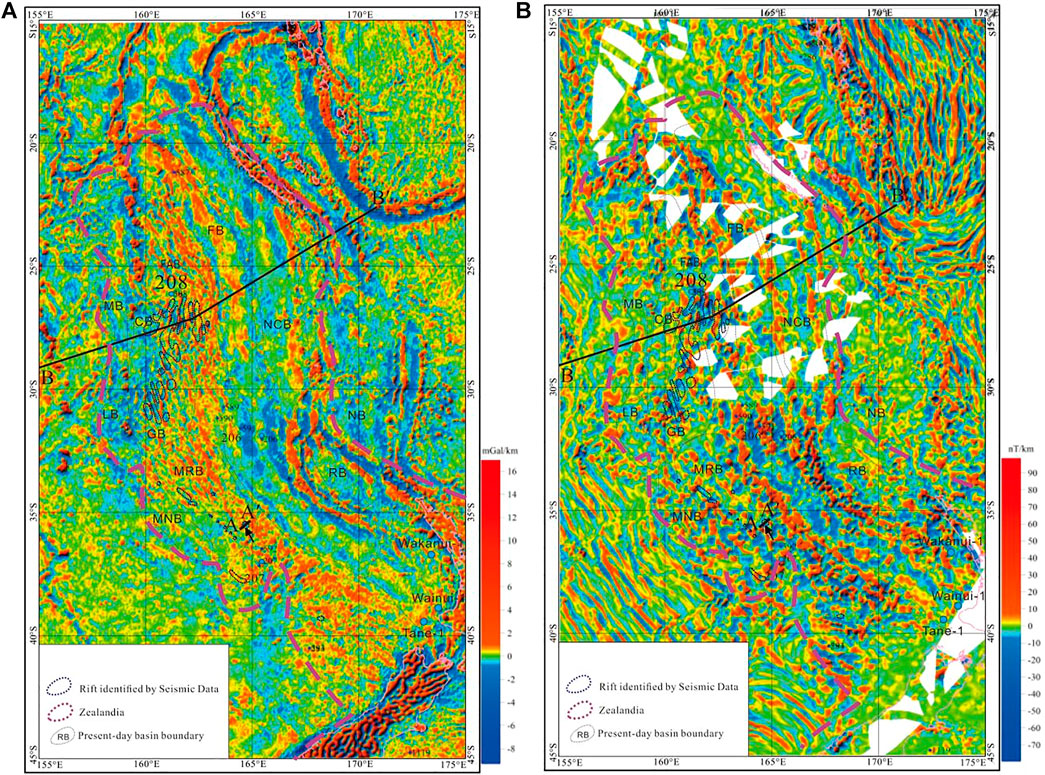
FIGURE 4. First-order vertical derivative of (A) gravity and (B) magnetic anomaly reduced to the pole. The solid black line pointed by the white arrow locates the position of the seismic section in Figure 3. (A) First-order vertical derivative of gravity anomaly. (B). First-order vertical derivative of magnetic anomaly reduced to the pole. The white area is the data blank area. Abbreviation of basin name (see Figure 1).
Finally, the identification of the distribution of the rift sedimentary layer. Considering the best vertical first-order derivative determined in the second step as the basic data, combined with the seismic profiles collected in the study area as constraints, the plane distribution of the rift layer in the study area was comprehensively identified (Figure 5), mainly by utilizing the negative region of the vertical first-order derivative of the gravity anomaly and supplementally utilizing the negative region of the vertical first-order derivative of polarized magnetic force anomaly.
The green shaded area represents the rift location identified by gravity and magnetic anomalies; the blue shaded area is the rift location identified by Bache et al. (2013) using seismic profiles. The detailed name of the basin is shown in Figure 1.
3.2 Time-depth conversion and selection of boundary conditions for thermal simulation
For modeling hydrocarbon generation history, conversion of data (including reflection seismic profiles) from time domain (two-way travel time, TWT) to depth domain (meters) was carried out by 3D-Move software. The model used in this study was based on a regional model using velocity data from the speed of velocity layers (Kroeger et al., 2017). PetroMod modeling is a well-established method to forward model the evolution of sedimentary basins by restoring the original basin conditions and adding sediment through time (Naeth et al., 2005; Beha et al., 2008; Hantschel and Kauerauf, 2009; Ondrak et al., 2009). Forward modeling is often used to reconstruct the thermal evolution of the hydrocarbon generation history of source rocks (Kroeger et al., 2017). The modern average heat flow value on the source rocks’ thermal evolution modeling is derived from Foucher and Scient (2006) and Kroeger et al. (2017), which is 55 mW/m2. The heat flow values of paleo-geological historical periods are comprehensively determined according to the dynamic background of the basin (Allen and Allen, 1990). The parameters of formation lithology are calculated according to the drilling data, and paleo-depth parameters are obtained from sedimentary facies analysis.
4 Results and discussion
4.1 Formation, evolution, and transformation of rift basins
The rifting time in Zealandia varies greatly from place to place; however, the migration of the rifting has obvious regularity. It has experienced extensive rifts, migrated to the east of the Lord Howe Ridge, migrated to the west of the Lord Howe Ridge, migrated to the west of the Dampier Ridge, and finally migrated to both sides of the Lord Howe Ridge and then experienced multiple stages of compression transformation and deep burial transformation. For a single rift basin in Zealandia, it can be roughly divided into the volcanic eruption rift I stage, rift Ⅱ stage, rift Ⅲ stage, compression transformation stage, stress relaxation subsistence stage, shear compression deformation stage, and thermal-gravity equilibrium subsidence stage (Figure 6). The initial profile of the evolution section was modified from the seismic profile line 206/04 (Van de Beuque et al., 2003).
4.1.1 Early Cretaceous intracontinental volcanic rift I stage (c.120–95 Ma)
At the initial continental rifting stage during the early Cretaceous (Barremian-Cenomanian), a large number of alkaline non-marine volcaniclastic and volcanic rocks were deposited in each rift basin all over Zealandia, with possible coal and lacustrine sediments as the result of the incipient extensional rifting. On the Lord Howe Rise, there is direct evidence of this volcanic phase in DSDP site 207, which penetrated subaerial to shallow marine rhyolite tuffs and flows (Van de Beuque et al., 1996). There is a depositional hiatus in Cenomanian between the volcanic rift I sequence and its overburden system in response to the collision between Zealandia and the Pacific Plate (Veevers, 2000a,b).
4.1.2 Late Cretaceous Cenomanian–Campanian rift Ⅱ stage (95–83 Ma)
In the Late Cretaceous (Cenomanian–Campanian, 95–83 Ma), the rift basins were mainly developed on the east side of the Lord Howe Ridge, which was filled with a set of continental or marine-continental coal-bearing volcanic clastic rocks, grading upward to a shallow marine clastic-dominated succession (Gibson et al., 2015). Drillings of Tane-1 and Wainui-1 and outcrops onshore New Caledonia revealed that coal seams and carbonaceous mudstones were developed in the southern end of the New Caledonia basin and the northwest sea basin of New Zealand on the east side of the Lord Howe Ridge (Paris and Lille, 1977; Stagg et al., 2002).
4.1.3 Late Cretaceous (Campanian)-earliest Eocene rift Ⅲ stage (83-52Ma)
Since the Late Campanian in the Late Cretaceous, rifting migrated to the west of Lord Howe ridge and rifted from south to north. From the Late Cretaceous (Campanian) to Eocene, the rift basins were mainly filled with siliciclastic to calcareous sandstones and mudstones of marginal marine to neritic origin, grading upward to fine-grained bathyal sediments in the deeper depocenters of rift basins (Gibson et al., 2015). Then, the deep-sea calcareous ooze was deposited in the Paleocene.
4.1.4 Earliest Eocene to early Oligocene extrusion transformation stage (52–33.7 Ma).
From earliest Eocene to early Oligocene, this convergence along the eastern margin of Zealandia from New Guinea to New Zealand caused rift basin inversion and compressional uplift denudation, manifesting as the absence of upper Eocene and even middle-lower Oligocene in the majority of the rift basins in the Lord Howe Rise (Stagg et al., 2002; Van de Beuque et al., 2003).
4.1.5 Mid-late Oligocene stress relaxation subsistence transformation stage (33.7-23.3 Ma)
With the weakening of subduction-compression, the rift basins entered the stage of stress relaxation depression and thermal subsidence transformation stage and were filled with a set of nannofossil ooze, chalk, and volcanic ash in the Oligocene (Gaina et al., 1998; Gibson et al., 2015).
4.1.6 Miocene shear compression extrusion transformation stage (23.3–5 Ma).
In the Miocene, the plate boundary began to propagate through New Zealand as a convergent boundary, initiated by Neogene clockwise rotation of the Pacific subduction system (Van de Beuque et al., 2003). Since the late Miocene, oblique compression along the Alpine Fault caused an uplift of the Macquarie Ridge. This pulsating shear compression resulted in the fold uplift, denudation of rift basins, and Miocene multiple depositional discontinuities in southeastern Zealandia.
4.1.7 Pliocene deep-buried transformation stage (5-0 Ma)
Since the Pliocene, under the regional dynamics of thermal gravity sedimentation, the region in rift basins has been strongly subsiding and was strongly buried by the thick deep-sea ooze.
4.2 Thermal history simulation and analysis
PetroMod thermal history systems modeling predicts that the Upper Cretaceous coal-measure source rocks at the bottom of the rift basins on the east side of the Lord Howe Ridge have entered the gas stage since the Miocene (Figure 7). In the Paleocene, the coal-measure source rock in the deep rifts in the central part of the New Caledonia Basin entered the hydrocarbon generation threshold (Figure 7C). In the Miocene, the coal-measure source rock in the deep rifts in the central part of the Fairway Basin and New Caledonia Basin entered the wet and dry stages, respectively (Figure 7B). At present, the thermal evolution degree of Upper Cretaceous coal-measure source rocks gradually increases from west to east and that at the bottom of the rifts in the Fairway Basin have entered the stage of generating wet gas, while most of the source rocks in the New Caledonia Basin have entered the stage of generating dry gas (Figure 7A).
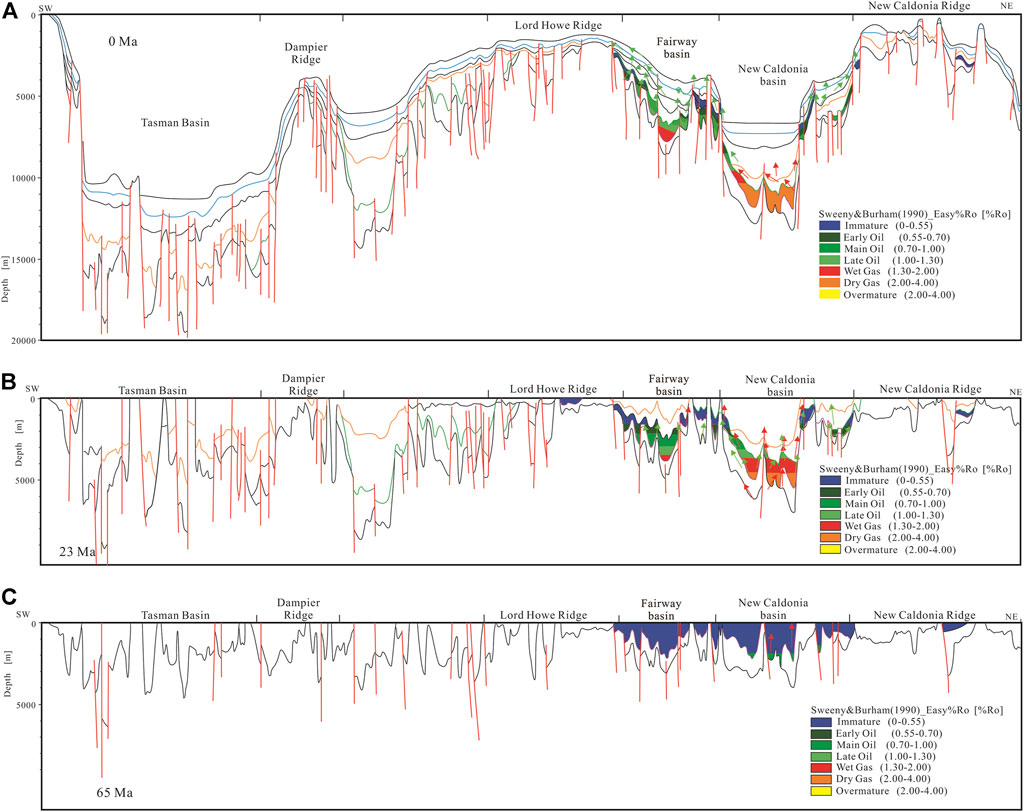
FIGURE 7. Large-section thermal history simulation profile across Zealandia (line 206/04 from Van de (Beuque et al. 2003).
4.3 Favorable zone forecast
Gas in hydrate deposits may come from thermogenic hydrocarbons deeper in the sediment pile (Claypool & Kaplan, 1974; Kvenvolden, 1995). The general coincidence of BSR-like distribution with the structural depression and depocenter in the Fairway Basin indicates a thermogenic component to the gas hydrates (Exon et al., 1998; Collot et al., 2009). The observed BSR-like depth of 0.7 s TWT below the seabed is consistent with the calculation of the predicted transition boundary in the eastern Lord Howe Rise and in the New Caledonia Basin based on the recorded thermal gradients (Exon et al., 1998). In the oceanic crust, sediment gas hydrates occur where bottom water temperatures approach 0 °C and water depths exceed about 300 m, and the lower limit of gas hydrate occurrence is up to about 2,000 m below the seafloor, determined by the geothermal gradient (Van de Beuque et al., 2003).
BSR-like are true BSRs in the Fairway and Taranaki basins, a thermogenic hydrocarbon gas source from the buried Upper Cretaceous coal-measure source rock. Such thermogenic gas could be generated from the organic-rich coal sediments in the rift basins and migrated up-dip onto the shallow strata through the Cretaceous-Paleogene extensional faults, perhaps in part beneath a seal of early forming hydrates. The shallow layer of rift basins shows potential for gas hydrate formation and exploration potential on the eastern side of the Lord Howe Rise (Figure 1), when the upper Cretaceous coal-measure source rocks are under the gas generation stage.
5 Conclusion
As the last area with unknown oil exploration potential, it has attracted the attention of energy scientists. Gravity and magnetic anomaly data, balanced cross-section, and thermal history modeling results show the following.
1) The rifting time in Zealandia varies greatly from place to place. It has experienced extensive rifts, migrated to the east of the Lord Howe Ridge, migrated to the west of the Lord Howe Ridge, migrated to the west of the Dampier Ridge, and finally migrated to both sides of the Lord Howe Ridge and then experienced multiple stages of compression transformation and deep burial transformation.
2) For a single rift basin in Zealandia, it can be roughly divided into the volcanic eruption rift I stage, rift Ⅱ stage, and/or rift Ⅲ stage, compression transformation stage, stress relaxation subsistence stage, shear compression deformation stage, and thermal-gravity equilibrium subsidence stage.
3) In the Late Cretaceous (Cenomanian-Campanian, 95–83 Ma), the continental or marine-continental coal-bearing clastic rock sequence was mainly developed in the rift basins on the east side of the Lord Howe Ridge.
4) The Upper Cretaceous coal source rocks were deposited, and their burial depth is higher than the gas generation threshold in the Fairway and New Caledonia basins and other basins on the eastern side of the Lord Howe Ridge. The deeply buried source rocks in the central part of the rift have entered the wet-late maturity stage since Cenozoic and may migrate along the fault and form a gas hydrate in the shallow seabed stable zone. The shallow layer of rift basins on the eastern side of the Lord Howe Ridge has the gas hydrate-forming potential.
Data availability statement
The original contributions presented in the study are included in the article/Supplementary Material; further inquiries can be directed to the corresponding author.
Author contributions
XF and the LS completed the writing of this thesis, and YY and YL participated in the clearing and editing of some drawings.
Funding
This study was supported by the Natural Science Foundation of China (No. 41102072) and the China Geological Survey Project (No. DD20160227).
Conflict of interest
The authors declare that the research was conducted in the absence of any commercial or financial relationships that could be construed as a potential conflict of interest.
Publisher’s note
All claims expressed in this article are solely those of the authors and do not necessarily represent those of their affiliated organizations, or those of the publisher, the editors, and the reviewers. Any product that may be evaluated in this article, or claim that may be made by its manufacturer, is not guaranteed or endorsed by the publisher.
References
Allen, P. A., and Allen, J. R. (1990). Basin analysis:principles and application. Oxford,England: Blackwell Scientific Publications, 282–283.
Auzende, J. M., Van de Beuque, S., Dickens, G., François, C., Lavoy, Y., Voutay, O., et al. (2000). Deep sea diapirs and bottom simulating reflflector in Fairway Basin (SW Pacific). Mar. Geophys. Res. 21, 579–587. doi:10.1023/a:1004848715044
Bache, F., Mortimer, N., Sutherland, R., Collot, J., Rouillard, P., Stagpoole, V., et al. (2013). Seismic stratigraphic record of transition from Mesozoic subduction to continental breakup in the Zealandia sector of eastern Gondwana. Gondwana Res. 26, 1060–1078. doi:10.1016/j.gr.2013.08.012
Beggs, J. M., Challis, G. A., and Cook, R. A. (1990). Basement geology of the Campbell plateau: Implications for correlation of the Campbell magnetic anomaly system. N. Z. J. Geol. Geophys. 33, 401–404. doi:10.1080/00288306.1990.10425696
Claypool, G. W., and Kaplan, I. R. (1974). “The origin and distribution of methane in marine sediments,” in Natural gases in marine sediments. Editor I. R. Kaplan (New York: Plenum), 99–139.
Cluzel, D., Aitchison, J. C., and Picard, C. (2001). Tectonic accretion and underplating of mafic terranes in the Late Eocene intraoceanic fore-arc of New Caledonia (Southwest Pacific); geodynamic implications. Tectonophysics 340, 23–59. doi:10.1016/s0040-1951(01)00148-2
Collot, J., Herzer, R., Lafoy, Y., and Geli, L. (2009). Mesozoic history of the fairway–aotea basin: Implications for the early stages of Gondwana fragmentation. Geochem. Geophys. Geosyst. 10, Q12019. doi:10.1029/2009gc002612
Corredorfo (1996). Three- dimensional geometry and kinematics of the Western thrust front of the eastern Cordillera. Columbia AAPG SEPM 5, 29–30.
Exon, N, F., Dickens, G. R., Auzende, J-M., Lafoy, Y., Symonds, P. A., and Van de Beuque, S. (1998). Gas hydrates and free gas on the Lord howe rise. PESA J. 26, 148–158.
Foucher, J. P., and Scient, P. (2006). Rapport de la campagne AUSFAIR/ZoNéCo-12. à borddu N/O Marion Dufresnes, 12–26.
Gaina, C., Müller, D., Royer, J. Y., Stock, J. M., Hardebeck, J. L., and Symonds, P. (1998). The tectonic history of the Tasman Sea; a puzzle with 13 pieces. J. Geophys. Res. 103, 12413–12433. doi:10.1029/98JB00386
Gaina, C., Müller, R. D., Royer, J.-Y., and Symonds, P. (1999). Evolution of the Louisiade triple junction. J. Geophys. Res. 104, 12927–12939. doi:10.1029/1999jb900038
Gamble, J. A., Wright, I. C., and Baker, J. A. (1993). Seafloor geology and petrology in the oceanic to continental transition zone of the kermadec-havre-taupo volcanic zone arc system, New Zealand: New Zealand. N. Z. J. Geol. Geophys. 36, 417–435. doi:10.1080/00288306.1993.9514588
Gibson, G. M., Roure, F., and Manatschal, G. (2015). Sedimentary basins and crustal processes at continental margins: From modern hyper-extended margins to deformed ancient analogues. Geol. Soc. Lond. Spec. Publ. 413, 9–33.
Kamp, P. J. J. (1986). The mid-Cenozoic Challenger rift system of Western New Zealand and its implications for the age of Alpine fault inception. Geol. Soc. Am. Bull. 97, 255–281.
Kroeger, K. F., Crutchley, G. J., Hill, M. G., and Pecher, I. A. (2017). Potential for gas hydrate formation at the northwest New Zealand shelf margin - new insights from seismic reflection data and petroleum systems modelling. Mar. Petroleum Geol. 83, 215–230. doi:10.1016/j.marpetgeo.2017.02.025
Kvenvolden, K. A. (1995). A review of the geochemistry of methane in natural gashydrate. Org. Geochem. 23, 997–1008. doi:10.1016/0146-6380(96)00002-2
Lafoy, Y., Brodien, I., Vially, R., and Exon, N. F. (2005). Structure of the basin and ridge system west of New Caledonia (southwest Pacific): A synthesis. Mar. Geophys. Res. (Dordr). 26, 37–50. doi:10.1007/s11001-005-5184-5
Luyendyk, B. (1995). Hypothesis for Cretaceous rifting of East Gondwana caused by subducted slab capture. Geology 23, 373–376. doi:10.1130/0091-7613(1995)023<0373:HFCROE>2.3.CO;2
McDougall, I., Maboko, M. A. H., Symonds, P. A., McCulloch, M. T., Williams, I. S., and Kudrass, H. R. (1994). Dampier ridge, Tasman Sea, as a stranded continental fragment. Aust. J. Earth Sci. 41, 395–406. doi:10.1080/08120099408728150
Mortimer, N., Campbell, H. J., Tulloch, A. J., King, P. R., Stagpoole, V. M., Wood, R. A., et al. (2016). Zealandia: Earth’s hidden continent. GSA Today 27–35. doi:10.1130/GSATG321A.1
Mortimer, N., Dunlap, W. J., Palin, J. M., Herzer, R. H., Hauff, F., and Clark, M. (2008b). Ultra-fast early Miocene exhumation of cavalli seamount, northland plateau, southwest Pacific ocean. N. Z. J. Geol. Geophys. 51, 29–42. doi:10.1080/00288300809509848
Mortimer, N., Hauff, F., and Calvert, A. T. (2008a). Continuation of the new england orogen, Australia, beneath the queensland plateau and Lord howe rise. Aust. J. Earth Sci. 55, 195–209. doi:10.1080/08120090701689365
Mortimer, N., Herzer, R. H., Gans, P. B., Parkinson, D. L., and Seward, D. (1998). Basement geology from three kings ridge to west Norfolk ridge, Southwest Pacific ocean: Evidence from petrology, geochemistry and isotopic dating of dredge samples. Mar. Geol. 148, 135–162. doi:10.1016/S0025-3227(98)00007-3
Mortimer, N., Hoernle, K., Hauff, F., Palin, J. M., Dunlap, W. J., Werner, R., et al. (2006). New constraints on the age and evolution of the wishbone ridge, Southwest Pacific cretaceous microplates, and zealandia–west Antarctica breakup. Geol. 34, 185–188. doi:10.1130/G22168.1
Mortimer, N., Rattenbury, M., King, P., Bland, K., Barrell, D., Bache, F., et al. (2014). High-level stratigraphic scheme for New Zealand rocks. N. Z. J. Geol. Geophys. 57, 402–419. doi:10.1080/00288306.2014.946062
Mortimer, N., Tulloch, A. J., and Ireland, T. R. (1997). Basement geology of Taranaki and wanganui basins, New Zealand: New Zealand. N. Z. J. Geol. Geophys. 40, 223–236. doi:10.1080/00288306.1997.9514754
Mortimer, N., Turnbull, R. E., Palin, J. M., Tulloch, A. J., Rollet, N., and Hashimoto, T. (2015). Triassic-jurassic granites on the Lord howe rise, northern Zealandia. Aust. J. Earth Sci. 62, 1–8. doi:10.1080/08120099.2015.1081984
Paris, J. P., and Lille, R. (1977). “New Caledonia: Evolution from permian to Miocene, mapping data and hypotheses about geotectonics,” in International symposium on dynamics in southwest pacifific, 27 august–2 september 1976, noumea, New Caledonia (Paris: Editions Technip), 195–208.
Pierrick, R., Julien, C., Rupert, S., Francois, B., Martin, P., Samuel, E., et al. (2015). Seismic stratigraphy and paleogeographic evolution of Fairway basin, northern Zealandia, southwest Pacific: From cretaceous Gondwana breakup to cenozoic Tonga–kermadec subduction. Basin Res. 29, 189–212. doi:10.1111/bre.12144
Ringis, J. (1972). The structure and history of the Tasman Sea and the southeast Australian margin. Sydney: Ph.D. thesisUniv. of New South Wales.
Schellart, W. P., Lister, G. S., and Toy, V. G. (2006). A Late Cretaceous and Cenozoic reconstruction of the Southwest Pacific region: Tectonics controlled by subduction and slab rollback processes. Earth-Science Rev. 76, 191–233. doi:10.1016/j.earscirev.2006.01.002
Shaw, R. D. (1979). On the evolution of the Tasman Sea and adjacent continental margins. Sydney, Australia: Ph.D. thesis, University of Sydney, 311.
Spandler, C., Rubatto, D., and Hermann, J. (2005). Late cretaceous-tertiary tectonics of the southwest pacific: Insights from U–Pb sensitive, high-resolution ion microprobe (SHRIMP) dating of eclogite facies rocks from New Caledonia. Tectonics 24, TC3003. doi:10.1029/2004TC001709
Stagg, H. M. J., Alcock, M. B., Borissova, I., and Moore, A. M. G. (2002). Geoscience Australia record. 2002/25.Geological framework of the southern Lord Howe Rise and adjacent areas
Stock, J., and Molnar, P. (1982). Uncertainties in the relative positions of the Australia, Antarctica, Lord howe, and pacific plates since the late cretaceous. J. Geophys. Res. 87 (B6), 4697–4714. doi:10.1029/jb087ib06p04697
R. P. Suggate, G. R. Stevens, and M. T. Te Punga (Editors) (1978). The geology of New Zealand (Wellington: New ZealandGovernment Printer), 819.
Tulloch, A. J., Kimbrough, D. L., and Wood, R. A. (1991). Carboniferous granite basement dredged from a site on the Southwest margin of the Challenger plateau, Tasman Sea: New Zealand. N. Z. J. Geol. Geophys. 34, 121–126. doi:10.1080/00288306.1991.9514449
Tulloch, A. J., Ramezani, J., Mortimer, N., Mortensen, J., van den Bogaard, P., and Maas, R. (2009). “Cretaceous felsic volcanism in New Zealand and Lord howe rise (Zealandia) as a precursor to final Gondwana break-up,” in Extending a continent: Architecture, rheology and heat budget. Editors U. Ring, and B. Wernicke (Canberra: Geological Society (London) Special Publication), 89–118.
Van de Beuque, S., Stagg, H. M. J., Sayers, J., Willcox, J. B., and Symonds, P. A. (2003). Geological framework of the northern Lord howe rise and adjacent areas, geoscience Australia record.
Veevers, J. J. (2000a). Change of tectono-stratigraphic regime in the Australian plate during the 99 Ma (mid-Cretaceous) and 43 Ma (mid-Eocene) swerves of the Pacific. Geology 28, 47–50. doi:10.1130/0091-7613(2000)028<0047:cotsri>2.3.co;2
Veevers, J. J. (2000b). “Chapter 16. Mid-cretaceous (99 Ma) and mid Eocene (43 Ma) events in the indo-Australian and antarctic plates and coeval swerves of the Pacific plate,” in Billion-year Earth history of Australia and neighbours in gondwanaland. Editor J. J. Veevers (Sydney: GEMOC Press), 102–109.
Keywords: thermal history simulation, natural gas hydrate, prospect prediction, Zealandia, development characteristics of the rift basins
Citation: Song L, Feng X, Yang Y and Li Y (2022) Distribution, development, transformation characteristics, and hydrate prospect prediction of the rift basins of northwest Zealandia in the Southwest Pacific. Front. Earth Sci. 10:997079. doi: 10.3389/feart.2022.997079
Received: 18 July 2022; Accepted: 22 August 2022;
Published: 28 September 2022.
Edited by:
Pibo Su, Guangzhou Marine Geological Survey, ChinaReviewed by:
Junpeng Wang, China University of Geosciences Wuhan, ChinaYonghe Sun, Chongqing University of Science and Technology, China
Fenquan Xie, Northeast Petroleum University, China
Copyright © 2022 Song, Feng, Yang and Li. This is an open-access article distributed under the terms of the Creative Commons Attribution License (CC BY). The use, distribution or reproduction in other forums is permitted, provided the original author(s) and the copyright owner(s) are credited and that the original publication in this journal is cited, in accordance with accepted academic practice. No use, distribution or reproduction is permitted which does not comply with these terms.
*Correspondence: Lijun Song, TGpzb25nQHhzeXUuZWR1LmNu