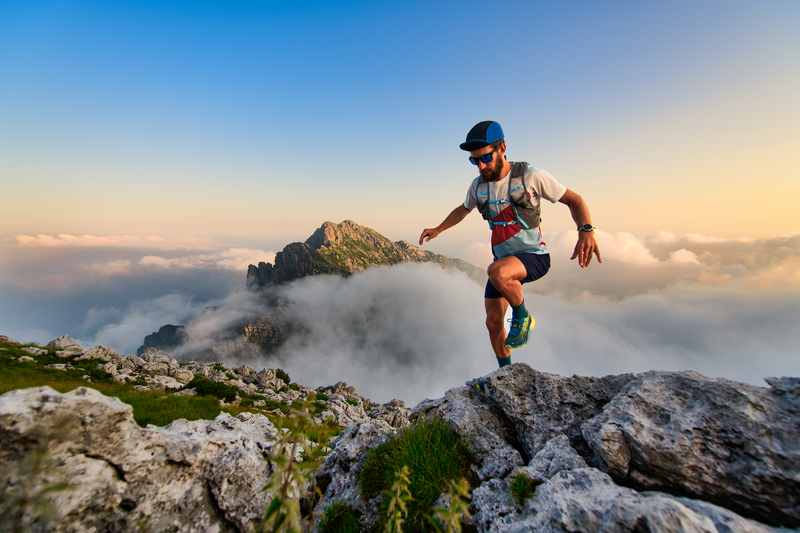
94% of researchers rate our articles as excellent or good
Learn more about the work of our research integrity team to safeguard the quality of each article we publish.
Find out more
HYPOTHESIS AND THEORY article
Front. Earth Sci. , 07 September 2022
Sec. Geochemistry
Volume 10 - 2022 | https://doi.org/10.3389/feart.2022.973645
The abundance of mantle-derived rocks and lavas, in combination with its tectonic evolution, render Mexico a perfect laboratory to investigate the chemical and the isotopic heterogeneity of the lithospheric mantle. New data on the composition of noble gases and CO2 in Mexican mantle xenoliths and lavas is reported. Our samples consist of six ultramafic nodules from the Durango Volcanic Field (DVF) and the San Quintin Volcanic Field (SQVF), monogenetic complexes belonging to the Mexican Basin and Range province; and four lavas from the Sierra Chichinautzin (SCN), a Quaternary monogenetic volcanic field located in the Mexican volcanic arc. Ne and Ar isotopes in fluid inclusions reveal mixing between atmospheric and MORB-like fluids (e.g., 40Ar/36Ar < 1,200). DVF and SQVF nodules record low 40Ar/36Ar and 4He/20Ne that confirm the existence of recycled atmospheric-derived noble gases in the local mantle. The averages of the Rc/Ra ratios (3He/4He corrected for atmospheric contamination) measured in Mexican localities are within the MORB-like range: DVF= 8.39 ± 0.24 Ra, SQVF = 7.43 ± 0.19 Ra and SCN lavas = 7.15 ± 0.33 Ra (1σ). With the aim of assessing the isotopic variability of the Mexican lithospheric mantle, the above results were compared with similar data previously obtained from ultramafic nodules found in the Ventura Espiritu Santo Volcanic Field (VESVF), another Quaternary monogenetic volcanic complex belonging the Basin and Range. The higher 3He/4He ratios in DVF relative to those reported for the VESVF and the SQVF are explained as reflecting different ages of mantle refertilization, triggered by the retreating of the Farallon slab (∼40 Ma ago) and associated delamination slab processes. We propose that the DVF mantle was refertilized more recently (<10 Ma ago) than the mantle beneath the SQVF and VESVF (∼40–20 Ma ago). On the other hand, He-Ne-Ar compositions of SCN olivines share similarities with VESVF xenoliths, suggesting a relatively homogeneous lithospheric mantle in central Mexico. Finally, DVF and the SCN samples exhibit δ13C values within the MORB range (comparable to other values previously reported in fluid inclusions and fumaroles from Popocatépetl, Colima—Ceboruco volcanoes). While we explain the MORB-like carbon signatures of the DVF samples as the result of the above-mentioned refertilization process, the SCN signatures likely reflect either (i) trapping of isotopically fractionated CO2 derived from magmatic degassing or (ii) a mantle source unaffected by subduction-related crustal carbon recycling.
The Basin and Range is an extensional province in western North America stretching more than 2,500 km from the Idaho-Montana border (United States) to central Mexico (Figure 1A; Dickinson, 2002; Henry and Aranda-Gómez, 1992); it derives its name from the distinctive morphology, characterized by elongated horst alternating with alluvial basins. It is generally accepted that the tectonic evolution of Basin and Range started 30 Ma ago (during the middle-late Oligocene) as a result of the rollback and consumption of the horizontally dipping Farallon slab beneath the North American plate (Atwater, 1989; Sedlock, 2003; Ferrari et al., 2012). The tectonic changes that resulted included: (i) the migration of the volcanic arc from the N-NW-trending Sierra Madre Occidental to the present-day east-west TMVB (30–7 Ma), (ii) the transition from subduction to a transform margin (formation of the San Andreas fault system and the Mendocino and Rivera triple junctions ≈20 Ma ago), and (iii) regional extension that produced both the Gulf of California (≈10–5 Ma ago) and the Basin and Range Province (Atwater, 1989; Severinghaus and Atwater, 1990; Sedlock et al., 1993; Ferrari et al., 1999, Ferrari et al., 2012; Nieto-Samaniego et al., 1999, Nieto-Samaniego et al., 2005; Bunge and Grand, 2000; Sedlock, 2003; Gómez-Tuena et al., 2007).
FIGURE 1. (A) The map shows the Mexican part of the Basin and Range Province. Adapted from Aranda-Gómez et al. (2000). VESVF: Ventura Espiritu Santo Volcanic Field, SDVF: Santo Domingo Volcanic Field, PiVF: Pinacate Volcanic Field, PaVF: Las Palomas Volcanic Field, Po: Potrillo maar, CVF: the Camargo Volcanic Field, DVF: Durango Volcanic Field, SQVF: San Quintin Volcanic Field, II: Isla Isabel, SCN: Sierra Chichinautzin. Contours of Sierra Madre Occidental, Sierra Madre Oriental, the Trans‐mexican Volcanic Belt and Mesa Central provinces were build based on Gómez-Tuena et al. (2007). (B) Closer view of the Trans‐mexican Volcanic Belt (TMVB) and location of some volcanoes where 3He/4He and δ13C have been reported. The dash line represents the limit of the Jalisco block located in western Mexico.
Like areas within the United States portion, the Mexican Basin and Range was accompanied by intraplate magmatism that generated several alkali basalt localities (Figure 1A; Basu, 1977; Gutmann, 1986; Aranda-Gómez and Ortega-Gutiérrez, 1987; Henry and Aranda-Gómez, 1992; Luhr and Aranda-Gomez, 1997). These volcanic fields represent a unique opportunity to study the characteristics of the local lithospheric mantle, since their deposits contain abundant mantle xenoliths (including both spinel peridotite varieties and pyroxenites; Basu, 1977; Gutmann, 1986; Aranda-Gómez and Ortega-Gutiérrez, 1987; Luhr et al., 1989; Pier et al., 1992; Luhr and Aranda-Gomez, 1997; Housh et al., 2010; Dávalos-Elizondo et al., 2016; Sandoval-Velasquez et al., 2021a); these (in view of the relatively rapid ascent of the host magma through the crust by short-lived monogenetic volcanic eruptions) are expected to retain the pristine mineralogy and geochemical/isotopic characteristics of the mantle source (Pier et al., 1989; Luhr and Aranda-Gomez, 1997; Jackson, 1998; Pearson et al., 2014).
Thus, Mexico is a geodynamically complex area characterized by the coexistence of two distinct tectonic regimes. While the central and northwestern parts of the country are affected by continental extension and intraplate magmatism in the Basin and Range Province, southern Mexico has been dominated by subduction and continental arc magmatism during the last 17 Ma in the Trans‐mexican Volcanic Belt (TMVB) (Figure 1; Henry and Aranda-Gómez, 1992; Ferrari et al., 1999; Ferrari et al., 2012; Aranda-Gómez et al., 2000; Dickinson, 2002; Sedlock, 2003; Gómez-Tuena et al., 2007).
In a previous work (Sandoval-Velasquez et al., 2021a), we investigated the isotopic composition of noble gases and CO2 in fluid inclusions (FI) trapped in mantle xenoliths collected in the Ventura Espiritu Santo Volcanic Field (VESVF; Figure 1A). These results, integrated with information on petrography, mineral chemistry and fluid inclusion compositions from the same suite of samples, revealed a complex geological evolution, in which the central Mexican upper mantle was affected by metasomatism, refertilization and recycling of atmospheric noble gases and crustal carbon derived from the Farallon slab subduction.
Here, we extend our study to mantle xenoliths and lavas collected in other monogenetic volcanic fields belonging to the Mexican Basin and Range and TMVB provinces. In detail, we analyze the composition of FI in nodules from the Durango Volcanic Field (DVF), located in the Durango State (north of the VESVF; see Figure 1A), and from the San Quintin Volcanic Field (SQVF), located in Baja California peninsula. We also present the isotopic composition of FI trapped in olivine phenocrysts from lavas erupted in the Sierra Chichinautzin Volcanic Field (SCN), a Quaternary monogenetic volcanic system located in the TMVB (Figure 1B). The objective is to study the influence of the Farallon subduction in the composition and evolution of the Mexican lithospheric mantle in terms of volatiles. Our hypothesis points to the existence of a refertilized mantle whose Ar-CO2 systematics have been modified by the introduction of air-derived noble gases and crustal materials inherited from the extinct Farallon subduction. We rely on noble gas and CO2 isotopes (coupled to petrological evidence), which are unique geodynamic tracers of the degassing history of the mantle, of the origin of Earth’s volatiles, of the relationship between different mantle components, and of the nature and signature of metasomatic fluids in the mantle (Deines, 2002; Dunai and Porcelli, 2002; Gurenko et al., 2006; Dasgupta and Hirschmann, 2010; Day and Hilton, 2011; Day and Hilton, 2020; Correale et al., 2012, Correale et al., 2015; Rizzo et al., 2018; Li et al., 2019; Sandoval-Velasquez et al., 2021a; Sandoval-Velasquez et al., 2021b; Bekaert et al., 2021).
The DVF is located in the Mesa Central province, near the eastern border of the Sierra Madre Occidental (Figure 1A). This field covers more than 2000 km2 and is composed of approximately 100 Pleistocene and Quaternary volcanic structures including maars, lava flows and cinder cones that represent at least 20 km3 of extruded magma (Albritton, 1958; Swanson, 1989; Aranda-Gómez et al., 1992; Luhr and Aranda-Gomez, 1997). In this work, we studied mantle xenoliths from the La Breña-El Jaguey maar complex, the most important xenolith locality in the DVF (Aranda-Gómez et al., 1992).
The La Breña -El Jaguey maar complex is located 45 km to the NW of Durango city (24° 25′ 04.69″N and 104° 32′ 04.86″W) and exhibits a peculiar morphology generated by the intersection of two different maars (Figure 2A): El Jaguey and La Breña. The El Jaguey is identified as a funnel-shaped maar with a diameter of 0.75 km, while La Breña is a nearly circular maar of 1.4 km in diameter (Swanson, 1989; Aranda-Gómez et al., 1992). These maars are believed to have formed from the interaction between an aquifer and a basanitic magma originated in the lithospheric mantle (Aranda-Gómez and Ortega-Gutiérrez, 1987; Henry and Aranda-Gómez, 1992; Luhr and Aranda-Gomez, 1997). No radiometric ages have been reported for these events, however, it is believed that the maar-related eruptions are Quaternary (Swanson, 1989; Aranda-Gómez et al., 1992).
FIGURE 2. (A) Image from Google Earth (23 June 2021) showing La Breña-El Jagüey maar complex. (B) Image from Google Earth (23 June 2021) showing the San Quintin Volcanic Field (SQVF) and sampling area. Media Luna (L), Woodford (W), Basu (B), Riveroll (R), Kenton (K), Picacho Vizcaíno (P), Sudoeste (S), Ceniza (C), Monte Mazo (M) and Isla San Martin (I). (C) Image from Google Earth (23 June 2021) showing the Sierra Chichinautzin (SCN) and sampling areas. (D) Mexico overview map including the SQVF, DVF, VESVF, and SCN localities.
Unlike the VESVF (see Sandoval-Velasquez et al., 2021a) and the DVF, the SQVF is situated in northwestern Mexico, in the Baja California state near the Mexico-USA border (Figure 1). The SQVF is the only mantle xenolith-bearing alkaline basalt complex identified in the Baja California Peninsula, and formed as consequence of opening of the Gulf of California (at 3.5 Ma) after subduction of the Farallon plate terminated at 12 Ma (Aranda-Gómez and Ortega-Gutiérrez, 1987; Luhr et al., 1995a; Luhr and Aranda-Gomez, 1997; Sedlock, 2003). Geochemical signatures of SQVF magmas, and the presence of mantle xenoliths, support similarity with deformation and tectonic style of the Basin and Range extensional province (Aranda-Gómez and Ortega-Gutiérrez, 1987; Luhr et al., 1989; Pier et al., 1989; Luhr et al., 1995a).
Volcanic activity in the SQVF started during the Pleistocene and continued through the Holocene (Luhr et al., 1995a; Luhr and Aranda-Gomez, 1997); some peperites and pillow lavas have also been identified in the volcanic field suggesting that the SQVF initiated as a group of subaqueous volcanoes that later emerged (Surtseyan-style activity; Németh and Kósik, 2020). The SQVF consists of 10 different volcanic complexes; each complex is characterized by a well-preserved scoria cone, several eruptive vents and lava flows (Luhr et al., 1995a; Luhr and Aranda-Gomez, 1997). These volcanic complexes are (Figures 2B,D): the Media Luna (L), Woodford (W), Basu (B), Riveroll (R), Kenton (K), Picacho Vizcaíno (P), Sudoeste (S), Ceniza (C), Monte Mazo (M) and Isla San Martin (I). Mantle xenoliths analyzed in this work come from the Media Luna cone.
The SCN is a Quaternary monogenetic volcanic field located along the Trans-Mexican Volcanic Belt (TMVB) front, and in between two Quaternary stratovolcanoes: Popocatepetl and Nevado de Toluca (Figures 1B, 2C; Márquez et al., 1999a; Márquez et al., 1999b; Schaaf et al., 2005; Meriggi et al., 2008). The SCN has been active over the last 40 ka producing more than 200 volcanic monogenetic structures (including several scoria cones, lava flows, lava domes, shield volcanoes and hydromagmatic craters) and approximately 470 km3 of extruded magma (Márquez et al., 1999a, Márquez et al., 1999b; Schaaf et al., 2005; Meriggi et al., 2008). Erupted rocks include basalts, basaltic andesites, andesites and dacites (Márquez et al., 1999b; Meriggi et al., 2008). The origin of the SCN is still controversial and different hypotheses have been set out in the literature. One model suggests that the calc-alkaline affinity and the LILE-Pb enrichments (identified in evolved rocks) are the result of partial melting of a subduction-related metasomatized mantle wedge (Meriggi et al., 2008; Straub et al., 2011; Straub et al., 2013; Straub et al., 2014; Straub et al., 2015). Other studies (Márquez et al., 1999a; Márquez et al., 1999b) argue that the above hypothesis is inconsistent with the presence of OIB-type (high-Nb) mafic rocks in the SCN; therefore, they propose an extensional origin for the monogenetic SCN. These authors suggest that the OIB geochemical affinity of the SCN basalts is a result of partial melting of a mantle not affected by subduction, and that the calc-alkaline affinity of the most evolved rocks derives from magma mixing between basaltic magmas and crustal-derived felsic magmas (Márquez et al., 1999a; Márquez et al., 1999b).
According to Luhr and Aranda-Gomez (1997), mantle xenoliths from the DVF are dominated by coarse-tabular and protogranular textures and are characterized by the presence of clusters of spinel (Sp), orthopyroxene (Opx) and clinopyroxene (Cpx) (possibly originated from the decompressive reaction of olivine and garnet). Olivine (Ol) and Opx crystals from DVF samples usually have diameters >2 mm, while Cpx and Sp exhibit variable diameters between 1—2 mm. SQVF xenoliths generally show strongly deformed fabrics with a bimodal grain-size distribution (porphyroclastic texture); samples may exhibit porphyroclasts of Ol and Opx with diameters greater than 5 mm surrounded by recrystallized neoblasts, with sizes <1 mm, of Ol, Opx, Cpx, and Sp. A common characteristic of DVF and SQVF xenoliths is the presence of interstitial glass veins and spongy rims in Cpx. Interstitial glasses have elevated SiO2 (54–57 wt%), Al2O3 (18–21 wt%), Na2O (2.5–6.1 wt%), K2O (1.5–5.7 wt%), P2O5 (0.06–0.81 wt%), and are MgO-depleted (2.5–3.6 wt%). Spongy rims in Cpx show elevated Mg#, are enriched in CaO and depleted in Al2O3 and Na2O, as observed in VESVF xenoliths. Luhr and Aranda-Gomez (1997) proposed that both glasses and spongy rims may be the result of either of two different processes: 1) partial melting or 2) the infiltration of volatile-rich melts reacting with the lithospheric mantle. The above authors also report pressures of 17.7 ± 1.7 kbar and fO2 values (ΔFMQ; where FMQ is the fayalite-magnetite-quartz buffer) of −0.2 ± 0.3 for the DVF xenoliths and pressures around 10.7 ± 2.5 kbar and fO2 (ΔFMQ) of 0.0 ± 0.2 for the SQVF samples. Luhr and Aranda-Gomez (1997) argued that the systemic increase of mantle xenolith’s oxygen fugacity from DVF to SQVF derives from subduction-related oxidation of the lithospheric mantle, due to the release of fluids from the subducted Farallon slab beneath the North American plate.
Petrological and isotopic results (Sr-Pb-Nd-He) for SCN lavas have proven that both andesites and basalts directly derive from the local mantle. According to Straub et al. (2008), Straub et al. (2011), these rocks show common geochemical characteristics that suggest a similar genetic mechanism. Andesites and basalts are consistently enriched in Ni and MgO (with melt Mg#∼ 70), they show similar ratios of trace elements (e.g., Sr/Y and Gd/Yb), and contain Ni-rich olivines with MORB-like 3He/4He ratios. Schaaf et al. (2005) and Straub et al. (2011) propose that the occurrence of high-Mg calc-alkaline and alkaline primitive magmas in the SCN suggest that magmas accumulate in small and short-lived magmatic systems with negligible crustal contamination. Moreover, using tracers such as 3He/4He and δ18O, Straub et al. (2022) demonstrated that olivine phenocrysts grow into mantle melts not contaminated by crustal materials. The opposite occurs for Popocatepetl lavas since generally reflect long crustal residence in shallow magma chambers and assimilation of Cretaceous limestones (Schaaf et al., 2005; Witter et al., 2005; Mangler et al., 2019).
Straub et al. (2008), Straub et al. (2011) argue that both andesites and basalts originated from partial melting of peridotites and coexisting silica-deficient and quartz-normative pyroxenites (located below the Moho) that were produced by the infiltration of silicic slab melts in the local mantle. After melting, a broad spectrum of high-Mg# basaltic, andesitic and dacitic melts were generated; during their ascent through the crust, these eventually undergo differentiation by fractional crystallization and recharge melt mixing in the overlying crust (just before the eruption), leading to Mg#<65 lavas.
Six peridotites (1 lherzolite from the SQVF and 4 lherzolites and 1 harzburgite from the DVF) and 4 lava samples (from the SCN) were analyzed in this study. Peridotites from the SQVF and the DVF were provided by the Department of Mineral Sciences of the Smithsonian Institution. SQVF sample was originally collected in the Media Luna cone, while DVF samples come from the La Breña -El Jaguey maar complex. SCN lavas are two calc-alkaline basaltic andesites (samples CH09-1 and CH09-9) and two high-Nb arc basalts (samples CH05-16 and MCH06-5). Sample CH05-16 was collected from the Texcal Flow, samples CH09-1and CH09-9 were collected in the Guespalapa volcano, while sample MCH-06-5 was obtained from the Chichinautzin volcano (see Table 1 and Figure 2 for coordinates).
Mantle xenoliths and lavas were crushed for noble gas and CO2 isotopic determinations following the same methods and analytical procedures described in Rizzo et al. (2018), Rizzo et al. (2021), Sandoval-Velasquez et al. (2021a), and Sandoval-Velasquez et al. (2021b). After crushing, samples were sieved with the aim of hand-picking crystals (with diameters >0.25 mm) of Ol, Opx, and Cpx from mantle xenoliths and only Ol crystals from lavas. Aliquots of crystals (weights of 0.5–2 g) were properly cleaned ultrasonically, accurately weighed and loaded into an ultra-high-vacuum (UHV) crusher for noble gas analyses. FI were released by single-step crushing at about 200 bar and room temperature (21°C). The gas mixture was purified in a stainless-steel ultra-high-vacuum preparation line to remove all gas species (N2, H2, H2O, and CO2) except noble gases. The first estimation of CO2 concentrations in FI was performed for each sample (manometric determination). He and Ne isotopes were analyzed using two different split-flight-tube mass spectrometers (Helix SFT-Thermo), while Ar isotopes were analyzed by a multi-collector mass spectrometer (Argus, GVI); typical blanks for He, Ne, and Ar were <10−15, <10−16, and <10−14 mol, respectively.
The measured 3He/4He ratios were corrected for atmospheric contamination based on the measured 4He/20Ne ratio and the values are expressed as Rc/Ra:
where RM/Ra and (He/Ne)M are the measured values and (He/Ne)A refers to the atmospheric value (0.318; Ozima and Podosek, 2002). 40Ar and 4He/20Ne values were also corrected for atmospheric contamination:
where 40Ar∗ is the corrected 40Ar, 40Ar/36Arair = 298.56 (Lee et al., 2006), βNe and βHe are the Bunsen solubility coefficients for Ne and He, respectively (IUPAC, 1979; Harðardóttir et al., 2018). Abundances of 20Ne, 21Ne, 22Ne, 36Ar, and 38Ar are reported. Analytical uncertainties (1σ) for 3He/4He, 20Ne/22Ne, 21Ne/22Ne and 40Ar/36Ar ratios are <4.4%, <1.0%, <6.8% and <2.2%, respectively. The 20Ne/22Ne and 21Ne/22Ne ratios were properly corrected (please see Rizzo et al., 2018 and Sandoval-Velasquez et al., 2021a).
The aliquots with the highest concentrations of CO2 (n = 6) were selected to determine the carbon isotopic composition of FI (13C/12C). Samples were properly cleaned, weighed and loaded in a crusher system consisting of a stainless-steel sample holder, a hydraulic crusher a glass sampler to freeze CO2, and a pump to ensure the vacuum inside the system. The gas mixture trapped in the FI was released and purified in a glass line equipped with a 626B Baratron® Absolute Capacitance Manometer MKS (measuring range 10−3−10−4 mbar), for the purification procedure and quantification of CO2 concentration (mol/g). The 13C/12C ratios were measured using a Thermo (Finnigan) Delta Plus XP CF-IRMS connected to a Trace GC gas chromatograph and a Thermo (Finnigan) GC/C III interface. Results are expressed in parts per thousand (‰; relative to the V-PDB international standard) using the delta notation (δ13C). The analytical error estimated as 1σ < 0.6‰. Detailed information about the methodology can be found in Gennaro et al. (2017), Rizzo et al. (2018) and Sandoval-Velasquez et al. (2021b).
The composition of FI trapped in mantle xenoliths and lavas is reported in Table 2 and Figure 3. Errors are reported as 1σ uncertainties. CO2 is the dominant volatile species in the FI; its concentration ranges from 8.33 × 10−11 to 1.54 × 10−8 mol/g. Samples from the DVF generally exhibit higher gas (4He, 40Ar*, and CO2) concentrations than those from SQVF. In general, 3He and 4He concentrations vary from 1.38 × 10−19 to 1.32 × 10−17 mol/g and 1.96 × 10−14 to 1.51 × 10−12 mol/g, respectively. 40Ar* (Ar content corrected for the atmospheric contamination) ranges from 3.02 × 10−14 to 2.17 × 10−12 mol/g. Rc/Ra values (3He/4He corrected for the atmospheric contamination) vary from 5.10 to 8.40 with the lowest values being observed in pyroxenes from SQVF (Figure 4). On average, the Rc/Ra ratios obtained in DVF xenoliths are higher than the ratios observed in the SQVF nodules. Pyroxenes generally show lower 3He/4He ratios than olivines, except for sample NMNH-37 Cpx that exhibits the highest ratio of the dataset (8.40 Ra; Table 2 and Figure 4A). Xenoliths from both localities do not exhibit systematic differences in air-normalized 4He/20Ne (X values) and 4He/40Ar*ratios (Figure 4B), which range from 36.0 to 645.1 and from 0.1 to 2.5, respectively. 40Ar/36Ar ratios measured in all samples are higher than in atmosphere (298.56), but the maximum value (1,031) is much lower than in Mid-ocean Ridge Basalts (MORB, ∼44,000; Moreira et al., 1998). 20Ne/21Ne and 21Ne/22Ne values vary from 9.80 ± 0.01 (1σ) to 12.57 ± 0.10 (1σ) and from 0.0290 ± 0.0003 (1σ) to 0.056 ± 0.0024 (1σ), respectively. The carbon isotopic composition of CO2 (δ13C values) reported for the DVF samples vary between −5.43‰ and −3.80‰.
TABLE 2. Fluid inclusion compositions from DVF—SQVF mantle xenoliths and SCN olivine-related lavas. Concentrations of noble gases isotopes and CO2 are reported in mol/g.
FIGURE 3. 4He vs (A) 40Ar∗ and (B) CO2 abundances measured in mantle xenoliths from the DVF- SQVF and in olivines from the SCN. VESVF values are from Sandoval-Velasquez et al. (2021a). Compositional fields for the Hyblean plateau, SW Poland and West Eifel were designed after Correale et al. (2015), Gennaro et al. (2017), Rizzo et al. (2018), Rizzo et al. (2021).
FIGURE 4. (A) 4He and (B) 4He/40Ar* vs. 3He/4He ratios corrected for atmospheric contamination (Rc/Ra). MORB range is reported at Rc/Ra = 8 ± 1 (Graham, 2002) and 4He/40Ar* from 1 to 5 (Yamamoto et al., 2009). Numbers represent the last two digits of the “Samples ID” reported in Table 2 for the DVF xenoliths. The dotted lines represent diffusive fractionation paths for helium, these were designed using the diffusion coefficient (D) of 3He, 4He and 40Ar for the solid mantle (D3He/D4He = 1.15 and D4He/D40Ar = 3.16; Burnard, 2004; Yamamoto et al., 2009) and a starting mantle composition of 3He = 1.56 × 10−17 mol/g, 4He =1.5 × 10−12 mol/g, 40Ar* = 6.0 × 10−13 mol/g, 4He/40Ar* = 2.5 (the average mantle production ratio) and a 3He/4He ratio =7.5 Ra. Further details about the model are provided in Sandoval-Velasquez et al. (2021a).
In SCN phenocrysts, FI are dominated by CO2 and show the highest concentrations in the dataset (1.17 × 10−8 to 1.29 × 10−6 mol/g; Figure 3B). Helium concentrations are also high compared to those in mantle xenoliths: 3He and 4He contents vary from 9.98 × 10−18 to 3.94 × 10−16 and from 1.00 × 10−12 to 3.99 × 10−11 mol/g, respectively. Rc/Ra (7.15 ± 0.14 Ra) and 4He/40Ar* ratios (ranging between 3.68 and 8.71) are within the MORB range with 4He/40Ar* ratios being higher than those observed in Mexican xenoliths. In general, samples exhibit 4He/20Ne >10,000 (X values) and 40Ar/36Ar >650 (except for sample MCH06-5) and δ13C values between −6.31 and −5.00‰.
The petrology and geochemistry of the mantle xenoliths studied here have previously been interpreted (Luhr and Aranda-Gomez, 1997) as evidence of a complex evolution of the Mexican Basin and Range source mantle, in which episodes of partial melting and/or metasomatism have occurred. As shown in Figure 5, most inclusions-hosting crystals have Mg# values well above the typical fertile mantle range (88.5–89.5), indicating a residual nature of the mantle source, e.g., that the mantle has suffered extensive partial melting.
FIGURE 5. Mg# vs. (A) Rc/Ra (3He/4He ratio corrected for atmospheric contamination) and vs. (B) 4He/40Ar*. Mg# values were obtained from Luhr and Aranda-Gomez (1997).
Partial mantle melting can impact the mantle noble gas signature (recorded in FI) in many different ways. Firstly, noble gases have different affinities for crystal and melt. Indeed, the olivine/melt distribution coefficients of Ar and He are min/meltDAr = 0.0011 ± 0.0006 and min/meltDHe = 0.00017 ± 0.00013, while for the cpx/melt system are min/meltDAr = 0.0011 ± 0.0007 and min/meltDHe = 0.0002 ± 0.0002 (Heber et al., 2007). The more incompatible (lower mineral/melt distribution coefficients) nature of He (relative to Ar) implies that He can more effectively escape from the mantle during partial melting, causing a decrease of the 4He/40Ar* of the mantle residuum (Burnard, 2004; Heber et al., 2007; Yamamoto et al., 2009). The 4He/40Ar* population of our samples supports the partial melting hypothesis (Figure 5A). While Ol crystals (having more refractory behavior during melting) exhibit 4He/40Ar* ratios well within the mantle production range, four out of five pyroxenes exhibit decreasing 4He/40Ar* ratios at increasing Mg# (Figure 5A).
Secondly, partial melting can also impact the helium isotopic signature by triggering diffusive fractionation processes (Burnard, 2004; Yamamoto et al., 2009). Noble gases can diffuse in minerals during the formation of melt-filled dissolution channels in the mantle as a result of partial melting or metasomatic episodes. This process, mainly affecting spinel and pyroxenes (Burnard, 2004; Yamamoto et al., 2009; Faccini et al., 2020), leads to isotopic fractionation because of the mass dependency of diffusion, which causes the lighter 3He to diffuse faster than 4He (D3He/D4He =1.15; Trull and Kurz, 1993; Burnard, 2004; Yamamoto et al., 2009). Similarly, 4He will escape faster than 40Ar* (D4He/D40Ar = 3.16; Burnard 2004; Yamamoto et al., 2009). Diffusive fractionation therefore ultimately results in He-depleted, lower 3He/4He - 4He/40Ar*compositions in the mantle/magma residuum. Again, four out of six pyroxenes in our sample suite exhibit lower 3He/4He ratios than the MORB-like upper mantle range (Figure 5B); samples from the SQVF (red symbols) exhibit an especially evident decrease in 3He/4He accompanied by a depletion in 4He concentrations and 4He/40Ar* (Figures 4A,B) that point to diffusive fractionation. We modeled this process using the methodology of Sandoval-Velasquez et al. (2021a), and find that the fractionation trend (see dashed red lines in Figure 4) reproduces well the He-poor, low 3He/4He signature SQVF pyroxenes, and of Cpx from nodules NMNH-20, and NMNH-18. It is also very possible that diffusive fractionation may have (at least partly) impacted the composition of samples NMNH-36 Cpx and NMNH-39 Opx/Cpx (Figure 4) as observed in some VESVF samples (Sandoval-Velasquez et al., 2021a).
Ultimately, we argue that melting-triggered diffusive fractionation is likely to have altered the pristine (pre-melting) He isotopic signature of samples NMNH-20 Opx/Cpx, NMNH-18 Cpx, NMNH—27 Opx/Cpx, and to a minor extent NMNH-36 Cpx and NMNH-39 Opx/Cpx. These samples are not considered further in the following sections.
Neon and argon isotopes measured in mantle rocks generally reflect mixing between mantle and atmospheric fluids that suggests a contamination of FI by air-derived noble gases (Farley and Poreda, 1993; Matsumoto et al., 2001; Graham, 2002; Ozima and Podosek, 2002; Hopp et al., 2007a; Martelli et al., 2011; Rizzo et al., 2018; Rizzo et al., 2021). While atmospheric contamination of FI can occur during eruption of the transporting magmas, or during exposure of mantle xenoliths to the surface, the recycling of atmospheric gases into the local mantle has also been proposed. Holland and Ballentine (2006) demonstrate that significant quantities of seawater-derived noble gases are continuously introduced into the convective mantle during subduction. Besides, Hopp et al. (2007a), Matsumoto et al. (2002), Rizzo et al. (2018), Rizzo et al. (2021), and Sandoval-Velasquez et al. (2021b) suggest the existence of recycled atmospheric fluids in the Subcontinetal Lithospheric mantle (SCLM; even far from present-day subduction zones) based on analysis of 3He/36Ar, 4He/20Ne, 20Ne/22Ne, 21Ne/22Ne, 36Ar/22Ne and 40Ar/36Ar ratios in FI trapped in ultramafic xenoliths. These studies prove the long-term preservation of air-derived noble gases in the SCLM located beneath intraplate settings, and invoke the effect of paleo-subduction events to explain the presence of these recycled atmospheric components in the mantle.
Our air-normalized 4He/20Ne (X values) vs. R/Ra (3He/4He ratio not corrected for atmospheric contamination) and 3He/36Ar vs. 40Ar/36Ar diagrams (Figures 6A,B) clearly support mixing between mantle and atmospheric fluids. When compared with the VESVF xenoliths, DVF and SQVF nodules show lower 4He/20Ne and 40Ar/36Ar ratios; on average, DVF xenoliths exhibit 4He/20Ne of 172.9 ± 149.4 and 40Ar/36Ar of 462.5 ± 128.5 (1σ); while the VESVF xenoliths show 4He/20Ne of 4,324.9 ± 6,812.9 and 40Ar/36Ar of 1962.5 ± 1958.5 (1σ). Besides, as in the case of the VESVF xenoliths (Sandoval-Velasquez et al., 2021a), 3He and 36Ar abundances are correlated (Figure 6C), supporting the hypothesis that the atmospheric component identified in DVF and SQVF nodules has been trapped at mantle conditions (e.g., Matsumoto et al., 2001).
FIGURE 6. (A) X (air-normalized 4He/20Ne) vs. R/Ra diagram, the dotted lines represent the binary mixing between air and an upper mantle source with R/Ra values between 6.5 and 8.5. Onto the lower-right portion of the image there is a closer view of our data; this image was designed using the same y axis of Figure 4 for comparison purposes. (B) 3He/40Ar vs. 40Ar/36Ar diagram. Dotted and solid lines represent binary mixing between air and an upper mantle source with 40Ar/36Ar of 298.56 and 44,000, respectively. The endmember 4He/40Ar* ratios define the curvature of the mixing hyperbola. SCN olivines fit the mixing line defined by a 4He/40Ar* ∼3 which is expected considering the magmatic nature of SCN lavas. (C) 36Ar vs. 3He concentrations.
Sandoval-Velasquez et al. (2021a) previously inferred the isotopic characteristics of the Mexican SCLM by applying the approach developed by Langmuir et al. (1978) and Hopp et al. (2007a) to the VESVF mantle nodules. Using this methodology, they estimated a40Ar/36Ar of ∼10,500 for the Mexican SCLM (with 4He/20Ne ratios <40,000) (Figure 6A). We find (Figure 7) that the SQVF and DVF FI fit the same model previously presented for the VESVF, therefore suggesting that the deep recycled atmospheric component is a regional (and not local) feature. We propose this regional atmospheric component to arise from subduction-related contamination of the Mexican SCLM during the eastward descent of the Farallon slab beneath the North American plate (∼70—11 Ma ago), driven by the release of slab derived oxidized hydrous fluids or water-bearing silicate melts as proposed for present-day subduction zones (Wood et al., 1990; Luhr and Aranda-Gomez, 1997; Parkinson and Arculus, 1999; Kelley and Cottrell, 2009).
FIGURE 7. 20Ne/22Ne vs. 40Ar/36Ar. Mixing curves are the result of mass-balance and isotopic mass balance equations using the parameters reported in Section 6.1.2. Modified from Sandoval-Velasquez et al. (2021a).
The average 3He/4He ratio of the lithospheric mantle beneath the DVF is 8.39 ± 0.24 Ra (1σ). In the case of the SQVF, only the olivine aliquot is representative of the local mantle, and has a 3He/4He ratio = 7.39 ± 0.20 Ra (Figure 4A). Both values fall within the MORB-like upper mantle range, which excludes the influence of a lower mantle component (plume hypothesis) beneath these localities (Rc/Ra values above nine are commonly associated to mantle plume localities such as Hawaii, Iceland, the East African Rift or the Canary Islands; Vance et al., 1989; McMurtry et al., 2019; Day and Hilton, 2020; Halldórsson et al., 2022). These results corroborate the hypothesis that the Quaternary monogenetic volcanism of the DVF and the SQVF was produced by continental extension.
The estimated 3He/4He average composition (8.39 ± 0.24 Ra) for DVF samples is less radiogenic than seen at other Basin and Range mantle xenolith-bearing localities, including VESVF (Figure 4A). Sandoval-Velasquez et al. (2021a) invoked a role of the subducted Farallon plate to explain the 3He/4He signature of VESVF xenoliths. In their hypothesis, the retreating of the Farallon slab (∼40 Ma ago) triggered the refertilization of the lithospheric mantle through the injection of 3He-rich asthenospheric melts (Lee, 2005; Nieto-Samaniego et al., 2005). Because of the relative proximity between the DVF and the VESVF, a similar geodynamic processes is expected (Figure 8). If we consider the tectonic configuration of the western margin of North America at the time of the Laramide Orogeny (74–40 Ma), in which the Farallon plate acted as a natural barrier between the lithospheric mantle and the asthenosphere (Figure 8B), it is reasonable to suppose that during the subsequent rollback and retreating, the VESVF mantle was exposed to, and refertilized by, the hot asthenosphere first (40–20 Ma ago Figure 8C); since then, the VESVF lithospheric mantle would have evolved in steady-state (Gautheron and Moreira, 2002), gradually becoming more radiogenic (due to 4He production by decay of U-Th isotopes) during the last 20 Ma (starting from a 3He/4He ratio ∼8.5 Ra, which is close to the highest ratio reported in our Mexican mantle xenoliths). As the retreating process continued, the mantle beneath the DVF would have been refertilized at a later stage, which might explain the higher 3He/4He ratios observed there. These arguments imply a shorter residence time (Rt < 20 Ma) of helium (less production of radiogenic 4He) for the DVF subcontinental mantle when compared with the VESVF results (Sandoval-Velasquez et al., 2021a; Figures 8D,E).
FIGURE 8. Evolution of the western margin of the North American plate during the Late Mesozoic and Cenozoic. (A) Subduction of the Farallon slab beneath North America during the Mesozoic. (B) Shallowing of the subduction angle and tectonic uplift of the Sierra Madre Oriental (SMOr; Laramide Orogeny). (C) Rollback and first break-off of the Farallon slab causing the introduction of asthenospheric fluids in the lithospheric mantle beneath the SQVF and VESVF. This process caused extensional deformation in the Basin and Range Province, refertilization of the lithospheric mantle and the second ignimbrite flare-up of the Sierra Madre Occidental (SMO). (D) Over time, the subduction angle increased and ceased triggering a second break-off episode and the subsequent proto-Gulf extension. Second refertilization event beneath SQVF? (E) The lithospheric mantle beneath the DVF is finally refertilized. Subduction ends in the western margin of Mexico and the SCLM beneath SQVF, DVF, and VESVF evolves in steady-state becoming more radiogenic over time. The Basin and Range extension facilitated the formation of mantle xenolith-bearing alkali basalt localities (e.g., the VESVF, SQVF, and DVF) in the Mesa Central and the Baja California Peninsula. Adapted from Lee (2005).
The above hypothesis can be tested estimating the refertilization age of the DVF mantle following the formula proposed by Ballentine and Burnard (2002) for the 4He production rate:
where [U] and [Th] are Uranium and Thorium concentrations in ppm. Assuming a U concentration between 0.01 and 0.03 ppm (and a Th/U = 3; Gautheron and Moreira, 2002) for the Mexican mantle xenoliths (Dávalos-Elizondo et al., 2016), it is possible to estimate a 4He production rate between 9.23 × 10−20 and 2.77 × 10−19 mol/g*yr. Considering a steady-state model (same as proposed for the VESVF; Sandoval-Velasquez et al., 2021a) for the lithospheric mantle beneath the DVF, the above-estimated 4He production rate, a starting 3He/4He =8.5 Ra, and 4He concentration = 4.5 × 10−11 mol/g (the maximum value identified for continental mantle xenoliths; Gautheron and Moreira, 2002), we estimate that a residence time (Rt) of 4 to 10Ma is needed to decrease the helium ratio from 8.5 to 8.39 Ra (the average 3He/4He values measured in DVF xenoliths). In other words, these ages support the idea that the DVF mantle was more recently refertilized than the mantle located beneath the VESVF, where a Rt of 40–20 Ma was estimated previously (Sandoval-Velasquez et al., 2021a). This would explain the higher 3He/4He ratios observed in Ol and Opx crystals from DVF xenoliths.
The SQVF 3He/4He ratio signature falls within the VESVF mantle range. Considering the steady-state model previously discussed, the most plausible explanation for the similarity in helium isotope compositions between SQVF and VESVF is that the mantle beneath both localities was refertilized at similar ages. In the case of a normal slab rollback, mantle refertilization should have in principle proceeded from east to west; therefore, in this scenario, the SQVF mantle should have been the last mantle portion to be refertilized by the asthenospheric melts. If this was the case, however, the 3He/4He signature of the SQVF mantle should be at least equal to (or even higher than) the DVF average (8.5Ra), which is opposite to what we observe.
To explain this low 3He/4He signature of SQVF, we turn our attention into the possible role played by the Farallon slab break-off (Figure 8C). According to Ferrari et al. (2012), the old Mexican magmatic arc i.e., the Sierra Madre Occidental (SMO; Figure 8B; Ferrari et al., 1999; Ferrari et al., 2002; Nieto-Samaniego et al., 1999), was generated in two different episodes of ignimbrite flare-up: the first occurred in the Oligocene (31.5–28 Ma) and the second during the Miocene (23.5–20 Ma). Ferrari et al. (2002) link the second episode of volcanism to a detachment (or break-off) of the Farallon slab that also promoted an extensional deformation in southern Baja California and central Mexico before the opening of the Gulf of California. During this break-off (Figure 8C; 24-20 Ma; Ferrari et al., 2002) the cold lithosphere would have been replaced by the hotter asthenosphere which abruptly increased the temperature at the base of the crust triggering the refertilization of the lithospheric mantle and the generation of silicic crustal magmas via mafic underplating. Subsequently, a second slab break-off would have occurred in the middle Miocene (∼14–11 Ma; Figure 8D) inducing a new extensional phase that probably triggered a new intrusion of the asthenosphere in the SCLM, the proto-Gulf extension, the partial melting of the mantle right below the present-day Gulf of California and the eruption of mafic melts that characterized the late Miocene mafic volcanism in the eastern side of the Gulf (Henry and Aranda-Gomez, 2000; Ferrari et al., 2002).
Ultimately, these break-off events may explain well why the SQVF mantle was refertilized synchronously with the VESVF and much earlier than DVF mantle (Figures 8C,D), ultimately justifying its relatively radiogenic He signature.
The lavas from SCN studied in this work have relatively high Mg# (>60) and 3He/4He ratios within the MORB-like upper mantle range (Figure 4), so it is reasonable to assume that the FI hosted in our olivine separates are (at least to some extent) representative of the fluids present in the SCN sub-arc mantle, as petrological observations suggest (Mg# ∼70, MgO between 7.15 and 9.60 wt% and high Ni >100 ppm; Straub et al., 2011). A comparison with data obtained for the VESVF, DVF, and SQVF xenoliths is therefore justified.
Olivines from the calc-alkaline basaltic andesites (samples CH09-1 and CH09-9) exhibit the highest He and CO2 concentrations in our Mexican dataset (4He = 2.46 × 10−11—CO2 =7.17 × 10−7 mol/g and 4He = 3.99 × 10−11—CO2 = 1.29x10−6 mol/g, respectively; Table 2 and Figure 3); samples CH05-16 and MCH06-5 (high-Nb basalts) exhibit higher concentrations (4He = 7.26 × 10−12—CO2 = 1.60 × 10−7 mol/g and 4He = 1.00 × 10−12 mol/g—CO2 = 1.17 × 10−8 mol/g, respectively) than in DVF and the SQVF nodules and comparable contents relative to the VESVF mantle xenoliths. The fact that SCN olivines exhibit gas concentrations comparable to, or even higher than, the VESVF nodules indicate a volatile-rich parental melt or entrapment depth of FI higher than in the mantle xenoliths. We believe that the hypothesis of a deeper entrapment of FI is unrealistic since relatively low Mg# that ranges from 60 to 70 and high 4He/40Ar* ratios >3 confirm the magmatic nature of our olivines. Despite the differences in gas contents observed in CH09-1 and CH09-9, all SCN samples exhibit regular Rc/Ra values comparable to the xenoliths from VESVF (on average 7.24 ± 0.33 Ra; 1σ) (Figure 4), suggesting that the olivines derive from an upper mantle with a similar signature.
Both basaltic andesites and alkaline basalts show higher 4He/40Ar* ratios than those observed in mantle xenoliths (Figure 4B), consistent with the magmatic nature of fluids entrapped in the olivine-phenocryst; during degassing of silicate melts, preferential early removal of Ar (which is less soluble than He during melt degassing processes) can lead to later trapping of fluids with high 4He/40Ar*ratios (Jambon et al., 1985; Burnard et al., 1997; Moreira and Sarda, 2000; Paonita and Martelli, 2007; Boudoire et al., 2018). It is worth noting that SCN olivine samples exhibit 4He/20Ne and 40Ar/36Ar falling within the range for VESVF mantle xenoliths, and along the mantle-air mixing trends (Figures 6A,B). Given the magmatic nature of the olivines, we cannot discard that the atmospheric component observed in SCN inclusions has been trapped at crustal depths; unlike helium, heavier noble gases (such as Ne and Ar) have accumulated in the atmosphere over the Earth’s history, to the point that atmospheric contamination challenges interpretation of isotopic ratios, particularly in magmatic products (Farley and Poreda, 1993; Farley and Neroda, 1998; Graham, 2002; Mukhopadhyay and Parai, 2019). Despite the above, the similarity with VESVF noble gas ratios is clear and suggests that SCN olivines are (to some extent) reflecting the signature (Ne-Ar ratios) of the local mantle.
According to petrological evidence (Straub et al., 2011), the SCN lavas derive from a sub-arc mantle affected by the infiltration of silicic crustal material from the subducted slab to the point that -secondary pyroxenite veins formed. If SCN magmas were completely related to subduction, then the fluid inclusion data should exhibit 40Ar/36Ar ratios similar to those observed in lavas and geothermal fluids from other “classic” subduction systems which generally exhibit 40Ar/36Ar < 400 (e.g., Andes, Lesser Antilles or Japan; Hilton et al., 2002; Lages et al., 2021a; Lages et al.,2021b). Figure 9 is a comparison of a selection of published 40Ar/36Ar averages for volcanic products from both subduction and intraplate settings worldwide. As observed, the average for SCN samples is higher than observed in subduction areas but is comparable to those ratios reported in zones where volcanism is related to intraplate extensional tectonics. Based on this evidence, we propose that SCN olivines reflect both subduction (as suggested by petrological data) and intraplate-related components (the latter with 40Ar/36Ar comparable to those reported for the VESVF mantle).
FIGURE 9. 40Ar/36Ar averages (lavas+geothermal fluids) for subduction and intraplate settings. Averages and uncertainties (1σ) were calculated based on the data reported in the literature (Petterson, 1992; Hilton et al., 2002; Italiano et al., 2017; Lages et al., 2021a; Lages et al., 2021b). Plotted MORB and air values are from Moreira et al. (1998) and Lee et al. (2006).
We conclude that, in terms of the gas concentrations and noble gas isotopic ratios, the SCN olivines reflect a sub-arc mantle with similar characteristics to those described for the VESVF mantle (Table 2; Figures 3, 4A, 6A, 10). It is therefore possible that both localities evolved under similar geodynamic conditions due to their proximity (Figure 1).
FIGURE 10. Variability of 3He/4He ratios along the Trans‐mexican Volcanic Belt (TMVB). Values for the Jalisco block, La Primavera caldera (LP) and Ceboruco and Colima volcanoes are from Taran et al. (2002). Fluid inclusion value for the Popocatépetl volcano is from Straub et al. (2011). Bubbling and surface gas for Popocatépetl-Izztaccíuatl volcanoes and for the Cuautla zones are from Inguaggiato et al. (2005). The crustal thickness profile was designed after Urrutia-Fucugauchi and Böhnel (1988) and Urrutia-Fucugauchi et al. (1999).
Our observations, with those of Straub et al. (2011), are among the first fluid inclusion results available for the TMVB, while the majority of the isotopic data previously reported in the literature are from analysis of superficial gas emissions such as fumaroles, bubbling and dissolved gases (e.g., Taran et al., 2002; Inguaggiato et al., 2005). However, the representativeness of these surficial gas emissions for understanding the feeding mantle source characteristics is increasingly questioned (Lages et al., 2021a; Lages et al., 2021b). Therefore, a comparison between FI (this work and Straub et al., 2011) and TMVB surficial gases is timely and useful.
The available information for the TMVB is presented in Figures 6A, 10, and includes samples collected in the Jalisco block, Colima and Ceboruco volcanoes, La Primavera caldera, Popocatepetl and Iztaccihuatl volcanoes, and the Cuautla zone (for their location see Figure 1B). In the 4He/20Ne vs. R/Ra plot (Figure 6A), it is straightforward to distinguish FI from superficial gases, with the latter typically exhibiting lower 3He/4He and X values. Our results therefore confirm that volatiles sampled in peripheral geothermal fields and/or low-temperature bubbling springs are affected by shallow contamination from meteoric, biogenic fluids that result in lower 3He/4He signatures than in FI (and high-temperature fumarole emissions; Sano et al., 1984; Williams et al., 1987; Lages et al., 2021a). These processes are especially relevant for dissolved water samples that also exhibit the lowest 4He/20Ne ratios (very close to the 4He/20Ne air value = 0.318; Ozima and Podosek, 2002) confirming a dominant contamination of surficial gases by atmospheric-derived gases. In the case of Popocatépetl volcano, the difference in Rc/Ra between FI and dissolved water (Figure 10) demonstrates the utility of the former to investigate the source mantle helium isotopic signature. Bubbling/dissolved gas samples from the Jalisco block and the Cuautla zone show extremely low 3He/4He ratios, with averages of 2.24 ± 1.69 Ra (1σ) and 1.66 ± 0.70 Ra (1σ), respectively. Thus, we consider that bubbling and dissolved gas data observed in Figures 6A, 10 underestimate the real 3He/4He signature of the local mantle.
Our δ13C results for the DVF samples (NMNH-39 Opx and Cpx) fall within the MORB-like range (between −5.43‰ and −3.80‰; Figures 11A,B), and bring therefore no evidence of the isotopically heavy, recycled crustal carbon component identified in the VESVF samples (Sandoval-Velasquez et al., 2021a). We propose that the fertile δ13C signature of the DVF mantle likely reflects the addition of MORB-type carbon during the recent (<11 Ma) mantle refertilization event by asthenospheric fluids (Figure 8E). This event may have masked any recycled crustal carbon signature eventually present in the local SCLM. In any case, the DVF vs. VESVF dichotomy points to δ13C mantle heterogeneities at regional scale.
FIGURE 11. (A) δ13C vs. CO2/3He ratios. (B) Closer view of A between −8 and +3‰. Because no information of CO2/3He is available for the Popocatépetl fumaroles, the δ13C average (blue dotted line) was plotted considering the highest CO2/3He value reported for the TMVB within the MORB-like range. This average is reported in Goff et al. (1998). Bibliographic references are provided in Figure 10. Very low (<−7‰) δ13C signature is found in low-temperature gas samples (bubbling gases and dissolved gases in water with T< 100°C) of the Jalisco block, the Cuautla zone and the Popocatepetl and Iztaccíhuatl volcanoes. These samples are not considered as representative of the mantle source since they likely record the effect of shallow secondary processes such as magmatic degassing (Javoy et al., 1978; Trull and Kurz, 1993; Boudoire et al., 2018), interaction with shallow aquifers or hydrothermal systems (Barry et al., 2013; Batista Cruz et al., 2019; Gilfillan et al., 2019; Rizzo et al., 2019), or result from a contamination by organic carbon (Sano and Marty, 1995).
SCN olivines likewise show a MORB-like upper mantle δ13C signature (Figure 11). Popocatépetl crater fumaroles (T. Lorenson and W. C. Evans, unpublished, as cited in Goff et al., 1998), bubbling gas data from La Primavera Caldera, and high-temperature (840°C) fumaroles of Colima volcano (Taran et al., 2002) exhibit similar MORB-type carbon (Figure 11). This negative 13C signature observed in SCN samples (relative to VESVF) can be explained by two distinct processes, taking in mind that crustal contamination in SCN lavas is though to be negligible (see Section 3). On the one hand, it is possible that SCN olivines (and Colima/Popocatépetl gases) record an isotopically fractionated CO2 derived by magmatic degassing. Carbon isotopes are fractionated by magmatic degassing (melt-vapor fractionation factor Δ: from +1.8 to +4.5; Javoy et al., 1978; Mattey, 1991; Aubaud et al., 2005; Paonita et al., 2012; Gennaro et al., 2017; Boudoire et al., 2018), so that residual melts that result from late-stage degassing are 12C-enriched (relative to parental, mantle-derived melts) because of preferential 13C loss to magmatic vapor (Javoy et al., 1978; Boudoire et al., 2018). The high 4He/40Ar*values (3–9) measured in FI of SCN olivines, well above the range observed in mantle xenoliths (3.1 > 4He/40Ar*> 0.1), are consistent with the magmatic degassing hypothesis. In this interpretation, the SCN inclusions (and possibly those from Popocatépetl and Colima volcanoes) would derive from extensive degassing of silicate parental melt with an originally heavier δ13C signature, similar to that observed in VESVF xenoliths. Alternatively, if we accept that the negative δ13C of SCN inclusions is source-related (i.e., that magmatic degassing plays a minor role), then the inferred sub-arc source at −4‰ to −7‰ would imply a negligible contribution of subduction-derived crustal carbon. This hypothesis would be consistent with the carbon data reported for other arc volcanoes such as Ceboruco, La Primavera and the neighbor Popocatépetl (Goff et al., 1998; Taran et al., 2002). In fact, Goff et al. (1998), Goff et al. (2001) and Aiuppa et al. (2017), indicate that the CO2-rich plume gases, emitted by Popocatépetl, are not directly related to subducted sediments (that are not C-rich at the Mexican trench; Plank, 2014), instead, it is the result of assimilation of crustal carbon materials (metamorphosed carbonate rocks).
The study of noble gases and CO2 in FI of mantle xenoliths from DVF and SQVF, together with a previous study on the VESVF, allowed investigating the noble gas and carbon isotopic signatures in the Mexican lithospheric mantle. In addition, olivine phenocrysts from SCN gave the opportunity to extend the study to the present subduction-related volcanism of the TMVB. The main outcomes are:
• The decrease of 4He/40Ar* ratios from olivines to pyroxenes in mantle xenoliths, coupled with a general increase in the Mg#, suggests a complex mantle source impacted by partial melting and/or metasomatic episodes;
• Ne and Ar systematics reveal mixing trends between atmospheric and MORB-like fluids. Based on the isotopic variability of our samples, mass-balance and isotopic mass balance equations were used in order to estimate the 40Ar/36Ar signature of the Mexican lithospheric mantle. DVF and SQVF samples confirm a maximum 40Ar/36Ar of ∼10,500 which supports the presence of an atmospheric component likely recycled via Farallon paleo-subduction.
• The inferred 3He/4He signature is 8.39 ± 0.24 Ra for the DVF xenoliths and 7.43 ± 0.19 Ra for SQVF xenoliths. Regional 3He/4He differences in the Basin and Range mantle are interpreted as the result of different ages of mantle refertilization caused by retreating of the Farallon slab (∼40 Ma ago) and subsequent slab delamination (break-off) episodes. Using a steady-state model in which the mantle becomes more radiogenic over time, we propose that the VESVF and the SQVF source mantle was refertilized earlier (at 40-20 Ma) than the DVF mantle (<10 Ma).
• Fluids trapped in SCN olivines have 3He/4He signatures similar to VESVF mantle xenoliths and suggest that the mantle beneath the two areas evolved under similar geodynamic conditions. The 3He/4He ratios are relatively homogeneous in the mantle source underneath Mexico.
• Contrary to what was observed in the VESVF xenoliths, the carbon isotopic composition of CO2 (δ13C values) reported for the DVF samples are more negative (between −5.43‰ and −3.80‰). As with helium ratios, these MORB-like carbon signatures are linked to the relatively recent refertilization event proposed for the DVF local mantle.
• δ13C values measured in SCN samples also falls within the MORB-like range and are comparable to those reported for the Popocatépel, Ceboruco and Colima fumaroles and bubbling gas obtained from La Primavera caldera. Unlike the evidence found in VESVF, the δ13C values in SCN and in the volcanoes from TMVB indicate that magmas likely result from the melting of mantle with a distinct carbon signature and content than that represented by VESVF xenoliths. This source would also show a negligible contamination by subduction-related crustal carbon.
The original contributions presented in the study are included in the article, further inquiries can be directed to the corresponding author.
AS-V performed analyses of fluid inclusions, elaborated and interpreted data, conceptualized models, and wrote the final version of the manuscript. AR and AA contributed to the conceptualization, methodology, writing-reviewing, editing, and supervision. SMS performed fieldwork, sampling, and write-reviewing. AG-T and RE-P helped with fieldwork and sampling. All authors contributed to manuscript revision, read, and approved the submitted version.
This research was funded by the Italian Minister (PRIN2017LMNLAW) and by the Deep Carbon Observatory.
This paper is part of the PhD (XXXIV cycle) of AS-V at the University of Palermo. We are extremely grateful to the Department of Mineral Sciences of the Smithsonian Institution for providing samples of Mexican mantle xenoliths from the Durango and San Quintin volcanic fields. We thank Mariano Tantillo and Mariagrazia Misseri for helping in sample preparation, the isotope analysis of noble gases and the CO2 extraction from FI performed in the noble gas laboratory of INGV-Palermo. We are also grateful to Ygor Oliveri and Giorgio Capasso for their help in the CO2 isotopic analysis performed in the INGV-Palermo stable isotopes laboratory. We wish to extend our special thanks to Prof. Paola Marianelli, Prof. Karoly Nemeth, and the Reviewer for their insightful comments and suggestions that improved our manuscript.
The authors declare that the research was conducted in the absence of any commercial or financial relationships that could be construed as a potential conflict of interest.
The reviewer MM declared a past co-authorship with the author RE-P to the handling editor.
All claims expressed in this article are solely those of the authors and do not necessarily represent those of their affiliated organizations, or those of the publisher, the editors and the reviewers. Any product that may be evaluated in this article, or claim that may be made by its manufacturer, is not guaranteed or endorsed by the publisher.
DVF, Durango Volcanic Field; SCLM, Subcontinetal Lithospheric Mantle; SCN, Sierra Chichinautzin; SQVF, San Quintin Volcanic Field; TMVB, Trans-mexican Volcanic Belt; VESVF, Ventura Espiritu Santo Volcanic Field.
Aiuppa, A., Fischer, T. P., Plank, T., Robidoux, P., and Di Napoli, R. (2017). Along-arc, inter-arc and arc-to-arc variations in volcanic gas CO2/ST ratios reveal dual source of carbon in arc volcanism. Earth. Sci. Rev. 168, 24–47. doi:10.1016/j.earscirev.2017.03.005
Albritton, C. C. (1958). Quaternary stratigraphy of the guadiana valley, Durango, Mexico. Geol. Soc. Am. Bull. 69, 1197. doi:10.1130/0016-7606(1958)69[1197:QSOTGV]2.0.CO;2
Aranda-Gómez, J. J., Henry, C. D., and Luhr, F. J. (2000). Evolución tectonomagmática post-paleocénica de la Sierra Madre Occidental y de la porción meridional de la provincia tectónica de Cuencas y Sierras, México. Bol. Soc. Geol. Mex. 53, 59–71. doi:10.18268/BSGM2000v53n1a3
Aranda-Gómez, J. J., Luhr, J. F., and Pier, G. (1992). The La Breña — El Jagüey maar complex, Durango, méxico: I. Geological evolution. Bull. Volcanol. 54, 393–404. doi:10.1007/BF00312321
Aranda-Gómez, J. J., and Ortega-Gutiérrez, F. (1987). “Mantle xenoliths in México,” in Mantle xenoliths (New York: John Wiley), 75–84.
Atwater, T. (1989). “Plate tectonic history of the northeast Pacific and western North America,” in The eastern pacific ocean and Hawaii. Editors E. L. Winterer, D. M. Hussong, and R. W. Decker (North America: Geological Society of America), 21–72. doi:10.1130/DNAG-GNA-N.21
Aubaud, C., Pineau, F., Hékinian, R., and Javoy, M. (2005). Degassing of CO2 and H2O in submarine lavas from the Society hotspot. Earth Planet. Sci. Lett. 235, 511–527. doi:10.1016/j.epsl.2005.04.047
Ballentine, C. J., and Burnard, P. G. (2002). Production, release and transport of noble gases in the continental crust. Rev. Mineral. Geochem. 47, 481–538. doi:10.2138/rmg.2002.47.12
Barry, P. H., Hilton, D. R., Fischer, T. P., de Moor, J. M., Mangasini, F., and Ramirez, C. (2013). Helium and carbon isotope systematics of cold “mazuku” CO2 vents and hydrothermal gases and fluids from Rungwe Volcanic Province, southern Tanzania. Chem. Geol. 339, 141–156. doi:10.1016/j.chemgeo.2012.07.003
Basu, A. R. (1977). Textures, microstructures and deformation of ultramafic xenoliths from San Quintin, Baja California. Tectonophysics 43, 213–246. doi:10.1016/0040-1951(77)90118-4
Batista Cruz, R. Y., Rizzo, A. L., Grassa, F., Bernard Romero, R., González Fernández, A., Kretzschmar, T. G., et al. (2019). Mantle degassing through continental crust triggered by active faults: The case of the Baja California Peninsula, Mexico. Geochem. Geophys. Geosyst. 20, 1912–1936. doi:10.1029/2018GC007987
Bekaert, D. V., Turner, S. J., Broadley, M. W., Barnes, J. D., Halldórsson, S. A., Labidi, J., et al. (2021). Subduction-driven volatile recycling: A global mass balance. Annu. Rev. Earth Planet. Sci. 49, 37–70. doi:10.1146/annurev-earth-071620-055024
Boudoire, G., Rizzo, A. L., Di Muro, A., Grassa, F., and Liuzzo, M. (2018). Extensive CO2 degassing in the upper mantle beneath oceanic basaltic volcanoes: First insights from Piton de la Fournaise volcano (La Réunion Island). Geochim. Cosmochim. Acta 235, 376–401. doi:10.1016/j.gca.2018.06.004
Bunge, H.-P., and Grand, S. P. (2000). Mesozoic plate-motion history below the northeast Pacific Ocean from seismic images of the subducted Farallon slab. Nature 405, 337–340. doi:10.1038/35012586
Burnard, P. (2004). Diffusive fractionation of noble gases and helium isotopes during mantle melting. Earth Planet. Sci. Lett. 220, 287–295. doi:10.1016/S0012-821X(04)00060-3
Burnard, P., Graham, D., and Turner, G. (1997). Vesicle-specific noble gas analyses of “popping rock”: Implications for primordial noble gases in Earth. Science 276, 568–571. doi:10.1126/science.276.5312.568
Correale, A., Martelli, M., Paonita, A., Rizzo, A., Brusca, L., and Scribano, V. (2012). New evidence of mantle heterogeneity beneath the Hyblean Plateau (southeast Sicily, Italy) as inferred from noble gases and geochemistry of ultramafic xenoliths. Lithos 132 (133), 70–81. doi:10.1016/j.lithos.2011.11.007
Correale, A., Paonita, A., Rizzo, A., Grassa, F., and Martelli, M. (2015). The carbon-isotope signature of ultramafic xenoliths from the Hyblean Plateau (southeast Sicily, Italy): Evidence of mantle heterogeneity. Geochem. Geophys. Geosyst. 16, 600–611. doi:10.1002/2014GC005656
Dasgupta, R., and Hirschmann, M. M. (2010). The deep carbon cycle and melting in Earth’s interior. Earth Planet. Sci. Lett. 298, 1–13. doi:10.1016/j.epsl.2010.06.039
Dávalos-Elizondo, M. G., Aranda Gómez, J. J., Levresse, G., and Cervantes-de la Cruz, K. E. (2016). Química mineral y geoquímica de xenolitos del manto del campo volcánico Santo Domingo, san luis potosí: Evidencias de procesos metasomáticos del manto bajo porciones de la Mesa central, méxico. Rev. Mex. Ciencias Geol. 33, 81–104.
Day, J. M. D., and Hilton, D. R. (2020). Heterogeneous mantle-derived helium isotopes in the CanaryIslands and other ocean islands. Geology 49, 120–124. doi:10.1130/G47676.1
Day, J. M. D., and Hilton, D. R. (2011). Origin of 3He/4He ratios in HIMU-type basalts constrained from Canary Island lavas. Earth Planet. Sci. Lett. 305, 226–234. doi:10.1016/j.epsl.2011.03.006
Deines, P. (2002). The carbon isotope geochemistry of mantle xenoliths. Earth. Sci. Rev. 58, 247–278. doi:10.1016/S0012-8252(02)00064-8
Dickinson, W. R. (2002). The Basin and range province as a composite extensional domain. Int. Geol. Rev. 44, 1–38. doi:10.2747/0020-6814.44.1.1
Dunai, T. J., and Porcelli, D. (2002). Storage and transport of noble gases in the subcontinental lithosphere. Rev. Mineral. Geochem. 47, 371–409. doi:10.2138/rmg.2002.47.10
Faccini, B., Rizzo, A. L., Bonadiman, C., Ntaflos, T., Seghedi, I., Grégoire, M., et al. (2020). Subduction-related melt refertilisation and alkaline metasomatism in the Eastern Transylvanian Basin lithospheric mantle: Evidence from mineral chemistry and noble gases in fluid inclusions. Lithos 364–365, 105516. doi:10.1016/j.lithos.2020.105516
Farley, K. A., and Neroda, E. (1998). Noble gases in the Earth’s mantle. Annu. Rev. Earth Planet. Sci. 26, 189–218. doi:10.1146/annurev.earth.26.1.189
Farley, K. A., and Poreda, R. J. (1993). Mantle neon and atmospheric contamination. Earth Planet. Sci. Lett. 114, 325–339. doi:10.1016/0012-821X(93)90034-7
Ferrari, L., López-Martínez, M., Aguirre-Díaz, G., and Carrasco-Núñez, G. (1999). Space-time patterns of cenozoic arc volcanism in central Mexico: From the Sierra Madre Occidental to the Mexican volcanic belt. Geology 27, 303–306. doi:10.1130/0091-7613(1999)027<0303:STPOCA>2.3.CO;2
Ferrari, L., López-Martínez, M., and Rosas-Elguera, J. (2002). Ignimbrite flare-up and deformation in the southern Sierra Madre Occidental, western Mexico: Implications for the late subduction history of the Farallon plate: Ignimbrite flare-up and deformation, western Mexico. Tectonics 21, 1–24. doi:10.1029/2001TC001302
Ferrari, L., Orozco-Esquivel, T., Manea, V., and Manea, M. (2012). The dynamic history of the Trans-Mexican Volcanic Belt and the Mexico subduction zone. Tectonophysics 523, 122–149. doi:10.1016/j.tecto.2011.09.018
Gautheron, C., and Moreira, M. (2002). Helium signature of the subcontinental lithospheric mantle. Earth Planet. Sci. Lett. 199, 39–47. doi:10.1016/S0012-821X(02)00563-0
Gennaro, M. E., Grassa, F., Martelli, M., Renzulli, A., and Rizzo, A. L. (2017). Carbon isotope composition of CO2-rich inclusions in cumulate-forming mantle minerals from Stromboli volcano (Italy). J. Volcanol. Geotherm. Res. 346, 95–103. doi:10.1016/j.jvolgeores.2017.04.001
Gilfillan, S. M. V., Györe, D., Flude, S., Johnson, G., Bond, C. E., Hicks, N., et al. (2019). Noble gases confirm plume-related mantle degassing beneath Southern Africa. Nat. Commun. 10, 5028. doi:10.1038/s41467-019-12944-6
Goff, F., Janik, C. J., Werner, C., Counce, D., Stimac, J. A., Siebe, C., et al. (1998). Geochemical surveillance of magmatic volatiles at Popocatépetl volcano, Mexico. GSA Bull. 110, 695–710. doi:10.1130/0016-7606(1998)110<0695:GSOMVA>2.3.CO;2
Goff, F., Love, S. P., Warren, R. G., Counce, D., Obenholzner, J., Siebe, C., et al. (2001). Passive infrared remote sensing evidence for large, intermittent CO2 emissions at Popocatépetl volcano, Mexico. Chem. Geol. 177, 133–156. doi:10.1016/S0009-2541(00)00387-9
Gómez-Tuena, A., Orozco-Esquivel, Ma.T., and Ferrari, L. (2007). “Igneous petrogenesis of the Trans-Mexican volcanic belt,” in Geology of méxico: Celebrating the centenary of the geological society of méxico. Editors S. A. Alaniz-Álvarez, and Á. F. Nieto-Samaniego (Boulder, Colorado: Geological Society of America), 129–181. doi:10.1130/2007.2422(05
Graham, D. W. (2002). Noble gas isotope geochemistry of Mid-ocean ridge and ocean island basalts: Characterization of mantle source reservoirs. Rev. Mineral. Geochem. 47, 247–317. doi:10.2138/rmg.2002.47.8
Gurenko, A. A., Hoernle, K. A., Hauff, F., Schmincke, H.-U., Han, D., Miura, Y. N., et al. (2006). Major, trace element and Nd–Sr–Pb–O–He–Ar isotope signatures of shield stage lavas from the central and western Canary Islands: Insights into mantle and crustal processes. Chem. Geol. 233, 75–112. doi:10.1016/j.chemgeo.2006.02.016
Gutmann, J. T. (1986). Origin of four and five-phase ultramafic xenoliths from Sonora, Mexico. Am. Mineralogist 71, 1079–1084.
Halldórsson, S. A., Hilton, D. R., Marshall, E. W., Ranta, E., Ingvason, A., Chakraborty, S., et al. (2022). Evidence from gas-rich ultramafic xenoliths for Superplume-derived recycled volatiles in the East African sub-continental mantle. Chem. Geol. 589, 120682. doi:10.1016/j.chemgeo.2021.120682
Harðardóttir, S., Halldórsson, S. A., and Hilton, D. R. (2018). Spatial distribution of helium isotopes in Icelandic geothermal fluids and volcanic materials with implications for location, upwelling and evolution of the Icelandic mantle plume. Chem. Geol. 480, 12–27. doi:10.1016/j.chemgeo.2017.05.012
Heber, V. S., Brooker, R. A., Kelley, S. P., and Wood, B. J. (2007). Crystal–melt partitioning of noble gases (helium, neon, argon, krypton, and xenon) for olivine and clinopyroxene. Geochim. Cosmochim. Acta 71, 1041–1061. doi:10.1016/j.gca.2006.11.010
Henry, C. D., and Aranda-Gomez, J. J. (2000). Plate interactions control middle–late Miocene, proto-Gulf and Basin and Range extension in the southern Basin and Range. Tectonophysics 318, 1–26. doi:10.1016/S0040-1951(99)00304-2
Henry, C. D., and Aranda-Gómez, J. J. (1992). The real southern Basin and Range: Mid- to late Cenozoic extension in Mexico. Geology 20, 701–704. doi:10.1130/0091-7613(1992)020<0701:TRSBAR>2.3.CO;2
Hilton, D., Fischer, T., and Marty, B. (2002). Noble gases and volatile recycling at subduction zones. Rev. Mineral. Geochem. 47, 319–370. doi:10.2138/rmg.2002.47.9
Holland, G., and Ballentine, C. J. (2006). Seawater subduction controls the heavy noble gas composition of the mantle. Nature 441, 186–191. doi:10.1038/nature04761
Hopp, J., Trieloff, M., Buikin, A., Korochantseva, E., Schwarz, W., Althaus, T., et al. (2007a). Heterogeneous mantle argon isotope composition in the subcontinental lithospheric mantle beneath the Red Sea region. Chem. Geol. 240, 36–53. doi:10.1016/j.chemgeo.2007.01.004
Housh, T. B., Aranda-Gómez, J. J., and Luhr, J. F. (2010). Isla Isabel (Nayarit, México): Quaternary alkalic basalts with mantle xenoliths erupted in the mouth of the Gulf of California. J. Volcanol. Geotherm. Res. 197, 85–107. doi:10.1016/j.jvolgeores.2009.06.011
Inguaggiato, S., Martin-Del Pozzo, A. L., Aguayo, A., Capasso, G., and Favara, R. (2005). Isotopic, chemical and dissolved gas constraints on spring water from Popocatepetl volcano (Mexico): Evidence of gas–water interaction between magmatic component and shallow fluids. J. Volcanol. Geotherm. Res. 141, 91–108. doi:10.1016/j.jvolgeores.2004.09.006
Italiano, F., Yuce, G., Di Bella, M., Rojay, B., Sabatino, G., Tripodo, A., et al. (2017). Noble gases and rock geochemistry of alkaline intraplate volcanics from the Amik and Ceyhan-Osmaniye areas, SE Turkey. Chem. Geol. 469, 34–46. doi:10.1016/j.chemgeo.2017.04.003
I. Jackson (Editor) (1998). The Earth’s mantle: Composition, structure, and evolution (Cambridge: Cambridge University Press). doi:10.1017/CBO9780511573101
Jambon, A., Weber, H. W., and Begemann, F. (1985). Helium and argon from an atlantic MORB glass: Concentration, distribution and isotopic composition. Earth Planet. Sci. Lett. 73, 255–268. doi:10.1016/0012-821X(85)90074-3
Javoy, M., Pineau, F., and Iiyama, I. (1978). Experimental determination of the isotopic fractionation between gaseous CO2 and carbon dissolved in tholeiitic magma: A preliminary study. Contr. Mineral. Pet. 67, 35–39. doi:10.1007/BF00371631
Kelley, K. A., and Cottrell, E. (2009). Water and the oxidation state of subduction zone magmas. Science 325, 605–607. doi:10.1126/science.1174156
Lages, J., Rizzo, A., Aiuppa, A., Robidoux, P., Aguilar, R., Apaza Choquehuayta, F., et al. (2021b). Crustal controls on light noble gas isotope variability along the Andean Volcanic Arc. Geochem. Perspect. Lett. 19, 45–49. doi:10.7185/geochemlet.2134
Lages, J., Rizzo, A. L., Aiuppa, A., Samaniego, P., Le Pennec, J. L., Ceballos, J. A., et al. (2021a). Noble gas magmatic signature of the Andean Northern Volcanic Zone from fluid inclusions in minerals. Chem. Geol. 559, 119966. doi:10.1016/j.chemgeo.2020.119966
Langmuir, C. H., Vocke, R. D., Hanson, G. N., and Hart, S. R. (1978). A general mixing equation with applications to Icelandic basalts. Earth Planet. Sci. Lett. 37, 380–392. doi:10.1016/0012-821X(78)90053-5
Lee, C. A. (2005). Trace element evidence for hydrous metasomatism at the base of the North American lithosphere and possible association with Laramide low‐angle subduction. J. Geol. 113, 673–685. doi:10.1086/449327
Lee, J.-Y., Marti, K., Severinghaus, J. P., Kawamura, K., Yoo, H.-S., Lee, J. B., et al. (2006). A redetermination of the isotopic abundances of atmospheric Ar. Geochim. Cosmochim. Acta 70, 4507–4512. doi:10.1016/j.gca.2006.06.1563
Li, K., Li, L., Pearson, D. G., and Stachel, T. (2019). Diamond isotope compositions indicate altered igneous oceanic crust dominates deep carbon recycling. Earth Planet. Sci. Lett. 516, 190–201. doi:10.1016/j.epsl.2019.03.041
Luhr, J. F., Aranda-Gómez, J. J., and Housh, T. B. (1995a). San Quintín volcanic field, Baja California norte, méxico: Geology, petrology, and geochemistry. J. Geophys. Res. 100, 10353–10380. doi:10.1029/95JB00037
Luhr, J. F., and Aranda-Gomez, J. J. (1997). Mexican peridotite xenoliths and tectonic terranes: Correlations among vent location, texture, temperature, pressure, and oxygen fugacity. J. Petrology 38, 1075–1112. doi:10.1093/petroj/38.8.1075
Luhr, J. F., Aranda-Gomez, J. J., and Pier, J. G. (1989). Spinel-lherzolite-bearing quaternary volcanic centers in san luis potosí, Mexico: 1. Geology, mineralology, and petrology. J. Geophys. Res. 94, 7916. doi:10.1029/JB094iB06p07916
Mangler, M., Prytulak, J., Gisbert, G., Delgado-Granados, H., and Petrone, C. M. (2019). Interplinian effusive activity at Popocatépetl volcano, Mexico: New insights into evolution and dynamics of the plumbing system. Volcanica 2, 45–72. doi:10.30909/vol.02.01.4572
Márquez, A., Oyarzun, R., Doblas, M., and Verma, S. P. (1999a). Alkalic (ocean-island basalt type) and calc-alkalic volcanism in the Mexican volcanic belt: A case for plume-related magmatism and propagating rifting at an active margin? Geol. 27, 51–54. doi:10.1130/0091-7613(1999)027<0051:AOIBTA>2.3.CO;2
Márquez, A., Verma, S. P., Anguita, F., Oyarzun, R., and Brandle, J. L. (1999b). Tectonics and volcanism of Sierra Chichinautzin: Extension at the front of the central Trans-Mexican volcanic belt. J. Volcanol. Geotherm. Res. 93, 125–150. doi:10.1016/S0377-0273(99)00085-2
Martelli, M., Bianchini, G., Beccaluva, L., and Rizzo, A. (2011). Helium and argon isotopic compositions of mantle xenoliths from Tallante and Calatrava, Spain. J. Volcanol. Geotherm. Res. 200, 18–26. doi:10.1016/j.jvolgeores.2010.11.015
Matsumoto, T., Chen, Y., and Matsuda, J.-I. (2001). Concomitant occurrence of primordial and recycled noble gases in the Earth’s mantle. Earth Planet. Sci. Lett. 185, 35–47. doi:10.1016/S0012-821X(00)00375-7
Matsumoto, T., Pinti, D. L., Matsuda, J.-I., and Umino, S. (2002). Recycled noble gas and nitrogen in the subcontinental lithospheric mantle: Implications from N-He-Ar in fluid inclusions of SE Australian xenoliths. Geochem. J. 36, 209–217. doi:10.2343/geochemj.36.209
Mattey, D. P. (1991). Carbon dioxide solubility and carbon isotope fractionation in basaltic melt. Geochim. Cosmochim. Acta 55, 3467–3473. doi:10.1016/0016-7037(91)90508-3
McMurtry, G. M., Dasilveira, L. A., Horn, E. L., DeLuze, J. R., and Blessing, J. E. (2019). High 3He/4He ratios in lower East Rift Zone steaming vents precede a new phase of Kilauea 2018 eruption by 8 months. Sci. Rep. 9, 11860. doi:10.1038/s41598-019-48268-0
Meriggi, L., MacÃ\-as, J. L., Tommasini, S., Capra, L., and Conticelli, S., 2008. Heterogeneous magmas of the quaternary Sierra Chichinautzin volcanic field (central Mexico): The role of an amphibole-bearing mantle and magmatic evolution processes. Rev. Mex. Cienc. GeolÃ3gicas 25, 197–216.
Moreira, M., Kunz, J., and Allègre, C. (1998). Rare gas systematics in popping rock: Isotopic and elemental compositions in the upper mantle. Science 279, 1178–1181. doi:10.1126/science.279.5354.1178
Moreira, M., and Sarda, P. (2000). Noble gas constraints on degassing processes. Earth Planet. Sci. Lett. 176, 375–386. doi:10.1016/S0012-821X(00)00010-8
Mukhopadhyay, S., and Parai, R. (2019). Noble gases: A record of Earth’s evolution and mantle dynamics. Annu. Rev. Earth Planet. Sci. 47, 389–419. doi:10.1146/annurev-earth-053018-060238
Németh, K., and Kósik, S. (2020). Review of explosive hydrovolcanism. Geosciences 10, 44. doi:10.3390/geosciences10020044
Nieto-Samaniego, Á. F., Alaniz-Álvarez, S. A., and Camprubí í Cano, A. (2005). La Mesa central de México: Estratigrafía, estructura y evolución tectónica cenozoica. Bol. Soc. Geol. Mex. 57, 285–318. doi:10.18268/BSGM2005v57n3a3
Nieto-Samaniego, Á. F., Ferrari, L., Alaniz-Alvarez, S. A., Labarthe-Hernández, G., and Rosas-Elguera, J. (1999). Variation of Cenozoic extension and volcanism across the southern Sierra Madre Occidental volcanic province, Mexico. Geol. Soc. Am. Bull. 111, 347–363. doi:10.1130/0016-7606(1999)111<0347:VOCEAV>2.3.CO;2
Paonita, A., Caracausi, A., Iacono-Marziano, G., Martelli, M., and Rizzo, A. (2012). Geochemical evidence for mixing between fluids exsolved at different depths in the magmatic system of Mt Etna (Italy). Geochim. Cosmochim. Acta 84, 380–394. doi:10.1016/j.gca.2012.01.028
Paonita, A., and Martelli, M. (2007). A new view of the He–Ar–CO2 degassing at mid-ocean ridges: Homogeneous composition of magmas from the upper mantle. Geochim. Cosmochim. Acta 71, 1747–1763. doi:10.1016/j.gca.2006.12.019
Parkinson, I. J., and Arculus, R. J. (1999). The redox state of subduction zones: Insights from arc-peridotites. Chem. Geol. 160, 409–423. doi:10.1016/S0009-2541(99)00110-2
Pearson, D. G., Canil, D., and Shirey, S. B. (2014). “Mantle samples included in volcanic rocks,” in Treatise on geochemistry (Elsevier), 169–253. doi:10.1016/B978-0-08-095975-7.00216-3
Petterson, D. B. (1992). Noble gas geochemistry of selected basalts and andesites: New Zealand, Tonga - kermadec and Vanuatu (PhD). Canberra: Australian National University.
Pier, J. G., Luhr, J. F., Podosek, F. A., and Aranda-Gómez, J. J. (1992). The La breña-el Jagüey maar complex, Durango, Mexico: II. Petrology and geochemistry. Bull. Volcanol. 54, 405–428. doi:10.1007/BF00312322
Pier, J. G., Podosek, F. A., Luhr, J. F., Brannon, J. C., and Aranda-Gómez, J. J. (1989). Spinel-lherzolite-bearing quaternary volcanic centers in san luis potosí, Mexico: 2. SR and ND isotopic systematics. J. Geophys. Res. 94, 7941. doi:10.1029/JB094iB06p07941
Rizzo, A. L., Caracausi, A., Chavagnac, V., Nomikou, P., Polymenakou, P. N., Mandalakis, M., et al. (2019). Geochemistry of CO2-rich gases venting from submarine volcanism: The case of kolumbo (hellenic volcanic arc, Greece). Front. Earth Sci. 7, 60. doi:10.3389/feart.2019.00060
Rizzo, A. L., Faccini, B., Casetta, F., Faccincani, L., Ntaflos, T., Italiano, F., et al. (2021). Melting and metasomatism in West Eifel and Siebengebirge Sub-Continental Lithospheric Mantle: Evidence from concentrations of volatiles in fluid inclusions and petrology of ultramafic xenoliths. Chem. Geol. 581, 120400. doi:10.1016/j.chemgeo.2021.120400
Rizzo, A. L., Pelorosso, B., Coltorti, M., Ntaflos, T., Bonadiman, C., Matusiak-Małek, M., et al. (2018). Geochemistry of noble gases and CO2 in fluid inclusions from lithospheric mantle beneath wilcza góra (lower silesia, southwest Poland). Front. Earth Sci. 6, 215. doi:10.3389/feart.2018.00215
Sandoval-Velasquez, A., Rizzo, A. L., Aiuppa, A., Remigi, S., Padrón, E., Pérez, N. M., et al. (2021b). Recycled crustal carbon in the depleted mantle source of El Hierro volcano, Canary Islands. Lithos 400–401, 106414. doi:10.1016/j.lithos.2021.106414
Sandoval-Velasquez, A., Rizzo, A. L., Frezzotti, M. L., Saucedo, R., and Aiuppa, A. (2021a). The composition of fluids stored in the central Mexican lithospheric mantle: Inferences from noble gases and CO2 in mantle xenoliths. Chem. Geol. 576, 120270. doi:10.1016/j.chemgeo.2021.120270
Sano, Y., and Marty, B. (1995). Origin of carbon in Fumarolic gas from island arcs. Chem. Geol. 119, 265–274. doi:10.1016/0009-2541(94)00097-R
Sano, Y., Nakamura, Y., Wakita, H., Urabe, A., and Tominaga, T. (1984). Helium-3 emission related to volcanic activity. Science 224, 150–151. doi:10.1126/science.224.4645.150
Schaaf, P., Stimac, J., Siebe, C., and Macías, J. L. (2005). Geochemical evidence for mantle origin and crustal processes in volcanic rocks from Popocatépetl and surrounding monogenetic volcanoes, Central Mexico. J. Pet. 46, 1243–1282. doi:10.1093/petrology/egi015
Sedlock, R. L. (2003). “Geology and tectonics of the Baja California Peninsula and adjacent areas,” in Tectonic evolution of northwestern Mexico and the southwestern USA (Boulder, Colorado: Geological Society of America). doi:10.1130/0-8137-2374-4.1
Sedlock, R. L., Ortega-Gutiérrez, F., and Speed, R. C. (1993). Tectonostratigraphic terranes and tectonic evolution of Mexico. Boulder, Colorado: Geological Society of America Special PapersGeological Society of America. doi:10.1130/SPE278
Severinghaus, J., and Atwater, T. (1990). “Chapter 1: Cenozoic geometry and thermal state of the subducting slabs beneath western North America,” in Geological society of America memoirs (Boulder, Colorado: Geological Society of America), 1–22. doi:10.1130/MEM176-p1
Straub, S., Batanova, V. G., Gómez-Tuena, A., Espinasa-Perena, R., Fleming, W. L., Bindeman, I. N., et al. 2022. Olivine Ca as proxy to mantle wedge depletion in the Trans-Mexican volcanic belt.
Straub, S. M., Gómez-Tuena, A., Bindeman, I. N., Bolge, L. L., Brandl, P. A., Espinasa-Perena, R., et al. (2015). Crustal recycling by subduction erosion in the central Mexican Volcanic Belt. Geochim. Cosmochim. Acta 166, 29–52. doi:10.1016/j.gca.2015.06.001
Straub, S. M., Gomez-Tuena, A., Stuart, F. M., Zellmer, G. F., Espinasa-Perena, R., Cai, Y., et al. (2011). Formation of hybrid arc andesites beneath thick continental crust. Earth Planet. Sci. Lett. 303, 337–347. doi:10.1016/j.epsl.2011.01.013
Straub, S. M., Gómez-Tuena, A., Zellmer, G. F., Espinasa-Perena, R., Stuart, F. M., Cai, Y., et al. (2013). The processes of melt differentiation in arc volcanic rocks: Insights from OIB-type Arc magmas in the central Mexican volcanic belt. J. Pet. 54, 665–701. doi:10.1093/petrology/egs081
Straub, S. M., LaGatta, A. B., Martin-Del Pozzo, A. L., and Langmuir, C. H. (2008). Evidence from high-Ni olivines for a hybridized peridotite/pyroxenite source for orogenic andesites from the central Mexican volcanic belt: Andesite petrogenesis in central mvb. Geochem. Geophys. Geosyst. 9, na. doi:10.1029/2007gc001583
Straub, S. M., Zellmer, G. F., Gómez-Tuena, A., Espinasa-Pereña, R., Martin-del Pozzo, A. L., Stuart, F. M., et al. (2014). A genetic link between silicic slab components and calc-alkaline arc volcanism in central Mexico. Geol. Soc. Lond. Spec. Publ. 385, 31–64. doi:10.1144/SP385.14
Swanson, E. R. (1989). A new type of maar volcano from the State of Durango-the El Jagüey-La Breña complex reinterpreted, 8. Ciudad de México: Universidad Nacional Autónoma de México, Revista del Instituto de Geología, 243–248.
Taran, Y., Inguaggiato, S., Varley, N., Capasso, G., and Favara, R. (2002). Helium and carbon isotopes in thermal waters of the Jalisco block, Mexico. Geofísica Int. 41.
Trull, T. W., and Kurz, M. D. (1993). Experimental measurements of 3He and 4He mobility in olivine and clinopyroxene at magmatic temperatures. Geochim. Cosmochim. Acta 57, 1313–1324. doi:10.1016/0016-7037(93)90068-8
Urrutia-Fucugauchi, J., and Böhnel, H. (1988). Tectonics along the Trans-Mexican volcanic belt according to palaeomagnetic data. Phys. Earth Planet. Interiors 52, 320–329. doi:10.1016/0031-9201(88)90124-0
Urrutia-Fucugauchi, J., Flores-Ruiz, J. H., Bandy, W. L., and Mortera-Gutiérrez, C. A. (1999). Crustal structure of the Colima rift, western Mexico: Gravity models revisited. GeofInt. 38, 205–216. doi:10.22201/igeof.00167169p.1999.38.4.503
Vance, D., Stone, J. O. H., and O’Nions, R. K. (1989). He, Sr and Nd isotopes in xenoliths from Hawaii and other oceanic islands. Earth Planet. Sci. Lett. 96, 147–160. doi:10.1016/0012-821X(89)90129-5
Williams, S. N., Sano, Y., and Wakita, H. (1987). Helium-3 emission from Nevado del ruiz volcano, Colombia. Geophys. Res. Lett. 14, 1035–1038. doi:10.1029/GL014i010p01035
Witter, J. B., Kress, V. C., and Newhall, C. G. (2005). Volcán Popocatépetl, Mexico. Petrology, magma mixing, and immediate sources of volatiles for the 1994–present eruption. J. Pet. 46, 2337–2366. doi:10.1093/petrology/egi058
Wood, B. J., Bryndzia, L. T., and Johnson, K. E. (1990). Mantle oxidation state and its relationship to tectonic environment and fluid speciation. Science 248, 337–345. doi:10.1126/science.248.4953.337
Keywords: Basin and Range province, Trans‐mexican Volcanic Belt, Mexican mantle xenoliths, arc lavas, fluid inclusions, noble gas isotopes, CO2 isotopes, carbon recycling
Citation: Sandoval-Velasquez A, Rizzo AL, Aiuppa A, Straub SM, Gomez-Tuena A and Espinasa-Perena R (2022) The heterogeneity of the Mexican lithospheric mantle: Clues from noble gas and CO2 isotopes in fluid inclusions. Front. Earth Sci. 10:973645. doi: 10.3389/feart.2022.973645
Received: 20 June 2022; Accepted: 04 August 2022;
Published: 07 September 2022.
Edited by:
Paola Marianelli, University of Pisa, ItalyReviewed by:
Martin Mangler, Durham University, United KingdomCopyright © 2022 Sandoval-Velasquez, Rizzo, Aiuppa, Straub, Gomez-Tuena and Espinasa-Perena. This is an open-access article distributed under the terms of the Creative Commons Attribution License (CC BY). The use, distribution or reproduction in other forums is permitted, provided the original author(s) and the copyright owner(s) are credited and that the original publication in this journal is cited, in accordance with accepted academic practice. No use, distribution or reproduction is permitted which does not comply with these terms.
*Correspondence: Andres Sandoval-Velasquez, YW5kcmVzbGliYXJkby5zYW5kb3ZhbHZlbGFzcXVlekBjb21tdW5pdHkudW5pcGEuaXQ=
Disclaimer: All claims expressed in this article are solely those of the authors and do not necessarily represent those of their affiliated organizations, or those of the publisher, the editors and the reviewers. Any product that may be evaluated in this article or claim that may be made by its manufacturer is not guaranteed or endorsed by the publisher.
Research integrity at Frontiers
Learn more about the work of our research integrity team to safeguard the quality of each article we publish.