- 1Department of Oceanography and Coastal Sciences, Louisiana State University, Baton Rouge, LA, United States
- 2Coastal Studies Institute, Louisiana State University, Baton Rouge, LA, United States
- 3United States Geological Survey, Wetland and Aquatic Research Center, Baton Rouge, LA, United States
- 4School of Meteorology, University of Oklahoma, Norman, OK, United States
- 5South Central Climate Adaptation Science Center, University of Oklahoma, Norman, OK, United States
- 6Department of Geography and Anthropology, Louisiana State University, Baton Rouge, LA, United States
Recent research has linked the climate variability associated with ocean-atmosphere teleconnections to impacts rippling throughout environmental, economic, and social systems. This research reviews recent literature through 2021 in which we identify linkages among the major modes of climate variability, in the form of ocean-atmosphere teleconnections, and the impacts to temperature and precipitation of the South-Central United States (SCUSA), consisting of Arkansas, Louisiana, New Mexico, Oklahoma, and Texas. The SCUSA is an important areal focus for this analysis because it straddles the ecotone between humid and arid climates in the United States and has a growing population, diverse ecosystems, robust agricultural and other economic sectors including the potential for substantial wind and solar energy generation. Whereas a need exists to understand atmospheric variability due to the cascading impacts through ecological and social systems, our understanding is complicated by the positioning of the SCUSA between subtropical and extratropical circulation features and the influence of the Pacific and Atlantic Oceans, and the adjacent Gulf of Mexico. The Southern Oscillation (SO), Pacific-North American (PNA) pattern, North Atlantic Oscillation (NAO) and the related Arctic Oscillation (AO), Atlantic Multidecadal Oscillation/Atlantic Multidecadal Variability (AMO/AMV), and Pacific Decadal Oscillation/Pacific Decadal Variability (PDO/PDV) have been shown to be important modulators of temperature and precipitation variables at the monthly, seasonal, and interannual scales, and the intraseasonal Madden-Julian Oscillation (MJO) in the SCUSA. By reviewing these teleconnection impacts in the region alongside updated seasonal correlation maps, this research provides more accessible and comparable results for interdisciplinary use on climate impacts beyond the atmospheric-environmental sciences.
Introduction
Ocean-atmosphere teleconnections—climate anomalies related to each other over large distances–and temporally-spatially defined climate modes link weather and climate variability occurring across time and space. Understanding such linkages can enhance skill in predicting weather and climate changes at seasonal and longer time scales, which in turn can be utilized by decision makers to anticipate the environmental, economic, and social impacts of such ocean-atmosphere variability, particularly in its extreme modes. At the synoptic scale, impacts of the major teleconnections as manifested on synoptic types (Sheridan and Lee, 2012; McGregor 2017) and hydroclimatic variability (McGregor, 2017) have been reviewed thoroughly. Regionally, similar work has been done recently for Australia (Frederiksen et al., 2014), the Horn of Africa (Bahaga et al., 2019), and the North Polar area (Bushra and Rohli, 2021). The objective of this research is to review the distinguishing features of the major modes of climate variability and their teleconnections that influence the atmospheric environment of the south-central United States (SCUSA)—defined here as the states of Arkansas, Louisiana, New Mexico, Oklahoma, and Texas—including a description of those impacts. While a literature review through 2021 is the primary tool for meeting the objective, the varying periods of analysis, data sets employed, and variables impacted complicates the comparison of previous work. Thus, this research also includes original maps of each teleconnection’s degree and geographic extent of influence on temperature and precipitation, using the 1950–2020 averaging period and a modern, high-resolution data set, for comparison to the consensus of previous work on the impacts of major teleconnections on temperature and precipitation in the SCUSA.
Overview of the primary ocean-atmosphere teleconnections
Teleconnection research has a rich history since the identification of the Southern Oscillation (SO; Walker and Bliss, 1932) as the “see-saw” in surface atmospheric pressure anomalies between the western and eastern tropical Pacific Ocean. Related and concurrent extreme anomalies in the ocean have been known for centuries. Specifically, the El Niño phenomenon is characterized by weakening or even reversal of the easterly trade winds in both hemispheres that results in cooler surface waters and shallower thermocline in the western tropical Pacific Ocean concurrent with anomalously warm near-surface waters in the eastern tropical Pacific Ocean, that produce a reduced tilt in the equatorial Pacific thermocline and reduces upwelling along the South American west coast. By contrast, the La Niña phenomenon involves a strengthening of the trade winds and the Walker circulation in general that results in warmer near-surface waters and a deepening of the thermocline in the western tropical Pacific Ocean and increased tilt in the equatorial Pacific thermocline that promotes stronger upwelling and colder near-surface waters in the eastern tropical Pacific Ocean.
Mid-20th century technological advances in upper-air data collection, along with research emphasizing the connection of the SO to El Niño (ENSO; Bjerknes, 1969), led to advances in understanding the SO, such as the identification of its 2- to 7-year variability (Trenberth, 1976; Trenberth, 1984), its relation to extratropical weather (van Loon and Rogers, 1981), complexity and geographically-varying ocean-air feedbacks (Alexander et al., 2002), and nonlinearity of impacts (Hoerling et al., 1997; Hsieh et al., 2006) primarily through variability in surface heat and moisture fluxes and their transport (Deser et al., 2010). Meanwhile, research on identifying other teleconnections of variability in the ocean-atmosphere system at various spatial and temporal scales continued, including recognition of the importance of the surface energy fluxes (Deser et al., 2010). In particular, Barnston and Livezey (1987) provided a thorough description and analysis of teleconnections that explain significant low-frequency variability in atmospheric flow.
The spatial domains of the SO and the other modes of climate variability to be considered in this research, as represented by correlations of the respective climate mode index with SST or another key defining atmospheric variable, are depicted in Figure 1. Among the dozens of modes of climate variability identified since the SO, the Pacific-North American (PNA) pattern (Wallace and Gutzler, 1981; Barnston and Livezey, 1987; Leathers and Palecki, 1992) is among the most prominent for the SCUSA. The PNA index is obtained by obtaining the second rotated empirical orthogonal function mode of the 500 mbar geopotential height across 0°-90°N, and may be an atmospheric response to forcing by ENSO (Renwick and Wallace, 1996; Straus and Shukla, 2002) with the strength of the response differing across and within ENSO phases (Wang et al., 2021) associated with barotropic instability in the atmosphere (Simmons et al., 1983) or other forcings (He and Wang, 2013; Liu et al., 2017), and with the extratropical response to ENSO changing over time (Soulard et al., 2019). The PNA pattern is expressed in the form of variability in the amplitude and phase of the atmospheric Rossby wave train. More specifically, an enhanced mid-tropospheric ridge over the western cordillera of North America and concurrent trough over the southeastern United States (i.e., positive PNA phase) is more commonly associated with the El Niño phase of ENSO, and a dampened or reversed ridge-trough configuration over the same action centers (i.e., negative PNA phase) is more typical in the La Niña phase. The PNA pattern has long been linked to North American climatic variability, including through its connection to tropical variability (Mo and Livezey, 1986), regarding temperature (Loikith and Broccoli, 2014), precipitation (Henderson and Robinson, 1994; Liu et al., 2014), and extreme weather events (Bentley et al., 2019). Recent research has identified a causal relationship to sea surface temperatures (SSTs) less directly related to ENSO, in the South China Sea (Zhang and Liang, 2021).
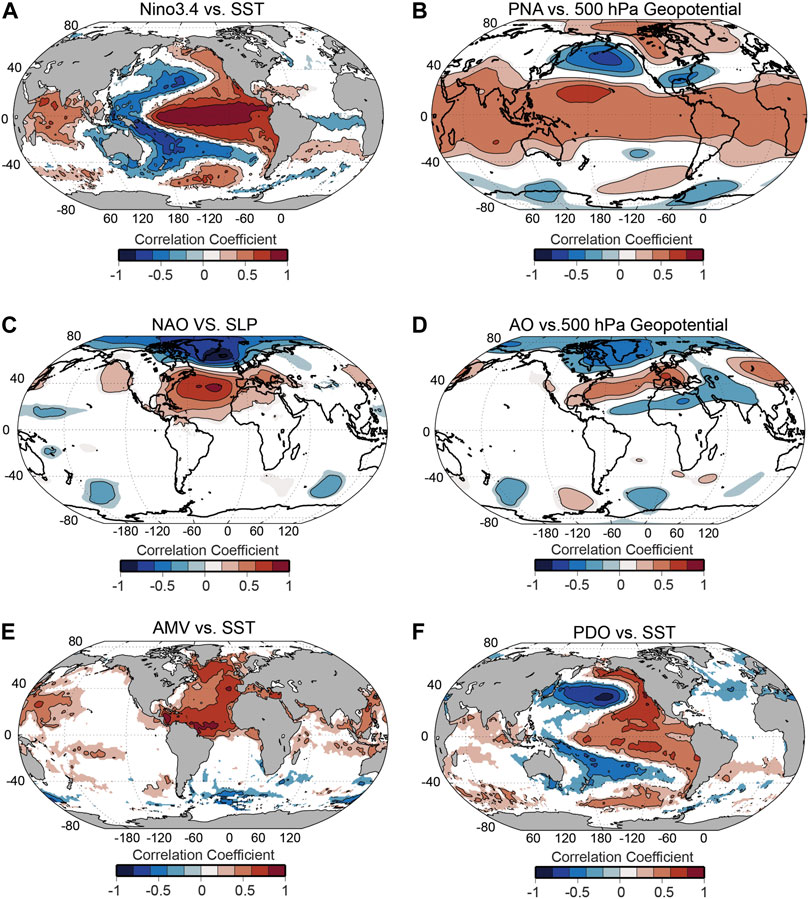
FIGURE 1. Centers of action for Nino3.4 (A), AMV (E), PDO (F), identified by mapping their correlations with Hadley sea surface temperature (SST; https://www.metoffice.gov.uk/hadobs/hadisst/); NAO (C), identified by mapping its correlation with sea-level pressure (ERA5; https://cds.climate.copernicus.eu/); and PNA (B) and AO (D), identified by mapping their correlations with 500 hPa geopotential (ERA5; https://cds.climate.copernicus.eu/). Only regions where correlations were significant (alpha = 0.1) are shown on the maps.
The North Atlantic Oscillation (NAO; Rogers, 1984; Barnston and Livezey, 1987; Lamb and Peppler, 1987; Hurrell and van Loon, 1997; Marshall et al., 2001) came to prominence first, as an observed simultaneous dipole or “see-saw” in near-surface air temperature anomalies between Greenland and Europe (Loewe, 1966; van Loon and Rogers, 1978). The NAO has been recognized as a major forcing mechanism for transporting energy to the North Atlantic Ocean via the Atlantic Meridional Overturning Circulation (AMOC), with abundant air-sea energy exchange (Rodwell et al., 1999), and eventually affecting Northern Hemisphere multidecadal precipitation patterns (Zhang et al., 2021). The NAO-related (Wallace, 2000; Rogers and McHugh, 2002) Arctic Oscillation (AO; Thompson and Wallace, 1998; Thompson and Wallace, 2000) is also known as the Northern Hemispheric Annular Mode (NAM; Thompson et al., 2000), but was first described comprehensively by Lorenz (1951) and since has gained increasing attention as a prominent source of atmospheric flow variability in North America. The degree of coupling between the NAO and AO is now known to depend on temperature in the climate system (Hamouda et al., 2021).
The Atlantic Multidecadal Oscillation (AMO; Schlesinger and Ramankutty, 1994; Kerr, 2000) is an important and impactful, though enigmatic, ocean-atmosphere mode of climate variability in SST observed at multi-decadal time scales. The AMO had originally been named because of its characterization as an approximately 50- to 80-year cycle in which SST in the North Atlantic Ocean undergoes multi-decadal-scale variability of approximately 0.4 C° between the extreme phases after removing the global trend (Enfield et al., 2001). The AMO is now referred to as the Atlantic Multidecadal Variability (AMV) as recent evidence (Mann, 2021) suggests that it may not be a true oscillation. Paleoclimate reconstructions of the AMV reveal multi-decadal variability before the instrumental period, yet land- (Gray et al., 2004) and ocean-based reconstructions are misaligned in phasing and timing (Poore et al., 2009; Kilbourne et al., 2014), and the Loop Current in the Gulf of Mexico plays an important but complicating role (DeLong et al., 2014), leaving continued unanswered questions as to the AMO being a true oscillation that extends back in time. Knight et al. (2005) linked the AMV to thermohaline circulation variability, and Dima and Lohmann (2007) proposed more specifically that the AMV is modulated as freshwater variability causes a thermohaline circulation adjustment, which triggers subsequent SST response with sea ice and wind feedbacks. Clement et al. (2015) found that AMV variability is primarily a response to mid-latitude atmospheric forcing, with the AMOC and similar oceanic circulation responding in turn to the AMV rather than forcing it. The most recent AMV-related warming began around 1997 (Ellis and Marston, 2020). Interestingly, between 1995 and 2008, the Atlantic coast of the United States was one of the few places in the North Atlantic in which SSTs were not anomalously warm (Alexander et al., 2014). Debate continues as to the extent to which the AMV is driven by processes internal to the atmosphere-ocean system (Ting et al., 2009; Han et al., 2016; Deser and Phillips, 2021) related closely to the AMOC (Fang et al., 2021), natural external variability such as volcanic forcing (Wang et al., 2017; Mann, 2021), or anthropogenic external aerosol forcing (Booth et al., 2012). Several studies suggest that some combination therein appears likely (Ting et al., 2014; Qin et al., 2020), with the NAO also potentially playing a role in the relative contributions (Watanabe and Tatebe, 2019). Zhang et al. (2019) expressed hope for future improvements in the most recent climate models for more complete representation of the role of the AMOC on AMV and its impacts.
The analogous amorphous mode of low-frequency ocean-atmosphere variability in the Pacific Ocean initially gained widespread attention through analyses of SST in the mid-latitude and tropical Pacific (Davis, 1976). Specifically, North Pacific Ocean SST anomalies tended to display a simultaneous seesaw between near-coast Alaska and the central North Pacific. Rogers (1990) noted an atmospheric sea-level pressure (SLP; and therefore flow) seesaw in the northeastern Pacific near Alaska known as the North Pacific Oscillation (NPO). Gershunov and Barnett (1998) subsequently referred to the “NPO” as the SST (rather than the SLP) anomaly seesaw, and others have referred to a “North Pacific mode” (Tanimoto et al., 1993; Barlow et al., 2001) to refer to the same phenomenon. This North Pacific SST seesaw was shown to be an important modulator of within-ENSO impacts (Gershunov and Barnett, 1998). The collapse of fisheries off the Pacific Northwest coast of the United States in 1976 that corresponded to a shift in North Pacific SST anomalies led to the naming of the Pacific Interdecadal Oscillation (Mantua et al., 1997), which was shortened to the Pacific Decadal Oscillation (PDO; Mantua et al., 1997; Biondi et al., 2001; Mantua and Hare, 2002; Newman et al., 2003; Schneider and Cornuelle, 2005). The “warm phase” (“cold phase”) of the PDO is conventionally considered to be the phase in which SSTs near North America are anomalously warm (cold). The recent period of rapid global warming is also associated with the PDO’s warm phase, and the slower warming period is associated with the cold phase, as internal cooling by heat storage and circulation changes in the Pacific Ocean occurs (Meehl et al., 2013).
Another widely-used climate index used to represent the mode of whole Pacific Ocean variability is the Interdecadal Pacific Oscillation (IPO) (Salinger et al., 2001; Folland et al., 2002). Further research has found three centers of action for Pacific SST anomalies known as the Tripole Index (TPI) that combines the PDO, IPO, and Southern Hemisphere PDO (SHPDO; Shakun and Shaman, 2009) or South Pacific Decadal Oscillation (SPDO; Hsu and Chen, 2011; DeLong et al., 2012) as the tripole of variability (Henley et al., 2015). The North Pacific Gyre Oscillation (NPGO; Di Lorenzo et al., 2008; Ceballos et al., 2009; Di Lorenzo and Mantua, 2016; Tranchant et al., 2019) is related to the PDO but is a distinct mode of variability. In recognition that multiple Pacific atmospheric features are occurring, that an anthropogenic influence is likely (Bonfils and Santer, 2011), and that like the AMV, the PDO may not be a true oscillation, recent research now uses Pacific Decadal Variability (PDV; Deser et al., 2012) rather than the PDO, with several PDV modes interacting with each other and ENSO (Shakun and Shaman, 2009; Newman et al., 2016). Paleoclimate reconstructions that extend the observational record back more than 300 years with corals confirm these differing modes based on their geographic location—the IPO in the South Pacific Convergence Zone region (Linsley et al., 2008), the SPDO; Hsu and Chen (2011) or Southern Hemisphere Decadal Oscillation (SHPDO) in the southwestern Pacific Ocean (DeLong et al., 2012), and the PDO in the northern Pacific (Felis et al., 2010). Since 1976, the PDO/PDV has generally been in a so-called warm phase but currently appears to be shifting toward a cold phase, with the naming convention of the phases corresponding to the SST anomalies near Alaska.
The ocean-atmosphere variability patterns described briefly here (ENSO, NAO, PNA, AMV, PDV) all produce impacts to temperature, precipitation, and a variety of other atmospheric and environmental variables such as prevailing winds and runoff/streamflow at varying locations and on a variety of time scales. Moreover, the changing influence of a teleconnection on local conditions (both across space and time), or non-stationarity, is an important influence (Stenseth et al., 2003). The variability patterns themselves can interact, increasing the complexity and capability for prediction, with compound impacts of multiple teleconnections (Swain et al., 2017) that can amplify or attenuate the signal. For example, Newman et al. (2003) described the relationship between ENSO and the PDO, and showed that the NPO-like atmospheric SLP signal is weaker than the oceanic signature in the form of ENSO- and PDO-related SSTs. Chiang and Vimont (2004) reported evidence of an interannual to decadal association in SST anomalies between the tropical Atlantic and Pacific near the ITCZ, which is linked to both ENSO and the NAO. Budikova (2005) found that AO-temperature relationship is modulated by the PDO. Mokhov and Smirnov (2006) described the nature of ENSO forcing of the NAO since the mid-20th century. Dong et al. (2018) showed the complicated influence of the IPO on ENSO- and PNA-pattern- related weather variability. Zhang and Delworth (2007) found that the AMV could contribute to variability in the PNA and the PDO and other similar Pacific patterns, independent of ENSO variability. Wu et al. (2011) concurred with the connection between the AMV and PDO, with PDO leading the AMV by 1 year. Most recently, Power et al. (2021) echoed that ENSO governs some, but certainly not all internal tropical Pacific decadal climate variability and change, as subtropical-tropical cells in the upper-ocean overturning circulation along with SST variability beyond the tropical Pacific are also important. However, even compounded and synergistic effects such as these may differ by teleconnection phase (e.g., Straus and Shukla, 2002). Assessment of the influence on complicated biological effects might be best characterized by the simultaneous influences of multiple teleconnections (Stenseth et al., 2003).
Teleconnections and the south-central United States
In recent years, the increased attention to the impacts of such ocean-atmosphere variability in environmental, economic, and social systems, especially at the regional and local scale (e.g., Steptoe et al., 2018), have made teleconnections an important part of our understanding of the climate system. One region influenced directly throughout natural and social systems is the south-central United States (SCUSA). Hopkinson et al. (2013) suggested that changes in hydrology during the coming century may be the most important impact of climate change on natural systems in the southeastern United States, which includes the eastern part of the SCUSA as defined here.
The SCUSA is an appropriate area of focus because of its expanding populations, diverse ecosystems, important agricultural and other economic sectors, significant potential for wind and solar energy generation, and geographic diversity (both highlands and lowlands, continental and coastal areas, arid West and humid East, water scarcity and water richness). The region also sits at the boundary of influences from the Pacific, Atlantic, and Gulf of Mexico, straddling the ecotone between humid and arid climates in the United States, with interplay between subtropical and extratropical circulation features and the influences of the Pacific and Atlantic Oceans and the adjacent Gulf of Mexico. These multiple influences lead to a lack of a dominant pattern of variability (e.g., ENSO, AMV), with projections for warming and drought looming (Naumann et al., 2018). This complicated environmental geography calls for the need to understand the climate variability in the SCUSA, particularly in light of the cascading impacts of these environmental influences through ecological and social systems.
The SCUSA is an understudied region vis-à-vis atmospheric variability and change that contains wide disparities in social vulnerability to environmental hazards (e.g., Mihunov et al., 2018). The SCUSA has a large proportion of vulnerable populations—in urban, rural, and borderlands, as well as indigenous nations, especially in Oklahoma but also in Louisiana (National Conference of State Legislatures, 2018), including the Biloxi-Chitimacha-Choctaw on Isle de Jean Charles. Moreover, despite its diverse ecoregions and lack of cohesiveness as a distinct hydroregion (Dethier et al., 2020), the region has relatively tight economic cohesiveness (Ó’hUallacháin, 2008), with hubs in Houston and the Dallas-Fort Worth metroplex, and major increases in urbanization at the expense of agricultural lands being forecasted (Ahn et al., 2002; Alig et al., 2004).
This research will focus on some important causes and effects of climate variability, specifically focusing on the SCUSA, but independently of that put forth in the Intergovernmental Panel on Climate Change (IPCC) Fifth and Sixth Assessment Reports (IPCC, 2014; IPCC, 2022). de Chazal and Rounsevell (2009) noted the importance of integrated analysis of biodiversity, land-use change, and climate change. Improvements in the understanding of linkages and feedbacks across the climate system in the SCUSA would provide tangible benefits, such as in agriculture (Klemm and McPherson, 2018) and fisheries management (Karnauskas et al., 2015).
Data analysis and methodology
One challenge to understanding teleconnection patterns and their relative importance across the SCUSA is a lack of consistency in prior work between data sets and methods used. Thus, a uniform set of analyses of the relationship between climate modes (ENSO, PNA, NAO, AO, AMV, and PDO) and temperature and precipitation in each meteorological season—December-January-February (DJF), March-April-May (MAM), June-July-August (JJA), and September-October-November (SON)—is undertaken, using a consistent method and data set.
Each mode of climate variability (ENSO, PNA, NAO, AO, AMV, and PDO) is identified using a commonly-defined statistically standardized index, aggregated to monthly resolution, and displayed in Figure 2. To measure ENSO, the Niño 3.4 index (SST anomaly in the region bounded by 5°N to 5°S, from 170°W to 120°W), with values estimated back to 1877 (Bunge and Clarke, 2009) and 1856 (Kaplan et al., 1998), was selected over the SLP-based Southern Oscillation Index (Ropelewski and Jones, 1987), Multivariate ENSO index (Wolter and Timlin, 2011), or another indicator, owing to its direct reliance on SST in the central and Eastern Pacific (Trenberth, 1997; Trenberth and Stepaniak, 2001). Positive values correspond to the El Niño phase, with negative indices representing La Niña. The NAO was identified using the station-based NAO index (Hurrell, 1995), which measures the strength of the NAO as the difference between SLP in Portugal and Iceland. The PNA index (Barnston and Livezey, 1987) uses principal components analysis of SLP across the Northern Hemisphere. The AMV index (Enfield et al., 2001) uses SST across the North Atlantic, and the PDO index (Hamamoto and Yasuda, 2021) is constructed using principal components analysis of SST across the North Pacific. Tree-ring-based reconstructions of the AMO (e.g., Wang et al., 2011) and PDO (e.g., D'Arrigo et al., 2001) have also been used to extend the record by hundreds of years. Statistically standardized time series of the indices are shown in Figure 2.
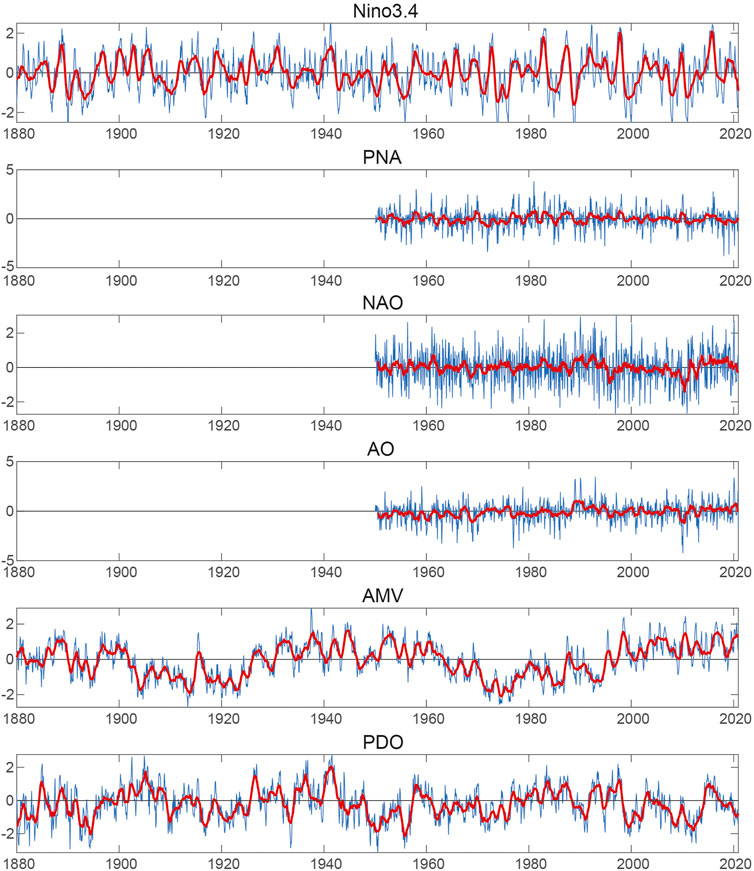
FIGURE 2. Time series of annual standardized climate indices for available periods of record (blue lines are monthly values, red are 13-month moving averages). NAO and AO indices are from the Climate Prediction Center; AMV and PDO indices are determined using the Extended Reconstructed Sea Surface Temperature (ERSST) data set version 5 (Huang et al., 2017). Source: https://climexp.knmi.nl/.
Monthly 2-m temperature and precipitation fields (preliminary/provisional from 1950 to 1978 and combined for 1979–2020) from the fifth-generation European Centre for Medium-range Weather Forecasts (ECMWF) Reanalysis (ERA5; Copernicus Climate Change Service, 2017) are aggregated into seasonal means (i.e., DJF, MAM, JJA, and SON) and correlated (separately) with each teleconnection index, for the 1950–2020 period. ERA5 is a reanalysis product that combines in-situ observations and numerical modeling and is available at 31-km horizontal resolution (Hersbach et al., 2020). Reanalysis products are typically used as gridded observations for synoptic- and large-scale atmospheric circulation and are adequate for pattern identification used herein. Each variable is linearly detrended at each grid point, and anomalies are calculated by removing the average monthly climatology. One area of caution in the interpretation of results is that current gridded data sets tend to suppress precipitation extremes in the SCUSA (Sun et al., 2019).
The relationship between each pattern of variability (e.g., ENSO) and each atmospheric variable (i.e., temperature or precipitation) is assessed for each season, such that a correlation analysis is conducted between the annual ENSO index time series and the (say) DJF temperature at each grid point across the continental U.S., although the focus here is on results for the SCUSA. DJF values were assigned to the year of their January-February components. Statistical significance at the 95 percent confidence interval is assessed by a two-tailed Student’s t-test, taking into account serial correlation (Bretherton et al., 1999).
Impacts of enso in the south-central United States
The literature suggests that, while every ENSO event is different (Capotondi et al., 2015), ENSO generally has more prominent and well-understood impacts on the SCUSA than other climate modes investigated. Danco and Martin (2018) observed the influence of ENSO on the low-level jet stream that advects heat and moisture through the SCUSA toward the Great Plains. ENSO has been shown to have a predictable influence on both temperature and precipitation in the SCUSA (Wang and Robertson, 2019), although Zhou et al. (2016) cautioned that prediction of ENSO-induced precipitation tends to underemphasize extremes substantially. The El Niño phase has been linked to positive cold-season geopotential height anomalies in the southeastern United States (Horel and Wallace, 1981) and negative cold-season temperature departures across the United States. Gulf Coast and northern Mexico, especially in southwestern Texas and adjacent northern Mexico (Hurrell, 1996; Torbenson et al., 2019). EL Niño events have also been found to be generally associated with positive precipitation anomalies throughout the same area (Ropelewski and Halpert, 1986; Ropelewski and Halpert, 1987; Wise et al., 2015; Torbenson et al., 2019), but with significant clustering of precipitation “hotspots” over the Gulf of Mexico (Munroe et al., 2014). Summertime El Niño conditions also increase precipitation in the subsequent winter with La Niña summers suppressing the upcoming winter’s precipitation in much of the SCUSA (Kurtzman and Scanlon, 2007). The La Niña phase is linked less conclusively to temperature and precipitation in the same area, but with tendencies for negative cold-season precipitation anomalies (Ropelewski and Halpert, 1989, 1996; Munroe et al., 2014).
Multiple causes of these anomalies have been noted. Vega et al. (1998) noted the importance of displacement of mid-tropospheric ridging near the SCUSA that supports ridging during La Niña events. Eichler and Higgins (2006) linked precipitation anomalies to displacements in storm tracks in North America and surrounding ocean environments. Sweet and Zervas (2011) emphasized the influence of an anomalously strong subtropical jet stream, which advects moisture (Sanchez-Rubio et al., 2011) that had been displaced eastward in the tropical Pacific Ocean during the El Niño event, with weakening of the subtropical jet and decreased cyclogenesis in the southeastern United States and SCUSA during La Niña events (Ropelewski and Halpert, 1986; Schmidt et al., 2001; McCabe and Muller, 2002). Bove et al. (1998) and Pielke and Landsea (1999) noted the decreases in upper-level vertical wind shear in the main hurricane development regions during La Niña events and increases during El Niño events, which can partially offset the “typical” anomalies during the early part of the cold season by increasing storm frequency during La Niña conditions and reducing storm frequency during El Niño conditions (Gray, 1984; Shapiro, 1987). The precipitation signature of ENSO is likely to be most important in the northern part of the SCUSA and the association with hurricanes is likely most influential in parts of the SCUSA where tropical cyclones represent an important part of the precipitation climatology, such as south coastal Texas and Louisiana, at the time of year when tropical cyclones may be a factor. However, ENSO’s influence on tropical cyclone frequencies for the southeastern United States. Gulf of Mexico coast is likely to be reduced compared to that on the United States. Atlantic Coast (Smith et al., 2007).
Wide spatial and temporal variability in ENSO-forced temperature and precipitation anomalies are to be expected in the SCUSA as elsewhere (Deser et al., 2018), with the magnitude and even the sign of the winter temperature (Yu et al., 2012a) and precipitation anomalies affected by the location of the Pacific warm and cold pools during El Niño events (Zhang et al., 2012). Whereas conventional El Niño events are characterized by warming in the eastern tropical Pacific Ocean, the so-called El Niño Modoki events (Ashok et al., 2007), also known as central-Pacific El Niño events (Kao and Yu, 2009), have anomalously warm SSTs in the central Pacific Ocean flanked by cooler SST to the east and west. As Atlantic tropical cyclone landfall frequencies are suppressed less during Modoki events than during conventional El Niño events on the Gulf of Mexico coast and Central America (Kim et al., 2009), precipitation anomalies in the SCUSA may depend on the type of El Niño event. The diverging hydrometeorological responses by type of ENSO event (i.e., “conventional” vs. Modoki, or east-Pacific vs. central-Pacific), such as in above vs. below-normal spring Mississippi River basin precipitation, soil water hydrology, and streamflow (Liang et al., 2014), requires careful attention to the type of ENSO event to assess the impact. Flanagan et al. (2019) noticed the importance of central Pacific SSTs for producing precipitation anomalies in the southern Great Plains, including parts of the SCUSA. Nevertheless, “conventional” ENSO events tend to have a greater overall influence than Modoki events for the tropical Atlantic Ocean (Taschetto et al., 2016) westward in the SCUSA (Yu et al., 2017). Other studies have found that other weather phenomena across the United States respond to extreme ENSO phases, such as tornadoes being more frequent and intense in the SCUSA during winter and early spring (Cook et al., 2017). The observation of increased prevalence of Modoki El Niño at the expense of “conventional” or eastern Pacific El Niño (Yu et al., 2012b; Liang et al., 2015) may have implications for future ENSO impacts in the SCUSA.
Results, based on ERA5 for the 1950–2020 period, illustrate the relatively strong but complex spatial and seasonal differences in ENSO influence on temperature and precipitation in the SCUSA. The most prominent temperature relationship in the SCUSA is in Texas in spring, when anomalously cold conditions occur in the El Niño events and warm conditions occur in La Niña events (Figure 3). Precipitation anomalies are also most prominent in Texas during the spring, with El Niño events associated with anomalously wet conditions and La Niña with drier-than-normal springs (Figure 4). Our results of generally wet anomalies during El Niño events in SON (Figure 4) align with recent research that suggests a temporally decreasing influence of El Niño events on southeastern United States precipitation (Mo, 2010) and increasing autumn precipitation anomalies in the eastern SCUSA and adjacent Mississippi and Alabama related to El Niño events (Bishop et al., 2019).
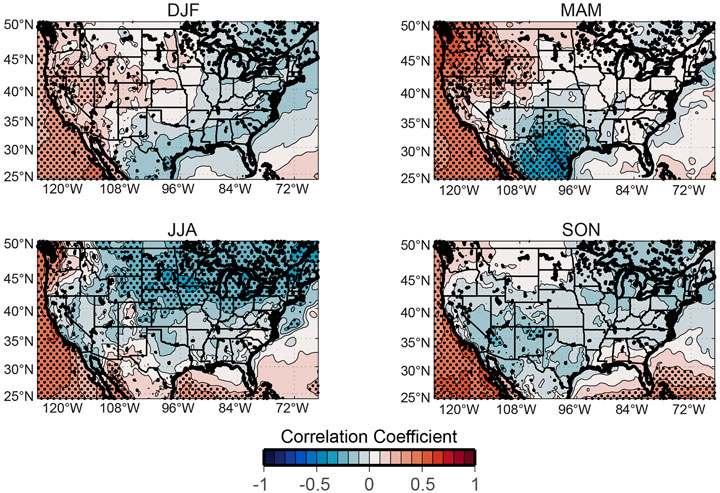
FIGURE 3. Correlation coefficients between the Niño 3.4 index and seasonal temperature across the continental United States (1950–2020); stippling indicates statistical significance (p < 0.05).
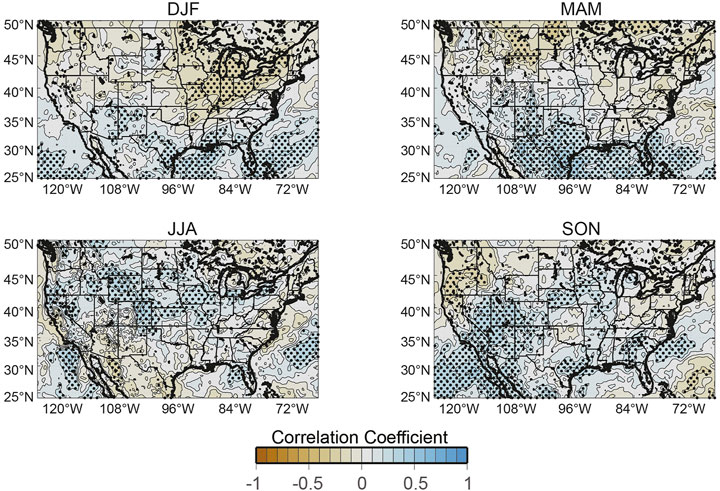
FIGURE 4. As in Figure 3, but for precipitation.
The Great Plains low-level jet (GPLLJ), the Caribbean low-level jet (CLLJ), and extratropical wave trains America (Krishnamurthy et al., 2015) likely force the broader signals in the SCUSA during spring. Analysis of reanalyzes/observations demonstrate that ENSO has a significant impact on the strength of the GPLLJ, with a significant negative correlation in the spring and a significant positive correlation in summer (Schubert et al., 2004; Weaver et al., 2009; Muñoz and Enfield, 2011; Krishnamurthy et al., 2015; Danco and Martin, 2018). Muñoz and Enfield (2011) discovered that colder SSTs in the Niño 3.4 region often result in a stronger GPLLJ in spring. Observations indicate wintertime La Niña induces high SLP anomalies over the Intra-Americas Sea the following spring through changes in the Walker and Hadley circulation. This leads to a strong CLLJ, which drives a stronger GPLLJ, with the GPLLJ and CLLJ significantly correlated in spring (Martin and Schumacher, 2011a). Lee et al. (2013) found that in April and May, cold SST anomalies in the Niño 4 region and warm SST anomalies in the Niño 1+2 region work together to result in increased moisture transport from the Gulf of Mexico, which could imply a stronger GPLLJ. Another study presented a similar pattern in the summer, with a Modoki (i.e., Central Pacific) El Niño weakening the GPLLJ and a conventional (i.e., East Pacific) El Niño strengthening it (Liang et al., 2015).
Going beyond traditional associations between ENSO vs. temperature and vs. precipitation, cascading socio-ecological connections to ENSO are also important in the SCUSA. For example, ENSO is an important control over “greenness,” as represented by the Enhanced Vegetation Index, and explains 10 to 25 percent of variability in greenness in south-central Texas and adjacent western
Texas and eastern New Mexico (Swain et al., 2017). Mishra et al. (2011) and Konapala et al. (2018) identified linkages between ENSO and streamflow variability in Texas, with the latter finding strong but spatially and temporally inconsistent associations between El Niño events and drought in the subsequent summer, particularly in southern Texas (Rajagopalan et al., 2000). The Texas and Oklahoma floods of May 2015 have also been linked to El Niño events (Wang et al., 2015), and periodic Texas drought in the pre-instrumental period in the region have also been noted (Stahle and Cleaveland, 1988); such variability is likely associated with ENSO and potentially other teleconnections. El Niño events also lead to warmer SST in the Gulf of Mexico and Caribbean Sea (see Figure 1A) that can drive coral bleaching events that can impact fisheries in the northern Gulf of Mexico (Schmidt and Luther, 2002; Tolan, 2007; Piazza et al., 2010; Gomez et al., 2019).
ENSO has numerous cascading impacts on atmospheric circulation (Rasmusson and Carpenter, 1982) and precipitation-dependent human activities in the SCUSA. For example, the linkage of El Niño events to anomalously weak surface winds, and therefore reduced ocean waves along the major shipping routes in the northernmost Pacific Ocean (Chen et al., 2012), impacts North America including the SCUSA, particularly considering the temporally increasing volume of trade with Asia. ENSO-forced salinity fluctuations in Louisiana estuaries contribute to decreased brown shrimp abundance following El Niño events (Piazza et al., 2010). The SCUSA will undoubtedly be impacted if recent research (Cai et al., 2021) suggesting a widening ENSO-induced SST variability and therefore enhanced warm-phase-ENSO warming, along with an eastward shift and intensification of the ENSO-related PNA pattern and the Pacific-South American patterns, is realized.
The future of ENSO is far from certain, as some recent work suggests more extreme El Niño and La Niña (e.g., Cai et al., 2015; Liu et al., 2021) events, while other research (e.g., Callahan et al., 2021) suggests that CO2 forcing will dampen ENSO events. Nevertheless, any future changes in the dominance of conventional vs. Modoki (or central-Pacific) El Niño events are likely to affect SCUSA temperatures, particularly in the southeastern region (Yu et al., 2012a). Modoki have been projected to become more common in the future relative to the more conventional El Niño-defined SST anomalies (Yeh et al., 2009), perhaps because of the effect of weakened equatorial easterlies that in turn flatten the eastern tropical Pacific’s thermocline (Ashok et al., 2007).
Impacts of the PNA pattern in the south-central United States
The PNA pattern has a substantial influence on both temperature and precipitation in the SCUSA (Wang and Robertson, 2019). Specifically, during the PNA positive phase, the SCUSA has been found to be anomalously cool (Hurrell, 1996) in all months except summer, when the PNA pattern is poorly defined (Leathers et al., 1991). Hardy and Henderson (2003) noted the proclivity for more frequent cold frontal passages in western Texas during the negative PNA pattern, thereby having implications on both temperature and precipitation. Dong et al. (2011) linked the PNA pattern causatively to cyclonic activity in the southwestern United States including much of the SCUSA, but they cautioned that the West Pacific teleconnection (Wallace and Gutzler, 1981; Barnston and Livezey, 1987) may provide an even more direct association. Somewhat wetter than normal conditions in the coastal SCUSA have also been found to occur during the positive mode (Wang and Robertson, 2019), although Leathers et al. (1991) had found only weak associations. Liu et al. (2014) observed a generally negative correlation between the winter PNA pattern and the oxygen isotope ratio (δ18O) of winter precipitation (including in nearly all of the SCUSA), showing the influence of moisture source and storm tracks on winter precipitation δ18O.
The ERA5-based analysis using the extended study period largely confirms results from the existing literature regarding the PNA-temperature relationship in the SCUSA, with generally negative correlations (i.e., anomalously low temperatures accompanying the positive PNA pattern, and vice versa) in meteorological winter and (especially) spring, and weak correlations in meteorological summer and autumn (Figure 5). Specifically, except for the extreme northern and western sectors, a negative relationship exists in meteorological winter (DJF) in the SCUSA, with the coastal southeastern corner following the pattern throughout much of the rest of the southeastern United States of significant negative correlations. By spring, the slight negative correlation in extreme northern and western SCUSA from winter is reversed, and nearly the entire SCUSA has significant negative correlation between PNA pattern and temperature. In summer, the pattern deteriorates, with the zero correlation line bisecting the SCUSA into western (negative) and eastern (positive) zones; only northeastern Arkansas joins much of the adjacent southeastern United States in displaying significant positive correlations. By autumn, even weaker relationships are found, not only across the SCUSA but also across the eastern two-thirds of the United States.
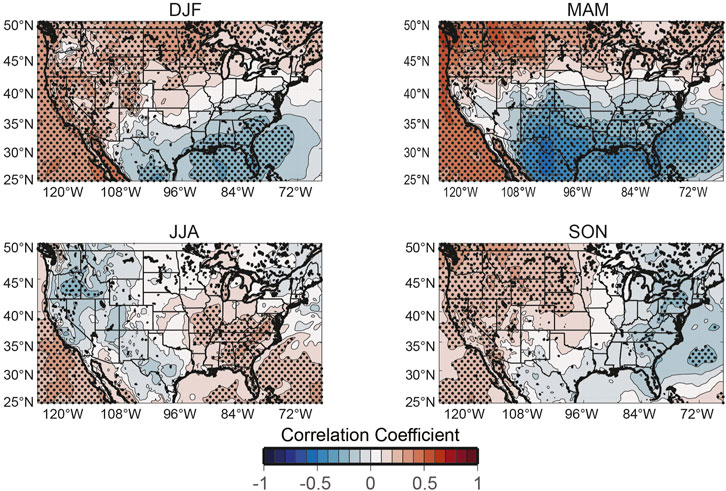
FIGURE 5. Correlation coefficients between the PNA pattern index and seasonal temperature across the continental United States (1950–2020); stippling indicates statistical significance (p < 0.05).
The PNA-precipitation spatial pattern in the SCUSA in the ERA5 for the extended period of years (1950–2020) is also largely similar to that described previously in the literature. Specifically, in winter and spring, relatively strong, significant positive correlations occur in much of the western and coastal SCUSA and adjacent Gulf of Mexico (Figure 6). A substantial zone of significant negative correlations exists to the northeast of the SCUSA, with its southwest-northeast orientation suggesting that the trough-to-ridge side of the Rossby wave characteristic of a negative PNA pattern brings anomalously wet conditions to the Ohio and Tennessee Valleys. However, that zone shrinks and moves eastward from winter to spring. In summer, the area of significant positive correlations in the SCUSA is largely confined to south-central Texas, perhaps due to the increase in albeit very weak cold frontal passages (Hardy and Henderson, 2003), with the zone of significant negative correlations
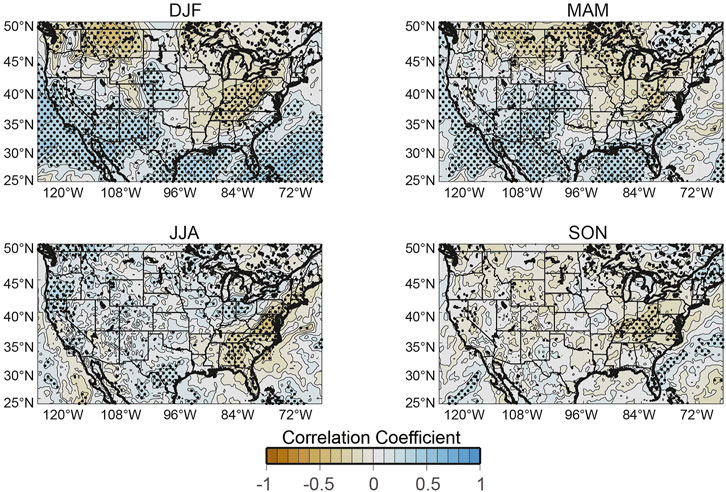
FIGURE 6. As in Figure 5, but for precipitation.
(i.e., PNA-induced trough leading to abundant precipitation) pushed well to the east of the SCUSA. In autumn, no areas of significance correlations are found in the SCUSA, with only isolated pockets of significant correlations elsewhere in the United States, such as over the Ohio and Tennessee valley and coastal Virginia and North Carolina (positive) and peninsular Florida and northern New England (negative).
Variability in the meridionality (i.e., north-to-south or south-to-north flowing) of the PNA-defined ridge-trough configuration, including both the temperature and precipitation anomalies shown here and previously in the literature, in turn contribute to fluctuations in other environmental and human systems in the SCUSA. For example, Rogers and Rohli (1991) and Rohli and Rogers (1993) linked PNA-induced variability to economic impacts to agriculture, including in the SCUSA. The PNA pattern has also been shown to have an important control over “greenness” in the western SCUSA, with particular importance in New Mexico and the Texas High Plains (Swain et al., 2017). Based on the relationships between the PNA pattern and temperature/precipitation shown here, many other primary and secondary impacts of the PNA pattern are likely to exist in the SCUSA. However, relationships between the PNA pattern and natural and human systems seem to be documented more thoroughly elsewhere, such as in the Great Lakes region (e.g., Rodionov and Assel, 2003; Yu et al., 2014).
Impacts of the NAO and NAM (AO) in the south-central United States
The seesaw in pressure anomalies between the northern and subtropical North Atlantic associated with the NAO produces a chain reaction of physical responses. The NAO contributes most dominantly to winter temperatures across much of the Northern Hemisphere (Barnston and Livezey, 1987; Hurrell, 1996). A northward displacement of the polar front jet stream during the NAO’s positive (i.e., “warm” or “high”) phase (Visbeck et al., 2001) generally contributes to anomalously warm and wet conditions in the eastern United States (Tootle et al., 2005), but with warm (Hurrell, 1996) and varied precipitation conditions over the SCUSA (Ning and Bradley, 2016; Wang and Robertson, 2019). Such conditions and their impacts are generally amplified in the cold season (Folland et al., 2009). Consistent with colder conditions in the negative (i.e., “cold” or “low”) phase of the NAO, Hardy and Henderson (2003) attributed their finding of increased cold frontal passages during the negative phase of the NAO in much of the SCUSA to troughing over the region and southerly displacement of the mid-latitude cyclone tracks. Negative phases of the NAO have also been shown to coincide with increased probability of cold air outbreaks across the United States (e.g., Walsh et al., 2001; Cellitti et al., 2006). Non-stationarity associated with the eastward shift of the NAO, particularly in summer (Sun and Wang, 2012), has likely been associated with changes in its influence over the SCUSA, and, similar to other teleconnections, temporally changing environmental, economic, and social impacts.
The inclusion of the ERA5 data over a longer period of time generally suggests that the correlation between temperature and the NAO index is typically insignificant in the SCUSA in all seasons. During winter, the correlation is positive across the majority of the United States, with areas of significance in the southeast and adjacent to and in the Gulf of Mexico, although not extending into the SCUSA (Figure 7). The implication is that zonal (i.e., west-east) flow hinders the meridional transport of Arctic and polar air masses into the United States. In MAM, areas of significant positive correlations include the Gulf of Mexico and adjacent peninsular Florida, and much of the West, with simultaneous negative correlations across the Northeast and adjacent Canada, but no significant areas in the SCUSA (Figure 7). In summer, correlations are negative, but insignificant across the SCUSA, excepting significant negative correlations in Arkansas and down the Mississippi River valley into Louisiana, extending from the Ohio River Valley, with insignificant correlations between temperature and the NAO in the SCUSA and most of the rest of the United States during SON (Figure 7).
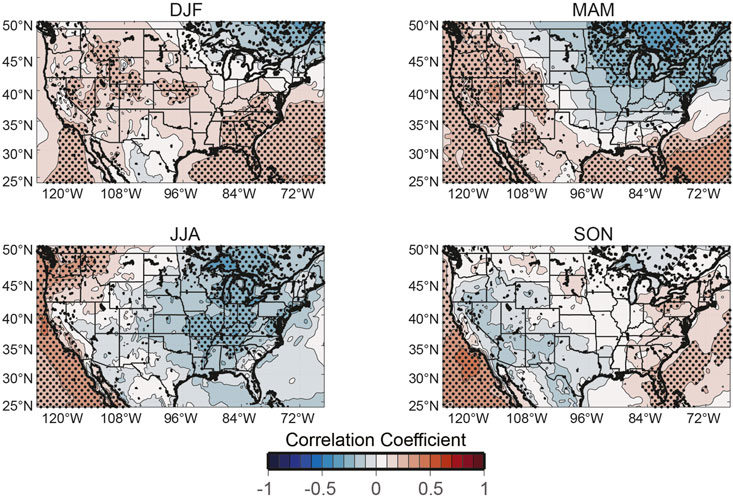
FIGURE 7. Correlation coefficients between the NAO index and seasonal temperature across the continental United States (1950–2020); stippling indicates statistical significance (p < 0.05).
The literature suggests that largest and most significant precipitation anomalies associated with the NAO tend to occur along the North Atlantic storm tracks, the Midwest, northeastern United States, and Europe (e.g., Bradbury et al., 2003; Weaver and Nigam, 2008). For example, strong correlations have been observed between central United States precipitation and the NAO index due to the influence of the GPLLJ in the summer. Ruiz-Barradas and Nigam (2005) and Weaver and Nigam (2008) found that negative phases of the NAO coincided with anomalously strong influx of moist Gulf of Mexico air into the United States interior and unseasonably high precipitation in the Midwest. Villarini et al. (2013) also found that in Iowa, the magnitude of the NAO index was a useful predictor in determining the occurrence of floods. However, because the positive NAO is tied to a strengthening of the North Atlantic Subtropical High, also known as the Bermuda-Azores anticyclone (BAA), which strengthens the northeast trade winds and promotes evaporation in the Caribbean Sea and Gulf of Mexico, with the opposite effects during the negative NAO phase (Kapala et al., 1998; Mächel et al., 1998), the effect of the NAO on the SCUSA is opposite to that farther north. Specifically, the approach of the trade winds to the northwestern Gulf of Mexico, after turning northward, advects increased moisture to the SCUSA during the positive NAO phase and reduced moisture during the negative NAO, thereby contributing to the precipitation anomalies in that area (Oglesby and Erickson, 1989).
The correlations in ERA5 between precipitation and the NAO index are shown in Figure 8, which illustrates the generally weak relationships in the SCUSA, as with temperature (Figure 7). The most extensive areas of significant correlations in the SCUSA occur in spring, when much of Oklahoma and Texas southward along the Texas-Louisiana border and adjacent coastal areas show a positive association between precipitation and the NAO index (Figure 8). In spring, zonality in the NAO is linked with above-normal precipitation, and meridionality brings drier air southward, suppressing precipitation totals, in these areas.
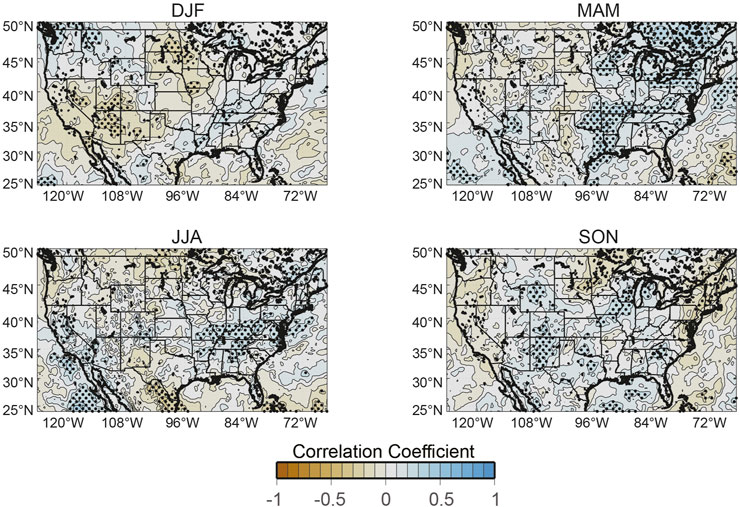
FIGURE 8. As in Figure 7, but for precipitation.
These positive correlations are consistent with the prior studies showing increased streamflow in the Midwest and into the Lower Mississippi River basin during the positive NAO phase. Some negative correlations occur in the westernmost SCUSA in DJF and the Texas-Mexico border in JJA (Figure 8). The NAO’s positive phase is linked to significantly greater streamflow than its negative phase in eastern Texas and western Louisiana (middle Mississippi River basin), which aligns prominently with the Ohio, Missouri, and Upper Mississippi basin (Tootle et al., 2005), thereby affecting Lower Mississippi stream discharge. Coleman and Budikova (2010) found that the significant 1993 and 2008 Midwest flood events were both associated with a positive NAO phase preceding the events, but the NAO was in a negative phase during the flooding events. The NAO is also known to alter the effects of ENSO on tropical cyclone activity by modulating SLP associated with the BAA and associated vertical wind shear (Lim et al., 2016).
Impacts of the AMV in the south-central United States
Previous research has identified linkages between the AMV and SCUSA temperatures, through the positive correlation between tropical Atlantic SST and high cloud cover, the latter of which is indicative of convection (Vaideanu et al., 2018). This cloud-cover connection is corroborated by the observation of positive correlations between minimum daily temperatures and the AMV in much of North America, including the SCUSA (Gan et al., 2019).
However, more emphasis has been placed on understanding the hydrometeorological and hydrological impacts of the AMV in the SCUSA. The AMV has been tied to precipitation variability in the United States primarily through its influence on the position and intensity of the BAA. A warm-phase AMV tends to weaken the BAA, which reduces low-level “back of high” moisture advection into the SCUSA (Kushnir et al., 2010). The relationship between tropical cyclone frequency and broad-scale teleconnections seems to be most robust in the Atlantic, particularly regarding the AMV at the interannual to decadal scales (Vimont and Kossin, 2007). The most complete picture is provided through the synergistic effects of ENSO and the AMV (Mo et al., 2009), as the combination of La Niña and AMV warm phase supports Atlantic-Gulf tropical cyclone activity and accumulated cyclone energy (Patricola et al., 2014). A paleoclimate reconstruction from a coral in eastern Caribbean suggests connection between AMV and tropical cyclone activity for 1923–1998 (Hetzinger et al., 2008). Other coral-based reconstructions find strong correlation with AMV and temperature proxies that extend this record back to 1751 (Kilbourne et al., 2008) and central Caribbean coral hydroclimate reconstruction varies with the AMV back to 1887 (von Reumont et al., 2018). However, Gulf of Mexico and Bahamian coral-based reconstructions suggest mixed results with no direct AMO relationship (DeLong et al., 2014) to coral-SST lagging the AMO by 6–9 years with a decreased relationship before ∼1800 (Saenger et al., 2009; Flannery et al., 2017). Sea surface temperature in the Caribbean Sea and the Gulf of Mexico plays a vital role in weather, hydroclimate, and extreme events in the SCUSA (Wang et al., 2006, 2008a, 2008b; Martin and Schumacher, 2011a, 2011b, 2012; Misra et al., 2014).
The positive mode of the AMV also appears to favor drought in the SCUSA (Enfield et al., 2001; Rogers and Coleman, 2003; McCabe et al., 2004; Mo et al., 2009). More recently, Torbenson and Stahle (2018) confirmed this relationship using tree-ring reconstructions by finding that the positive (warm) AMV phase is strongly associated with central United States negative precipitation anomalies in autumn (confirmed by Knight et al., 2006), and also in spring and summer (confirmed by Nigam et al., 2011), and with negative streamflow anomalies along the northern and western Gulf of Mexico coast (Tootle et al., 2005). Cook et al. (2014) found that while drought in the SCUSA is positively correlated to the AMV index, drought in the westernmost SCUSA is linked more strongly to La Niña events. The mechanism responsible for such anomalies is the diversion of westward trade wind-associated moisture flow southward of the Gulf of Mexico (Méndez and Magaña, 2010). Perhaps the most striking example of impacts from the AMV on SCUSA drought is the evidence from a tree-ring reconstruction of the 1838 drought, which is likely to have contributed to many deaths during the so-called Trail of Tears (Torbenson and Stahle, 2018). Recent work supports these general findings, as long-term future decreases in streamflow associated with the AMV is projected for the southeastern United States, including Louisiana (Sadeghi et al., 2019), although synergistic impacts between the AMV and ENSO must also be considered (Torbenson et al., 2019).
Results from ERA5 analysis on the extended study period confirm a statistically significant positive relationship between the AMV index and the SCUSA temperature for most of the year (Figure 9), particularly summer and autumn, and weak, insignificant correlations in spring. When combined with results from the previous studies, it appears likely that Atlantic tropical cyclone season would be impacted strongly by AMV-related variability through its impacts on Gulf of Mexico SSTs (Poore et al., 2009). Other paleoclimate-based research has suggested that cool North Atlantic SSTs, El Niño-like conditions, and the negative phase of the NAO were associated with fewer hurricanes during the Maunder Minimum (Trouet et al., 2016). AMV-precipitation relationships, on the other hand, are weaker in the SCUSA, with negative correlations strongest in autumn in the interior SCUSA (Figure 10) when Gulf of Mexico and Caribbean SSTs peak. Perhaps the coastal areas display the weaker relationship due to the influence of tropical cyclones that are energized by the warmer waters in the summer and autumn months.
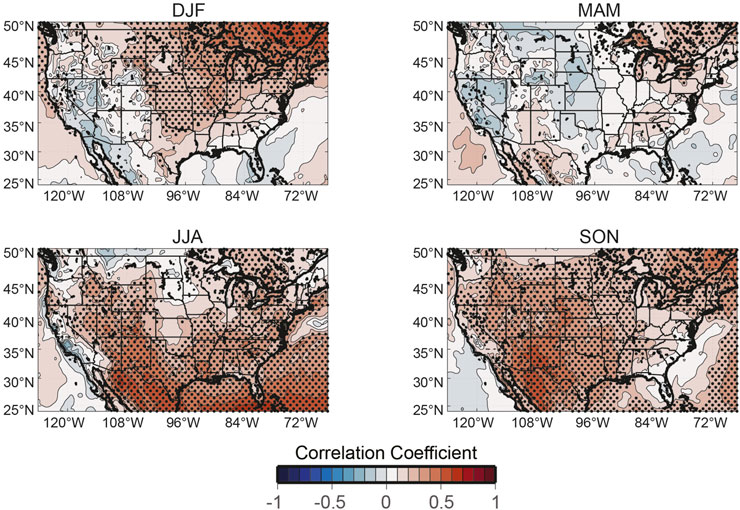
FIGURE 9. Correlation coefficients between the AMV index and seasonal temperature across the continental United States (1950–2020); stippling indicates statistical significance (p < 0.05).
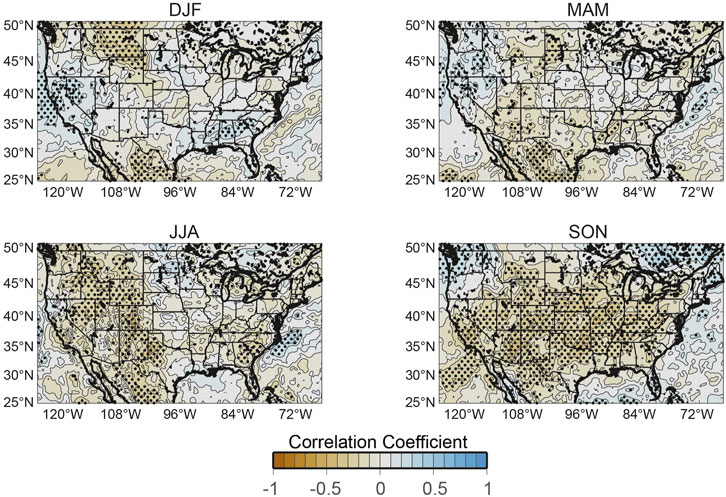
FIGURE 10. As in Figure 9, but for precipitation.
Impacts of the PDO in the south-central United States
The role of the PDO in the observed cooler period over the central United States, including the SCUSA, in the last quarter of the 20th century amid substantial warming elsewhere, has been noted (Kumar et al., 2013; Pan et al., 2013; Pan et al., 2017). Responses to PDO-related temperature variability in the SCUSA are stronger in winter and spring than summer and autumn, with a tendency for negative temperature anomalies (Figure 11), as represented by anomalously low geopotential height fields, during warm-phase PDO, and vice versa (Mills and Walsh, 2013). Winter precipitation (Kurtzman and Scanlon, 2007) and extreme precipitation (Zhang et al., 2010) have been shown to be greater in the SCUSA during the positive (warm) PDO phase than during the negative (cold) phase. The PDO-like IPO has also been linked to precipitation anomalies of opposing sign between China and the southwestern U.S., including the SCUSA, with particular amplification of the relationship when the AMV is in the opposite phase (Yang et al., 2019). McCabe et al. (2004) identified the linkage between cooling associated with the cold- (i.e., negative) phase PDO and drought across the conterminous United States, including the SCUSA. Ford et al. (2017) found that below-normal precipitation in the SCUSA is forced by a negative PDO, which had already been associated with a prolonged Medieval megadrought (MacDonald and Case, 2005), accompanied by a positive AMV. Barlow et al. (2001) recognized the synergistic influence of the cold phase of both ENSO and the PDO on the historic drought of the early to middle 1950s in the SCUSA. ERA5 analysis on the extended study period confirms the negative correlation between PDO phase and SCUSA temperature, with the relationship statistically significant across large swaths of the SCUSA in winter, spring, and summer and in the Gulf of Mexico in winter and spring months (Figure 11). Anomalously warm Pacific waters associated with the PDO produce positive precipitation anomalies (and vice versa) in the western and coastal SCUSA in all seasons and in the Gulf of Mexico in all seasons except autumn (Figure 12).
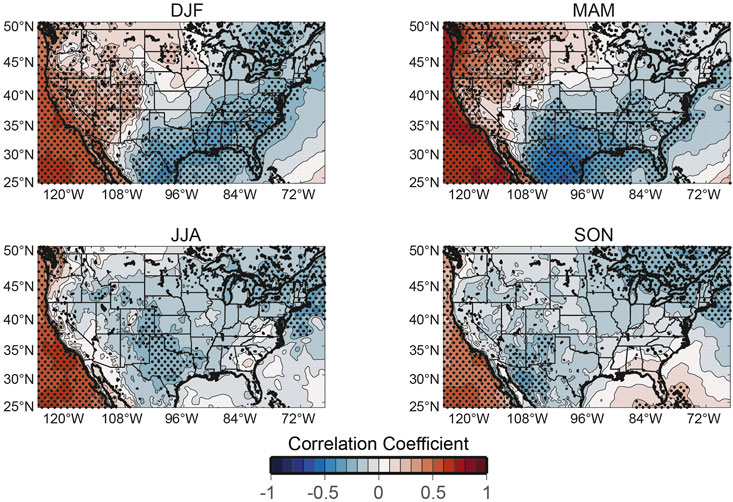
FIGURE 11. Correlation coefficients between the PDO index and seasonal temperature across the continental United States (1950–2020); stippling indicates statistical significance (p < 0.05).
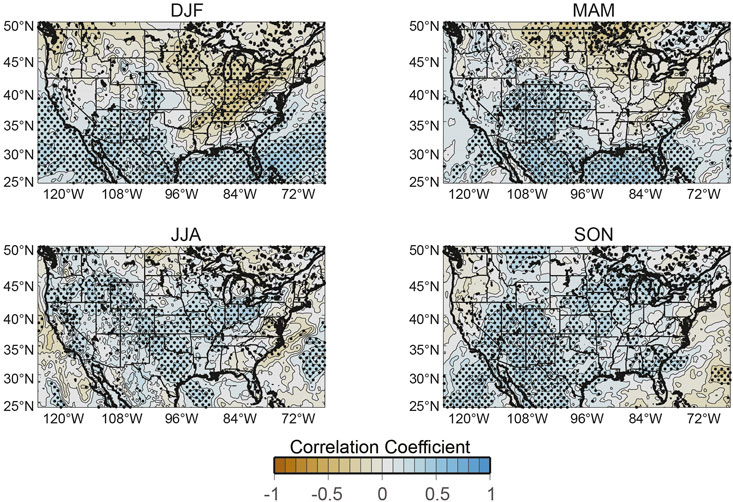
FIGURE 12. As in Figure 11, but for precipitation.
Tootle et al. (2005) linked the PDO’s warm phase with significantly enhanced streamflow along the eastern Texas and Louisiana coast, and in eastern Oklahoma, with Sagarika et al. (2015) and Rodgers et al. (2020) concurring with these findings in the SCUSA but with low explained variance (Mantua et al., 1997). Khedun et al. (2012) elaborated on the distinction between the PDO’s winter and spring influence (particularly when working in tandem with El Niño) on the upper vs. lower Rio Grande, thereby somewhat mitigating extremes in water availability. Pascolini-Campbell et al. (2017) showed the importance of the positive PDO (and negative AMV) as a driver of anomalously positive streamflow in the Upper Rio Grande basin.
Intraseasonal variability: The madden-julian oscillation
Whereas the focus of this research is on seasonal-scale modes of climate variability and their teleconnections, the intra-seasonal tropical Madden-Julian Oscillation (MJO; Zhang, 2005) deserves mention because of its proximity to and influence on the SCUSA and because teleconnections are now recognized to exert an untapped source of weather predictability at the intraseasonal scale (Stan et al., 2017). Also known in earlier literature as the 30–60-day Oscillation (e.g., Tokioka et al., 1988; Ferranti et al., 1990) or 40–50-day Oscillation (e.g., Madden and Julian, 1994), Roland Madden and Paul Julian discovered the oscillation when analyzing zonal wind anomalies in the tropical Pacific Ocean (Madden and Julian, 1971). The MJO is characterized by a geographically-propagating sequence of convective pulses as revealed by spatial and temporal fluctuations in outgoing longwave radiation (Figure 13). The location of MJO- induced convective bursts define the eight MJO phases, which are represented by an index developed by Wheeler and Hendon (2004) based on a pair of empirical orthogonal functions of the combined fields of near-equatorially averaged 850-hPa zonal wind, 200-hPa zonal wind, and satellite-observed outgoing longwave radiation. Phase 1 has relatively benign convection ubiquitously, while Phases 2 through 6 support vigorous convection in a west-to-east direction proceeding from the equatorial central Indian Ocean to New Guinea, and Phases 7 and 8 have milder convective clusters from the central to eastern equatorial Pacific.
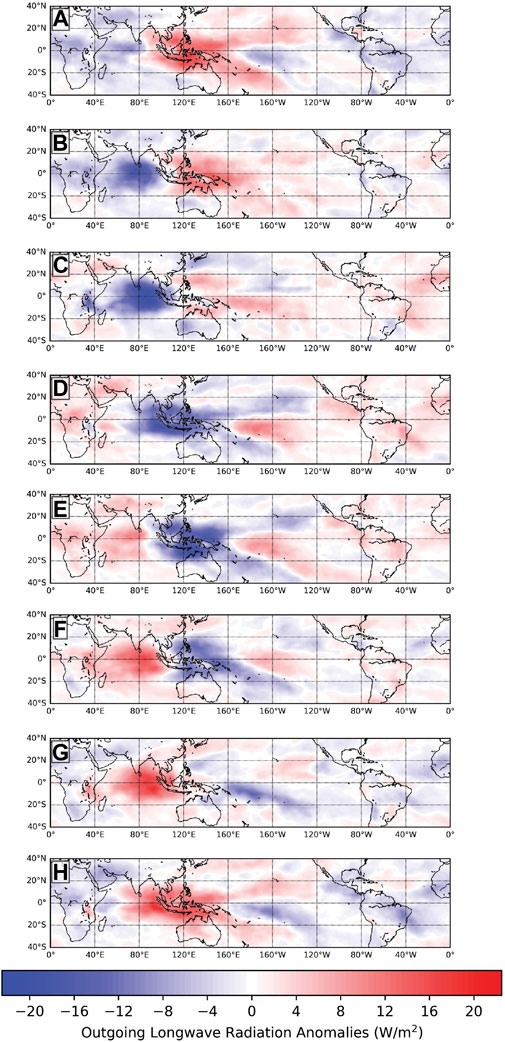
FIGURE 13. Outgoing longwave radiation anomalies by Madden-Julian Oscillation phase, using data from 1979 to 2014. Source: Caparotta (2018). (A–H) represent MJO Phase 1 through 8 respectively..
The MJO phase has been linked to extratropical atmospheric variability, forced largely by convection in the western tropical Pacific (Lukens et al., 2017) through the modulation of circulation, including through other teleconnections including ENSO (Lee et al., 2019), the PNA pattern (Schreck et al., 2013; Zhou et al., 2020; Toride and Hakim, 2021) and the NAO (Cassou, 2008; Lin et al., 2009). Moon et al. (2011) recognized the interplay between ENSO and MJO in modulating MJO impacts.
Regardless of the extent to which other teleconnections exacerbate or mitigate the effects, the MJO is known to be related to North American weather and climate through its impact on circulation. Research has related the MJO to variability in the jet stream (Barrett, 2019) and associated storm tracks (Grise et al., 2013; Zheng et al., 2018) and snowstorms (Moon et al., 2012), temperatures (Zhou et al., 2012), precipitation (Jones, 2000; Martin and Schumacher, 2011b), extreme precipitation (Jones et al., 2011), moisture in atmospheric rivers (Jones and Carvalho, 2014; Baggett et al., 2017), and tropical cyclones (Vitart, 2009) impacting North America. As periods of active MJO activity have been identified as important modes of cold-season circulation predictability in the Northern Hemisphere extratropics (Jones et al., 2004), it is not surprising that improvements in forecast skill for extreme precipitation were found with MJO active Phases 1, 2, 7, and 8, and in strong-magnitude cases of Phases 3, 4, 7, and 8 (Jones et al., 2011).
Somewhat less predictability for SCUSA temperature and precipitation is afforded by MJO phase than in most other areas of North America (Rodney et al., 2013). There is a suggestion of positive winter temperature anomalies in the SCUSA during Phase 4, and in the eastern SCUSA during Phase 6, and positive precipitation anomalies in the northern SCUSA during Phase 5 (Zhou et al., 2012), with Phases 4, 5, and 6 characterized by convective clusters that move across the Maritime Continent into the western tropical Pacific, respectively. Caparotta (2018) observed increased storminess in the Gulf of Mexico region during Phases 7, 8, and 1, which show relatively weak convective clusters, propagating from the central to eastern Pacific. Gulf of Mexico tropical cyclones have also been identified to be modulated by the MJO, with westerly low-level winds in the eastern Pacific far more supportive of cyclogenesis than easterly (Maloney and Hartmann, 2000; Klotzbach and Oliver, 2015), and with Phase 8 associated with enhanced likelihoods for Gulf tropical cyclogenesis (Klotzbach, 2014).
While causes of MJO-influenced intraseasonal precipitation variability in the SCUSA have been investigated thoroughly, including due to mesoscale convective systems (Fritsch et al., 1986) and most recently using isotopic data (Sun et al., 2019), relatively few studies have isolated the regional impact of the MJO. Thompson and Roundy (2013) observed a robust link between March-May violent tornado days in the United States, with strong representation in the SCUSA, and Phase 2, which is manifested as a deep trough over the western and central United States and veering wind anomalies from the south to the southwest with increasing height. Guo et al. (2017) noted the dominance of frequency over intensity of MJO-related cyclonic activity, including over the SCUSA. MJO influences on cyclogenesis and severe convective storms with damaging winds, hail, and/or tornadoes in the United States. Gulf Coastal region, including parts of SCUSA, have also been identified (Caparotta, 2018).
Recent research has begun to suggest possible future changes in the MJO. Zhou et al. (2020) proposed that an eastward shift in the subtropical jet exit region will extend the MJO influence more strongly eastward, toward the SCUSA. Despite the potential, little research exists connecting the MJO beyond atmospheric conditions to other human and environmental systems in the SCUSA.
Discussion and summary/conclusions
Multiple ocean-atmosphere modes of variability and their teleconnections explain a significant amount of variability in low-frequency atmospheric flow at seasonal and longer time scales in the south-central United States (SCUSA). While a rich literature about these teleconnections has been amassed, the use of different data sets over different periods of record with results compiled over different temporal units (i.e., months, seasons, years) complicates comparison of those results. A thorough review of recent research through 2021 regarding the environmental impacts of the teleconnections from each major mode of climate variability in the SCUSA, with a focus on temperature and precipitation, is conducted here, independently of that put forth in the Intergovernmental Panel on Climate Change (IPCC) Fifth and Sixth Assessment Reports (IPCC 2014; IPCC 2022) and the United States. National Climate Assessments. Correlation fields are then generated for each climate mode’s index vs. seasonal temperature and (separately) precipitation using a modern, high-resolution data set from 1950 to 2020. Qualitative comparison reveals consistencies and a few differences between the literature and this analysis. In general, the teleconnections associated with the modes of climate variability examined have different degrees of impact seasonally and spatially across the SCUSA, with the spatial impact in the SCUSA varying particularly in the west-east direction. The ERA5 reanalysis product provides a more complete data set for our analysis, but because it is not comprised of direct observations, differences may occur from earlier assessments that used other data sets.
El Niño-Southern Oscillation (ENSO) is found here to have different impacts on the SCUSA than has been revealed in previous work. The fact that ENSO events, particularly Modoki (or central-Pacific ENSO), which may play an increasing role in the future, differ substantially from each other in terms of their physical characteristics and their impacts may explain the discrepancies with and among the previous studies. The most prominent temperature relationship in the SCUSA is in Texas in spring, when anomalously cool conditions occur in the El Niño phase and warm conditions occur in La Niña. Precipitation anomalies are also most prominent in Texas in spring, with El Niño associated with anomalously wet conditions and La Niña having drier-than-normal conditions in the spring.
As in most of the rest of the United States, the Pacific-North American (PNA) pattern relates to temperature only in winter and spring in the SCUSA, where a negative relationship exists in meteorological winter (DJF). This suggests that mid-tropospheric ridging over the Rocky Mountain cordillera is linked to anomalously warm temperatures and mid-tropospheric troughing in the same area is associated with anomalously cold conditions. Regarding precipitation, the positive relationship in much of the western coastal SCUSA and adjacent Gulf of Mexico means that Rocky Mountain ridging brings more precipitation than average, as that region tends to be under the influence of a downstream trough, while Rocky Mountain troughing makes this area drier than usual.
Although the relationship between the North Atlantic Oscillation (NAO) and temperature is weak in the SCUSA, some extensive areas of significant correlations to precipitation occur in spring, when much of Oklahoma and Texas southward along the Texas-Louisiana border and adjacent coastal areas show a relationship. More specifically, with strongly zonal flow associated with the NAO associated with increased precipitation in those areas and weaker zonality linked to suppressed precipitation.
The two large, amorphous climate modes frequently defined based on sea-surface temperatures in the Atlantic and Pacific Oceans also have teleconnections and impacts on the SCUSA. Unlike most other modes of climate variability, the Atlantic Multidecadal Oscillation (AMO; also known as the Atlantic Multidecadal Variability (AMV)) shows its strongest influence in the SCUSA in summer. The warm-phase AMV is tied to anomalously warm SCUSA temperatures, and vice versa. AMV-precipitation relationships, on the other hand, are weaker in SCUSA, with negative correlations strongest in autumn in the interior SCUSA. This analysis verifies the existing literature regarding the impacts of the Pacific Decadal Oscillation (PDO) on the SCUSA. A slight tendency for below-normal temperatures, especially in the cold season, and above-normal precipitation in the SCUSA are apparent during the warm phase, with generally opposite results in the cold-phase PDO. The AMV influences the northern Gulf of Mexico temperatures in summer and autumn whereas the PDO has greater influence in the winter and spring. The AMV has little influence over northern Gulf of Mexico precipitation but the PDO influences precipitation for all seasons except autumn, suggesting a quasi-persistent atmospheric teleconnection between the Gulf of Mexico and the North Pacific Ocean.
Collectively, the SO, NAO, NAM (AO), PNA pattern, AMV, PDO, and MJO ocean-atmosphere teleconnections set up a cascade of linkages (Terjung, 1976) that vary seasonally across the SCUSA that can ripple across environmental, economic, and social systems. One example of such cascading influence is the future impacts of atmospheric and land-use land-cover variability in forest ecosystems (Sohngen and Brown, 2006). Trigo et al. (2002) conducted a similar analysis on effects of the NAO on Europe and the adjacent North Atlantic waters. In general, the teleconnection information collected in previous studies and summarized here could be directed toward more human-environmental systems research. To date, most such work has focused on ENSO but utility exists for connecting other teleconnection-based forcing patterns, particularly the PNA pattern, AMV, and PDO, with human-environmental systems in the SCUSA.
Moreover, whereas the climate modes and their teleconnections described here are important modulators of atmospheric and hydroclimatic variability in the SCUSA, other teleconnections, particularly those identified by Barnston and Livezey (1987) and/or the circumglobal (Branstator, 2002) and extratropical Asian-Bering-North American (Yu et al., 2018) patterns, may also exert an influence on weather and climate in this region, as these latter two modes have been shown to have a comparable influence to the PNA pattern on North American climate (e.g., Yu et al., 2019). It is also possible that other identified or as-yet-unidentified teleconnection patterns could also be important for understanding SCUSA climate and cascading climatic impacts. These modes and their impacts could change in a changing climate. For example, non-stationarity associated with the eastward shift of the NAO, particularly in summer (Sun and Wang, 2012), has likely been associated with changes in its influence over the SCUSA.
Author contributions
The four authors contributed equally to the development of the idea and project planning. RR led the research, writing, and editing effort, but with contributions from all four authors. GS conducted statistical analyses of the reanalysis data sets and produced and revised the figures.
Funding
This research is supported by the Department of the Interior South Central Climate Adaptation Science Center Cooperative Agreement G19AC00086 and the USGS Ecosystems Mission Area facilitated interactions. KD and EM supported by NSF Award Number 2102931.
Acknowledgments
The authors appreciate the support of the South Central Climate Adaptation Science Center and the Teleconnection Working Group for their contributions to the development of this study. We thank our students and postdocs, particularly Kylie Palmer, for their contributions to this study and its development. Any use of trade, firm, or product names is for descriptive purposes only and does not imply endorsement by the United States Government.
Conflict of interest
The authors declare that the research was conducted in the absence of any commercial or financial relationships that could be construed as a potential conflict of interest.
Publisher’s note
All claims expressed in this article are solely those of the authors and do not necessarily represent those of their affiliated organizations, or those of the publisher, the editors and the reviewers. Any product that may be evaluated in this article, or claim that may be made by its manufacturer, is not guaranteed or endorsed by the publisher.
References
Ahn, S. E., Plantinga, A. J., and Alig, R. J. (2002). Determinants and projections of land use in the South Central United States. South. J. Appl. For. 26 (2), 78–84. doi:10.1093/sjaf/26.2.78
Alexander, M. A., Bladé, I., Newman, M., Lanzante, J. R., Lau, N-. C., and Scott, J. D. (2002). The atmospheric bridge: The influence of ENSO teleconnections on air–sea interaction over the global oceans. J. Clim. 15 (16), 2205–2231. doi:10.1175/1520-0442(2002)015<2205:TABTIO>2.0.CO;2
Alexander, M. A., Kilbourne, K. H., and Nye, J. A. (2014). Climate variability during warm and cold phases of the Atlantic Multidecadal Oscillation (AMO) 1871–2008. J. Mar. Syst. 133, 14–26. doi:10.1016/j.jmarsys.2013.07.017
Alig, R. J., Kline, J. D., and Lichtenstein, M. (2004). Urbanization on the US landscape: Looking ahead in the 21st century. Landsc. Urban Plan. 69 (2–3), 219–234. doi:10.1016/j.landurbplan.2003.07.004
Ashok, K., Behera, S. K., Rao, S. A., Weng, H., and Yamagata, T. (2007). El Niño Modoki and its possible teleconnection. J. Geophys. Res. 112 (C11), C11007. doi:10.1029/2006JC003798
Baggett, C. F., Barnes, E. A., Maloney, E. D., and Mundhenk, B. D. (2017). Advancing atmospheric river forecasts into subseasonal‐to‐seasonal timescales. Geophys. Res. Lett. 44 (14), 7528–7536. doi:10.1002/2017GL074434
Bahaga, T. K., Fink, A. H., and Knippertz, P. (2019). Revisiting interannual to decadal teleconnections influencing seasonal rainfall in the Greater Horn of Africa during the 20th century. Int. J. Climatol. 39 (5), 2765–2785. doi:10.1002/joc.5986
Barlow, M., Nigam, S., and Berbery, E. H. (2001). ENSO, Pacific decadal variability, and US summertime precipitation, drought, and stream flow. J. Clim. 14 (9), 2105–2128. doi:10.1175/1520-0442(2001)014<2105:EPDVAU>2.0.CO;2
Barnston, A. G., and Livezey, R. E. (1987). Classification, seasonality and persistence of low-frequency atmospheric circulation patterns. Mon. Weather Rev. 115 (6), 1083–1126. doi:10.1175/1520-0493(1987)115<1083:CSAPOL>2.0.CO;2
Barrett, B. S. (2019). Connections between the madden–julian oscillation and surface temperatures in winter 2018 over eastern north America. Atmos. Sci. Lett. 20 (1), e869. doi:10.1002/asl.869
Bentley, A. M., Bosart, L. F., and Keyser, D. (2019). A climatology of extratropical cyclones leading to extreme weather events over central and eastern North America. Mon. Weather Rev. 147 (5), 1471–1490. doi:10.1175/MWR-D-18-0453.1
Biondi, F., Gershunov, A., and Cayan, D. R. (2001). North Pacific decadal climate variability since 1661. J. Clim. 14 (1), 5–10. doi:10.1175/1520-0442(2001)014<0005:NPDCVS>2.0.CO;2
Bishop, D. A., Williams, A. P., Seager, R., Fiore, A. M., Cook, B. I., Mankin, J. S., et al. (2019). Investigating the causes of increased twentieth-century fall precipitation over the southeastern United States. J. Clim. 32 (2), 575–590. doi:10.1175/JCLI-D-18-0244.1
Bjerknes, J. (1969). Atmospheric teleconnections from the equatorial Pacific1. Mon. Weather Rev. 97 (3), 163–172. doi:10.1175/1520-0493(1969)097<0163:atftep>2.3.co;2(1969)097<0163:ATFTEP>2.3.CO;2
Bonfils, C., and Santer, B. D. (2011). Investigating the possibility of a human component in various Pacific decadal oscillation indices. Clim. Dyn. 37 (7), 1457–1468. doi:10.1007/s00382-010-0920-1
Booth, B. B. B., Dunstone, N. J., Halloran, P. R., Andrews, T., and Bellouin, N. (2012). Aerosols implicated as a prime driver of twentieth-century North Atlantic climate variability. Nature 484 (7393), 228–232. doi:10.1038/nature10946
Bove, M. C., Elsner, J. B., Landsea, C. W., Niu, X., and O'Brien, J. J. (1998). Effect of El Niño on US landfalling hurricanes, revisited. B. Am. Meteorol. Soc. 9 (11), 2477–2482. doi:10.1175/1520-0477(1998)079<2477:EOENOO>2.0.CO;2
Bradbury, J. A., Keim, B. D., and Wake, C. P. (2003). The influence of regional storm tracking and teleconnections on winter precipitation in the northeastern United States. Ann. Assoc. Am. Geogr. 93 (3), 544–556. doi:10.1111/1467-8306.9303002
Branstator, G. (2002). Circumglobal teleconnections, the jet stream waveguide, and the North Atlantic Oscillation. J. Clim. 15 (14), 1893–1910. doi:10.1175/1520-0442(2002)015<1893:CTTJSW>2.0.CO;2
Bretherton, C. S., Widmann, M., Dymnikov, V. P., Wallace, J. M., and Bladé, I. (1999). The effective number of spatial degrees of freedom of a time-varying field. J. Clim. 12 (7), 1990–2009. doi:10.1175/1520-0442(1999)012<1990:TENOSD>2.0.CO;2
Budikova, D. (2005). Impact of the pacific decadal oscillation on relationships between temperature and the arctic oscillation in the USA in winter. Clim. Res. 29 (3), 199–208. doi:10.3354/cr029199
Bunge, L., and Clarke, A. J. (2009). A verified estimation of the El Niño index Niño-3.4 since 1877. J. Clim. 22 (14), 3979–3992. doi:10.1175/2009JCLI2724.1
Bushra, N., and Rohli, R. V. (2021). Relationship between atmospheric teleconnections and the Northern Hemisphere's circumpolar vortex. Earth Space Sci. 8 (9), e2021EA001802. doi:10.1029/2021EA001802
Cai, W. J., Wang, G. J., Santoso, A., McPhaden, M. J., Wu, L. X., Jin, F.- F., et al. (2015). Increased frequency of extreme La Niña events under greenhouse warming. Nat. Clim. Chang. 5 (2), 132–137. doi:10.1038/NCLIMATE2492
Cai, W., Santoso, A., Collins, M., Dewitte, B., Karamperidou, C., Kug, J.- S., et al. (2021). Changing El niño-southern oscillation in a warming climate. Nat. Rev. Earth Environ. 2 (9), 628–644. doi:10.1038/s43017-021-00199-z
Callahan, C. W., Chen, C., Rugenstein, M., Bloch-Johnson, J., Yang, S., and Moyer, E. J. (2021). Robust decrease in El Niño/Southern Oscillation amplitude under long-term warming. Nat. Clim. Chang. 11 (9), 752–757. doi:10.1038/s41558-021-01099-2
Caparotta, S. P. (2018). Madden-julian oscillation relationships with cool season cyclogenesis, daily precipitation, and cool season severe weather frequencies in the Gulf of Mexico region (Baton Rouge, LA: Louisiana State University). Ph.D. Dissertation.
Capotondi, A., Wittenberg, A. T., Newman, M., Di Lorenzo, E., Yu, J.- Y., Braconnot, P., et al. (2015). Understanding ENSO diversity. Bull. Am. Meteorol. Soc. 96 (6), 921–938. doi:10.1175/BAMS-D-13-00117.1
Cassou, C. (2008). Intraseasonal interaction between the madden-julian oscillation and the north atlantic oscillation. Nature 455 (7212), 523–527. doi:10.1038/nature07286
Ceballos, L. I., Di Lorenzo, E., Hoyos, C. D., Schneider, N., and Taguchi, B. (2009). North Pacific Gyre Oscillation synchronizes climate fluctuations in the eastern and Western boundary systems. J. Clim. 22 (19), 5163–5174. doi:10.1175/2009JCLI2848.1
Cellitti, M. P., Walsh, J. E., Rauber, R. M., and Portis, D. H. (2006). Extreme cold air outbreaks over the United States, the polar vortex, and the large‐scale circulation. J. Geophys. Res. 111 (D2), D02114. doi:10.1029/2005JD006273
Chen, J.- M., Hsieh, C.- M., and Liu, J.- S. (2012). Possible influences of ENSO on winter shipping in the North Pacific. Terr. Atmos. Ocean. Sci. 23 (4), 397–411. doi:10.3319/TAO.2012.03.02.01(A
Chiang, J. C. H., and Vimont, D. J. (2004). Analogous Pacific and Atlantic meridional modes of tropical atmosphere-ocean variability. J. Clim. 17 (21), 4143–4158. doi:10.1175/JCLI4953.1
Clement, A., Bellomo, K., Murphy, L. N., Cane, M. A., Mauritsen, T., Rädel, G., et al. (2015). The Atlantic Multidecadal Oscillation without a role for ocean circulation. Science 350 (6258), 320–324. doi:10.1126/science.aab3980
Coleman, J. S. M., and Budikova, D. (2010). Atmospheric aspects of the 2008 midwest floods: A repeat of 1993? Int. J. Climatol. 30 (11), 1645–1667. doi:10.1002/joc.2009
Cook, A. R., Leslie, L. M., Parsons, D. B., and Schaefer, J. T. (2017). The impact of El Niño–Southern Oscillation (ENSO) on winter and early spring US tornado outbreaks. J. Appl. Meteorol. Climatol. 56 (9), 2455–2478. doi:10.1175/JAMC-D-16-0249.1
Cook, B. I., Smerdon, J. E., Seager, R., and Cook, E. R. (2014). Pan-continental droughts in North America over the last millennium. J. Clim. 27 (1), 383–397. doi:10.1175/JCLI-D-13-00100.1
Copernicus Climate Change Service (2017). ERA5: Fifth generation of ECMWF atmospheric reanalyses of the global climate. Copernicus climate change Service climate data store (CDS). Available at: https://www.ecmwf.int/en/forecasts/datasets/reanalysis-datasets/era5 (Last accessed: April 20, 2022).
D'Arrigo, R., Villalba, R., and Wiles, G. (2001). Tree-ring estimates of Pacific decadal climate variability. Clim. Dyn. 18 (3–4), 219–224. doi:10.1007/s003820100177
Danco, J., and Martin, E. R. (2018). Understanding the influence of ENSO on the Great Plains low-level jet in CMIP5 models. Clim. Dyn. 51 (4), 1537–1558. doi:10.1007/s00382-017-3970-9
Davis, R. E. (1976). Predictability of sea-surface temperature and sea-level pressure anomalies over North Pacific Ocean. J. Phys. Oceanogr. 6 (3), 249–266. doi:10.1175/1520-0485(1976)006<0249:POSSTA>2.0.CO;2
de Chazal, J., and Rounsevell, M. D. A. (2009). Land-use and climate change within assessments of biodiversity change: A review. Glob. Environ. Change 19 (2), 306–315. doi:10.1016/j.gloenvcha.2008.09.007
DeLong, K. L., Flannery, J. A., Poore, R. Z., Quinn, T. M., Maupin, C. R., Lin, K., et al. (2014). A reconstruction of sea surface temperature variability in the southeastern Gulf of Mexico from 1734–2008 CE using cross-dated Sr/Ca records from the coral Siderastrea siderea. Paleoceanography 29 (5), 403–422. doi:10.1002/2013PA002524
DeLong, K. L., Quinn, T. M., Taylor, F. W., Lin, K., and Shen, C.- C. (2012). Sea surface temperature variability in the southwest tropical Pacific since AD 1649. Nat. Clim. Chang. 2 (11), 799–804. doi:10.1038/NCLIMATE1583
Deser, C., Alexander, M. A., Xie, S.- P., and Phillips, A. S. (2010). Sea surface temperature variability: Patterns and mechanisms. Annu. Rev. Mar. Sci. 2010 (2), 115–143. doi:10.1146/annurev-marine-120408-151453
Deser, C., and Phillips, A. S. (2021). Defining the internal component of Atlantic Multidecadal Variability in a changing climate. Geophys. Res. Lett. 48 (22), e2021GL095023. doi:10.1029/2021GL095023
Deser, C., Phillips, A. S., Tomas, R. A., Okumura, Y. M., Alexander, M. A., Capotondi, A., et al. (2012). ENSO and pacific decadal variability in the community climate system model version 4. J. Clim. 25 (8), 2622–2651. doi:10.1175/JCLI-D-11-00301.1
Deser, C., Simpson, I. R., Phillips, A. S., and McKinnon, K. A. (2018). How well do we know ENSO’s climate impacts over North America, and how do we evaluate models accordingly? J. Clim. 31 (13), 4991–5014. doi:10.1175/JCLI-D-17-0783.1
Dethier, E. N., Sartain, S. L., Renshaw, C. E., and Magilligan, F. J. (2020). Spatially coherent regional changes in seasonal extreme streamflow events in the United States and Canada since 1950. Sci. Adv. 6 (49), eaba5939. doi:10.1126/sciadv.aba5939
Di Lorenzo, E., and Mantua, N. (2016). Multi-year persistence of the 2014/15 North Pacific marine heatwave. Nat. Clim. Chang. 6 (11), 1042–1047. doi:10.1038/nclimate3082
Di Lorenzo, E., Schneider, N., Cobb, K. M., Franks, P. J. S., Chhak, K., Miller, A. J., et al. (2008). North Pacific Gyre Oscillation links ocean climate and ecosystem change. Geophys. Res. Lett. 35 (8), L08607. doi:10.1029/2007GL032838
Dima, M., and Lohmann, G. (2007). A hemispheric mechanism for the atlantic multidecadal oscillation. J. Clim. 20 (11), 2706–2719. doi:10.1175/JCLI4174.1
Dong, B., Dai, A., Vuille, M., and Timm, O. E. (2018). Asymmetric modulation of ENSO teleconnections by the interdecadal Pacific oscillation. J. Clim. 31 (18), 7337–7361. doi:10.1175/JCLI-D-17-0663.1
Dong, X., Xi, B., Kennedy, A., Feng, Z., Entin, J. K., Houser, P. R., et al. (2011). Investigation of the 2006 drought and 2007 flood extremes at the Southern Great Plains through an integrative analysis of observations. J. Geophys. Res. 116, D03204. doi:10.1029/2010JD014776
Eichler, T., and Higgins, W. (2006). Climatology and ENSO-related variability of North American extratropical cyclone activity. J. Clim. 19 (10), 2076–2093. doi:10.1175/JCLI3725.1
Ellis, A. W., and Marston, M. L. (2020). Late 1990s’ cool season climate shift in eastern North America. Clim. Change 162 (3), 1385–1398. doi:10.1007/s10584-020-02798-z
Enfield, D. B., Mestas-Nuñez, A. M., and Trimble, P. J. (2001). The Atlantic multidecadal oscillation and its relation to rainfall and river flows in the continental U.S. Geophys. Res. Lett. 28 (10), 2077–2080. doi:10.1029/2000GL012745
Fang, S. W., Khodri, M., Timmreck, C., Zanchettin, D., and Jungclaus, J. (2021). Disentangling internal and external contributions to Atlantic multidecadal variability over the past millennium. Geophys. Res. Lett. 48 (23), e2021GL095990. doi:10.1029/2021GL095990
Felis, T., Suzuki, A., Kuhnert, H., Rimbu, N., and Kawahata, H. (2010). Pacific Decadal Oscillation documented in a coral record of North Pacific winter temperature since 1873. Geophys. Res. Lett. 37 (14). doi:10.1029/2010GL043572
Ferranti, L., Palmer, T. N., Molteni, F., and Klinker, E. (1990). Tropical extratropical interaction associated with the 30-60 day oscillation and its impact on medium and extended range prediction. J. Atmos. Sci. 47 (18), 2177–2199. doi:10.1175/1520-0469(1990)047<2177:TEIAWT>2.0.CO;2
Flanagan, P. X., Basara, J. B., Furtado, J. C., Martin, E. R., and Xiao, X. (2019). Role of sea surface temperatures in forcing circulation anomalies driving U.S. Great Plains pluvial years. J. Clim. 32 (20), 7081–7100. doi:10.1175/JCLI-D-18-0726.1
Flannery, J. A., Richey, J. N., Thirumalai, K., Poore, R. Z., and DeLong, K. L. (2017). Multi-species coral Sr/Ca-based sea-surface temperature reconstruction using Orbicella faveolata and Siderastrea siderea from the Florida Straits. Palaeogeogr. Palaeoclimatol. Palaeoecol. 466, 100–109. doi:10.1016/j.palaeo.2016.10.022
Folland, C. K., Knight, J., Linderholm, H. W., Fereday, D., Ineson, S., and Hurrell, J. W. (2009). The summer North Atlantic oscillation: Past, present, and future. J. Clim. 22 (5), 1082–1103. doi:10.1175/2008JCLI2459.1
Folland, C. K., Renwick, J. A., Salinger, M. J., and Mullan, A. B. (2002). Relative influences of the interdecadal pacific oscillation and ENSO on the south pacific convergence zone. Geophys. Res. Lett. 29 (13), 1643. doi:10.1029/2001gl014201
Ford, T. W., Quiring, S. M., and Frauenfeld, O. W. (2017). Multi‐decadal variability of soil moisture-temperature coupling over the contiguous United States modulated by Pacific and Atlantic sea surface temperatures. Int. J. Climatol. 37 (3), 1400–1415. doi:10.1002/joc.4785
Frederiksen, C. S., Zheng, X., and Grainger, S. (2014). Teleconnections and predictive characteristics of Australian seasonal rainfall. Clim. Dyn. 43 (5–6), 1381–1408. doi:10.1007/s00382-013-1952-0
Fritsch, J. M., Kane, R. J., and Chelius, C. R. (1986). The contribution of mesoscale convective weather systems to the warm-season precipitation in the United States. J. Clim. Appl. Meteor. 25 (10), 1333–1345. doi:10.1175/1520-0450(1986)025<1333:TCOMCW>2.0.CO;2
Gan, Z., Guan, X., Kong, X., Guo, R., Huang, H., Huang, W., et al. (2019). The key role of Atlantic Multidecadal Oscillation in minimum temperature over North America during global warming slowdown. Earth Space Sci. 6 (3), 387–397. doi:10.1029/2018EA000443
Gershunov, A., and Barnett, T. P. (1998). Interdecadal modulation of ENSO teleconnections. Bull. Am. Meteorol. Soc. 79 (12), 2715–2725. doi:10.1175/1520-0477(1998)079<2715:IMOET>2.0.CO;2
Gomez, F. A., Lee, S.- K., Hernandez, F. J., Chiaverano, L. M., Muller-Karger, F. E., Liu, Y., et al. (2019). ENSO-induced co-variability of salinity, plankton biomass and coastal currents in the Northern Gulf of Mexico. Sci. Rep. 9 (1), 178. doi:10.1038/s41598-018-36655-y
Gray, S. T., Graumlich, L. J., Betancourt, J. L., and Pederson, G. T. (2004). A tree-ring based reconstruction of the Atlantic Multidecadal Oscillation since 1567 AD. Geophys. Res. Lett. 31 (12), L12205. doi:10.1029/2004GL019932
Gray, W. M. (1984). Atlantic seasonal hurricane frequency. Part 1: El Niño and 30 mb quasi-biennial oscillation influences. Mon. Weather Rev. 112 (9), 1649–1668. doi:10.1175/1520-0493(1984)112<1649:ASHFPI>2.0.CO;2
Grise, K. M., Son, S.- W., and Gyakum, J. R. (2013). Intraseasonal and interannual variability in North American storm tracks and its relationship to equatorial Pacific variability. Mon. Weather Rev. 141 (10), 3610–3625. doi:10.1175/MWR-D-12-00322.1
Guo, Y., Shinoda, T., Lin, J., and Chang, E. K. (2017). Variations of northern hemisphere storm track and extratropical cyclone activity associated with the Madden-Julian Oscillation. J. Clim. 30 (13), 4799–4818. doi:10.1175/JCLI-D-16-0513.1
Hamamoto, M., and Yasuda, I. (2021). Synchronized interdecadal variations behind regime shifts in the Pacific Decadal Oscillation. J. Oceanogr. 77 (3), 383–392. doi:10.1007/s10872-021-00592-8
Hamouda, M. E., Pasquero, C., and Tziperman, E. (2021). Decoupling of the arctic oscillation and north atlantic oscillation in a warmer climate. Nat. Clim. Change 11 (2), 137–142. doi:10.1038/s41558-020-00966-8
Han, Z., Luo, F., Li, S., Gao, Y., Furevik, T., and Svendsen, L. (2016). Simulation by CMIP5 models of the Atlantic multidecadal oscillation and its climate impacts. Adv. Atmos. Sci. 33 (12), 1329–1342. doi:10.1007/s00376-016-5270-4
Hardy, J. W., and Henderson, K. G. (2003). Cold front variability in the southern United States and the influence of atmospheric teleconnection patterns. Phys. Geogr. 24 (2), 120–137. doi:10.2747/0272-3646.24.2.120
He, S., and Wang, H. (2013). Oscillating relationship between the East Asian winter monsoon and ENSO. J. Clim. 26 (24), 9819–9838. doi:10.1175/JCLI-D-13-00174.1
Henderson, K. G., and Robinson, P. J. (1994). Relationships between the Pacific/North American teleconnection patterns and precipitation events in the south‐eastern USA. Int. J. Climatol. 14 (3), 307–323. doi:10.1002/joc.3370140305
Henley, B. J., Gergis, J., Karoly, D. J., Power, S., Kennedy, J., and Folland, C. K. (2015). A tripole index for the interdecadal Pacific oscillation. Clim. Dyn. 45 (11–12), 3077–3090. doi:10.1007/s00382-015-2525-1
Hersbach, H., Bell, B., Berrisford, P., Hirahara, S., Horanyi, A., Muñoz-Sabater, J., et al. (2020). The ERA5 global reanalysis. Q. J. R. Meteorol. Soc. 146 (730), 1999–2049. doi:10.1002/qj.3803
Hetzinger, S., Pfeiffer, M., Dullo, W. C., Keenlyside, N., Latif, M., and Zinke, J. (2008). Caribbean coral tracks Atlantic Multidecadal Oscillation and past hurricane activity. Geol. 36 (1), 11–14. doi:10.1130/G24321A.1
Hoerling, M. P., Kumar, A., and Zhong, M. (1997). El Niño, La Niña, and the nonlinearity of their teleconnections. J. Clim. 10 (8), 1769–1786. doi:10.1175/1520-0442(1997)010<1769:ENOLNA>2.0.CO;2
Hopkinson, C. S., Covich, A. P., Craft, C. B., DeLong, K. L., Doyle, T. W., Flanagan, N., et al. (2013). “The effects of climate change on natural ecosystems of the Southeast USA,” in Climate of the southeast United States: Variability, change, impacts, and vulnerability. Editors K. T. Ingram, K. Dow, L. Carter, and J. Anderson (Washington, D.C.: Island Press), 237–270. Available at: https://link.springer.com/content/pdf/10.5822%2F978-1-61091-509-0.pdf (Last accessed: April 20, 2022).
Horel, J. D., and Wallace, J. M. (1981). Planetary-scale atmospheric phenomena associated with the Southern Oscillation. Mon. Weather Rev. 109 (4), 813–829. doi:10.1175/1520-0493(1981)109<0813:PSAPAW>2.0.CO;2
Hsieh, W. W., Wu, A., and Shabbar, A. (2006). Nonlinear atmospheric teleconnections. Geophys. Res. Lett. 33 (7), L07714. doi:10.1029/2005GL025471
Hsu, H.- H., and Chen, Y.- L. (2011). Decadal to bi‐decadal rainfall variation in the Western pacific: A footprint of south Pacific decadal variability? Geophys. Res. Lett. 38 (3), L03703. doi:10.1029/2010GL046278
Huang, B., Thorne, P. W., Banzon, V. F., Boyer, T., Chepurin, G., Lawrimore, J. H., et al. (2017). Extended reconstructed sea surface temperature, version 5 (ERSSTv5): Upgrades, validations, and intercomparisons. J. Clim. 30 (20), 8179–8205. doi:10.1175/JCLI-D-16-0836.1
Hurrell, J. W. (1995). Decadal trends in the north atlantic oscillation: Regional temperatures and precipitation. Science 269 (5224), 676–679. doi:10.1126/science.269.5224.676
Hurrell, J. W. (1996). Influence of variations in extratropical wintertime teleconnections on Northern Hemisphere temperature. Geophys. Res. Lett. 23 (6), 665–668. doi:10.1029/96GL00459
Hurrell, J. W., and van Loon, H. (1997). Decadal variations in climate associated with the north Atlantic oscillation. Clim. Change 36 (3–4), 301–326. doi:10.1023/A:1005314315270
IPCC (2014). in Climate change 2014: Impacts, adaptation, and vulnerability. Part A: Global and sectoral aspects. Editors Field, C. B., Barros, V. R., Dokken, D. J., Mach, K. J., Mastrandrea, M. D., Bilir, T. E., et al. (Cambridge, United Kingdom and New York, NY, U.S.A.: Cambridge University Press), 1132. Contribution of Working Group II to the Fifth Assessment Report of the Intergovernmental Panel on Climate Change.
IPCC (2022). in Climate change 2022: Impacts, adaptation, and vulnerability. Contribution of working Group II to the Sixth assessment report of the intergovernmental Panel on climate change. Editors Pörtner, H.-O., Roberts, D. C., Tignor, M., Poloczanska, E. S., Mintenbeck, K., Alegría, A., et al. (Cambridge, United Kingdom and New York, NY, U.S.A.: Cambridge University Press).
Jones, C., and Carvalho, L. M. (2014). Sensitivity to Madden–Julian oscillation variations on heavy precipitation over the contiguous United States. Atmos. Res. 147, 10–26. doi:10.1016/j.atmosres.2014.05.002
Jones, C., Gottschalck, J., Carvalho, L. M., and Higgins, W. (2011). Influence of the Madden-Julian oscillation on forecasts of extreme precipitation in the contiguous United States. Mon. Weather Rev. 139 (2), 332–350. doi:10.1175/2010MWR3512.1
Jones, C. (2000). Occurrence of extreme precipitation events in California and relationships with the Madden-Julian oscillation. J. Clim. 13 (20), 3576–3587. doi:10.1175/1520-0442(2000)013<3576:OOEPEI>2.0.CO;2
Jones, C., Waliser, D. E., Lau, K. M., and Stern, W. (2004). The Madden-Julian oscillation and its impact on Northern Hemisphere weather predictability. Mon. Weather Rev. 132 (6), 1462–1471. doi:10.1175/1520-0493(2004)132<1462:TMOAII>2.0.CO;2
Kao, H.- Y., and Yu, J.- Y. (2009). Contrasting eastern-pacific and central-pacific types of ENSO. J. Clim. 22 (3), 615–632. doi:10.1175/2008JCLI2309.1
Kapala, A., Mächel, H., and Flohn, H. (1998). Behaviour of the centres of action above the Atlantic since 1881. Part II: Associations with regional climate anomalies. Int. J. Climatol. 18 (1), 23–36. doi:10.1002/(SICI)1097-0088(199801)18:1<23::AID-JOC226>3.0.CO;2-7
Kaplan, A., Cane, M. A., Kushnir, Y., Clement, A. C., Blumenthal, M. B., and Rajagopalan, B. (1998). Analyses of global sea surface temperature 1856-1991. J. Geophys. Res. 103 (C9), 18567–18589. doi:10.1029/97JC01736
Karnauskas, M., Schirripa, M. J., Craig, J. K., Cook, G. S., Kelbe, C. R., Agar, J. J., et al. (2015). Evidence of climate-driven ecosystem reorganization in the Gulf of Mexico. Glob. Chang. Biol. 21, 2554–2568. doi:10.1111/gcb.12894
Kerr, R. A. (2000). A North Atlantic climate pacemaker for the centuries. Science 288 (5473), 1984–1985. doi:10.1126/science.288.5473.1984
Khedun, C. P., Mishra, A. K., Bolten, J. D., Beaudoing, H. K., Kaiser, R. A., Giardino, J. R., et al. (2012). Understanding changes in water availability in the Rio Grande/Rio Bravo del Norte basin under the influence of large-scale circulation indices using the Noah land surface model. J. Geophys. Res. 117, D05104. doi:10.1029/2011JD016590
Kilbourne, K. H., Alexander, M. A., and Nye, J. A. (2014). A low latitude paleoclimate perspective on Atlantic multidecadal variability. J. Mar. Syst. 133, 4–13. doi:10.1016/j.jmarsys.2013.09.004
Kilbourne, K. H., Quinn, T. M., Webb, R., Guilderson, T., Nyberg, J., and Winter, A. (2008). Paleoclimate proxy perspective on Caribbean climate since the year 1751: Evidence of cooler temperatures and multidecadal variability. Paleoceanography 23 (3), PA3220. doi:10.1029/2008PA001598
Kim, H.- M., Webster, P. J., and Curry, J. A. (2009). Impact of shifting patterns of Pacific Ocean warming on North Atlantic tropical cyclones. Science 325 (5936), 77–80. doi:10.1126/science.1174062
Klemm, T., and McPherson, R. A. (2018). Assessing decision timing and seasonal climate forecast needs of winter wheat producers in the South-central United States. J. Appl. Meteorol. Climatol. 57 (9), 2129–2140. doi:10.1175/JAMC-D-17-0246.1
Klotzbach, P. J., and Oliver, E. C. (2015). Modulation of Atlantic basin tropical cyclone activity by the Madden-Julian Oscillation (MJO) from 1905 to 2011. J. Clim. 28 (1), 204–217. doi:10.1175/JCLI-D-14-00509.1
Klotzbach, P. J. (2014). The Madden-Julian Oscillation’s impacts on worldwide tropical cyclone activity. J. Clim. 27 (6), 2317–2330. doi:10.1175/JCLI-D-13-00483.1
Knight, J. R., Allan, R. J., Folland, C. K., Vellinga, M., and Mann, M. E. (2005). A signature of persistent natural thermohaline circulation cycles in observed climate. Geophys. Res. Lett. 32 (20), L20708. doi:10.1029/2005GL024233
Knight, J. R., Folland, C. K., and Scaife, A. A. (2006). Climate impacts of the atlantic multidecadal oscillation. Geophys. Res. Lett. 33, L17706. doi:10.1029/2006GL026242
Konapala, G., Veettil, A. V., and Mishra, A. K. (2018). Teleconnection between low flows and large-scale climate indices in Texas River basins. Stoch. Environ. Res. Risk Assess. 32 (8), 2337–2350. doi:10.1007/s00477-017-1460-6
Krishnamurthy, L., Vecchi, G. A., Msadek, R., Wittenberg, A., Delworth, T. L., and Zeng, F. (2015). The seasonality of the Great Plains low-level jet and ENSO relationship. J. Clim. 28 (11), 4525–4544. doi:10.1175/JCLI-D-14-00590.1
Kumar, S., Kinter, J., Dirmeyer, P. A., Pan, Z., and Adams, J. (2013). Multidecadal climate variability and the “warming hole” in North America: Results from CMIP5 twentieth- and twenty-first-century climate simulations. J. Clim. 26 (11), 3511–3527. doi:10.1175/JCLI-D-12-00535.1
Kurtzman, D., and Scanlon, B. R. (2007). El niño–southern oscillation and pacific decadal oscillation impacts on precipitation in the southern and central United States: Evaluation of spatial distribution and predictions. Water Resour. Res. 43 (10), W10427. doi:10.1029/2007WR005863
Kushnir, Y., Seager, R., Ting, M., Naik, N., and Nakamura, J. (2010). Mechanisms of tropical Atlantic SST influence on North American precipitation variability. J. Clim. 23 (21), 5610–5628. doi:10.1175/2010JCLI3172.1
Lamb, P. J., and Peppler, R. A. (1987). North Atlantic oscillation: Concept and an application. B. Am. Meteorol. Soc. 68 (10), 1218–1225. doi:10.1175/1520-0477(1987)068<1218:NAOCAA>2.0.CO;2
Leathers, D. J., and Palecki, M. A. (1992). The Pacific/North American teleconnection pattern and United States climate. Part II: Temporal characteristics and index specification. J. Clim. 5 (7), 707–716. doi:10.1175/1520-0442(1992)005<0707:TPATPA>2.0.CO;2
Leathers, D. J., Yarnal, B., and Palecki, M. A. (1991). The Pacific North-American teleconnection pattern and United-States climate. 1. Regional temperature and precipitation associations. J. Clim. 4 (5), 517–528. doi:10.1175/1520-0442(1991)004<0517:TPATPA>2.0.CO;2
Lee, R. W., Woolnough, S. J., Charlton‐Perez, A. J., and Vitart, F. (2019). ENSO modulation of MJO teleconnections to the north atlantic and Europe. Geophys. Res. Lett. 46 (22), 13535–13545. doi:10.1029/2019GL084683
Lee, S. K., Atlas, R., Enfield, D., Wang, C., and Liu, H. (2013). Is there an optimal ENSO pattern that enhances large-scale atmospheric processes conducive to tornado outbreaks in the United States? J. Clim. 26 (5), 1626–1642. doi:10.1175/JCLI-D-12-00128.1
Liang, Y.- C., Lo, M.- H., and Yu, J.- Y. (2014). Asymmetric responses of land hydroclimatology to two types of El Niño in the Mississippi River Basin. Geophys. Res. Lett. 41, 582–588. doi:10.1002/2013GL058828
Liang, Y.-C., Yu, J.- Y., Lo, M.- H., and Wang, C. (2015). The changing influence of El Niño on the Great Plains low-level jet. Atmos. Sci. Lett. 16, 512–517. doi:10.1002/asl.590
Lim, Y. K., Schubert, S. D., Reale, O., Molod, A. M., Suarez, M. J., and Auer, B. M. (2016). Large-scale controls on Atlantic tropical cyclone activity on seasonal time scales. J. Clim. 29 (18), 6727–6749. doi:10.1175/JCLI-D-16-0098.1
Lin, H., Brunet, G., and Derome, J. (2009). An observed connection between the north atlantic oscillation and the madden-julian oscillation. J. Clim. 22 (2), 364–380. doi:10.1175/2008JCLI2515.1
Linsley, B. K., Zhang, P. P., Kaplan, A., Howe, S. S., and Wellington, G. M. (2008). Interdecadal-decadal climate variability from multicoral oxygen isotope records in the South Pacific Convergence Zone region since 1650 A.D. Paleoceanography 23 (2), PA2219. doi:10.1029/2007PA001539
Liu, Y., Li, Z., Lin, X., Yang, J. C., Zhang, T., Luan, M., et al. (2021). Impacts of COVID-19 on black carbon in two representative regions in China: Insights based on online measurement in beijing and tibet. Geophys. Res. Lett. 48 (22), e2021GL092770. doi:10.1029/2021GL092770
Liu, Z., Yoshimura, K., Bowen, G. J., and Welker, J. M. (2014). Pacific-North American teleconnection controls on precipitation isotopes (δ18O) across the contiguous United States and adjacent regions: A GCM-based analysis. J. Clim. 27 (3), 1046–1061. doi:10.1175/JCLI-D-13-00334.1
Liu, Z., Yoshimura, K., Buenning, N. H., Jian, Z., and Zhao, L. (2017). The response of winter Pacific North American pattern to strong volcanic eruptions. Clim. Dyn. 48 (11), 3599–3614. doi:10.1007/s00382-016-3287-0
Loewe, F. (1966). The temperature see-saw between Western Greenland and Europe. Weather 21 (7), 241–246. doi:10.1002/j.1477-8696.1966.tb02865.x
Loikith, P. C., and Broccoli, A. J. (2014). The influence of recurrent modes of climate variability on the occurrence of winter and summer extreme temperatures over North America. J. Clim. 27 (4), 1600–1618. doi:10.1175/JCLI-D-13-00068.1
Lorenz, E. N. (1951). Seasonal and irregular variations of the northern hemisphere sea-level pressure profile. J. Meteor. 8 (1), 52–59. doi:10.1175/1520-0469(1951)008<0052:SAIVOT>2.0.CO;2
Lukens, K. E., Feldstein, S. B., Yoo, C., and Lee, S. (2017). The dynamics of the extratropical response to Madden–Julian Oscillation convection. Q. J. R. Meteorol. Soc. 143 (703), 1095–1106. doi:10.1002/qj.2993
MacDonald, G. M., and Case, R. A. (2005). Variations in the pacific decadal oscillation over the past millennium. Geophys. Res. Lett. 32 (8), L08703. doi:10.1029/2005GL022478
Mächel, H., Kapala, A., and Flohn, H. (1998). Behaviour of the centres of action above the Atlantic since 1881. Part I: Characteristics of seasonal and interannual variability. Int. J. Climatol. 18 (1), 1–22. doi:10.1002/(SICI)1097-0088(199801)18:1<1::AID-JOC225>3.0.CO;2-A
Madden, R. A., and Julian, P. R. (1971). Detection of a 40-50 day oscillation in the zonal wind in the tropical Pacific. J. Atmos. Sci. 28 (5), 702–708. doi:10.1175/1520-0469(1971)028<0702:DOADOI>2.0.CO;2
Madden, R. A., and Julian, P. R. (1994). Observations of the 40-50-Day tropical oscillation – a review. Mon. Weather Rev. 122 (5), 814–837. doi:10.1175/1520-0493(1994)122<0814:OOTDTO>2.0.CO;2
Maloney, E. D., and Hartmann, D. L. (2000). Modulation of hurricane activity in the Gulf of Mexico by the madden-julian oscillation. Science 287 (5460), 2002–2004. doi:10.1126/science.287.5460.2002
Mann, M. E. (2021). Beyond the hockey stick: Climate lessons from the Common Era. Proc. Natl. Acad. Sci. U. S. A. 118 (39), e2112797118. doi:10.1073/pnas.2112797118
Mantua, N. J., and Hare, S. R. (2002). The pacific decadal oscillation. J. Oceanogr. 58 (1), 35–44. doi:10.1023/A:1015820616384
Mantua, N. J., Hare, S. R., Zhang, Y., Wallace, J. M., and Francis, R. C. (1997). A pacific interdecadal climate oscillation with impacts on salmon production. Bull. Am. Meteorol. Soc. 78 (6), 1069–1079. doi:10.1175/1520-0477(1997)078<1069:APICOW>2.0.CO;2
Marshall, J., Kushnir, Y., Battisti, D., Chang, P., Czaja, A., Dickson, R., et al. (2001). North Atlantic climate variability: Phenomena, impacts and mechanisms. Int. J. Climatol. 21 (15), 1863–1898. doi:10.1002/joc.693
Martin, E. R., and Schumacher, C. (2011b). Modulation of caribbean precipitation by the madden-julian oscillation. J. Clim. 24 (3), 813–824. doi:10.1175/2010JCLI3773.1
Martin, E. R., and Schumacher, C. (2011a). The Caribbean Low-Level Jet and its relationship with precipitation in IPCC AR4 models. J. Clim. 24 (22), 5935–5950. doi:10.1175/JCLI-D-11-00134.1
Martin, E. R., and Schumacher, C. (2012). The relationship between tropical warm pool precipitation, sea surface temperature, and large-scale vertical motion in IPCC AR4 models. J. Atmos. Sci. 69 (1), 185–194. doi:10.1175/JAS-D-11-0104.1
McCabe, G. J., and Muller, R. A. (2002). Effects of ENSO on weather-type frequencies and properties at New Orleans, Louisiana, USA. Clim. Res. 20 (2), 95–105. doi:10.3354/cr020095
McCabe, G. J., Palecki, M. A., and Betancourt, J. L. (2004). Pacific and Atlantic Ocean influences on multidecadal drought frequency in the United States. Proc. Natl. Acad. Sci. U. S. A. 101 (12), 4136–4141. doi:10.1073/pnas.0306738101
McGregor, G. (2017). Hydroclimatology, modes of climatic variability and stream flow, lake and groundwater level variability: A progress report. Prog. Phys. Geogr. Earth Environ. 41 (4), 496–512. doi:10.1177/0309133317726537
Meehl, G. A., Hu, A., Arblaster, J. M., Fasullo, J., and Trenberth, K. E. (2013). Externally forced and internally generated decadal climate variability associated with the Interdecadal Pacific Oscillation. J. Clim. 26 (18), 7298–7310. doi:10.1175/JCLI-D-12-00548.1
Méndez, M., and Magaña, V. (2010). Regional aspects of prolonged meteorological droughts over Mexico and Central America. J. Clim. 23 (5), 1175–1188. doi:10.1175/2009JCLI3080.1
Mihunov, V. V., Lam, N. S. N., Zou, L., Rohli, R. V., Bushra, N., Reams, M. A., et al. (2018). Community resilience to drought hazard in the south-central United States. Ann. Am. Assoc. Geogr. 108 (3), 739–755. doi:10.1080/24694452.2017.1372177
Mills, C. M., and Walsh, J. E. (2013). Seasonal variation and spatial patterns of the atmospheric component of the Pacific decadal oscillation. J. Clim. 26 (5), 1575–1594. doi:10.1175/JCLI-D-12-00264.1
Mishra, A. K., Singh, V. P., and Ozger, M. (2011). Seasonal streamflow extremes in Texas river basins: Uncertainty, trends, and teleconnections. J. Geophys. Res. 116, D08108. doi:10.1029/2010JD014597
Misra, V., Li, H., and Kozar, M. (2014). The precursors in the Intra-Americas Seas to seasonal climate variations over North America. J. Geophys. Res. Oceans 119 (5), 2938–2948. doi:10.1002/2014JC009911
Mo, K. C. (2010). Interdecadal modulation of the impact of ENSO on precipitation and temperature over the United States. J. Clim. 23 (13), 3639–3656. doi:10.1175/2010JCLI3553.1
Mo, K. C., and Livezey, R. E. (1986). Tropical-extratropical geopotential height teleconnections during the northern hemisphere winter. Mon. Weather Rev. 114 (12), 2488–2515. doi:10.1175/1520-0493(1986)114<2488:TEGHTD>2.0.CO;2
Mo, K. C., Schemm, J. K. E., and Yoo, S. H. (2009). Influence of ENSO and the atlantic multidecadal oscillation on drought over the United States. J. Clim. 22 (22), 5962–5982. doi:10.1175/2009JCLI2966.1
Mokhov, I. I., and Smirnov, D. A. (2006). El niño–southern oscillation drives North Atlantic oscillation as revealed with nonlinear techniques from climatic indices. Geophys. Res. Lett. 33 (3), L03708. doi:10.1029/2005GL024557
Moon, J.- Y., Wang, B., and Ha, K.- J. (2011). ENSO regulation of MJO teleconnection. Clim. Dyn. 37 (5–6), 1133–1149. doi:10.1007/s00382-010-0902-3
Moon, J.- Y., Wang, B., and Ha, K.- J. (2012). MJO modulation on 2009/10 winter snowstorms in the United States. J. Clim. 25 (3), 978–991. doi:10.1175/jcli-d-11-00033.1
Muñoz, E., and Enfield, D. (2011). The boreal spring variability of the Intra-Americas low-level jet and its relation with precipitation and tornadoes in the eastern United States. Clim. Dyn. 36, 247–259. doi:10.1007/s00382-009-0688-3
Munroe, R., Crawford, T., and Curtis, S. (2014). Geospatial analysis of space-time patterning of ENSO forced daily precipitation distributions in the Gulf of Mexico. Prof. Geogr. 66 (1), 91–101. doi:10.1080/00330124.2013.765291
National Conference of State Legislatures (2018). Federal and state recognized tribes. Available at: http://www.ncsl.org/research/state-tribal-institute/list-of-federal-and-state-recognized-tribes.aspx (Last accessed: April 20, 2022).
Naumann, G., Alfieri, L., Wyser, K., Mentaschi, L., Betts, R. A., Carrao, H., et al. (2018). Global changes in drought conditions under different levels of warming. Geophys. Res. Lett. 45 (7), 3285–3296. doi:10.1002/2017GL076521
Newman, M., Alexander, M. A., Ault, T. R., Cobb, K. M., Deser, C., Di Lorenzo, E., et al. (2016). The pacific decadal oscillation, revisited. J. Clim. 29 (12), 4399–4427. doi:10.1175/JCLI-D-15-0508.1
Newman, M., Compo, G. P., and Alexander, M. A. (2003). ENSO-Forced variability of the pacific decadal oscillation. J. Clim. 16 (23), 3853–3857. doi:10.1175/1520-0442(2003)016<3853:EVOTPD>2.0.CO;2
Nigam, S., Guan, B., and Ruiz-Barradas, A. (2011). Key role of the atlantic multidecadal oscillation in 20th century drought and wet periods over the Great Plains. Geophys. Res. Lett. 38, L16713. doi:10.1029/2011GL048650
Ning, L., and Bradley, R. S. (2016). NAO and PNA influences on winter temperature and precipitation over the eastern United States in CMIP5 GCMs. Clim. Dyn. 46 (3–4), 1257–1276. doi:10.1007/s00382-015-2643-9
Oglesby, R. J., and Erickson, D. J. (1989). Soil moisture and the persistence of North American drought. J. Clim. 2 (11), 1362–1380. doi:10.1175/1520-0442(1989)002<1362:SMATPO>2.0.CO;2
Ó’hUallacháin, B. (2008). Regional growth transition clubs in the United States. Pap. Reg. Sci. 87 (1), 33–53. doi:10.1111/j.1435-5957.2007.00151.x
Pan, Z., Liu, X., Kumar, S., Gao, Z., and Kinter, J. (2013). Intermodel variability and mechanism attribution of central and southeastern US anomalous cooling in the twentieth century as simulated by CMIP5 models. J. Clim. 26 (17), 6215–6237. doi:10.1175/JCLI-D-12-00559.1
Pan, Z., Shi, C., Kumar, S., and Gao, Z. (2017). North Pacific SST forcing on the central United States “warming hole” as simulated in CMIP5 coupled historical and uncoupled AMIP experiments. Atmosphere-Ocean 55 (1), 57–77. doi:10.1080/07055900.2016.1261690
Pascolini-Campbell, M., Seager, R., Pinson, A., and Cook, B. I. (2017). Covariability of climate and streamflow in the Upper Rio Grande from interannual to interdecadal timescales. J. Hydrol.- Reg. Stud. 13 (2017), 58–71. doi:10.1016/j.ejrh.2017.07.007
Patricola, C. M., Saravanan, R., and Chang, P. (2014). The impact of the El niño-southern oscillation and atlantic meridional mode on seasonal atlantic tropical cyclone activity. J. Clim. 27 (14), 5311–5328. doi:10.1175/JCLI-D-13-00687.1
Piazza, B. P., LaPeyre, M. K., and Keim, B. D. (2010). Relating large-scale climate variability to local species abundance: ENSO forcing and shrimp in Breton sound, Louisiana, USA. Clim. Res. 42 (3), 195–207. doi:10.3354/cr00898
Pielke, R. A., and Landsea, C. N. (1999). La Niña, El Niño, and atlantic hurricane damages in the United States. B. Am. Meteorol. Soc. 80 (10), 2027–2034. doi:10.1175/1520-0477(1999)080<2027:LNAENO>2.0.CO;2
Poore, R. Z., DeLong, K. L., Richey, J. N., and Quinn, T. M. (2009). Evidence of multidecadal climate variability and the Atlantic Multidecadal Oscillation from a Gulf of Mexico sea-surface temperature-proxy record. Geo-Mar. Lett. 29 (6), 477–484. doi:10.1007/s00367-009-0154-6
Power, S., Lengaigne, M., Capotondi, A., Khodri, M., Vialard, J., Jebri, B., et al. (2021). Decadal climate variability in the tropical Pacific: Characteristics, causes, predictability, and prospects. Science 374 (6563), eaay9165. doi:10.1126/science.aay9165
Qin, M., Dai, A., and Hua, W. (2020). Quantifying contributions of internal variability and external forcing to Atlantic multidecadal variability since 1870. Geophys. Res. Lett. 47 (22), e2020GL089504. doi:10.1029/2020GL089504
Rajagopalan, B., Cook, E., Lall, U., and Ray, B. K. (2000). Spatiotemporal variability of ENSO and SST teleconnections to summer drought over the United States during the twentieth century. J. Clim. 13 (24), 4244–4255. doi:10.1175/1520-0442(2000)013<4244:SVOEAS>2.0.CO;2
Rasmusson, E. M., and Carpenter, T. A. (1982). Variations in tropical sea surface temperature and surface wind fields associated with the Southern Oscillation/El Niño. Mon. Weather Rev. 110 (5), 354–384. doi:10.1175/1520-0493(1982)110<0354:VITSST>2.0.CO;2
Renwick, J. A., and Wallace, J. M. (1996). Relationships between north Pacific wintertime blocking, El Niño, and the PNA pattern. Mon. Weather Rev. 124 (9), 2071–2076. doi:10.1175/1520-0493(1996)124<2071:RBNPWB>2.0.CO;2
Rodgers, K., Roland, V., Hoos, A., Crowley-Ornelas, E., and Knight, R. (2020). An analysis of streamflow trends in the southern and southeastern US from 1950–2015. Water 12 (12), 3345. doi:10.3390/w12123345
Rodionov, S., and Assel, R. A. (2003). Winter severity in the Great Lakes region: A tale of two oscillations. Clim. Res. 24 (1), 19–31. doi:10.3354/cr024019
Rodney, M., Lin, H., and Derome, J. (2013). Subseasonal prediction of wintertime North American surface air temperature during strong MJO events. Mon. Weather Rev. 141 (8), 2897–2909. doi:10.1175/MWR-D-12-00221.1
Rodwell, M. J., Rowell, D. P., and Folland, C. K. (1999). Oceanic forcing of the wintertime North Atlantic oscillation and European climate. Nature 398 (6725), 320–323. doi:10.1038/18648
Rogers, J. C., and Coleman, J. S. M. (2003). Interactions between the atlantic multidecadal oscillation, El Niño/La Niña, and the PNA in winter Mississippi valley stream flow. Geophys. Res. Lett. 30 (10), 1518. doi:10.1029/2003GL017216
Rogers, J. C. (1990). Patterns of low-frequency monthly sea level pressure variability (1899–1986) and associated wave cyclone frequencies. J. Clim. 3 (12), 1364–1379. doi:10.1175/1520-0442(1990)003<1364:POLFMS>2.0.CO;2
Rogers, J. C., and Rohli, R. V. (1991). Florida citrus freezes and polar anticyclones in the Great-Plains. J. Clim. 4 (11), 1103–1113. doi:10.1175/1520-0442(1991)004<1103:FCFAPA>2.0.CO;2
Rogers, J. C. (1984). The association between the north atlantic oscillation and the southern oscillation in the Northern hemisphere. Mon. Weather Rev. 112 (10), 1999–2015. doi:10.1175/1520-0493(1984)112<1999:TABTNA>2.0.CO;2
Rogers, J., and McHugh, M. J. (2002). On the separability of the north atlantic oscillation and arctic oscillation. Clim. Dyn. 19 (7), 599–608. doi:10.1007/s00382-002-0247-7
Rohli, R. V., and Rogers, J. C. (1993). Atmospheric teleconnections and citrus freezes in the southern United States. Phys. Geogr. 14 (1), 1–15. doi:10.1080/02723646.1993.10642464
Ropelewski, C. F., and Halpert, M. S. (1987). Global and regional scale precipitation patterns associated with the El-Niño Southern Oscillation. Mon. Weather Rev. 115 (8), 1606–1626. doi:10.1175/1520-0493(1987)115<1606:GARSPP>2.0.CO;2
Ropelewski, C. F., and Halpert, M. S. (1986). North American precipitation and temperature patterns associated with the El Niño southern oscillation (ENSO). Mon. Weather Rev. 114 (12), 2352–2362. doi:10.1175/1520-0493(1986)114<2352:NAPATP>2.0.CO;2
Ropelewski, C. F., and Halpert, M. S. (1989). Precipitation patterns associated with the high index phase of the Southern Oscillation. J. Clim. 2 (3), 268–284. doi:10.1175/1520-0442(1989)002<0268:PPAWTH>2.0.CO;2
Ropelewski, C. F., and Halpert, M. S. (1996). Quantifying southern oscillation-precipitation relationships. J. Clim. 9 (5), 1043–1059. doi:10.1175/1520-0442(1996)009<1043:QSOPR>2.0.CO;2
Ropelewski, C. F., and Jones, P. D. (1987). An extension of the Tahiti–Darwin southern oscillation index. Mon. Weather Rev. 115 (9), 2161–2165. doi:10.1175/1520-0493(1987)115<2161:AEOTTS>2.0.CO;2
Ruiz-Barradas, A., and Nigam, S. (2005). Warm season rainfall variability over the U.S. Great Plains in observations, NCEP and ERA-40 reanalyses, and NCAR and NASA atmospheric model simulations. J. Clim. 18 (11), 1808–1830. doi:10.1175/JCLI3343.1
Sadeghi, S., Tootle, G., Elliott, E., Lakshmi, V., Therrell, M., Kam, J., et al. (2019). Atlantic Ocean sea surface temperatures and southeast United States streamflow variability: Associations with the recent multi-decadal decline. J. Hydrol. X. 576, 422–429. doi:10.1016/j.jhydrol.2019.06.051
Saenger, C., Cohen, A. L., Oppo, D. W., Halley, R. B., and Carilli, J. E. (2009). Surface-temperature trends and variability in the low-latitude North Atlantic since 1552. Nat. Geosci. 2 (7), 492–495. doi:10.1038/ngeo552
Sagarika, S., Kalra, A., and Ahmad, S. (2015). Interconnections between oceanic–atmospheric indices and variability in the US streamflow. J. Hydrol. X. 525, 724–736. doi:10.1016/j.jhydrol.2015.04.020
Salinger, M. J., Renwick, J. A., and Mullan, A. B. (2001). Interdecadal Pacific oscillation and south Pacific climate. Int. J. Climatol. 21 (14), 1705–1721. doi:10.1002/joc.691
Sanchez-Rubio, G., Perry, H. M., Biesiot, P. M., Johnson, D. R., and Lipcius, R. N. (2011). Oceanic-atmospheric modes of variability and their influence on riverine input to coastal Louisiana and Mississippi. J. Hydrol. X. 396 (1–2), 72–81. doi:10.1016/j.jhydrol.2010.10.034
Schlesinger, M. E., and Ramankutty, N. (1994). An oscillation in the global climate system of period 65–70 years. Nature 367 (6465), 723–726. doi:10.1038/367723a0
Schmidt, N., Lipp, E. K., Rose, J. B., and Luther, M. E. (2001). ENSO influences on seasonal rainfall and river discharge in Florida. J. Clim. 14 (4), 615–628. doi:10.1175/1520-0442(2001)014<0615:EIOSRA>2.0.CO;2
Schmidt, N., and Luther, M. E. (2002). ENSO impacts on salinity in Tampa Bay, Florida. Estuaries 25 (2002), 976–984. doi:10.1007/BF02691345
Schneider, N., and Cornuelle, B. D. (2005). The forcing of the Pacific decadal oscillation. J. Clim. 18 (21), 4355–4373. doi:10.1175/JCLI3527.1
Schreck, C. J., Cordeira, J. M., and Margolin, D. (2013). Which MJO events affect North American temperatures? Mon. Weather Rev. 141 (11), 3840–3850. doi:10.1175/MWR-D-13-00118.1
Schubert, S. M., Suarez, J., Pegion, P. J., Koster, R. D., and Bacmeister, J. T. (2004). Causes of long-term drought in the U.S. Great Plains. J. Clim. 17 (3), 485–503. doi:10.1175/1520-0442(2004)017<0485:COLDIT>2.0.CO;2
Shakun, J. D., and Shaman, J. (2009). Tropical origins of North and south pacific decadal variability. Geophys. Res. Lett. 36 (19), L19711. doi:10.1029/2009GL040313
Shapiro, L. J. (1987). Month-to-month variability of the Atlantic tropical circulation and its relationship to tropical storm formation. Mon. Weather Rev. 115 (11), 1598–1614. doi:10.1175/1520-0493(1987)115<2598:MTMVOT>2.0.CO;2
Sheridan, S., and Lee, C. C. (2012). Synoptic climatology and the analysis of atmospheric teleconnections. Prog. Phys. Geogr. Earth Environ. 36 (4), 548–557. doi:10.1177/0309133312447935
Simmons, A. J., Wallace, J. M., and Branstator, G. W. (1983). Barotropic wave propagation and instability, and atmospheric teleconnection patterns. J. Atmos. Sci. 40 (6), 1363–1392. doi:10.1175/1520-0469(1983)040<1363:BWPAIA>2.0.CO;2
Smith, S. R., Brolley, J., O’Brien, J. J., and Tartaglione, C. A. (2007). ENSO’s impact on regional U.S. hurricane activity. J. Clim. 20 (7), 1404–1414. doi:10.1175/JCLI4063.1
Sohngen, B., and Brown, S. (2006). The influence of conversion of forest types on carbon sequestration and other ecosystem services in the South Central United States. Ecol. Econ. 57 (4), 698–708. doi:10.1016/j.ecolecon.2005.06.001
Soulard, N., Lin, H., and Yu, B. (2019). The changing relationship between ENSO and its extratropical response patterns. Sci. Rep. 9, 6507. doi:10.1038/s41598-019-42922-3
Stahle, D. W., and Cleaveland, M. K. (1988). Texas drought history reconstructed and analyzed from 1698 and 1980. J. Clim. 1 (1), 59–74. doi:10.1175/1520-0442(1988)001<0059:TDHRAA>2.0.CO;2
Stan, C., Straus, D. M., Frederiksen, J. S., Lin, H., Maloney, E. D., and Schumacher, C. (2017). Review of tropical‐extratropical teleconnections on intraseasonal time scales. Rev. Geophys. 55 (4), 902–937. doi:10.1002/2016RG000538
Stenseth, N. C., Ottersen, G., Hurrell, J. W., Mysterud, A., Lima, M., Chan, K. S., et al. (2003). Review article. Studying climate effects on ecology through the use of climate indices: the north atlantic oscillation, El Niño southern oscillation and beyond. Proc. R. Soc. Lond. B 270 (1529), 2087–2096. doi:10.1098/rspb.2003.2415
Steptoe, H., Jones, S. E. O., and Fox, H. (2018). Correlations between extreme atmospheric hazards and global teleconnections: Implications for multihazard resilience. Rev. Geophys. 56 (1), 50–78. doi:10.1002/2017RG000567
Straus, D. M., and Shukla, J. (2002). Does ENSO force the PNA? J. Clim. 15 (17), 2340–2358. doi:10.1175/1520-0442(2002)015<2340:DEFTP>2.0.CO;2
Sun, C. J., Shanahan, T. M., and Partin, J. (2019). Controls on the isotopic composition of precipitation in the south-central United States. J. Geophys. Res. Atmos. 124 (14), 8320–8335. doi:10.1029/2018JD029306
Sun, J., and Wang, H. (2012). Changes of the connection between the summer North Atlantic oscillation and the east asian summer rainfall. J. Geophys. Res. 117, D08110. doi:10.1029/2012JD017482
Swain, S., Abeysundara, S., Hayhoe, K., and Stoner, A. M. K. (2017). Future changes in summer MODIS-based enhanced vegetation index for the South-Central United States. Ecol. Inf. 41 (2017), 64–73. doi:10.1016/j.ecoinf.2017.07.007
Sweet, W. V., and Zervas, C. (2011). Cool-season sea level anomalies and storm surges along the U.S. East Coast: Climatology and comparison with the 2009/10 El Niño. Mon. Weather Rev. 139 (7), 2290–2299. doi:10.1175/MWR-D-10-05043.1
Tanimoto, Y., Iwasaka, N., Hanawa, K., and Toba, Y. (1993). Characteristic variations of sea surface temperature with multiple time scales in the North Pacific. J. Clim. 6 (6), 1153–1160. doi:10.1175/1520-0442(1993)006<1153:CVOSST>2.0.CO;2
Taschetto, A. S., Rodrigues, R. R., Meehl, G. A., McGregor, S., and England, M. H. (2016). How sensitive are the Pacific–tropical North Atlantic teleconnections to the position and intensity of El Niño-related warming? Clim. Dyn. 46 (5), 1841–1860. doi:10.1007/s00382-015-2679-x
Terjung, W. (1976). Climatology for geographers. Ann. Assoc. Am. Geogr. 66 (2), 199–220. doi:10.1111/j.1467-8306.1976.tb01086.x
Thompson, D. B., and Roundy, P. E. (2013). The relationship between the Madden-Julian Oscillation and US violent tornado outbreaks in the spring. Mon. Weather Rev. 141 (6), 2087–2095. doi:10.1175/MWR-D-12-00173.1
Thompson, D. W. J., and Wallace, J. M. (1998). The Arctic Oscillation signature in the wintertime geopotential height and temperature fields. Geophys. Res. Lett. 25 (9), 1297–1300. doi:10.1029/98GL00950
Thompson, D. W., and Wallace, J. M. (2000). Annular modes in the extratropical circulation. Part I: Month-to-month variability. J. Clim. 13 (5), 1000–1016. doi:10.1175/1520-0442(2000)013<1000:AMITEC>2.0.CO;2
Thompson, D. W., Wallace, J. M., and Hegerl, G. C. (2000). Annular modes in the extratropical circulation. Part II: Trends. J. Clim. 13 (5), 1018–1036. doi:10.1175/1520-0442(2000)013<1018:AMITEC>2.0.CO;2
Ting, M., Kushnir, Y., and Li, C. (2014). North Atlantic multidecadal SST oscillation: External forcing versus internal variability. J. Mar. Syst. 133, 27–38. doi:10.1016/j.jmarsys.2013.07.006
Ting, M., Kushnir, Y., Seager, R., and Li, C. (2009). Forced and internal twentieth-century SST trends in the North Atlantic. J. Clim. 22 (6), 1469–1481. doi:10.1175/2008JCLI2561.1
Tokioka, T., Yamazaki, K., Kitoh, A., and Ose, T. (1988). The equatorial 30–60 day oscillation and the Arakawa-Schubert penetrative cumulus parameterization. J. Meteorological Soc. Jpn. 66 (6), 883–901. doi:10.2151/jmsj1965.66.6_883
Tolan, J. M. (2007). El niño-southern oscillation impacts translated to the watershed scale: Estuarine salinity patterns along the Texas Gulf coast, 1982 to 2004. Estuar. Coast. Shelf Sci. 72 (1–2), 247–260. doi:10.1016/j.ecss.2006.10.018
Tootle, G. A., Piechota, T. C., and Singh, A. (2005). Coupled oceanic-atmospheric variability and U.S. streamflow. Water Resour. Res. 41 (12), W12408. doi:10.1029/2005WR004381
Torbenson, M. C. A., Stahle, D. W., Howard, I. M., Burnette, D. J., Villanueva-Diaz, J., Cook, E. R., et al. (2019). Multidecadal modulation of the ENSO teleconnection to precipitation and tree growth over subtropical North America. Paleoceanogr. Paleoclimatology 34 (5), 886–900. doi:10.1029/2018PA003510
Torbenson, M. C. A., and Stahle, D. W. (2018). The relationship between cool and warm season moisture over the central United States, 1685–2015. J. Clim. 31 (19), 7909–7924. doi:10.1175/JCLI-D-17-0593.1
Toride, K., and Hakim, G. J. (2021). Influence of low-frequency PNA variability on MJO teleconnections to North American atmospheric river activity. Geophys. Res. Lett. 48, e2021GL094078. doi:10.1029/2021GL094078
Tranchant, B., Pujol, I., Di Lorenzo, E., and Legeais, J.- F. (2019). the North pacific gyre oscillation. J. Oper. Oceanogr. 12 (S1), s29–s31.
Trenberth, K. E. (1984). Signal versus noise in the southern oscillation. Mon. Weather Rev. 112 (2), 326–332. doi:10.1175/1520-0493(1984)112<0326:SVNITS>2.0.CO;2
Trenberth, K. E. (1976). Spatial and temporal variations of the southern oscillation. Q. J. R. Meteorol. Soc. 102 (433), 639–653. doi:10.1002/qj.49710243310
Trenberth, K. E., and Stepaniak, D. P. (2001). Indices of El Niño evolution. J. Clim. 14 (8), 1697–1701. doi:10.1175/1520-0442(2001)014<1697:LIOENO>2.0.CO;2
Trenberth, K. E. (1997). The definition of El Niño. B. Am. Meteorol. Soc. 78 (12), 2771–2777. doi:10.1175/1520-0477(1997)078<2771:TDOENO>2.0.CO;2
Trigo, R. M., Osborn, T. J., and Corte-Real, J. M. (2002). The North Atlantic Oscillation influence on Europe: Climate impacts and associated physical mechanisms. Clim. Res. 20 (1), 9–17. doi:10.3354/cr020009
Trouet, V., Harley, G. L., and Domínguez-Delmás, M. (2016). Shipwreck rates reveal Caribbean tropical cyclone response to past radiative forcing. Proc. Natl. Acad. Sci. U. S. A. 113 (12), 3169–3174. doi:10.1073/pnas.1519566113
Vaideanu, P., Dima, M., and Voiculescu, M. (2018). Atlantic Multidecadal Oscillation footprint on global high cloud cover. Theor. Appl. Climatol. 134 (3–4), 1245–1256. doi:10.1007/s00704-017-2330-3
van Loon, H., and Rogers, J. C. (1978). Seesaw in winter temperatures between Greenland and northern Europe. Part I: General description. Mon. Weather Rev. 106 (3), 296–310. doi:10.1175/1520-0493(1978)106<0296:TSIWTB>2.0.CO;2
van Loon, H., and Rogers, J. C. (1981). The Southern Oscillation. Part II: Associations with changes in the middle troposphere in the northern winter. Mon. Weather Rev. 109 (6), 1163–1168. doi:10.1175/1520-0493(1981)109<1163:TSOPIA>2.0.CO;2
Vega, A. J., Rohli, R. V., and Henderson, K. G. (1998). The Gulf of Mexico mid-tropospheric response to El Niño and La Niña forcing. Clim. Res. 10 (2), 115–125. doi:10.3354/cr010115
Villarini, G., Smith, J. A., Vitolo, R., and Stephenson, D. B. (2013). On the temporal clustering of US floods and its relationship to climate teleconnection patterns. Int. J. Climatol. 33 (3), 629–640. doi:10.1002/joc.3458
Vimont, D. J., and Kossin, J. P. (2007). The Atlantic meridional mode and hurricane activity. Geophys. Res. Lett. 34 (7), L07709. doi:10.1029/2007GL029683
Visbeck, M. H., Hurrell, J. W., Polvani, L., and Cullen, H. M. (2001). the North atlantic oscillation: Past, present, and future. Proc. Natl. Acad. Sci. U. S. A. 98 (23), 12876–12877. doi:10.1073/pnas.231391598
Vitart, F. (2009). Impact of the Madden Julian Oscillation on tropical storms and risk of landfall in the ECMWF forecast system. Geophys. Res. Lett. 36, L15802. doi:10.1029/2009GL039089
von Reumont, J., Hetzinger, S., Garbe‐Schönberg, D., Manfrino, C., and Dullo, C. (2018). Tracking interannual‐to multidecadal‐scale climate variability in the Atlantic Warm Pool using central Caribbean coral data. Paleoceanogr. Paleoclimatology 33 (4), 395–411. doi:10.1002/2018PA003321
Walker, G. T., and Bliss, E. W. (1932). “World weather V,” in Memoirs of the Royal Meteorological Society. Berks, England: Royal Meteorological Soc, 44, 53–84.
Wallace, J. M., and Gutzler, D. S. (1981). Teleconnections in the geopotential height field during the Northern Hemisphere winter. Mon. Weather Rev. 109 (4), 784–812. doi:10.1175/1520-0493(1981)109<0784:TITGHF>2.0.CO;2
Wallace, J. M. (2000). North Atlantic Oscillation/annular mode: Two paradigms – one phenomenon. Q. J. R. Meteorol. Soc. 126 (564), 791–805. doi:10.1256/smsqj.56401
Walsh, J. E., Phillips, A. S., Portis, D. H., and Chapman, W. L. (2001). Extreme cold outbreaks in the United States and Europe, 1948–99. J. Clim. 14 (12), 2642–2658. doi:10.1175/1520-0442(2001)014<2642:ECOITU>2.0.CO;2
Wang, C., Enfield, D. B., Sang-ki, L., and Landsea, C. W. (2006). Influences of the atlantic warm pool on western hemisphere summer rainfall and atlantic hurricanes. J. Clim. 19 (12), 3011–3028. doi:10.1175/JCLI3770.1
Wang, C., Lee, S.- K., and Enfield, D. B. (2008a). Atlantic warm pool acting as a link between atlantic multidecadal oscillation and atlantic tropical cyclone activity. Geochem. Geophys. Geosyst. 9 (5), Q05V03. doi:10.1029/2007GC001809
Wang, C., Lee, S.- K., and Enfield, D. B. (2008b). Climate response to anomalously large and small Atlantic warm pools during the summer. J. Clim. 21 (11), 2437–2450. doi:10.1175/2007JCLI2029.1
Wang, J., Yang, B., Ljungqvist, F. C., Luterbacher, J., Osborn, T. J., Briffa, K. R., et al. (2017). Internal and external forcing of multidecadal Atlantic climate variability over the past 1, 200 years. Nat. Geosci. 10 (7), 512–517. doi:10.1038/NGEO2962
Wang, L., and Robertson, A. W. (2019). Week 3–4 predictability over the United States assessed from two operational ensemble prediction systems. Clim. Dyn. 52 (9–10), 5861–5875. doi:10.1007/s00382-018-4484-9
Wang, S.- Y. S., Huang, W.- R., Hsu, H.- H., and Gillies, R. R. (2015). Role of the strengthened El Niño teleconnection in the may 2015 floods over the southern Great Plains. Geophys. Res. Lett. 42 (19), 8140–8146. doi:10.1002/2015GL065211
Wang, X., Brown, P. M., Zhang, Y., and Song, L. (2011). Imprint of the atlantic multidecadal oscillation on tree-ring widths in northeastern Asia since 1568. PloS One 6 (7), e22740. doi:10.1371/journal.pone.0022740
Wang, Y., Hu, K., Huang, G., and Tao, W. (2021). Asymmetric impacts of El Niño and La Niña on the Pacific–North American teleconnection pattern: The role of subtropical jet stream. Environ. Res. Lett. 16 (11), 114040. doi:10.1088/1748-9326/ac31ed
Watanabe, M., and Tatebe, H. (2019). Reconciling roles of sulphate aerosol forcing and internal variability in Atlantic multidecadal climate changes. Clim. Dyn. 53 (7), 4651–4665. doi:10.1007/s00382-019-04811-3
Weaver, S. J., and Nigam, S. (2008). Variability of the Great Plains low-level jet: Large-scale circulation context and hydroclimate impacts. J. Clim. 21 (7), 1532–1551. doi:10.1175/2007JCLI1586.1
Weaver, S. J., Schubert, S., and Wang, H. (2009). Warm season variations in the low-level circulation and precipitation over the central United States in observations, AMIP simulations, and idealized SST experiments. J. Clim. 22 (20), 5401–5420. doi:10.1175/2009JCLI2984.1
Wheeler, M. C., and Hendon, H. H. (2004). An all-season real-time multivariate MJO index: Development of an index for monitoring and prediction. Mon. Weather Rev. 132 (8), 1917–1932. doi:10.1175/1520-0493(2004)132<1917:AARMMI>2.0.CO;2
Wise, E. K., Wrzesien, M. L., Dannenberg, M. P., and McGinnis, D. L. (2015). Cool-season precipitation patterns associated with teleconnection interactions in the United States. J. Appl. Meteorol. Climatol. 54 (2), 494–505. doi:10.1175/JAMC-D-14-0040.1
Wolter, K., and Timlin, M. S. (2011). El Niño/Southern Oscillation behaviour since 1871 as diagnosed in an extended multivariate ENSO index (MEI.ext). Int. J. Climatol. 31 (7), 1074–1087. doi:10.1002/joc.2336
Wu, S., Liu, Z., Zhang, R., and Delworth, T. L. (2011). On the observed relationship between the pacific decadal oscillation and the atlantic multi-decadal oscillation. J. Oceanogr. 67 (1), 27–35. doi:10.1007/s10872-011-0003-x
Yang, Q., Ma, Z., Wu, P., Klingaman, N. P., and Zhang, L. (2019). Interdecadal seesaw of precipitation variability between North China and the southwest United States. J. Clim. 32 (10), 2951–2968. doi:10.1175/JCLI-D-18-0082.1
Yeh, S.- W., Kug, J.- S., Dewitte, B., Kwon, M.- H., Kirtman, B. P., and Jin, F.- F. (2009). El Niño in a changing climate. Nature 461 (7263), 511–514. doi:10.1038/nature08316
Yu, B., Lin, H., and Soulard, N. (2019). A comparison of North American surface temperature and temperature extreme anomalies in association with various atmospheric teleconnection patterns. Atmos. (Basel). 10 (4), 172. doi:10.3390/atmos10040172
Yu, B., Lin, H., Wu, Z. W., and Merryfield, W. J. (2018). The Asian-Bering-North American teleconnection: Seasonality, maintenance, and climate impact on North America. Clim. Dyn. 50 (5–6), 2023–2038. doi:10.1007/s00382-017-3734-6
Yu, J.-Y., Lu, M.- M., and Kim, S. T. (2012a). A change in the relationship between tropical central Pacific SST variability and the extratropical atmosphere around 1990. Environ. Res. Lett. 7, 034025. doi:10.1088/1748-9326/7/3/034025
Yu, J. -Y., Zou, Y. H., Kim, S. T., and Lee, T. (2012b). The changing impact of El Niño on US winter temperatures. Geophys. Res. Lett. 39, L15702. doi:10.1029/2012GL052483
Yu, L. J., Zhong, S. Y., Heilman, W. E., and Bian, X. D. (2017). A comparison of the effects of El Niño and El Niño Modoki on subdaily extreme precipitation occurrences across the contiguous United States. J. Geophys. Res.─Atmos. 122 (14), 7401–7415. doi:10.1002/2017JD026683
Yu, L., Zhong, S., Bian, X., Heilman, W. E., and Andresen, J. A. (2014). Temporal and spatial variability of frost‐free seasons in the Great Lakes region of the United States. Int. J. Climatol. 34 (13), 3499–3514. doi:10.1002/joc.3923
Zhang, C. D. (2005). Madden-julian oscillation. Rev. Geophys. 43 (2), RG2003. doi:10.1029/2004RG000158
Zhang, J., Liu, Y. S., Sun, C., Li, J. P., Ding, R. Q., Xie, F., et al. (2021). On the connection between AMOC and observed land precipitation in northern hemisphere: A comparison of the AMOC indicators. Clim. Dyn. 56 (1–2), 651–664. doi:10.1007/s00382-020-05496-9
Zhang, R., and Delworth, T. L. (2007). Impact of the atlantic multidecadal oscillation on North pacific climate variability. Geophys. Res. Lett. 34 (23), L23708. doi:10.1029/2007GL031601
Zhang, R., Sutton, R., Danabasoglu, G., Kwon, Y. O., Marsh, R., Yeager, S. G., et al. (2019). A review of the role of the Atlantic meridional overturning circulation in Atlantic multidecadal variability and associated climate impacts. Rev. Geophys. 57 (2), 316–375. doi:10.1029/2019RG000644
Zhang, W. J., Jin, F. F., Ren, H. L., Li, J. P., and Zhao, J. X. (2012). Differences in teleconnection over the North Pacific and rainfall shift over the USA associated with two types of El Niño during boreal autumn. J. Meteorological Soc. Jpn. 90 (4), 535–552. doi:10.2151/jmsj.2012-407
Zhang, X., Wang, J., Zwiers, F. W., and Groisman, P. Y. (2010). The influence of large-scale climate variability on winter maximum daily precipitation over North America. J. Clim. 23 (11), 2902–2915. doi:10.1175/2010JCLI3249.1
Zhang, Y. C., and Liang, X. S. (2021). The causal role of South China Sea on the Pacific-North American teleconnection pattern. Clim. Dyn. doi:10.1007/s00382-021-06070-7
Zheng, C., Chang, E. K.- M., Kim, H.- M., Zhang, M., and Wang, W. (2018). Impacts of the Madden–Julian oscillation on storm-track activity, surface air temperature, and precipitation over North America. J. Clim. 31 (15), 6113–6134. doi:10.1175/JCLI-D-17-0534.1
Zhou, S. T., L'Heureux, M., Weaver, S., and Kumar, A. (2012). A composite study of the MJO influence on the surface air temperature and precipitation over the continental United States. Clim. Dyn. 38 (7–8), 1459–1471. doi:10.1007/s00382-011-1001-9
Zhou, W., Yang, D., Xie, S.- P., and Ma, J. (2020). Amplified Madden–Julian oscillation impacts in the Pacific–North America region. Nat. Clim. Chang. 10 (7), 654–660. doi:10.1038/s41558-020-0814-0
Keywords: El Niño/Southern Oscillation (ENSO), North Atlantic Oscillation (NAO), Arctic Oscillation (AO), Atlantic Multidecadal Variability (AMV), Pacific Decadal Oscillation (PDO), Pacific/North American (PNA) pattern, Madden-Julian Oscillation (MJO), climate impacts
Citation: Rohli RV, Snedden GA, Martin ER and DeLong KL (2022) Impacts of ocean-atmosphere teleconnection patterns on the south-central United States. Front. Earth Sci. 10:934654. doi: 10.3389/feart.2022.934654
Received: 02 May 2022; Accepted: 15 July 2022;
Published: 23 August 2022.
Edited by:
Bin Yu, Environment and Climate Change, CanadaReviewed by:
Jin-Yi Yu, University of California, Irvine, United StatesShengping He, University of Bergen, Norway
Copyright © 2022 Rohli, Snedden, Martin and DeLong. This is an open-access article distributed under the terms of the Creative Commons Attribution License (CC BY). The use, distribution or reproduction in other forums is permitted, provided the original author(s) and the copyright owner(s) are credited and that the original publication in this journal is cited, in accordance with accepted academic practice. No use, distribution or reproduction is permitted which does not comply with these terms.
*Correspondence: Robert V. Rohli, cm9obGlAbHN1LmVkdQ==; Gregg A. Snedden, c25lZGRlbmdAdXNncy5nb3Y=