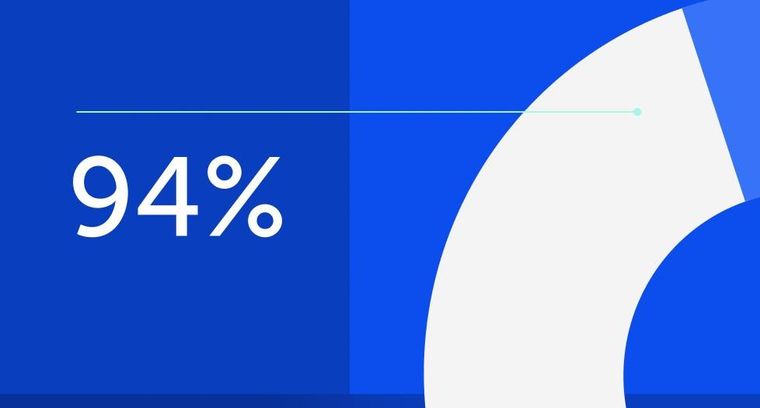
94% of researchers rate our articles as excellent or good
Learn more about the work of our research integrity team to safeguard the quality of each article we publish.
Find out more
ORIGINAL RESEARCH article
Front. Earth Sci., 13 January 2023
Sec. Geochemistry
Volume 10 - 2022 | https://doi.org/10.3389/feart.2022.996583
This article is part of the Research TopicVolcanic and Tectonic Degassing: Fluid Origin, Transport and ImplicationsView all 11 articles
Investigations of the hot spring water and gas in the volcanic region are involved in assessing geothermal resources and understanding groundwater circulation, volcano, and earthquake activities. The origins of water and gas of the hot springs, lakes, rivers, and rain in the Arxan volcanic region (AVR), northeastern (NE) China, were investigated by conducting a field survey and geochemical analysis. The low electrical conductivity (40–835 μS/cm) and low total dissolved solids (TDS, 23.83–540.00 mg/L) of the water samples indicate that they are fresh water. δ18O and δD values of the water samples range from −4.1% to −16.0% and from −61.3% to −119.9%, respectively. Enrichment of heavy isotopes in the rainwater and the crater lake waters was caused by evaporation. The component H2O of the water samples predominantly originated from the meteoric water, with less than 1 vol% contributed by deep-earth fluids. Ions in the rain sample were predominantly derived from sea salt and continental aerosol. Ions in the surface water samples had multiple origins (mineral dissolution, atmospheric, and anthropogenic sources). While the ions in the hot spring water were predominantly derived from both the dissolution of rocks and deep-earth fluids, the latter contributed 73%–87% of Cl− and 86%–99% of Na+ to the hot spring waters. Gases from the hot springs were composed of more than 95% N2 and less than 5% O2 and Ar, with 3He/4He ratios of 0.14–1.17 RA (RA=1.4×10−6). Excess N2, Ar, He, and CO2 of the hot springs were mainly derived from both the crust and upper mantle. About 3%–23% of the total He in the bubbling gases from the crater lake waters and hot springs is derived from the mantle, implying a supplement of heat energy from the mantle to the geothermal systems. Significantly, about 12% of the He dissolved in the Budonghe water is derived from the mantle, indicating that plenty of mantle-derived heat transported by deep-earth fluids keeps the river water from freezing. Our results indicate that Cl and Na ions and 3He/4He ratio are the feasible geochemical indicators for source partitioning of geothermal fluids.
The origins of geothermal fluids in the volcanic areas are involved in investigations of natural resources, environment, and hydrological cycle. Ion concentrations and isotope ratios of groundwater are the efficient indicators of water origin, circulation process of groundwater, geothermal potential, and volcanic activity (Giggenbach et al., 1993; Shaw et al., 2003; Taran, 2009, 2011; Benavente et al., 2016; Tardani et al., 2016; Rizzo et al., 2019). Therefore, geochemical observations of fluids can reveal the fluid origins, magmatic and seismic processes, and dynamic processes in the deep earth.
It is a major challenge in geoscience to decipher the complexity of fluids and minerals in the earth’s interior. Sources of dissolved solids in groundwater can be divided into three categories: dissolution of minerals in circulation, deep-earth fluids, and atmospheric and anthropogenic sources. The deep-earth fluids, meaning waters, gases, and supercritical fluids that are derived from the deep crust and mantle, may be composited with different genetic types of fluids such as initial, magmatic, metamorphic, deep hydrothermal, and diagenetic fluids that usually mix into geothermal fluids. The origins of ions and gases can be traced by using concentrations and isotopic ratios of the chemical species and techniques of statistical analysis (Han et al., 2019; Li, 2020; Chen et al., 2021). A variable non-sea-salt contribution usually causes the slope to differ from the seawater ratio and the intercept to differ from zero (Keene et al., 1986). Cl− and Na+ are the most reliable indicators for discriminating and partitioning sources of ions in the spring waters because they tend to exist in aqueous solution during water circulation, while other ions are partially fixed in secondary minerals.
Ion concentrations, δ18O and δD, of water have been widely used for investigating the origins of geothermal fluids. For instance, the high values of δ18O, δD, δ13C, and Cl− concentrations of spring waters in Oita Plain, Japan, implied an origin of andesitic-magmatic steam or metamorphic water, while the low values of δD and Li and B concentrations of groundwater indicated meteoric water (Amita et al., 2005). Li et al. (2012) investigated the water vapor sources of meteoric water in NE China using δ18O and δD data. The hydrochemical data indicated that the main stream water of the Kherlen River in eastern Mongolia, neighboring to the west of the Arxan volcanic region (AVR), were recharged by meteoric water from the headwater region of more than 1,650 m altitude (Tsujimura et al., 2007). δ18O and δD values of the lake, river, and well waters in the Lake Hulun Basin neighboring to the north of the AVR indicated that the waters originated from meteoric water and suffered evident evaporation (Gao et al., 2018).
The concentrations of gaseous components, He/Ne, 4He/20Ne, N2/Ar, 3He/4He, CO2/3He, and δ13C, provide insight on the sources of the gaseous species in the geothermal and volcanic systems (Giggenbach et al., 1993; Shaw et al., 2003; Du et al., 2005; Taran, 2009; 2011; Xu et al., 2013; Benavente et al., 2016; Rizzo et al., 2019; Bini et al., 2022), secondary processes of geothermal fluids (Zhang et al., 2016; Barry et al., 2020), volcanic and seismic activities (Chen et al., 2014; Zhou et al., 2015; Tardani et al., 2016), and contribution of volcanic gas to environment (Sun et al., 2020; Zhao et al., 2021). The regional variations in 3He/4He, δ13C of CO2, δ15N, and 87Sr/86Sr values of hydrothermal fluids along an intra-arc fault system in the southern Volcanic Zone of the Chilean Andes indicated the geothermal gas was a mixture of mantle-derived gas and radiogenic gas in the crust (Tardani et al., 2016). The molecular and isotopic data of hot spring fluids and fumarolic gases in the Tateyama volcanic hydrothermal system in Japan (Seki et al., 2019), the Tengchong volcanic area (Du et al., 2005) and western Sichuan Province (Du et al., 2006) in southwestern China, and the Wudalianchi volcanic field (Du et al., 1999; Xu et al., 2013), Changbaishan volcano (CBV) (Wei et al., 2016), and the Songliao continental rift system (Zhao et al., 2019) in NE China indicated the gases were predominantly derived from the mantle and crustal sources.
The mantle worldwide and regionally appears heterogeneous. The crust and upper mantle in NE China are characterized by fluctuation of Moho depth, mantle uplift in the rift valley and geophysical and geochemical heterogeneities (Jia and Zhang, 2020). The crust thickness in the Greater Khingan Range orogenic belt ranges from 34.5 to 43.5 km, while it becomes 32.4–36.2 km in the Songliao Basin in the east (Li et al., 2014; Jia and Zhang, 2020). The statistic histogram of 279 3He/4He ratios of mantle xenoliths in eastern China shows multiple peaks with a wider range from 0.1 to 12 RA (excluding eight individual data from 12.1 to 33 RA; RA is atmospheric 3He/4He =1.4×10−6) (Cui et al., 2022). Isotopic ratios of noble gases in mantle xenoliths in the orogenic belt and rift valley indicate that the mantle in NE China is heterogeneous. Obviously, the geochemical characteristics of the mantle in NE China differ from those in the middle ocean ridge and volcanic arc (Sano and Marty, 1995; Shaw et al., 2003; Roulleau et al., 2015).
The previous investigations in AVR mainly involved geology, volcanology, geothermics and hydrology (Tang, 1984; Sun, 1999; Zhang, 2017; Gu et al., 2017; Chen et al., 2021). The hydrogeochemical investigations for 36 hot springs (defined as their temperatures being 5°C higher than the local annual atmospheric temperature) around the Hot Spring Museum, a natural museum established for tourism and spa in the Arxan city, suggested that the hot spring waters were chemically classified into Ca·Na-HCO3·SO4, Na·Ca-HCO3, and Na-HCO3 (Gu et al., 2017; Zhang, 2017), and the residence time of high-temperature hot spring waters ranges from 70 a to 90 a, and that of low-temperature hot springs/shallow groundwater is about 10 a (Gu et al., 2017). Recently, the groundwater in the basalts of Arxan was considered exogenous water from the Tibetan Plateau based on the data of the water balance relationship and δ18O and δD values (Chen et al., 2021). Zhao et al. (2021) reported that gases from two hot springs in the AVR were mainly composed of N2 with low 3He/4He ratios (c. 0.1 RA), high 4He/20Ne ratios (150–380), and δ13C values of CO2 (-6.2% to -13.6%). They concluded that the spring gases were mainly derived from the crust. So far, the origins of hot spring fluids in the AVR remain debated. This paper aims at revealing the contribution of deep-earth fluids to the geothermal system in the AVR based on the molecular and ion concentrations and isotope compositions of the hot spring fluids.
The AVR is famous for a lot of hot springs and Quaternary volcanoes. It is located at the west margin of northeastern (NE) China, where volcanic eruptions were very violent in the Cenozoic Era and produced more than 590 volcanoes and about 50,000 km2 of exposed basalt (Liu et al., 2001). The altitudes in the AVR range from 900 m to 1,700 m (Figure 1A). The annual mean temperature is −2.5°C, monthly averages of temperature range from 10.5°C to −31.2°C with the lowest temperature of -45.7°C recorded on 1st January 2001. Annual precipitation was 450 mm during 1951–2015, of which 80%–90% fell during June–September, whereas annual evaporation capacity is up to 1,116 mm (Gu et al., 2017; Chen et al., 2021).
FIGURE 1. (A) Topography of the study area and vicinity, showing main faults and the sampling sites (the image from Google Earth); the inset of the DEM image shows the location of the study area; sampling sites: Ⅰ- Tuofengling; Ⅱ- Dujuanhu; Ⅲ- Tianchi; Ⅳ- Dichi; Ⅴ- Budonghe; Ⅵ- Jinjianggou; Ⅶ- Wuliquan; Ⅷ- the Hot Spring Museum. (B) Hot spring locations at the sampling site no. Ⅷ, 45 springs occur in an area of 700 m long from north to south and 70 m wide from east to west (modified after Zhang (2017)). (C) Geological map of the Arxan volcanic region (square C in A); 1 - Holocene fluvial sediments; 2 - Neogene basalts, andesitic basalt, and andesite; 3 - Late Jurassic rhyolite and rhyolitic pyroclastic rocks; 4 - Paleozoic clastic rocks intercalated limestone; 5 - moyite; 6 - fault; 7 - sampling site and number (modified after Xie et al. (2011)). (D) Distribution of Holocene volcanism and sampling sites (square D in A), 1 - Holocene fluvial sediments; 2 - Late Jurassic rhyolite and rhyolitic pyroclastic rocks; 3 - Holocene alkali olivine basalts; 4 - Holocene crater; 5 - sampling site and number (modified after Fan et al. (2011)).
The AVR tectonically belongs to the Greater Khingan Range orogenic belt that connects with the northern part of the continental rift valley on the east. There are mainly five groups of faults in the study area: NEE, NNE, NW, NNW, and EW trending faults. The NEE-trending Halaha River fault cuts the lithosphere with a length of ca. 500 km. The deep-cut faults provide two channels for Quaternary magma migration from the mantle: one displays a high temperature and fluid-enriched body approaching the depth of 10–12 km, and another has become cold above a depth of 30 km (Tang et al., 2005). The series of NNW-trending basement rifts deeply cut the crust, favoring the deep cycle of groundwater and the formation of hot springs.
Mesozoic igneous rocks are widely distributed in the AVR with a small area of Paleozoic clastic strata (Figure 1C). The Paleozoic strata are composed of Late Ordovician metamorphic clastic rocks and volcanic-sedimentary clastic rocks, Late Silurian metamorphic clastic rocks, and Early–Middle Devonian clastic rocks sandwiched with biogenic limestone. The Mesozoic strata are mainly Late Jurassic rhyolitic lava and volcanic clastic rocks. The Cenozoic strata are Neogene clastic rocks and black basalts and Quaternary sediments (Fan et al., 2011; Xie et al., 2011).
Magmatic rocks in the study area are mainly the Early Indosinian and the Late-Middle Yanshanian porphyroid potassic granite, monzonitic granite, and moyite and Neogene and Quaternary basalts (Figures 1C, D). Alkaline basalts in the Greater Khingan Range are characterized by high MgO and Ni concentrations, high CaO/Al2O3 ratios, enrichment of large lithophile elements and positive Nb-Ta anomalies, and moderately depleted Sr–Nb–Hf isotopic ratios. The basalts could be derived from the deep primary mantle, possibly from ancient primordial peridotite (Fan et al., 2003; Xue et al., 2019). Quaternary basalts in the AVR cover an area of ca. 100 km2 and erupted from more than 54 craters in the Middle-Late Pleistocene and Holocene (Fan et al., 2011). The Yanshan Volcano is the youngest volcanic crater and has a radiocarbon age of 2,000 years (Bai et al., 2005).
Groundwaters in the study area can be classified into three types: (1) pore water in Quaternary fluvial sediments, discharging through river beds; (2) bedrock fissure water in Late Jurassic volcanic rocks, recharged by meteoric water and discharged through underflow to the river valley; and (3) vein-type fissure water in the fault zones (Chen et al., 2021). Surface waters include river water (the Halaha River and the Arshangole River), barrier lakes, and crater lakes. There are 45 hot springs around the Hot Spring Museum in Arxan town (Figure 1B), whose water temperatures range from 2 to 40°C. A unique phenomenon is that the distance between the low-temperature hot spring (2°C) and the high-temperature hot spring (40°C) is just 0.3 m, which could be caused by different amounts of deep-earth fluid recharge.
The heat flow in the Greater Khingan Range is 40.2 mW/m2. The estimated Moho heat flow is 33.2 mW/m2 (Sun, 1999). Though the regional heat flow is low, three geothermal fields have been found in the AVR (Qi et al., 2012). One is the Jinjiangguo (JJG) Valley geothermal field, which is composed of two geothermal zones controlled by the NE and NNW trending faults in granite and characterized by lower resistance. The geothermal water age was dated as 8,150 ± 90 a (Qi et al., 2012). The second one is the Yinjianggou Valley (YJG) geothermal field, which is controlled by a NNW-trending fault. The third one is the Wuliqiao geothermal field, which is controlled by EW- and NNW-trending faults (Qi et al., 2012). The thermal energy is derived from the mantle (magma) and radiogenic heat in the crust (Qi et al., 2012). The heat energy of the hot springs around the Hot Spring Museum could be mainly derived from radiogenic heat in the crust (Sun, 1999).
The water and gas samples were collected from the AVR in August 2010 (Figure 1). The field measurements of water temperature, electrical conductivity (EC), and pH were conducted with the portable instruments. The samples for hydrochemical analysis were collected with the 250-ml plastic bottles, and for stable isotopic analysis of H and O with the 2-ml plastic bottles. The gas samples were collected by the gas drainage method in 1,000-ml glass bottles and sealed with rubber caps (Du et al., 2006). Totally, 17 water samples were collected from the springs, crater lakes, river, and rain, and eight gas samples were collected including seven bubbling gases and one dissolved gas in the water of the Budonghe River (at the sampling site Ⅴ in Figure 1). The rain sample is the thundershower water dropped down from the building rooftop after filtering dust particles.
Concentrations of Li+, Na+, NH4+, K+, Ca2+, Mg2+, F−, Cl−, NO3−, and SO42- were measured with a Dionex ICS-900 ion chromatography system with the standard configuration (reproducibility within ±2%). The HCO3− concentrations were measured by the standard titration procedures with a ZDJ-100 potentiometric titrator (reproducibility within ±2%) (Chen et al., 2014). Ion charge balance errors (I.B.) of the hydrochemical data are less than ±5%. The total dissolved solid (TDS) value was calculated by,
TABLE 1. Ion concentrations and isotopic ratios of the water samples from the Arxan volcanic region.
The N2, O2, Ar, CO2, CH4, and He concentrations of the gas sample were analyzed with a Finnigan MAT-271 mass spectrometer, with a precision of ±0.1%. Helium and neon isotope compositions of the gas samples were measured with an MM5400 mass spectrometer at the Laboratory of Gas Geochemistry, the Institute of Geology and Geophysics, the Chinese Academy of Sciences. δ13C values of CH4 and CO2 were measured with the GC-IRMS analytical system, a gas chromatography (Agilent 6890)–stable isotope ratio mass spectrometer, and the values of C13C are reported relative to PDB in per mill with an error of ±0.5‰ (Li et al., 2007; Zhou et al., 2015). It is worthy of mention that the He concentration and 4He/20Ne ratios of dissolved gas in the Budonghe water are double of atmospheric values. The 3He/4He ratio was less than the atmospheric ratio, which indicates the sample was not contaminated by air during sampling and analysis.
The temperatures of the hot spring waters are in a range of 2.3°C–37.5°C. The water temperatures of the springs no. 5 at the Hot Spring Museum and Wuliquan (at 2.5 km northwest Arxan town) are lower than those of Budonghe water (7.5°C). The water samples have low electric conductivity (40–835 μS/cm) and low TDS (23.83–540.00 mg/L). TDS values of the surface (river and lake) waters are lower than those of the hot spring waters. Abundance of the majority cations and anions is generally in the order of Na+>Ca2+>Mg2+>K+>NH4+ and HCO3−>SO42−>Cl−, NO3−>F−, respectively. δ18O and δD values of the water samples range from -4.1% to −16.0% and -61.3% to -119.9%, respectively. The lake waters are more enriched in heavy isotopes (18O and D) than the hot spring waters (Table 1).
The molecular and isotope compositions of the gas samples are listed in Table 2 including some published data (Zhao et al., 2021) in the AVR for comparison. The N2 concentrations of the gas samples from the AVR are more than 95%, and others together are less than 5%. 3He/4He ratios are in a range of 0.20×10−7 ∼ 1.64×10−6. 4He/20Ne ratios of the surface waters are approximately equal to the atmospheric value (0.32), but those of hot spring waters are much higher than the atmospheric value. CO2 concentrations are lower (0.13%–0.41%) and δ13C of CO2 from -22.3% to -6.2%. δ13C of methane (C1) from Tianchi is -51.5%, indicating the biogenic origin of C1, but δ13C of C1 from the geothermal well in JJG is 1.4‰, hinting at an abiogenic origin. 21Ne/22Ne and 20Ne/22Ne ratios are approximated by the atmospheric values (0.029 and 9.78, respectively).
TABLE 2. Molecular and isotope compositions of gases from the springs and lakes in the Arxan volcanic region.
Most δ18O and δD values of the water samples are scattered nearby the global meteoric water line (GMWL, δD = 8.17δ18O + 10.35, Rozanski et al., 1993) and the local meteoritic water line (LMWL) in NE China (δD = 7.20δ18O − 2.39, Li et al., 2012), but some shift far to the right side of the lines (Figure 2). The coordinates (−13.1% and −97.0%) of the intersection between the GMWL and LMWL can be regarded as the mean value of the initial source water (Han et al., 2019). The slop and intercept of the LMWL are smaller than those of the GMWL, indicating evaporation effect and multiple origins of waters (Tsujimura et al., 2007; Li et al., 2012; Han et al., 2019). The isotopic data of the spring and river waters in the AVR, the spring waters in Wudalianchi (Du et al., 1999) and CBV (Shangguan et al., 1996), and the river waters in Oita Plain, Japan (Amita et al., 2005), are scattered along the GMWL, indicating that those waters are mainly derived from the Pacific Ocean. This conclusion is supported by the fact that the meteoric water in northeastern China is mainly derived from the Pacific Ocean during the summer monsoon season. The wide ranges of δ18O and δD values (Table 1) indicated that the different kinds of waters experienced different processes of isotopic fractionation.
FIGURE 2. Plot of δ18O vs. δD; dashed line (GMWL) is the global meteoric water line (Rozanski et al., 1993); sold line (LMWL) is the local meteoric water line (Li et al., 2012); red circle is the intersection of the GMWL and LMWL; red solid circle is the rain sample; for comparison, our data and the reported data of spring and river waters in the AVR (Gu et al., 2017; Li, 2020; Chen et al., 2021), Oita HSW and Oita RW - hot spring water and river water in Oita Plain and Arita - deep geothermal fluid in Japan (Amita et al., 2005), WDLC - spring water in the Wudalianchi volcanic area (Du et al., 1999), CBS - data of hot spring water in the Tianchi volcanic area in Changbai Mountains (Shangguan et al., 1996), and Hulun Lake - lake water data (Liang et al., 2017) were plotted.
δ18O and δD values of the rain sample (AW-0) are scattered far from the LMWL (Figure 2). The heavier isotope compositions of the rain sample are concordant with those of rain in the Hulun Lake Basin (Liang et al., 2017), NE China (Li et al., 2012), and eastern Mongolia in summer (Tsujimura et al., 2007). Therefore, the heavier isotope compositions of the rain sample can be attributed to the seasonal isotopic variations of meteoric water. In the east-west extended climate zone from the Greater Khingan Range to the Mongolia Plateau, the isotopic compositions of H and O in meteoric water are characterized by obviously seasonal variation of more negative values in winter than those in summer (Tsujimura et al., 2007; Gao et al., 2018; Li, 2020).
The surface water samples (AW-1–AW-5) were relatively enriched in heavy isotopes but depleted in the TDS (<62 mg/L) (Table 1; Figure 2). δ18O and δD values of water samples from the Dichi (a crater lake of the maar volcano) and Budonghe are similar. The water samples from the Tianchi and Tuofengling (TFL) Tianchi, two crater lakes on the volcanic cones, are more enriched in heavy isotopes. The Dujuehu is a barrier lake, whose water is more enriched in D (Table 1). The d-excess (δD-8.17δ18O) of the lake water samples is much lower than that of the LMWL (Figure 2). Such isotopic shifts can be mainly attributed to the evaporation of raindrops and lake water caused by higher evaporation capacities.
δD and δ18O values of seven water samples collected at the Hot Spring Museum and three water samples from the hot springs in JJG and previous results (Sun, 1999; Gu et al., 2017; Li, 2020; Chen et al., 2021) scattered nearby the LMWL, which indicates that the hot spring waters in the AVR mainly originated from meteoric water (Figure 2). The depletion of heavy isotopes in the hot spring waters can be explained by the air temperature effect because the isotopic fractionations of O and H in meteoric water are mainly related to the thermal dynamics of water vapor condensation. Both δD and δ18O values of meteoric water and surface air temperature in north China (latitude higher than 36°N) have positive correlation. δ18O/T gradient was 0.52‰/°C, and δD/T gradient was 4.27‰/°C (Zhang et al., 2008). Let us take the Hailun station in the Songliao Basin (47.45°N, 126.93°E, an altitude of 236 m, an annual mean of 5.5°C, and 480 km to NE Arxan) of the Chinese Network of Isotopes in Precipitation as a reference, the mean values of δ18O and δD of meteoric water are −12.5% and −92.2% at the Hailun station (Liu et al., 2014). The temperature difference of 8.0°C between Hailun and Arxan results in the δ18O and δD differences of 4.2% and 34.2%, respectively. Consequently, the δ18O and δD values of meteoric water in Arxan are estimated to be, respectively, -16.7% and -126.4%. In reverse, the isotopic coordinates of intersection between the GMWL and LMWL, regarding the mean value of recharge source water, minus the O and H isotope fractionation caused by decreasing temperature, are equal to −17.3% and 121.2%, respectively, which are concordant with the isotopic ratios of the spring waters in the AVR (Table 1).
The TDS values of all water samples range from 23.83 to 540.00 mg/L, indicating the waters are fresh. The waters can be chemically classified into four groups, namely, Na-HCO3, Ca-HCO3, Ca·Mg-HCO3, and Na·Ca-HCO3 by Sokolov’s method (Sokolov, 1966). All the spring water samples are Na-HCO3 type, four samples from the crater lakes are Ca·Mg-HCO3, Na·Ca-HCO3, Ca-HCO3, and Na-HCO3, one from the Budonghe River is Ca·Mg-HCO3, and a rain sample is Ca-HCO3 type (Table 1).
The results of the correlation analysis for the hydrochemical parameters show that there is a strong positive correlation (r > 0.8, Table 3) between TDS and Li+, Na+, K+, NH4+, HCO3−, Cl−, F−, and SO42-, indicating that the TDS of the water samples is mainly controlled by deep-earth fluids and the dissolution of rocks. This is supported by the higher Na+ and Cl− concentrations, TDS, and temperatures of the hot spring waters and the residence time (Gu et al., 2017). Cui et al. (2022) reported that 85% of the TDS of continental meteoric water was composed of SO42-, Ca2+, NO3−, NH4+, and Cl−, and NO3− was mainly anthropogenic origin. NO3− has shown an obviously positive correlation with NH4+, Cl−, Ca2+, and K (r> 0.5) and a weak positive correlation with TDS (Table 3), indicating the contribution of atmospheric and anthropogenic sources, While the weak negative correlation between the couple ions of Mg-Li, Mg-Na, SO4-Mg, HCO3-Mg, and TDS-Mg (Table 3) may reflect water–rock interaction (dissolution, ion exchange and deposition).
In the diagram of Na–Mg–Cl concentrations (Figure 3), the data of water samples from the AVR scatter far from the marine precipitation lines (Keene et al., 1986) and show evidently different slops, indicating the contribution of those ions from marine origin is negligible. The lines of Cl-Na and Cl-TDS in the water samples and solutions leaching granodiorite at ambient conditions (Du et al., 2010) have similar slopes and intersections, while those of Na-Mg are different. TDS, Cl, and Na in the samples are much higher than those in the leaching solutions (Figure 3), which indicate the ions mainly originate from Na-Cl-enriched deep geothermal fluids and the dissolution of Na-bearing minerals.
FIGURE 3. (A) Diagram of Na–Mg–Cl concentrations; the inset is at the enlarged left bottom corner; red dashed point and black solid lines stand for marine precipitation data from Keene et al. (1986); blue-dashed and brown-dashed point lines for solutions of soaking granodiorite grains for 168 h, which were extended for comparison, and two points for data of soaking 2,000-μm grains of granodiorite (Gran) and trachyandesite (Tran) under the ambient condition for 12 h (Du et al., 2010); point lines for data of this study. (B) Diagram of Na-TDS-Cl; the inset is at the enlarged left bottom corner; the short extended dashed line for data of solutions of soaking granodiorite grains of different sizes under the ambient condition for 168 h (Du et al., 2010).
Plagioclase is a main component of the granite, granodiorite, and andesitic basalts that are widely distributed in the AVR (Figure 1). Na and Ca cations can be dissolved into solution through the reactions between plagioclase and CO2-bearing water.
The decomposition reactions of plagioclase can be promoted by an increase in temperature, CO2 partial pressure, and a mixture of Cl-S-enriched deep-earth fluids.
The Mg/Na and K/Na ratios of the rain and surface water samples from the AVR are concordant with those of marine precipitation (Keene et al., 1986) and water-soluble ions (Mg/Na=0.6 and K/Na=0.4) in aerosol samples in NE China (Shen et al., 2007). This indicates that the ions in the rain sample and the surface waters are mainly derived from sources of continental aerosol, sea salt, and rock dissolution.
The Cl− concentrations of the rain and surface water samples are much lower than those of the hot spring waters, except for the sample AW-14 (Table 1; Figure 3), indicating the contribution of atmospheric and anthropogenic Cl to the hot spring waters is neglectable. Additionally, the values of Cl− and Na+ concentrations and Mg/Na, Cl/Na, and Cl/Mg ratios of rock solutions differ from those of the hot spring water samples (Figure 3). The Na+ concentration in the solution is about 2 magnitudes higher than Cl− concentration if granitic and andesitic rocks are dissolved in equal proportion. The experiments of soaking basalt and trachyandesite grains display that concentrations of Cl−, SO42-, and Na+ approach the highest values in short time, and then, the highest value of Cl− of about 3 mg/L show no obvious variation with increasing soaking time, while others varied with soaking time (Du et al., 2010). Similarly, the observed and experimental results indicated that the meteoric waters picked up chloride more rapidly than they would be congruent dissolution of basalts, resulting in Cl− of 1–5 mg/L in the spring water (Gislason and Hans, 1987; Gislason et al., 1993). Such data indicate that the amount of Cl− in groundwater contributed by meteoric water dissolving igneous rocks ranges from 2 to 4 mg/L. Therefore, the ions in the hot spring water could be a mixture of rock solution and deep-earth fluids.
Cl− percentages of dissolution of rock and deep-earth fluids in the hot spring waters can be estimated by the two-member linear mixing model (Figure 4). Assuming Cl− concentration of the end member of dissolution of rock be 3 mg/L (Gislason and Hans, 1987; Du et al., 2010) and that of the end member of deep-earth fluids be 20,000 mg/L (Oita deep geothermal water Cl: 18,649–23,787 mg/L and Na: 12,213–15,813 mg/L (Amita et al., 2005)), the volume percentages of deep-earth fluids in the AVR hot spring waters were estimated in the range from 0.041 to 0.103; in other words, 73%–87% of Cl− in the hot spring waters were derived from deep-earth fluids. Moreover, taking the Na+ concentration of 3 mg/L in the 168-h soaking solution (Du et al., 2010) as the end member of dissolution of igneous rocks and that of 13,000 mg/L in Oita deep-earth fluids as another, the volume percentages of deep-earth fluids in the spring waters were estimated in the range of 0.14–1.66; that is, 86%–99% of Na+ in the hot spring waters were derived from deep-earth fluids (Table 1). Obviously, less than 1 vol% of deep-earth fluids mixed in the hot spring waters can be ignored when assessing groundwater volume, but the contributions of ions and heat energy from the deep-earth fluids to the geothermal system are significant.
FIGURE 4. Source partitioning of Na–Mg–Cl ions in the AVR waters; data of deep-earth fluid after Amita et al. (2005); Cl AVR and Mg AVR are in this study; others (solutions of soaking granodiorite and trachyandesite) are after Du et al. (2010); LPR is the local precipitation region (rectangle); WRR is the water–rock reaction region (oval); DTR is the deep-earth fluid region; AMW displays approximately andesitic-magmatic water.
The measured N–He–Ar abundance system in AVR obviously differs from the gaseous components in air-saturated water (ASW) (Table 2). The ratios of N2/O2 and Ar/O2 of the samples are 44–67 and 0.07–1.11, respectively, except for 137.6 and 3.1 of the gas samples from the hot springs in JJG, which obviously differ from those of air (3.7 and 0.04) and those in ASW (1.81 and 0.03 (Weiss, 1970)). The N2/He ratios of the gas samples range from 116.4 to 663.0, obviously differing from the atmospheric (15,000) and ASW values (11,400 (Weiss, 1970; Taran, 2011)). He concentrations in the gas samples are approximately 4 magnitudes larger than the atmospheric value, but the N2/He ratios are about 2 magnitudes lower than ASW’s value, and the 4He/20Ne ratios of 257.8–385.3 of the hot spring gases are much higher than the atmospheric value (0.318 (Porcelli et al., 2002)) and ASW’s value (0.222 at 3°C (Weiss, 1971)). The disparities of molecular parameters between the samples and air or ASW likely indicate that amounts of atmospheric N2 and He carried by meteoric water to the hot spring gases are not significant.
Sources of gases can be illustrated by the He–Ar–N2 ternary diagram (Giggenbach et al., 1993; Rizzo et al., 2019; Bini et al., 2022). O2 and Ar in hot springs are often considered to be derived from air based on the fact that O2 and Ar concentrations in the atmosphere are much higher than those in the lithosphere, and hot spring water is mainly derived from meteoric water. In the case where all gaseous components in underground water originate from air, a part or all of the oxygen consumed by microbe respiration and the oxidation of organic and inorganic matter in the water circuit may result in N2-rich gas. The low CO2 concentrations in the gas samples indicate that the O2 consumption of microbe respiration and the oxidation of organic matter in the hydrological circuit are neglectable. Data of our two gas samples are plotted nearby a magmatic source in the He–Ar–N2 ternary diagram (Figure 5, Taran, 2011), indicating the gaseous components mainly originate from deep-earth fluids (Benavente et al., 2016). The data of the same hot springs obtained at different times (Zhao et al., 2021) shift to the air source in Figure 5, probably hinting at temporal variation or air contamination. The excess N2 (N2*), Ar (Ar*), and amount of non-atmospheric components can be estimated using the concentration of N2/O2 and Ar/O2 ratios of ASW (Taran, 2009). Assuming the measured O2 is derived from air and without consideration of O2 consume in the hydrological circuit, Ar* can be estimated by Ar*= (Ar-O2/20.5)/Ar, and excess N2 can be estimated by N* =(N2-1.814 O2)/N2 (Table 2). The calculated results indicated that more than 95% of N2 and more than 91% of Ar in the gas samples were of no-atmospheric origin (Table 2). N2*/Ar* ratios ranged from 52.21 to 88.04, less than those of geothermal gases in the volcanic arc (107–388 (Roulleau et al., 2015)) and mantle (350 (Taran, 2011)). The low N2*/Ar* ratios can be attributed to the contribution of radiogenic Ar produced by 40K that is relatively enriched in the granodiorite and alkaline basaltic rocks (Figure 1). Higher concentration He can be attributed to mantle-derived He and radiogenic He produced by the U-Th series enriched in the granodiorite and acid-intermediate volcanic rock in the crust.
FIGURE 5. Diagram of He–Ar–N2 concentrations of the hot spring gases in the Arxan volcanic region, M - magmatic source; H - hydrothermal source (Taran, 2011).
In most cases, helium in geothermal fluids originates from atmospheric, crustal, and mantle sources (Sano et al., 1985; Ballentine et al., 2002; Zhou et al., 2015; Bini et al., 2022). Several methods were proposed for source partitioning of He in geothermal gases. For example, Sano et al. (1985) used an equation set to calculate the He percentages of atmospheric, crust, and mantle sources on the basis of the given 3He/4He and 20Ne/4He ratios of those sources. Using a plot of CO2/3He vs. δ13C with the given values of the same parameters for the atmospheric, crust, and mantle sources, the source partitioning of He and CO2 in the geothermal gases can be illustrated (Sano and Marty, 1995; Hilton, 1996). Using the corrected 3He/4He ratios deducted for atmospheric He by the 4He/20Ne ratio of air or ASW, the no-atmospheric He can be estimated by the two-end member model of crust and mantle sources (Ballentine et al., 2002; Zhou et al., 2015).
The samples of gases in the hot springs in AVR are characterized by low 3He/4He and CO2/3He ratios (Table 2), which obviously differ from those of hydrothermal fluids in the Wudalianchi volcanic area (low 3He/4He and high CO2/3He) in the rift valley (Du et al., 1999; Xu et al., 2013) and Tianchi volcanic area (high 3He/4He and low CO2/3He) neighboring the rift valley to the west in NE China (Shangguan et al., 1996), and much less than those of volcanic and geothermal gases in the volcanic arc and middle ocean ridge (Sano and Marty, 1995; Shaw et al., 2003). The 4He/20Ne ratios of the gas samples are in the range of 0.34–385.3, and 4He concentrations and 3He/4He ratios of the spring gases are independent (Table 2). The CO2/3He ratios of the gas samples are 3 magnitudes less than those of the mantle (MORB-type) (1.5×109 (Sano and Marty, 1995)), 3–5 magnitudes less than the values of fumaroles and hot springs in the volcanic areas in Japan (7.74×109–1.18×1011 (Sano and Marty, 1995)) and in the Nicaraguan volcanic front (Shaw et al., 2003), and 8 magnitudes less than the values of sediment and limestone (1×1013 (Sano and Marty, 1995; Xu et al., 2013)). Therefore, it can be concluded that that He in the gas samples has multiple origins.
The 20Ne/22Ne ratios of the gas samples are close to the atmospheric value, and the 21Ne/22Ne ratios are slightly less than the atmospheric value (Table 2), indicating that Ne is mainly derived from air. Assuming all the 20Ne in the geothermal gases is derived from air, the measured 3He/4He ratios of geothermal gases can be corrected by 4He/20Ne ratios of air or air-saturated water (Hilton, 1996; Ballentine et al., 2002; Xu et al., 2013; Zhou et al., 2015; Zhao et al., 2021). For eliminating the air contribution resulted from air dissolved in meteoric water into groundwater, the measured 3He/4He ratios were corrected by the ASW’s 4He/20Ne ratio using the equation, (3He/4He)* = {(3He/4He)sample—r}/(1—r), where r = (4He/20Ne)(air or ASW)/(4He/20Ne)sample (Xu et al., 2013). ASW’s 4He/20Ne ratio was calculated using the data of He and Ne solubility in water at 3 °C (4.814×10−5 ml/kg and 21.71×10−5 ml/kg (Weiss, 1971)), and 20Ne content is 90.48% of total Ne (Porcelli et al., 2002), regardless of isotopic fractionations of He and Ne caused by air dissolution. The corrected 3He/4He ratios of the gas samples from the Tianchi, JJG Tianchi crater lakes, the Budonghe water, and spring no. 0 differ evidently from the measured ratios, but those of the hot spring gas samples with high 4He/20Ne ratios and high He concentrations show no change (Table 2). The percentages of atmospheric He and no-atmospheric He in the gas samples can be calculated by the following equation:
where
Helium derived from the crustal and mantle origins can be quantitatively estimated by the two-member mixing model using the ASW (or air)-corrected 3He/4He ratios (Ballentine et al., 2002; Zhou et al., 2015; Seki et al., 2019; Zhao et al., 2021). Traditionally, assuming the mantle be homogenous, the 3He/4He ratios of the mantle and crust sources were proposed to be 8 ± 1 RA and 0.02 RA, respectively (Sano and Marty, 1995). However, the chemical heterogeneity of the upper mantle must be emphasized to estimate the amount of mantle-derived He in the hot spring gas, which is involved in assessing heat flux and potential heat resources in the geothermal fields. The 3He/4He ratios of basalts and mantle xenoliths in the different tectonic regions such as the mid-ocean ridge, volcanic arc, subcontinent, and orogenic zone evidently differ from each other and vary in a wide region within the same tectonic unit (Graham, 2002; Porcelli et al., 2002; Xu and Liu, 2002; Zhao et al., 2021; Randazzo et al., 2022). The 3He/4He ratio for the European subcontinental lithospheric mantle source was proposed as 6.1 ± 0.9 RA (Randazzo et al., 2022). The isotopic compositions of noble gases in mantle peridotite xenoliths in eastern China vary from place to place and are obviously lower than MORB values (Cui et al., 2022). The lithospheric mantle and the asthenosphere in the continent area are important sources of gases in the upper mantle, which differs from the orogenic ranges to the rift valley in NE China (Xu et al., 2013). The mantle peridotite xenoliths in the Arxan–Chahe region (Liu et al., 2001; Sui et al., 2012) are geochemically similar to those in the Hannuoba region (Song and Frey, 1989; E and Zhao, 1987). Both the Arxan and Hannuoba volcanic regions are located in the orogenic belt of the Greater Khingan Range–Taihang Mountains, neighboring the rift valley on the east. The alkali basalts in the two regions formed in Miocene–Holocene, of which the REE pattens and spiderweb diagrams are similar (Zhao, 2010). The mantle xenoliths found in the two regions are mainly Al-rich lherzolites (E and Zhao, 1987). Therefore, the upper mantle from the Hannuoba region to the south part of the Greater Khingan Range can be considered the same, implying that there is no obvious difference between the mantle sources of He in both the AVR and Hannuoba region in the Cenozoic Era. Therefore, 3He/4He of the mantle source in the AVR can be represented by the average (2.1×10−6) of 3He/4He (n=31) of mantle peridotite xenoliths in the Hannuoba region (Cui et al., 2022). On the assumption that the 3He/4He ratios of the crustal and upper mantle sources are 2×10−8 and 2.1×10−6, respectively, contributions of mantle-derived He to the total He of the hot spring gases were estimated in a range of 3%–23% using the ASW-corrected 3He/4He ratios and percentages of no-atmospheric He in the total He and percentages of crustal He range from 9 to 94 (Table 2). Source partitioning can be illustrated by the diagram of 3He/4He vs. 4He/20Ne (Figure 6). The percentages of mantle-derived He in the high-temperature spring gases in Figure 6 are slightly higher than the calculated values (Table 2). In consideration of the uncertainty of the different methods, however, it is clear that the calculated results are in general comparable with the diagram of 3He/4He vs. 4He/20Ne (Figure 6). He percentages of the primary mantle source in the gas samples range from 1% to 6%, estimated with the MORB value (Table 2). The bubble gas in the Tuofenglin crater lake has the highest percentage of mantle He and is composed of 68% of atmospheric He, 23% of mantle He, and 9% of crustal He. The high percentages of mantle He are found in the crater lakes (Figure 1), indicating that the mantle He migrates upwards to the surface through fractures in the channel of magma migration and mixes with atmospheric and crustal He during migration. The contribution of atmospheric He to the gases in the high-temperature hot springs in the Jinjianggou area is negligible. Gas in the low-temperature spring of no. 0 around the Hot Spring Museum (Figure 1B) contains about 3% of atmospheric He, but gas in the high-temperature hot spring on no. 34 lack of atmospheric He (Table 2; Figure 6). Such a small amount of atmospheric He may be carried into the spring by cool water recharge containing dissolved atmospheric He. Specially, the amount of mantle-derived He in the Budonghe water was estimated as high as 12%, which indicated that plenty of heat energy was transported by deep-earth fluids from the upper mantle to the Budonghe area in the Wuliqiao geothermal field. He in the hot spring gases with high concentrations is predominantly of crust origin, with less than 10% of mantle-derived He, indicating He accumulation in the geothermal reservoirs. The mantle-derived He emits upwards to the surface through the deep-cut faults and transports plenty of heat energy to the geothermal systems, which is supported by the data that about 80% of total heat flow is derived from the mantle in the study area (Sun, 1999).
Concentrations of CO2 in the hot spring gases in the AVR are less than 1%, and δ13C values are in the range of −15.9–−22.3% (Table 2). There are three scenarios for the origin of CO2 in the spring gases in the AVR. The first one is that CO2 in the hot springs is likely derived from the mantle based on the mean value of −22.6% (n = 105) of CO2 in mantle xenoliths and minerals enclosed in Cenozoic basalts in eastern China (Cui et al., 2022). The statistical result of the δ13C values of CO2 in the mantle xenoliths worldwide also shows a bimodal distribution with peak values of −5% and -25% (Deines, 2002). The second one is that the CO2 is likely to have originated from biogenic and metamorphic gases in the crust. The δ13C values of CO2 originating from Jurassic coal seams are in a range of −11%–−28% (Du and Liu, 1991). Biogenic CO2 of concentrations less than 6% in the gas reservoirs and gas seepages in China has δ13C values between −10% and −22% (Dai et al., 1996). The third one is that the CO2 is most likely a mixture of biogenic and abiogenic CO2. δ13C data (−4.7%–-6.4%) of CO2 in the fumarolic gases at the Longonot Volcano, Kenya, indicated a magmatic origin with minor contributions from biogenic CO2 (Robertson et al., 2016).
Nitrogen, in some instances, is the main component in hot spring, fumarole, and volcanic gases, which were identified as a mixture of atmospheric, mantle, and crustal N2 (Roulleau et al., 2015; Tardani et al., 2016; Zhao et al., 2021). N2 is the predominant component in the hot spring gases in the AVR, and δ15N values are in a range of +1.3–+1.9% (Table 2). The excess N2 content is larger than 92%. Combining with 4He/20Ne and 3He/4He ratios and the lack of organic matter to produce lot of metamorphic nitrogen in the study area, it can be considered that the excess N2 mainly originated from the mantle with mixing crust-derived N2, as reported by Zhao et al. (2021).
The origins of the spring water and gases in the AVR were traced by the hydro- and gas-chemical data. Contributions of deep-earth fluids to the geothermal systems were estimated using Cl− and Na+ concentrations, ASW-corrected 3He/4He ratios, and the regional mantle helium isotope ratio in consideration of the heterogeneity of the upper mantle. The conclusions are remarked as follows:
1 H2O in the river, lakes, and spring waters predominantly originate from meteoric water. The small amount (<1%) of H2O derived from deep-earth fluids seems negligible for assessing the volume of geothermal fluid, but the ion contributions of deep-earth fluids to the hot spring water are significant. Ions in the rain sample were mainly derived from sea salt and continental aerosol. Ions in the surface waters have multiple sources of the continental aerosol, sea salt, rock dissolution, and anthropogenic sources, while ions in the hot spring waters are predominantly derived from deep-earth fluids. That 73%–87% of Cl− and 86%–99% of Na+ in the hot spring waters may be derived from deep-earth fluids.
2 Enrichment of heavy isotopes in the rainwater can be attributed to isotopic fractionation caused by raindrop evaporation. Heavier isotope compositions of the waters in the crater lakes may be caused by the evaporation process due to the higher evaporation capacity in the study area.
3 Atmospheric neon dissolution in the lake waters likely approached the balance state. CH4 in the hot springs isotopically displays a biogenic origin. Excess N2, Ar, and CO2 in the hot spring gases could be predominantly derived from both the crust and upper mantle sources.
4 Contributions of the mantle-derived He to bubble gases in the hot spring were estimated in a range of 3%–23%. High percentages of mantle-derived He in the bubbling gases in the crater lakes indicate gases in the mantle emit upwards through the channel for magma migration; 12% of the total He of dissolved gas in the Budonghe water is derived from the mantle, indicating deep-earth fluids transport continuously plenty of heat to the Budonghe and the geothermal systems.
The original contributions presented in the study are included in the article/Supplementary Material; further inquiries can be directed to the corresponding authors.
YC, LL, CX, JL, ZC, and JD conducted the field survey. YC and JD processed the data and prepared the first draft. All co-authors edited the manuscript.
This work was supported by the National Key Research and Development Program (2019YFC1509203), the Open Foundation of the United Laboratory of High Pressure Physics and Earthquake Science (2019HPPES08), and the National Natural Science Foundation of China (41403099).
The authors are grateful to Ruijie Zhang in the Arxan Observatory of Volcano for his help in the field trip and Zhaofei Liu and Jianan Huang for drawing the figures. They are extremely grateful to the four reviewers for their constructive comments and patience during discussion.
The authors declare that the research was conducted in the absence of any commercial or financial relationships that could be construed as a potential conflict of interest.
The handling editor MZ declared a past co-authorship with the author YL.
All claims expressed in this article are solely those of the authors and do not necessarily represent those of their affiliated organizations, or those of the publisher, the editors, and the reviewers. Any product that may be evaluated in this article, or claim that may be made by its manufacturer, is not guaranteed or endorsed by the publisher.
Amita, K., Ohsawa, S., Du, J., and Yamada, M. (2005). Origin of Arima-type deep thermal water from hot spring wells in Oita Plain, eastern Kyushu, Japan. Hot Spring Sci. 55 (2), 64–77. (in Japanese wiith English abstract).
Bai, Z., Tian, M., and Wu, F. (2005). Yanshan, gaoshan: Two active volcanoes of the volcanic cluster in arxan, inner Mongolia. Earthq. Res. China 21 (1), 113–117. (In Chinese with English abstract).
Ballentine, C. J., Burgess, R., and Marty, B. (2002). “Tracing fluid origin, transport and interaction in the crust,” in Mineralogy and Geochemistry 47 (1), 539–614.doi:10.2138/rmg.2002.47.13
Barry, P. H., Negrete-Aranda, R., Spelz, R. M., Seltzer, A. M., Bekaert, D. V., Virrueta, C., et al. (2020). Volatile sources, sinks and pathways: A helium-carbon isotope study of baja California fluids and gases. Chem. Geol. 550, 119722. doi:10.1016/j.chemgeo.2020.119722
Benavente, O., Tassi, F., Reich, M., Aguilera, F., Capecchiacci, F., Gutiérrez, F., et al. (2016). Chemical and isotopic features of cold and thermal fluids discharged in the Southern Volcanic Zone between 32.5°S and 36°S: Insights into the physical and chemical processes controlling fluid geochemistry in geothermal systems of Central Chile. Chem. Geol. 420, 97–113. doi:10.1016/j.chemgeo.2015.11.010
Bini, G., Chiodini, G., Caliro, S., Tassi, F., Vaselli, O., Rizzo, A. L., et al. (2022). Nitrogen, helium, and argon reveal the magmatic signature of fumarole gases and episodes of outgassing from upper-crustal magma reservoirs: The case of the Nisyros caldera (Aegean Arc, Greece). Geochim. Cosmochim. Acta 335, 68–84. doi:10.1016/j.gca.2022.08.028
Chen, J., Wang, W., and Ma, F. (2021). Recharge source and Genesis analysis of Cenozoic basalt groundwater in Arshan. J. Hohai Univ. Nat. Sci.) 49 (3), 249–256. (In Chinese with English abstract). doi:10.3876/j.issn.1000-1980.2021.03.007
Chen, Z., Du, J., Zhou, X., Yi, L., Liu, L., Xie, C., et al. (2014). Hydrochemistry of the hot springs in Western Sichuan province related to the Wenchuan MS 8.0 earthquake. Sci. World J. 2014 (1), 1–13. doi:10.1155/2014/901432
Cui, Y., Sun, F., and Du, J. (2022). Methods for identification of seismic geochemical precursors and source partitioning of hot spring fluids in Eastern Chinese Mainland. J. Seismol. Res. 45 (2), 199–216. (In Chinese with English abstract). doi:10.20015/j.cnki.ISSN1000-0666.2022.0053
Dai, J., Song, Y., and Da, C. (1996). Geochemistry and accumulation of carbon dioxide gases in China. Am. Assoc. Pet. Geol. Bull. 80, 1615–1626. doi:10.1306/64EDA0D2-1724-11D7-8645000102C1865D
Deines, P. (2002). The carbon isotope geochemistry of mantle xenoliths. Earth. Sci. Rev. 58 (3-4), 247–278. doi:10.1016/S0012-8252(02)00064-8
Du, J., Amida, K., Ohsawa, S., Zhang, Y., Kang, C., and Yamada, M. (2010). Experimental evidence on imminent and short-term hydrochemical precursors for earthquake. App. Geochem. 25, 586–592. doi:10.1016/j.apgeochem.2010.01.015
Du, J., Cheng, W., Zhang, Y., Jian, C., Guan, Z., Liu, W., et al. (2006). Helium and carbon isotopic compositions of thermal springs in the earthquake zone of Sichuan, Southwestern China. J. Asian Earth Sci. 26, 533–539. doi:10.1016/j.jseaes.2004.11.006
Du, J., Li, S., Zhao, Y., Ren, J., Sun, R., and Duanmu, H. (1999). Geochemical characteristics of gases from the volcanic area Wudalianchi, Northeastern China. Acta Geol. Sin. 73 (2), 103–107. doi:10.1111/j.1755-6724.1999.tb00830.x
Du, J., Liu, C., Fu, B., Zhang, Y., Wang, C., Wang, H., et al. (2005). Variations of geothermometry and chemical-isotopic compositions of hot spring fluids in the Rehai geothermal field, southwestern China. J. Volcanol. Geotherm. Res. 142 (3-4), 243–261. doi:10.1016/j.jvolgeores.2004.11.009
Du, J., and Liu, W. (1991). Isotopic geochemistry of nonhydrocarbons in natural gas from sanshui basin, guangdong province. Chin. J. Geochem. 10 (4), 318–325. doi:10.1007/BF02841092
Fan, Q., Zhao, Y., Li, D., Wu, Y., Sui, J., and Zheng, D. (2011). Studies on Quaternary volcanism stages of Halahe river and Chaoer river area in the Great Xing’an Range: Evidence from K-Ar dating and volcanic geology features. Acta Pet. Sin. 27 (10), 2827–2832.
Fan, W., Guo, F., Wang, Y. J., and Lin, G. (2003). Late Mesozoic calc-alkaline volcanism of post-orogenic extension in the northern Da Hinggan Mountains, northeastern China. J. Volcanol. Geotherm. Res. 121 (1), 115–135. doi:10.1016/S0377-0273(02)00415-8
Gao, H., Li, C., Sun, B., Shi, X., Zhao, S., and Fan, C. (2018). Characteristics of hydrogen and oxygen stable isotopes in Lake Hulun Basin and its indicative function in evaporation. J. Lake Sci. 30 (1), 211–219. doi:10.18307/2018.0121
Giggenbach, W. F., Sano, Y., and Wakita, H. (1993). Isotopes of He, and CO2 and CH4 contents in gases produced along the New Zealand part of a convergent plate boundary. Geochim. Cosmochim. Acta 57, 3427–3455. doi:10.1016/0016-7037(93)90549-C
Gislason, S. R., and Hans, P. E. (1987). Meteoric water-basalt interactions. I: A laboratory study. Geochim. Cosmochim. Acta 51 (10), 2827–2840. doi:10.1016/0016-7037(87)90161-X
Gislason, S. R., Veblen, D. R., and Livi, K. J. T. (1993). Experimental meteoric water-basalt interactions: Characterization and interpretation of alteration products. Geochim. Cosmochim. Acta 57 (7), 1459–1471. doi:10.1016/0016-7037(93)90006-I
Graham, D. W. (2002). “Noble gas isotope geochemistry of mid-ocean ridge and ocean island basalts: Characterization of mantle source reservoirs,” in Mineralogy and Geochemistry (1), 47, 247–318.doi:10.2138/rmg.2002.47.8
Gu, X., Zhang, Q., Cui, Y., Shao, J., Xiao, Y., Zhang, P., et al. (2017). Hydrogeochemistry and genesis analysis of thermal and mineral springs in arxan, northeastern China. Water 9 (1), 61–17. doi:10.3390/w9010061
Han, Z., Shi, X., Jia, K., Sun, B., Zhao, S., and Fu, C. (2019). Determining the discharge and recharge relationships between lake and groundwater in Lake Hulun using hydrogen and oxygen isotopes and chloride ions. Water 11 (2), 264. doi:10.3390/w11020264
Hilton, D. R. (1996). The helium and carbon isotope systematics of a continental geothermal system: results from monitoring studies at Long Valley caldera (California, U.S.A.). Chem. Geol. 127, 269–295. doi:10.1016/0009-2541(95)00134-4
Jia, Z., and Zhang, G. (2020). Teleseismic tomography for imaging the upper mantle beneath northeast China. Appl. Sci. (Basel). 10 (13), 4557. doi:10.3390/app10134557
Keene, W. C., Pszenny, A. A. P., Galloway, J. N., and Hawley, M. E. (1986). Sea-salt corrections and interpretation of constituent ratios in marine precipitation. J. Geophys. Res. 91 (D6), 6647. doi:10.1029/JD091iD06p06647
Li, J. (2020). “Isotopic hydrochemical study of geothermal fluids in volcanic geothermal system,”. M.A. thesis (Xi’an, China: Chang’an University). (In Chinese with English abstract).
Li, X., Zhang, M., Ma, Q., Li, Y., Wang, S., and Wang, B. (2012). Characteristics of stable isotopes in precipitation over northeast China and its water vapor sources. J. Env. Sci. 33 (9), 2924–2931. (In Chinese with English abstract).
Li, Y., Gao, R., Yao, Y., Mi, S., Li, W., Xiong, X., et al. (2014). The crust velocity structure of Da Hinggan Ling orgenic belt and the basins on both sides. Prog. Geophys. 29 (1), 315–541. doi:10.6038/pg20140110
Li, Z., Tao, M., Li, L., Wang, Z., Du, L., and Zhang, M. (2007). Determination of isotope composition of dissolved inorganic carbon by gas chromatography-conventional isotope-ratio mass spectrometry. Chin. J. Anal. Chem. 35, 1455–1458. doi:10.1016/S1872-2040(07)60089-9
Liang, L., Li, C., Shi, X., Sun, B., Wang, J., and Zhou, J. (2017). Characteristics of hydrogen and oxygen isotopes of surface and ground water and the analysis of source of Lake water in Hulun Lake. Wetl. Sci. 15 (3), 385–390. doi:10.13248/j.cnki.wetlandsci.2017.03.010
Liu, J., Han, J., and Fyfe, W. S. (2001). Cenozoic episodic volcanism and continental rifting in northeast China and possible link to Japan Sea development as revealed from K-Ar geochronology. Tectonophysics 339, 385–401. doi:10.1016/S0040-1951(01)00132-9
Liu, J., Song, X., Yuan, G., Sun, X., and Yang, L. (2014). Stable isotopic compositions of precipitation in China. Tellus B Chem. Phys. Meteorology 66 (1), 22567. doi:10.3402/tellusb.v66.22567
Porcelli, D., Ballentine, C. J., and Wieler, R. (2002). “An overview of noble gas geochemistry and cosmochemistry,” in Noble gases in Geochemistry and cosmochemistry. Editors D. Porcelli, C. Ballentine, and R. Wieler Rev. Mineral. Geochem. (Washington DC: Mineralogical Society of America), 47, 1–19.
Qi, F., Zhang, F., Lu, S., and Sun, Y. (2012). The geothermal geological features of Arxan mountains. Jinlin Geol. 31 (2), 109–102. (In Chinese with English abstract).
Randazzo, P., Caracausi, A., Aiuppa, A., Cardellini, C., Chiodini, G., Apollaro, C., et al. (2022). Active degassing of crustal CO2 in areas of tectonic collision: A case study from the Pollino and Calabria sectors (Southern Italy). Front. Earth Sci. 10, 946707. doi:10.3389/feart.2022.946707
Rizzo, A. L., Caracausi, A., Chavagnac, V., Nomikou, P., Polymenakou, P. N., Mandalakis, M., et al. (2019). Geochemistry of CO2-rich gases venting from submarine volcanism: The Case of Kolumbo (Hellenic Volcanic Arc, Greece). Front. Earth Sci. 7, 60. doi:10.3389/feart.2019.00060
Robertson, E., Biggs, J., Edmonds, M., Clor, L., Fischer, T. P., Vye-Brown, C., et al. (2016). Diffuse degassing at Longonot volcano, Kenya: Implications for CO2 flux in continental rifts. J. Volcanol. Geotherm. Res. 327, 208–222. doi:10.1016/j.jvolgeores.2016.06.016
Roulleau, E., Vinet, N., Sano, Y., Takahata, N., Shinohara, H., Ooki, M., et al. (2015). Effect of the volcanic front migration on helium, nitrogen, argon, and carbon geochemistry of hydrothermal/magmatic fluids from Hokkaido volcanoes, Japan. Chem. Geol. 414, 42–58. doi:10.1016/j.chemgeo.2015.08.006
Rozanski, K., Araguas-Araguas, L., and Gonfiantinni, R. (1993). “Isotopic patterns in modern global precipitation,” in The climate change in continental isotopic records. Editors P. K. Swart, K. C. Lohmann, and J. McKenzie (Washington, DC, USA: American Geophysical Union), 78, 1–36. doi:10.1029/GM078p0001
Sano, Y., and Marty, B. (1995). Origin of carbon in fumarolic gas from island arcs. Chem. Geol. 119 (1-4), 265–274. doi:10.1016/0009-2541(94)00097-R
Sano, Y., Nakamura, Y., and Wakita, H. (1985). Areal distribution of 3He/4He ratios in the Tohoku district, Northeastern Japan. Chem. Geol. Isot. Geosci. Sect. 52 (1), 1–8. doi:10.1016/0168-9622(85)90004-1
Seki, K., Ohba, T., Aoyama, S., Ueno, Y., Sumino, H., Kanda, W., et al. (2019). Variations in thermal state revealed by the geochemistry of fumarolic gases and hot-spring waters of the Tateyama volcanic hydrothermal system, Japan. Bull. Volcanol. 81, 8. doi:10.1007/s00445-018-1264-7
Shangguan, Z., Kong, L., Sun, F., and Gao, S. (1996). Deep-seated fluid components and stable isotopic compositions in Tianchi volcanic area, Changbaishan Mountains. Chin. J. Geol. 31 (1), 54–64. (In Chinese with English abstract).
Shaw, A. M., Hilton, D. R., Fischer, T. P., Walker, J., and Alvarado, G. (2003). Contrasting He–C relationships in Nicaragua and Costa Rica: insights into C cycling through subduction zones. Earth Planet. Sci. Lett. 214, 499–513. doi:10.1016/S0012-821X(03)00401-1
Shen, Z., Cao, J., Arimoto, R., Zhang, R., Jie, D., Liu, S., et al. (2007). Chemical composition and source characterization of spring aerosol over Horqin sand land in northeastern China. J. Geophys. Res. 112, D14315. doi:10.1029/2006JD007991
Sokolov, D. S. (1966). Hydrogeology in U.S.S.R (Moscow: Publishing House Nedra), 1, 432. (in Russian).
Song, Y., and Frey, F. A. (1989). Geochemistry of peridotite xenoliths in basalt from Hannuoba, Eastern China: Implications for subcontinental mantle heterogeneity. Geochim. Cosmochim. Acta 53, 97–113. doi:10.1016/0016-7037(89)90276-7
Sui, J., Fan, Q., and Xu, Y. (2012). Discovery of peridotite xenoliths from the Nuomin river Quaternary volcaniv field, the Grea Xing’an Range, and its geological significance. Acta petro. Sin. 28 (4), 1130–1138.
Sun, C. (1999). “Study of geothermal structure of hot springs in Arxan,”. master's thesis (Changchun, China: Jilin University). (In Chinese with English abstract).
Sun, Y., Guo, Z., and Fortin, D. (2020). Carbon dioxide emission from monogenetic volcanoes in the Mt. Changbai volcanic field, NE China. Int. Geol. Rev. 63 (14), 1803–1820. doi:10.1080/00206814.2020.1802782
Tang, J., Wang, J., Chen, X., Zhao, G., and Zhan, Y. (2005). A Preliminary Investigation on Electric Structure of the Crust and Upper Mantle in Arxan Volcanic Area. Chin. J. Geophys. 48 (1), 214–221. doi:10.1002/cjg2.642
Tang, S. (1984). Feature of the Late Jurassic volcanic structure and genetic mechanism of hot springs in Arxan. Jilin Geol. 38(1), 54–65. (In Chinese with English abstract).
Taran, Y. A. (2009). Geochemistry of volcanic and hydrothermal fluids and volatile budget of the Kamchatka–Kuril subduction zone. Geochim. Cosmochim. Acta 73, 1067–1094. doi:10.1016/j.gca.2008.11.020
Taran, Y. A. (2011). N2, Ar, and He as a tool for discriminating sources of volcanic fluids with application to Vulcano. Bull. Volcanol. 73 (4), 395–408. doi:10.1007/s00445-011-0448-1
Tardani, D., Reich, M., Roulleau, E., Takahata, N., Sano, Y., Pérez-Flores, P., et al. (2016). Exploring the structural controls on helium, nitrogen and carbon isotope signatures in hydrothermal fluids along an intra-arc fault system. Geochim. Cosmochim. Acta 184, 193–211. doi:10.1016/j.gca.2016.04.031
Tsujimura, M., Abe, Y., Tanaka, T., Shimada, J., Higuchi, S., Yamanaka, T., et al. (2007). Stable isotopic and geochemical characteristics of groundwater in Kherlen River basin, a semi-arid region in eastern Mongolia. J. Hydrol. X. 333 (1), 47–57. doi:10.1016/j.jhydrol.2006.07.026
Wei, F., Xu, J., Shangguan, Z., Pan, B., Yu, H., Wei, W., et al. (2016). Helium and carbon isotopes in the hot springs of Changbaishan Volcano, northeastern China: A material connection between Changbaishan Volcano and the west Pacific plate? J. Volcanol. Geotherm. Res. 327, 398–406. doi:10.1016/j.jvolgeores.2016.09.005
Weiss, R. F. (1971). Solubility of helium and neon in water and seawater. J. Chem. Eng. Data 16 (2), 235–241. doi:10.1021/je60049a019
Weiss, R. F. (1970). The solubility of nitrogen, oxygen and argon in water and seawater. Deep Sea Res. Oceanogr. Abstr. 17 (4), 721–735. doi:10.1016/0011-7471(70)90037-9
Xie, H., Tian, J., Wu, L., Zhu, Y., Wu, C., Shen, K., et al. (2011). Age of ore-forming granites in Aershan area of Inner Mongolia and its significance for prospecting. J. Jilin Univ. (Earth Sci. Ed.) 41 (5), 1432–1440.
Xu, S., and Liu, C. (2002). Abundance and isotope ratios of noble gas in mantle xenoliths from northeastern China. Chin. Sci. Bull. 47 (2), 141–146. (In Chinese).
Xu, S., Zheng, G., Nakai, S., Wakita, H., Wang, X., and Guo, Z. (2013). Hydrothermal He and CO2 at Wudalianchi intra-plate volcano, NE China. J. Asian Earth Sci. 62, 526–530. doi:10.1016/j.jseaes.2012.11.001
Xue, X., Chen, L., Liu, J., He, Y., Wang, X., Zeng, G., et al. (2019). Primordial peridotitic mantle component in asthenosphere beneath Northeast China: Geochemical evidence from Cenozoic basalts of Greater Khingan Range. Earth Sci. 44 (4), 1143–1158. doi:10.3799/dqkx.2019.951
Zhang, L., Chen, Z., Nie, Z., Liu, F., Jia, Y., and Zhang, X. (2008). Correlation between δ18O in precipitation and surface air temperature on different time-scale in China. Nucl. Tech. 31(9), 715–720. (In Chinese with English abstract).
Zhang, P. (2017). “Hydrochemical characteristics and water rock interaction of Arxan Springs,”. master’s theses (Beijing, China: China University of Geosciences).
Zhang, W., Du, J., Zhou, X., and Wang, F. (2016). Mantle volatiles in spring gases in the Basin and Range Province on the west of Beijing, China: Constraints from helium and carbon isotopes. J. Volcanol. Geotherm. Res. 309, 45–52. doi:10.1016/j.jvolgeores.2015.10.024
Zhao, D. (1987). Cenozoic Basalts and Plutonic Inclusions in Eastern China, Beijing, China: Science Press, 490. (In Chinese)
Zhao, W., Guo, Z., Lei, M., Zhang, M., Ma, L., Fortin, D., et al. (2019). Volcanogenic CO2 degassing in the Songliao continental rift system, NE China. Geofluids 2019, 1–14. doi:10.1155/2019/8053579
Zhao, W., Guo, Z., Liu, J., Zhang, M., Sun, Y., Lei, M., et al. (2021). Fluxes and Genesis of carbon dioxide emissions from Cenozoic volcanic fields in NE China. Ata Pet. Sin. 37 (4), 1255–1269. doi:10.18654/1000-0569/2021.04.17
Zhao, Y. (2010). dissertation. Beijing, China: Institute of Geology, China Earthquake Administration.Study on Geology and Geochemistry of Quaternary Volcanoes in the Da Hinggan Ling Mountains,
Keywords: geothermal system, Arxan volcanic region, hot spring water, 3He/4He ratio, fluids
Citation: Cui Y, Sun F, Liu L, Xie C, Li J, Chen Z, Li Y and Du J (2023) Contribution of deep-earth fluids to the geothermal system: A case study in the Arxan volcanic region, northeastern China. Front. Earth Sci. 10:996583. doi: 10.3389/feart.2022.996583
Received: 17 July 2022; Accepted: 14 November 2022;
Published: 13 January 2023.
Edited by:
Maoliang Zhang, Tianjin University, ChinaCopyright © 2023 Cui, Sun, Liu, Xie, Li, Chen, Li and Du. This is an open-access article distributed under the terms of the Creative Commons Attribution License (CC BY). The use, distribution or reproduction in other forums is permitted, provided the original author(s) and the copyright owner(s) are credited and that the original publication in this journal is cited, in accordance with accepted academic practice. No use, distribution or reproduction is permitted which does not comply with these terms.
*Correspondence: Ying Li, c3ViZHVjdGlvbjZAaG90bWFpbC5jb20=; Jianguo Du, amlhbmd1b2R1QGhvdG1haWwuY29t
Disclaimer: All claims expressed in this article are solely those of the authors and do not necessarily represent those of their affiliated organizations, or those of the publisher, the editors and the reviewers. Any product that may be evaluated in this article or claim that may be made by its manufacturer is not guaranteed or endorsed by the publisher.
Research integrity at Frontiers
Learn more about the work of our research integrity team to safeguard the quality of each article we publish.