- 1Guangzhou Marine Geological Survey, China Geological Survey, Guangzhou, China
- 2Southern Marine Science and Engineering Guangdong Laboratory (Guangzhou), Guangzhou, China
- 3National Engineering Research Center of Gas Hydrate Exploration and Development, Guangzhou, China
The study of deepwater channels is important for the understanding of the sedimentary evolution mechanism and the sedimentary process of the marginal sea. In 2019, thick pore-filling gas hydrate with high saturation was firstly discovered in the Quaternary sands of the Qiongdongnan Basin (QDNB), which expanded the reservoir types of gas hydrates in the South China Sea. However, the distribution of sand-related channels is not well characterized, which limits the ability to predict sand reservoirs with gas hydrate. Using integrated 2D/3D seismic, multi-beam, well logging, and coring data, the current study documents the distribution characteristics of channel systems in the Quaternary strata and discusses their controlling factors. The integrated analysis shows that the channel-related sedimentary facies include channel-filling facies, levee facies, crevasse splay facies, and lobes facies. A total of six periods of channel systems is identified in the Quaternary strata. There are obvious distribution differences between the Channel 1 and Channel 3 systems when comparing the western, middle, and eastern sections: the channels in the western and eastern sections are mainly dominated by near straight V-shaped channels, while the middle section mainly consists of large braided channels, where channel-levee sedimentary facies developed. Compared with the distribution of the Central Canyon that developed in the Miocene, the Channel 1 and Channel 3 systems in the western section show southward migration since the Miocene. The distribution and evolution of Quaternary channels were likely collectively controlled by seafloor morphology, tectonic movement, sea-level fluctuations, and provenance supply. Tectonic movement controls seafloor morphology, which directly controls the flow of channels and their distribution characteristics; provenance supply determines the scale and sedimentary characteristics of each channel. The periodic changes in sea-level determine the evolution of multi-stage channel systems. This study has implications for the prediction of gas hydrate–bearing sands in the Quaternary QDNB and deepens our understanding of the Quaternary tectonic and sedimentary evolution in the QDNB.
1 Introduction
Gas hydrates are crystalline compounds of water and gas molecules, mainly methane, which form under stable high-pressure and low-temperature conditions (Sloan, 2003), and are regarded as a promising new clean energy resource (Moridis et al., 2013; Collett, 2014; Chong et al., 2016). Being able to find highly saturated gas hydrate ore is a crucial link in the gas hydrate exploration and development process. Of the total quantity of gas hydrate resources available globally, 97% is mainly distributed in the deep-water sedimentary system, such as mass transport deposits, deep-water turbidity fans, and channel-levee facies (Yu and Zhang, 2005; Behseresht and Bryant, 2012; Liang et al., 2018; Santra et al., 2020). Theoretically, the high deposition rate of coarse grain deposits not only provides a good fluid transport pathway for the formation of gas hydrates, but also acts as the perfect reservoir for its accumulation (Sha et al., 2009; Egawa et al., 2015). For gas hydrate production, coarse grain reservoirs have good porosity, high permeability, and high stability. This reservoir type is the priority target for mining, as was the case with the great breakthroughs in sandstone gas hydrate exploration in the Mallik delta and Alaska continental slope. Many countries, such as Japan, the United States, Canada, South Korea, and India, are targeting coarse grain reservoirs for gas hydrate test production. Reservoirs of gas hydrate drilling areas with high investigation and research levels, such as the Nankai Trough and the Gulf of Mexico basin (Alaminos Canyon area, Walker Ridge area, and Green Canyon area) are located in the channel-levee facies or turbidity deposits (Uchida and Tsuji, 2004; Boswell et al., 2009; 2012; Scholz et al., 2012; Waite et al., 2019). Therefore, one of the important tasks for gas hydrate exploration is to find dominant sedimentary facies that might provide favorable reservoirs.
High saturation diffusion gas hydrates in the Quaternary sandy sediments were found for the first time during the gas hydrate drilling expedition of the Qiongdongnan Basin (hereinafter referred to as QDNB) in 2019. This tremendous breakthrough enriched the reservoir types of gas hydrate exploration in China. Finding potential high-quality sandy reservoirs requires the ability to better predict the distribution characteristics of sand bodies. Through the interpretation of high-resolution 3D seismic data of the drilling area in QDNB in 2019, it has been concluded that sand bodies with highly saturated gas hydrate belong to channel-levee facies deposition (Meng et al., 2021). At present, the study of Quaternary sediments in the QDNB is limited to the submarine shallow surface, because of its high scientific value in the study of monsoon evolution and events causing abrupt climate change (Xu et al., 2010; Wang et al., 2014; Huang et al., 2013; Hu et al., 2014; Liu et al., 2010; Wang et al., 2014; Yan et al., 2016). With the discovery of hydrate in the QDNB, the distribution, development, and formation mechanism of mass transported deposits (hereinafter referred to as MTDs) and their relationship with gas hydrate have also been studied (Meng et al., 2021; Cheng et al., 2021). The deepwater channel system is the main mode of sand transport, and controls the distribution of sand bodies. Unfortunately, the identification, controlling factors of sinuous channel in the Pleistocene strata of the limited 3D area in the southwest margin slope area of the QDNB has rarely been studied (Yuan et al., 2009; Yuan et al., 2010a, b; Wang et al., 2015), there has been no research on the distribution and evolutionary mechanism of channels in the Quaternary strata of the QDNB.
In addition, deep-sea sediments are the most precious carrier of information regarding the evolution of the Earth system. The QDNB in the northern part of the South China Sea (SCS) is a typical marginal sea basin, and its sediments record the dynamic processes of climate change, tectonic uplift, and sea-level change, as well as the sedimentary archives of the dynamic processes of the deep continental margin lithosphere (Covault et al., 2010; Lin, et al., 2015; Gong et al., 2016; Romans et al., 2016). Therefore, the study of Quaternary channel systems in the QDNB will not only provide guidance for the prediction of sand distribution, which is important for the prediction of highly saturated gas hydrate occurrences, but also deepen our current understanding of the sedimentary processes and evolution mechanism of the marginal sea (e.g., Matenco et al., 2013; Gong et al., 2016, Gong et al., 2018; Walsh et al., 2016).
Channel systems can develop on the slope, at the base of slope, and on the basin floor. channels tend to be shallower and exhibit a sinuous and distributary pattern on the basin floor (Weimer et al., 2006). Side-scan sonar image, bathymetry map, and 3D seismic data are often used to study the morphologies of channel systems on the seabed surface (Kenyon and Millington, 1995; Mitchum and Wach, 2002; Fildani and Normark, 2004). Amplitude extraction map and coherence map from 3D seismic are used to identify the channel shape and size (Saller et al., 2004). Most of previous work pay more attention to the channel morphologies, internal sedimentary characteristic, depositional model, sedimentary processes within sinuous channels, however, few work was done on the channel system evolution on basin level. This is one of the purposes of our study, hoping to bring some inspiration to similar basins or areas globally.
Therefore, the objectives of this study are to 1) identify the characteristics of Quaternary channels, 2) clarify the lateral distribution characteristics and evolution of channel systems in different periods, and 3) discuss the factors controlling the distribution of channel systems.
2 Geological Setting
The QDNB is located in the northwestern slope of the SCS (Figure 1A). This basin is adjacent to the Yinggehai Basin to the northwest, the slope of Hainan Island to the north, the Pearl River Mouth Basin to the northeast, and the Yongle Uplift to the south (Figure 1B). The QDNB mainly consists of five first-order tectonic units: the Northern Depression, the Northern Uplift, the Central Depression, the Southern Low Uplift, and the Southern Depression. The Quaternary channel systems in our study are mainly located in the Central Depression.
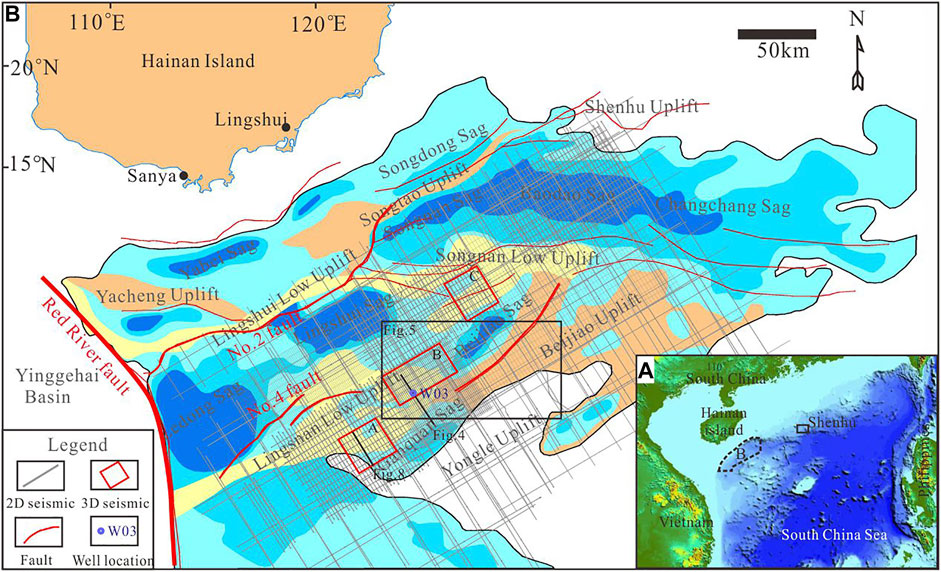
FIGURE 1. (A) The location of the QDNB. (B) The tectonic units of the QDNB. The gray lines denote 2D seismic lines, and the red rectangles denote 3D seismic areas.
The QDNB is a Cenozoic passive continental marginal basin, with a water depth ranging from 300 to 2,600 m and an area of about 8.3 × 104 km2. The QDNB mainly underwent two stages of tectonic evolution, the Eocene–Oligocene rifting stage and the Early Miocene-Quaternary thermal subsidence stage (Zhao et al., 2015). The filling sequences in the basin are mainly composed of Paleocene, Neogene, and Quaternary strata. From bottom to top, the Paleocene mainly contains Eocene and Oligocene strata (the Yacheng and Lingshui formations); the Neogene strata include Miocene (the Sanya, Meishan, and Huangliu formations) and Pliocene (the Yinggehai Formation) strata; and the Quaternary strata include the Ledong Formation (Figure 2). The Early Oligocene Yacheng Formation consists of marsh to coastal plain facies; the lower Lingshui Formation consists of fan delta facies; the upper Lingshui–Meishan formations consist of littoral to neritic facies; and the Huangliu–Ledong formations mainly consist of bathyal to abyssal facies.
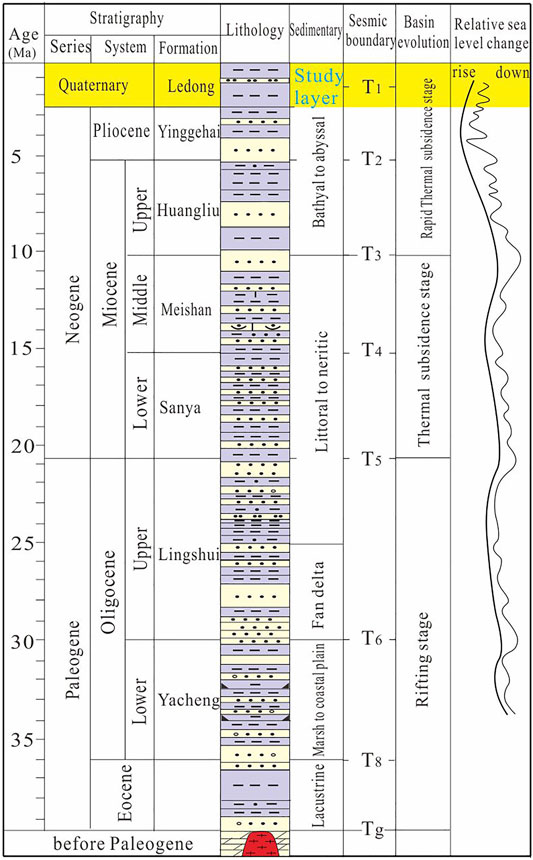
FIGURE 2. Tectono-stratigraphic column of the QDNB. Note: the yellow areas show the Quaternary strata.
Since the Middle Miocene, this basin as a whole entered the rapid subsidence stage, and the continental slope system began to form in the northern margin of the basin under the action of the depression. In the Late Miocene, the northern margin of the basin showed obvious shelf-slope break and entered the stage of rapid subsidence. The northwestern provenance was sufficient, and the continental slope moved forward rapidly due to the influence of high-speed sediments. The channel that developed along the shelf margin reflects the enhancement of sediment transport capacity to the sea during this period.
From the Pleistocene to the present, the QDNB has been in a period of highstand systems tract since the sea-level began to decline gradually. In addition to the large-scale delta depositional system that developed in the northwest shelf-slope break, the whole area is in a semi-bathyal sedimentary environment, which is mainly composed of fine-grained argillaceous rocks. The last stage of the prograding reflector pushed seaward more than the previous stages, indicating that the range of sea-level decline was small and frequent during the late Pleistocene to the late Holocene, and the range of sea-level decline was large at the end of the Holocene.
3 Data and Methods
2D/3D seismic, coring, well logging, and multi-beam data were comprehensively used to study the Quaternary channel systems in the QDNB. A total of 34,000 km2 of 2D seismic data and 1,900 km2 of 3D seismic data were acquired by the Guangzhou Marine Geological Survey from 2005 to 2021 (Figure 1). 3D seismic data in areas A, B, and C offer a range of visualization and attribute analysis that can provide specific information on the development of channel systems. 2D seismic data that cover the whole basin were used to track the distribution of the channel system in the QDNB. Two drilling expeditions in 2019 and 2021 acquired a large quantity of well logging and coring data, which provide a lot of geological information that is closely related to gas hydrates. One typical drilling well (W03) and its coring samples were used to study the vertical channel evolution.
A total of 57,300 km2 of multi-beam data that nearly cover the entire QDNB were used to identify the seabed channels and the variation in seabed morphology.
Well-seismic correlation was carried out to establish the corresponding relationship between the lithology of Well W03 and the plane characteristics of seismic attributes. Core calibration and seismic attribute analysis were used to identify channels and to study channel-related sedimentary facies. Multi-layer high-resolution seismic sedimentology research was used to establish the evolution history of channel systems.
4 Results
4.1 Evidence of Quaternary Channel Systems
Gas hydrate–bearing sands were found for the first time during gas hydrate drilling in area B of the QDNB. Three sets of sand layers were drilled, and the lithology of the sand layer is mainly silt. The sedimentary facies is characterized by the interactive deposition of channel-levee facies and MTDs. Three-stage sedimentary high-frequency cycles were identified, with muds at the bottom and sands as the top in each cycle (Figure 3), The cycles show the repetition of sedimentary facies association (MTD and channel-levee facies). MTD represents the beginning of an event deposition, while channel -levee facies represents the relative termination of the event deposition. The three-stage channels and MTDs were clearly identified in the seismic profile that crosses Well W03 and these have a good corresponding relationship with the three sets of sand layers and MTDs that were encountered in the drilling cores (Figure 4). Therefore, the comprehensive calibration of seismic drilling and the cores not only confirms the existence of the Quaternary channels, but also well identifies the channel stages near the seabed. At least in area B, the three channel stages exhibit southeast migration (Figure 4). Because the drilling did not extend through the Quaternary strata, the 2D/3D seismic data are the only data that can be used to identify channels deep beneath the seabed. Through the interpretation of a large quantity of seismic data in the QDNB, a total of six channel stages were identified, which are described in detail later.
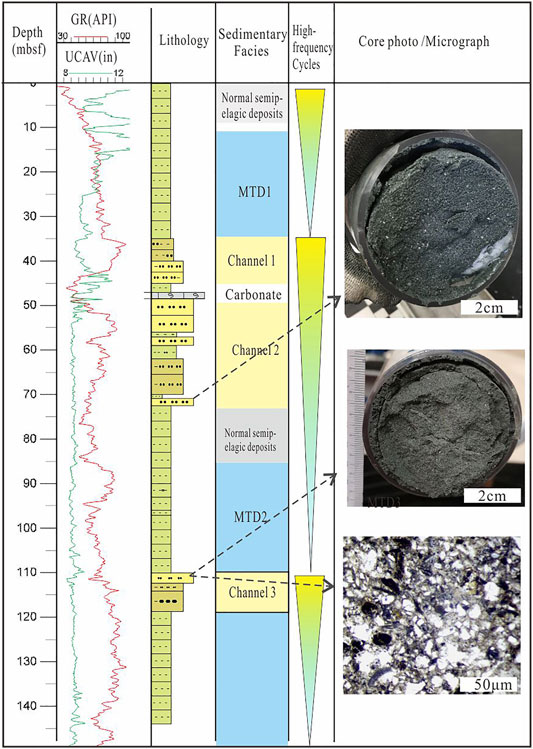
FIGURE 3. Comprehensive sedimentary column of Well W03 in area B. Note: The dashed lines with arrow show the depth location of core samples.
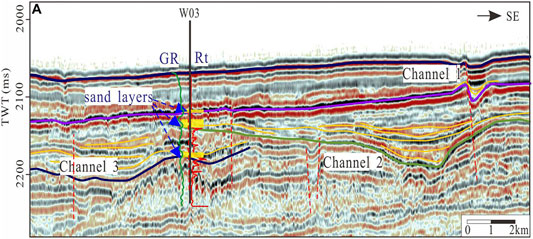
FIGURE 4. 2D seismic profile that crosses Well W03 in area B. Three channels are shown in seismic profiles and three sand layers are verified from Well W03. Note: The location of seismic profile in Figure 1B.
Several discontinuity seabed channels were observed clearly from the multi-beam data in the deep-sea area of the central QDNB. Combined with the change in topography, the main direction of channel flow is concluded to be from SE to NE. Theoretically, the channel should be continuous, however, most of the area is flat with deep-sea mud, and only a few parts of remnant channels can be seen from the multi-beam data (Figure 5). Therefore, the channels are in the extinction stage, which is the latest period of the channel system in the QDNB.
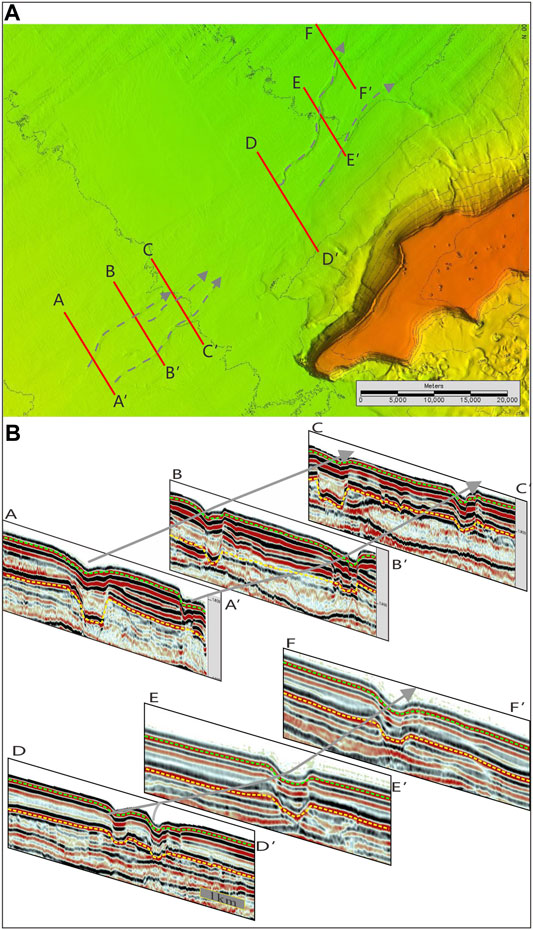
FIGURE 5. (A) The seabed channels identified from multi-beam data and seismic data in the QDNB. Note: The red lines show the location of seismic profile in (B), and the grey arrow lines shows the distribution of remnant seabed channels; (B) The seismic profiles present the channel-filling process. Note: The gray arrows show the direction of seabed channels, the yellow dashed lines show the sequence interface during the formation of the latest channel, the green dashed lines show the sequence interface of seabed.
4.2 Channel-Related Seismic Facies and Sedimentary Facies Identification
4.2.1 Channel-Related Seismic Facies
The most intuitive seismic facies in the Quaternary QDNB is the channel-filling seismic facies, which is flat at the top and has a bulge at the bottom, and its bottom boundary is generally U-shaped or V-shaped, and the adjacent underlying strata are usually truncated to varying degrees. A V-shaped bottom boundary represents high turbidity current scour and rapid deposition of the channel, while a U-shaped bottom boundary represents low turbidity current scour and slow deposition of the channel. The channel-filling seismic facies is mainly characterized by strong amplitude and low frequency, with parallel or subparallel internal structures, and with both sides or one side having in-phase axis overlap above the boundary of the underlying concave filling boundary.
Based on the internal stacking patterns within the channel, four filling seismic facies were identified. These facies types were used to assist in interpreting the extent of the channel facies and its lateral, upper, and lower boundaries.
1) Parallel filling facies: The internal reflection of the in-phase axis generally has a parallel or subparallel structure (Figure 6). This is a typical case of a local erosive channel, usually indicating submarine canyon or turbidite channel-filling.
2) Progradation filling facies: The internal reflection is parallel to the underlying denudation reflection, and there is obvious onlap to the upward-dip direction and truncation to the downward-dip direction (Figure 6). The internal reflection wave is inclined with weak accretion, which is similar to the underwater delta fan.
3) Onlap filling facies: The external shape is similar to that of the parallel filling facies, and the internal reflection is uniform, parallel to the gently divergent structure, with high continuity and variable amplitude at both ends, and slightly higher than that of the underlying strata (Figure 6). This represents the late development stage of the channel.
4) Multistage filling facies: The seismic profile is characterized by multiple filling facies in the same period and vertical superposition or migration in different periods (Figure 6), indicating the development of multiple channels and multi-stage channels.
4.2.2 Sedimentary Facies Identification
Based on the division of seismic facies, through the comprehensive interpretation of geological setting, sea-level changes, drilling data, and seismic attributes, the hydrodynamic condition, sedimentary environment, and its specific sedimentation were analyzed, and then the corresponding sedimentary facies were determined.
Channels with scales greater than the seismic resolution are easier to identify; for example, the Central Canyon developed in the Miocene of the QDNB. However, in addition to some regions or periods where the channel scale is relatively large and be identified from seismic profiles, many channels are small in scale that cannot be identified easily from seismic profiles, and their transverse distribution characteristics are more difficult to determine. Combined with the identification of sand layers from drilling, interpretation of seismic data, and 3D seismic horizon attribute analysis, the distribution characteristics of channels where the sand layer is located can be well explained. Through the tracing of channels by 2D/3D data, the sedimentary facies, distribution characteristics, and scale of Channel 3 system were identified in areas A, B, and C, respectively (Figure 7).
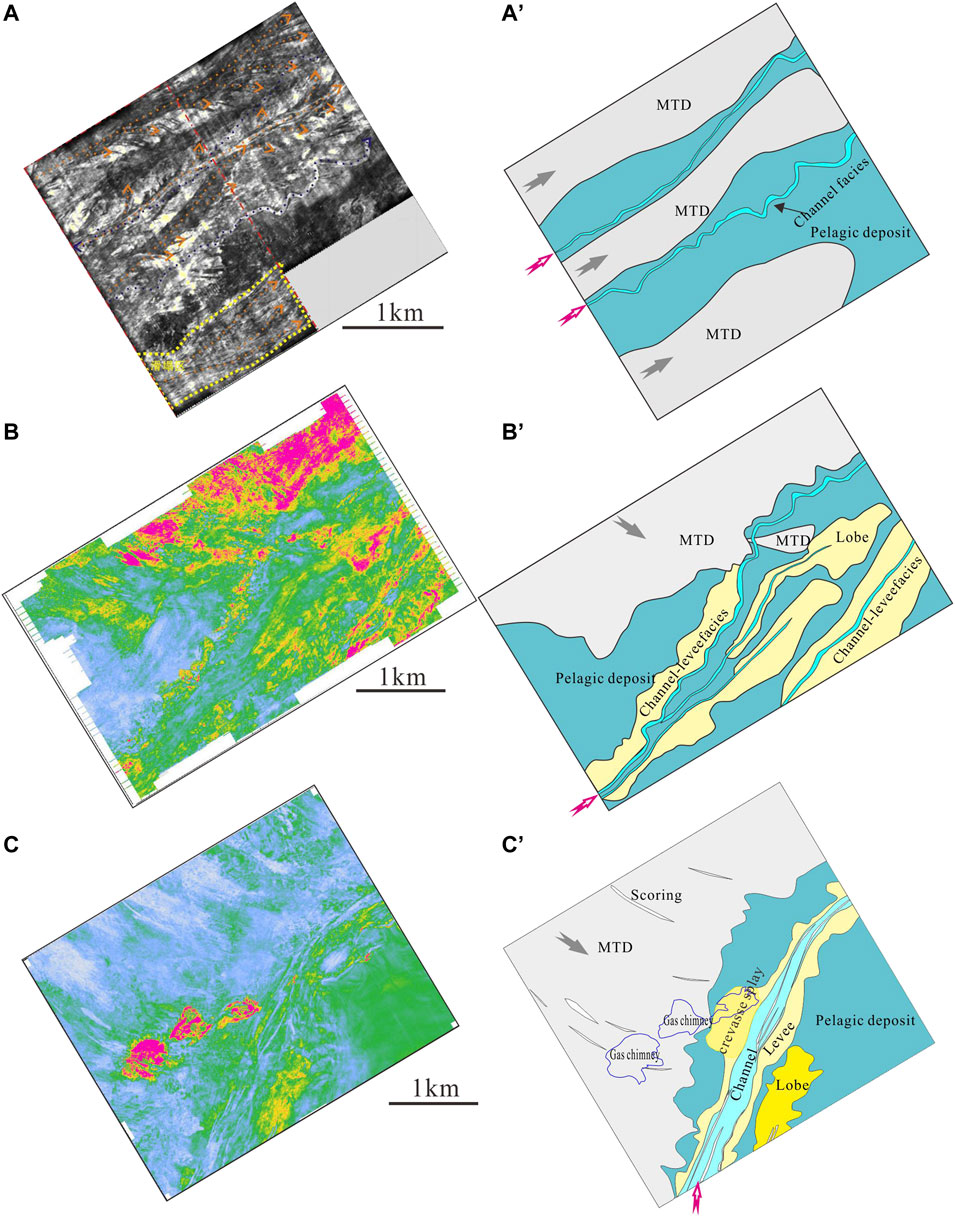
FIGURE 7. Attributes and sedimentary facies of the Channel 3 system in areas A, B, and C of the QDNB. (A) Minimum amplitude attribute map of the Channel 3 system in area A; (A′) Sedimentary facies map of Channel 3 system in area A; (B) RMS amplitude attribute map of the Channel 3 system in area B; (B′) Sedimentary facies map of Channel 3 system in area B; (C) RMS amplitude attribute map of the Channel 3 system in area C; (C′) Sedimentary facies map of Channel 3 system in area C. Note: The red arrows show the flow direction of channels, and the gray arrows shows the direction of MTDs.
A mostly channel-levee sedimentary system (Figure 7) developed in the Quaternary QDNB, which is composed of channel-filling facies, levee facies, crevasse splay facies, and channel terminal lobes facies. Channels usually maintain turbidity current deposition, which represents long-term and long-distance sediment transport. The levee is formed by gravity flow out of channel edge and extends laterally, due to the rapid decrease in gravity flow velocity, the levee near the channel is very thick, and thin far away from the channel. Crevasse splay and channel terminal lobes were found in Channel 3 system of area C (Figure 7).
4.3 The Distribution of Multi-Stage Channels
Through the analysis and comparison of the seismic phase axis contact relationship and seismic attribute characteristics of areas A, B, and C, it was determined that the Quaternary strata can be divided into seven sub-sequences (S1–S7) (Figure 8). The seismic interpretation and attribute analysis found that the distribution of channels is clearly shown in S1–S6 (Figure 9). The corresponding channel system for each sequence is named Channel 1 system, Channel 2 system, and so on. Considering that gas hydrate–bearing sands are mainly developed in the Channel 3 system, and multi-beam data can provide sufficient information for the study of near seabed channels (Channel 1 system), the Channel 3 system and Channel 1 system were used to study the distribution characteristics of channel systems in the Quaternary QDNB.
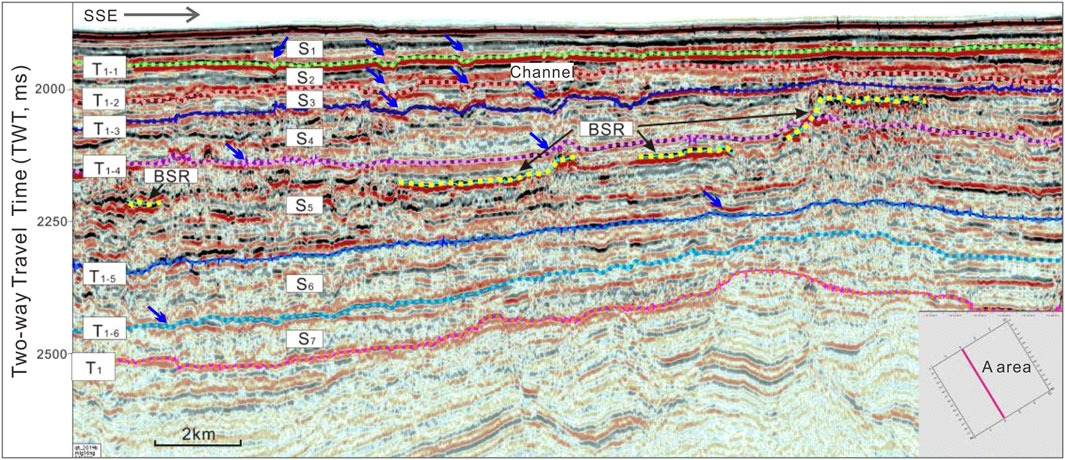
FIGURE 8. Sequence stratigraphy and 6 periods of channel systems of the Quaternary strata in area A. Note: The blue arrows show the locations of channels. The location of this seismic profile is in Figure 1B.
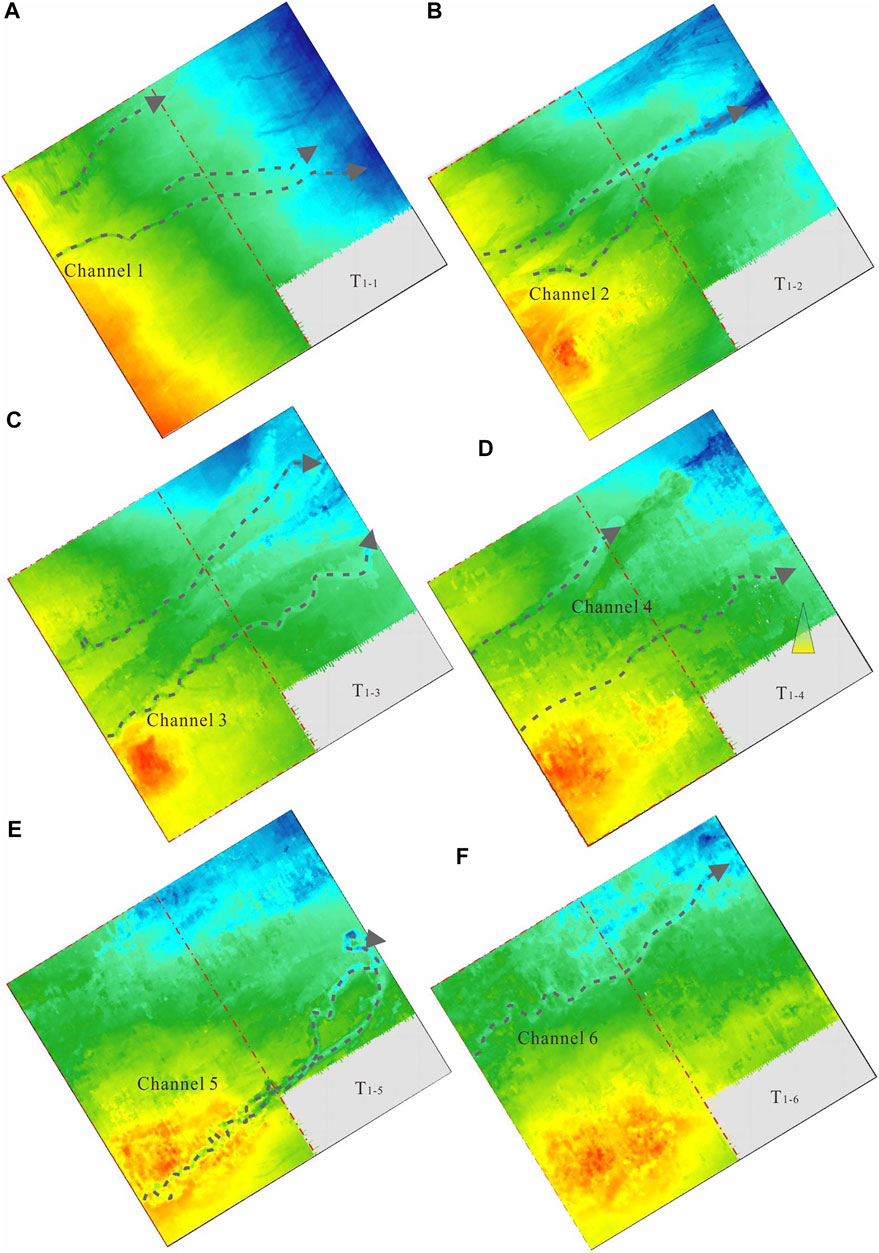
FIGURE 9. Sequence boundary structure maps of the Quaternary strata in area A (A) Structure map of T1-1 sequence boundary; (B) Structure map of T1-2 sequence boundary; (C) Structure map of T1-3 sequence boundary; (D) Structure map of T1-4 sequence boundary; (E) Structure map of T1-5 sequence boundary; (F) Structure map of T1-6 sequence boundary. Note: The grey dash lines show the channel systems, and the arrows indicate the channel flow directions.
Combined with 2D seismic data, the distribution of channels in the QDNB was tracked, and the distribution characteristics of the Channel 3 and Channel 1 systems in the whole QDNB were obtained. It can be seen that the channel-levee sedimentary system is widely developed (Figures 7, 8), especially in the middle of the central depression zone of the QDNB.
There are obvious distribution differences on the Channel 3 and Channel 1 systems between the western, middle, and eastern sections (Figure 10).
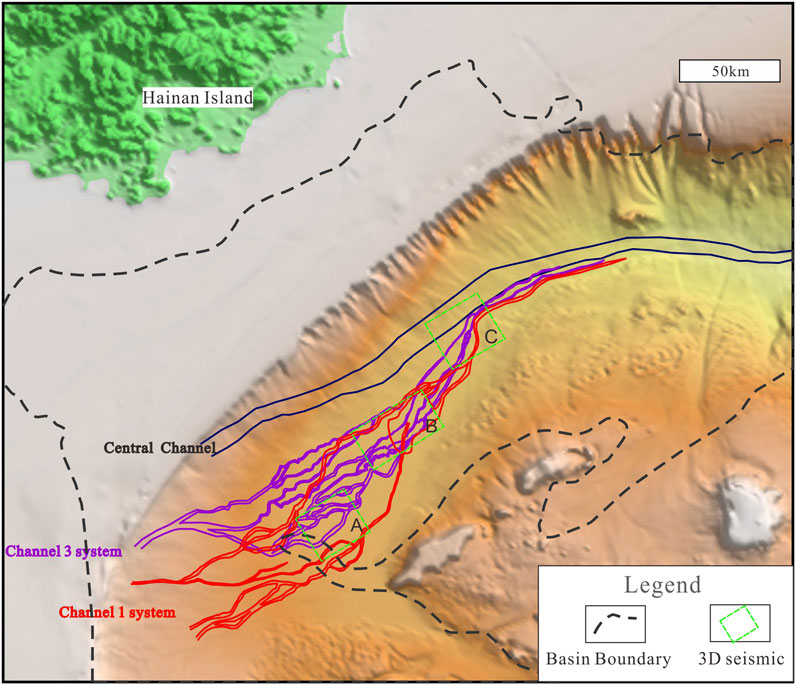
FIGURE 10. The distribution of the Channel 1 and 3 systems and the Central channel in the QDNB. Note: The purple solid line shows the distribution of Channel 3 system traced from quaternary hydrate-bearing sands in C area; the read solid line shows the distribution Channel 1 system near seabed, which is the latest period of the Quaternary channel systems; the blue solid line shows the distribution of the Central channel that developed at the Pliocene strata; the black dashed line shows the boundary of the QDNB.
4.3.1 Distribution Characteristics of the Channel 3 System
One nearly straight channel, showing a V-shape in the seismic profile, is developed in the western section of the central depression of the QDNB. The channel is located at the lower part of the continental slope. Therefore, the channel in this area mainly erodes the continental shelf. The superposition mode is mainly in the lateral order superposition, reflecting the obvious lateral accumulation due to the directional action of the bottom current. The downcutting effect of the channel is slightly weakened eastward, and the restraining effect of the channel is gradually weakened. Therefore, the channel formed several branches in the southeast direction (Figure 10).
The channel system in the central section has many branches and meanders to the east, showing the characteristics of a large braided channel system. Far extending levees were developed on both sides of the channel, and the north side was mainly developed in large areas. The channels in the middle section are located in the submarine plain, and the erosion of downcutting is weakened. The single channel identified from the seismic profile is mainly of a wide U-type, with obvious levees developed on its flanks. The channel-levee system is mainly superimposed by vertical disordered superposition. As the restriction of the channel is further reduced, channels in this area show vertical disordered superposition erosion or accretion.
Several branch channels in the eastern section converge into one. There are still deposits of levees, lobes, and crevasses in area C. Drilling confirms that the thickness of levees is 6–8 m. As the eastern part of the central depression zone of the QDNB is close to the northwest sub-basin, the seafloor terrain becomes steeper. It inherits the topographic characteristics of the Central Canyon development period; therefore, multiple channels converge into one channel. The channel here presents a V-shaped straight channel.
4.3.2 Distribution Characteristics of the Channel 1 System
Similar to the Channel 3 system, three near straight channels are also apparent in the western section of the Channel 1 system, and a large braided channel system in the middle section that converges into a straight channel again in the western section.
The Channel 1 system differs from the Channel 3 system in the following ways: 1) In the western section, Channel 1 system has three near straight channels and locates more to the south; 2) In the middle section, there are fewer branch channels, with two main channels in the north and south, and a large submarine fan in area B; 3) In the eastern section, the channel system is similar to Channel 3, but almost devoid of sandy deposits.
5 Discussion
5.1 What are the Controlling Factors on the Distribution of Quaternary Channel Systems?
The direct factors affecting the morphology, evolution, and sedimentary characteristics of deep-water channels include submarine topographic slope, sedimentary hydrodynamic conditions, and sediment grain size. Indirect factors include tectonic movement, sea-level change, and provenance supply. The possible controlling factors on channel distributions in the Quaternary QDNB are discussed below in detail.
5.1.1 Seafloor Morphology
Topography is a prerequisite for the formation of channels, which determines and influences the location and morphology of channel development (Armitage, 2009). A profile (Line 1) of multi-beam data was extracted where the latest period channels (Channel 1 system) of the QDNB is located (Figure 11A), and the variations in seabed elevation and slope were obtained. It was found that the seabed elevation gradually decreases from SW to NE, with an elevation difference of 1,411 m (Figure 11B). There are significant slope differences between the western, middle, and eastern sections. The slope of the western section varies greatly, ranging from 0.6° to 0.3°; the slope of the middle section is generally about 0.2°, which is relatively flat; while in the eastern section, the slope becomes steeper again (Figure 11C).
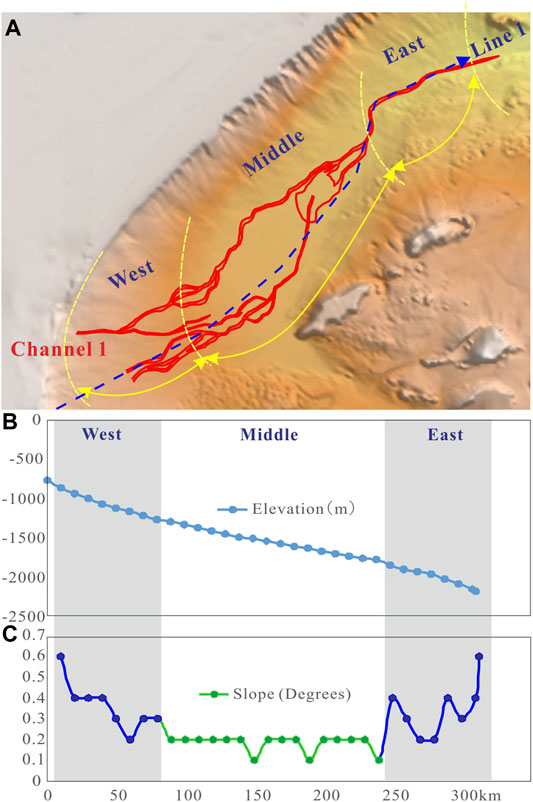
FIGURE 11. (A) The distribution characteristics of the Channel 1 system and seabed multi-beam data. Note: line 1 is the plan position of elevation and slope variation maps in (B,C). (B) The elevation variation of line 1 from SW to NE; (C) The slope variation of line 1 from SW to NW.
Combined with the above distribution characteristics of the Channel 1 system, it was found that there is a good correlation between slope variation and the development of the channel system.
In the western section, the slope is steep and the channels are near straight. With the descending of the slope, the channels form branches, but are still dominated by near straight form, and the erosion is mainly below the channel. The sediments are mostly passing deposits, and the levee-overflow deposits are not developed, and the channel is completely filled by argillaceous deposits in the later period (Figure 5).
In the middle section, the slope is relatively gentle, and with weakened downcutting and strengthened flooding of the deepwater channel, the curved channel develops, and a large lobe deposit is developed near the east.
In the eastern section, the slope becomes steeper again, and the two channels converge into one, and the channel morphology inherits the characteristics of the Central Canyon; with insufficient provenance supply, the channel is mainly covered by thin layers of pelagic sediment.
5.1.2 Tectonic Movement
The Central Canyon runs across the QDNB from east to west, which is about 570 km long and 9–39 km wide. The main body of the canyon began to develop in the Late Miocene (10 Ma) and ended in the Pliocene. It is not only an important pathway for transporting sediments from shallow water to deep water, but also a site for the deposition of detrital materials. The Central Canyon plays a pivotal role in the tectonic sedimentary evolution of the QDNB, and is closely related to hydrocarbon accumulation (Wang, 2012), so the degree of research on its architecture, sedimentation process, provenance sources, and controlling factors is relatively high (Gong et al., 2011; Su et al., 2014; Wang et al., 2016).
The distribution of the Central Canyon in the Miocene is also depicted in the distribution map of the Quaternary channel system in the QDNB (Figure 10). The Quaternary channels in the eastern section are almost superposed with the Central Canyon, which can be clearly seen in the seismic profile. However, in the western section, the development of the Quaternary channel systems show southward migration, and the migration amplitude increases westward, up to more than 100 km. This indicates the southward migration of the provenance system from the Central Canyon and Quaternary channel system. The question is why was there such a great migration distance since 10.5 Ma? The immediate factor is most likely the relative topographic decline to the south, and the southward migration of the subsidence center. The main factor that can change the regional subsidence center could be tectonic movement.
The large faults in the west of the QDNB mainly include the Red River fault and No. 2 fault. A great deal of research has been conducted on the tectonic activity of the Red River fault and its control on the tectonic and sedimentary evolution of the Yinggehai Basin and QDNB (Xie et al., 2006; Lei et al., 2021; Xie et al., 2021), with one of the big discoveries being the late Miocene strike-slip reversal (Sun et al., 2003; Wang et al., 2016; Zhu et al., 2009). The Red River fault in the western QDNB shifted from sinistral strike-slip to dextral movement at about 5.5 Ma (Figure 1). The dextral strike-slip movement may have continued into the Quaternary period, which resulted in the faster subsidence in the southwest of the QDNB compared with the northwest, and the subsidence center moved southward. At the same time, the slope-subparallel basement faults (No.4 fault) shifted to dextral slip (Graham, 2016). In addition, the No. 2 fault may have also been active, and all of these slight tectonic activity contributions have changed the morphology since the Miocene, as exemplified by the greater subsidence in the south compared to the north, and the greater subsidence in the east compared to the west. Finally, these contributed to the southward migration of the channel provenance in the QDNB from the Miocene to the present (Figure 8). The southward migration of channels represents the southward migration of the subsidence center, while the southward migration of the subsidence center is the joint geological response of the dextral rotation of the Red River strike-slip fault, No. 4 fault, and No. 2 fault (Figure 1). Due to the southward migration of subsidence center, as well as the rapid southward migration of passive continental margin (Gong et al., 2019; Chen et al., 2020), the northwest provenance continued to prograde from north to south, and result to the southward migration of low terrain and channel systems. Therefore, the southward migration of channel systems can be considered as the result of the combined action of tectonic and sedimentation.
5.1.3 Sea-level Fluctuations
Frequent sea-level changes can affect provenance supply and lead to the development of multi-stage channels. The six stages of Quaternary channels in the QDNB are often inter-deposited with MTDs (Figure 3), which may have a good relationship with sea-level changes. The periodic sea-level change in the QDNB is controlled by the combined effect of global sea-level change and regional crustal subsidence (especially thermal subsidence), therefore, the sea-level change in the QDNB is different from the global sea-level rise and fall cycle (Haq et al., 1987; Hao et al., 2000). At present, there is a lack of information regarding the continuous period and amplitude of Quaternary sea-level change in the QDNB (Bintanja et al., 2005; Yu and Chen, 2009), but the basic consensus is that there are multiple periods of sea-level change and the overall sea-level is declining (Chen et al., 2020). However, the multi-stage channel development can also provide some enlightenment for the study of Quaternary sea-level change in the QDNB.
5.1.4 Provenance Supply
The flow direction of channels in the Quaternary QDNB is from west to east, which is basically consistent with that of the Central Canyon. Therefore, the provenance of Quaternary channels can be compared with that of the Central Canyon. Most scholars believe that the provenance of the Central Canyon mainly came from the Red River system (Lin et al., 2001; Su et al., 2019; Lyu et al., 2021) and the Red River submarine fan in the Yinggehai Basin, which is considered to be the direct source supplying the Central Canyon (Wang et al., 2011; Xie, 2020; Xu et al., 2020). However, some researchers believe that it originated from central and northern Vietnam (Zhang et al., 2017; Su et al., 2019) and the provenance of Hainan Island (Lin et al., 2001; Cao et al., 2013).
It should be noted that the western part of the Quaternary channel system migrated about 100 km southward compared with the Central Canyon (Figure 8), so the provenance of the Quaternary may be less contributed by the Red River system and more influenced by the Vietnamese river system. In addition, although the channel length of the Quaternary is similar to that of the Central Canyon, the channel width is significantly narrower than that of the Central Canyon (Figure 8), which also indicates a significant decrease in sediment transport, and could indirectly reflect a significant decrease in sediment supply in the provenance area. The decrease of provenance supply is the result of the interaction of Himalayan movement, sea-level and climate changes. As for how these factors affect the provenance supply, it is a complex and synergistic mechanism that has not been solved yet, but it reflects the disappearance of the channel systems to some extent. That, in turn, could shed light on tectonic movement, sea-level and climate changes.
The development of the Quaternary channel system in the QDNB is the result of multi-factor synthesis: the tectonic movement controls seafloor morphology, which directly controls the flow of channels and their distribution characteristics, and the provenance supply determines the scale of each channel and the sedimentary characteristics. The periodic changes in sea-level determine the evolution of the multi-stage channel systems.
5.2 The Prediction of Gas Hydrate-Bearing Sand Reservoirs
Deepwater channel system is an important transport pathway for sand sediments. Sand bodies can be deposited by channel-filling deposits, levee deposits, crevasse splay and lobes, which are often regarded as favorable oil and gas reservoirs (Morris, and Busby-Spera, 1990; Babonneau et al., 2002; Wynn et al., 2002; Abreu et al., 2003; Beaubouef, 2004).
Under the limit of low temperature and high pressure, the gas hydrate can only occur in shallow sediments under the seabed. Taken the QDNB as a case, the lower limit of gas hydrate occurrence depth confirmed by drilling is about 200 mbsf. Therefore, it is necessary to study the characteristics of shallow channel systems. Based on our study, the Quaternary channels in the QDNB are branching from southwest and gradually converging toward east. In the western section, few coarse grain sands may exist in channel-filling facies; in the middle section, the sea floor morphology is flat and the braided channel becomes dense. It is speculated that there are both channel-filling, levees and crevasse splay deposits, with the possibility of multi-stage superposition of sand bodies. Therefore, the favorable shallow sand bodies (mainly belonging to channel-filling deposits, levees, crevasse splay deposits and lobes deposits) in the QDNB may be developed in the middle section where channel developed. This study can provide sedimentological basis for the prediction of gas hydrate-bearing sand reservoirs in the QDNB, and more 3D seismic, drilling and logging data are necessary to better predict sand reservoirs in the future. In addition, the identification and prediction of high saturated gas hydrates in sand reservoirs also needs comprehensive analysis of gas hydrate stability zone, sufficient gas source and favorable structure pathway.
6 Conclusion
Using the integrated 2D/3D seismic, multi-beam, well logging, and coring data, the current study documents the distribution characteristics of the channel system and its controlling factors in the Quaternary strata of the QDNB. The integrated analysis shows the following observation that:
1) The channel-related sedimentary facies include the channel-filling facies, levee facies, crevasse splay facies, and lobes facies; six periods of channel systems are identified in the Quaternary strata.
2) There are obvious distribution differences in the Channel 1 and Channel 3 systems between the western, middle, and eastern sections: the channels in the western and eastern sections are mainly dominated by near straight V-shaped channels, while the middle section mainly consists of large braided channels, where a channel-levee sedimentary system developed.
3) Compared with the distribution of the Central Canyon, the channels in the western section show southward migration since the Miocene.
4) The distribution and evolution of the Quaternary channels were likely collectively controlled by the seafloor morphology, tectonic movement, sea-level fluctuations, and provenance supply. Tectonic movement controls seafloor morphology, which directly controls the flow of channels and their distribution characteristics, and the provenance supply determines the scale of each channel and their sedimentary characteristics. The periodic changes in sea-level determine the evolution of multi-stage channel systems.
5) It is predicted that the favorable shallow sand bodies (mainly belonging to channel-filling deposits, levees, crevasse splay deposits and lobes deposits) in the QDNB may be developed in the middle section where channel developed.
Data Availability Statement
The original contributions presented in the study are included in the article/Supplementary Material, further inquiries can be directed to the corresponding author.
Author Contributions
MM: Conceptualization, Writing—Original Draft, JL: Supervision, ZK: Review, JR: Data collection, YH: Redrafted and Figure drawing, WD: Software, YG: Suggestion. All authors contributed to manuscript revision, read and approved the submitted version.
Funding
This work was supported by the National Natural Science Foundation of China (grant numbers 42102144; 42176215), Key Special Project for Introduced Talents Team of Southern Marine Science and Engineering Guangdong Laboratory (Guangzhou) (grant number GML2019ZD0102), and the China Geological Survey Project (grant number DD20221705).
Conflict of Interest
The authors declare that the research was conducted in the absence of any commercial or financial relationships that could be construed as a potential conflict of interest.
Publisher’s Note
All claims expressed in this article are solely those of the authors and do not necessarily represent those of their affiliated organizations, or those of the publisher, the editors and the reviewers. Any product that may be evaluated in this article, or claim that may be made by its manufacturer, is not guaranteed or endorsed by the publisher.
Acknowledgments
The authors wish to thanks to those who contributed to the success of the China National Gas Hydrate Program Expeditions 6 (GMGS6) and all personnel involved in seismic data acquisition and processing. Editor Jinan Guan and two reviewers for their insightful and helpful reviews are greatly appreciated. We thank LetPub for its linguistic assistance during the preparation of this manuscript.
References
Abreu, V., Sullivan, M., Pirmez, C., and Mohrig, D. (2003). Lateral Accretion Packages (LAPs): An Important Reservoir Element in Deep Water Sinuous Channels. Mar. Petroleum Geol. 20, 631–648. doi:10.1016/j.marpetgeo.2003.08.003
Armitage, D. A. (2009). High-Resolution Architectural Evolution of Depositional Elements in Deep-Marine Slope Environments: The Quaternary Niger Delta slope, Quaternary southwest Grand Banks slope, Canada, and Cretaceous Tres Pasos Formation. Doctoral dissertation, Chile: Stanford University
Babonneau, N., Savoye, B., Cremer, M., and Klein, B. (2002). Morphology and Architecture of the Present Canyon and Channel System of the Zaire Deep-Sea Fan. Mar. Petroleum Geol. 19, 445–467. doi:10.1016/s0264-8172(02)00009-0
Beaubouef, R. T. (2004). Deep-water Leveed-Channel Complexes of the Cerro Toro Formation, Upper Cretaceous, Southern Chile. Bulletin 88, 1471–1500. doi:10.1306/06210403130
Behseresht, J., and Bryant, S. L. (2012). Sedimentological Control on Saturation Distribution in Arctic Gas-Hydrate-Bearing Sands. Earth Planet. Sci. Lett. 341-344, 114–127. doi:10.1016/j.epsl.2012.06.019
Bintanja, R., van de Wal, R. S. W., and Oerlemans, J. (2005). Modelled Atmospheric Temperatures and Global Sea Levels over the Past Million Years. Nature 437 (7055), 125–128. doi:10.1038/nature03975
Boswell, R., Collett, T. S., Frye, M., Shedd, W., McConnell, D. R., and Shelander, D. (2012). Subsurface Gas Hydrates in the Northern Gulf of Mexico. Mar. Petroleum Geol. 34, 4–30. doi:10.1016/j.marpetgeo.2011.10.003
Boswell, R., Shelander, D., Lee, M., Latham, T., Collett, T., Guerin, G., et al. (2009). Occurrence of Gas Hydrate in Oligocene Frio Sand: Alaminos Canyon Block 818: Northern Gulf of Mexico. Mar. Petroleum Geol. 26, 1499–1512. doi:10.1016/j.marpetgeo.2009.03.005
Cao, L., Jiang, T., Wang, Z., Zhang, D., and Sun, H. (2013). Characteristics of Heavy Minerals and Their Implications for Neogene Provenances Evolution in Qiongdongnan Basin. J. Central South Univ. Sci. Technol. 44 (5), 230–240. (In Chinese with English abstract).
Chen, S., Steel, R., Wang, H., Zhao, R., and Olariu, C. (2020). Clinoform Growth and Sediment Flux into Late Cenozoic Qiongdongnan Shelf Margin, South china Sea. Basin Res. 32 (1), 302–319. doi:10.1111/bre.12400
Cheng, C., Jiang, T., Kuang, Z., Ren, J., Liang, J., Lai, H., et al. (2021). Seismic Characteristics and Distributions of Quaternary Mass Transport Deposits in the Qiongdongnan Basin, Northern South China Sea. Mar. Petroleum Geol. 129, 105–118. doi:10.1016/j.marpetgeo.2021.105118
Chong, Z. R., Yang, S. H. B., Babu, P., Linga, P., and Li, X.-S. (2016). Review of Natural Gas Hydrates as an Energy Resource: Prospects and Challenges. Appl. Energy 162, 1633–1652. doi:10.1016/j.apenergy.2014.12.061
Covault, J. A., Romans, B. W., Fildani, A., McGann, M., and Graham, S. A. (2010). Rapid Climatic Signal Propagation from Source to Sink in a Southern California Sediment‐Routing System. J. Geol. 118, 247–259. doi:10.1086/651539
Egawa, K., Nishimura, O., Izumi, S., Fukami, E., Jin, Y., Kida, M., et al. (2015). Bulk Sediment Mineralogy of Gas Hydrate Reservoir at the East Nankai Offshore Production Test Site. Mar. Petroleum Geol. 66, 379–387. doi:10.1016/j.marpetgeo.2015.02.039
Fildani, A., and Normark, W. R. (2004). Late Quaternary Evolution of Channel and Lobe of Monterey Fan[J]. Mar. Geol. 206 (1). doi:10.1016/j.margeo.2004.03.001
Gong, C., Blum, M. D., Wang, Y., Lin, C., and Xu, Q. (2018). Can Climatic Signals Be Discerned in a Deep-Water Sink?: An Answer from the Pearl River Source-To-Sink Sediment-Routing System. GSA Bull. 130, 661–677. doi:10.1130/b31578.1
Gong, C., Qi, K., Ma, Y., Li, D., and Xu, H. (2019). Tight Coupling Between the Cyclicity of Deep-Water Systems and Rising-Then-Flat Shelf-Edge Pairs Along the Submarine Segment of the Qiongdongnan Sediment-Routing System. J. Sediment. Res. 89 (10), 956–975. doi:10.2110/jsr.2019.47
Gong, C., Steel, R. J., Wang, Y., Lin, C., and Olariu, C. (2016). Shelf-margin Architecture Variability and its Role in Sediment-Budget Partitioning into Deep-Water Areas. Earth-Science Rev. 154, 72–101. doi:10.1016/j.earscirev.2015.12.003
Gong, C., Wang, Y., Zhu, W., Li, W., Xu, Q., and Zhang, J. (2011). The Central Submarine Canyon in the Qiongdongnan Basin, Northwestern South China Sea: Architecture, Sequence Stratigraphy, and Depositional Processes. Mar. Petroleum Geol. 28 (9), 1690–1702. doi:10.1016/j.marpetgeo.2011.06.005
Graham, S. (2016). 3-D Seismic Characterization of Submarine Landslides on a Pliocene-Pleistocene Siliciclastic Continental Slope. South China Sea.
Hao, Y., Chen, P., and Wan, X. (2000). Late Tertiary Sequence Stratigraphy and Sea Level Changes in Yinggehai-Qiongdongnan Basin. Geoscience 14 (3), 237–245.
Haq, B. U., Hardenbol, J., and Vail, P. R. (1987). Chronology of Fluctuating Sea Levels since the Triassic. Science 235, 1156–1167. doi:10.1126/science.235.4793.1156
Hu, B., Li, J., Cui, R., Wei, H., Zhao, J., Li, G., et al. (2014). Clay Mineralogy of the Riverine Sediments of Hainan Island, South China Sea: Implications for Weathering and Provenance. J. Asian Earth Sci. 96, 84–92. doi:10.1016/j.jseaes.2014.08.036
Hu, B., Wang, L., Yan, W., Liu, S., Cai, D., Zhang, G., et al. (2013). The Tectonic Evolution of the Qiongdongnan Basin in the Northern Margin of the South China Sea. J. Asian Earth Sci. 77, 163–182. doi:10.1016/j.jseaes.2013.08.022
Huang, D., Du, J., Deng, B., and Zhang, J. (2013). Distribution Patterns of Particle-Reactive Radionuclides in Sediments off Eastern Hainan Island, China: Implications for Source and Transport Pathways. Cont. Shelf Res. 57, 10–17. doi:10.1016/j.csr.2012.04.019
Kenyon, N. H., and Millington, J. (1995). “Contrasting Deep-Sea Depositional Systems in the Bering Sea,” Atlas of Deep Water Environments: Architectural Style in Turbidite Systems. K. T. Pickering, R. N. Hiscott, N. H. Kenyon, F. R. Lucchi, and R. Smith (London: Chapman & Hall), 196–202. doi:10.1007/978-94-011-1234-5_28
Lei, C., Ren, J., Pei, J., Liu, B., Zuo, X., Liu, J., et al. (2021). Tectonics of the Offshore Red River Fault Recorded in the Junction of the Yinggehai and Qiongdongnan Basins. Sci. China Earth Sci. 64 (11), 1893–1908. (In Chinese with English abstract. doi:10.1007/s11430-020-9796-2
Liang, J., Wang, H., and Su, P. (2018). Study on Controlling Factors of Natural Gas Hydrate Accumulation. Beijing: Geological publishing house.
Lin, C., Liu, J., Cai, S., Zhang, Y., Lv, M., and Li, J. (2001). Sedimentary Composition and Development Background of Large-Scale Incised Valley and Submarine Gravity Flow System in Yinggehai-Qiongdongnan Basin. Sci. China 46 (1), 69–72. (in Chinese with English abstract). doi:10.3321/j.issn:0023-074X.2001.01.018
Lin, C., Xia, Q., Shi, H., and Zhou, X. (2015). Geomorphological Evolution, Source to Sink System and Basin Analysis. Earth Sci. Front. 22 (1), 9–20. (In Chinese with English abstract). doi:10.13745/j.esf.2015.01.002
Liu, Z., Colin, C., Li, X., Zhao, Y., Tuo, S., Chen, Z., et al. (2010). Clay Mineral Distribution in Surface Sediments of the Northeastern South China Sea and Surrounding Fluvial Drainage Basins: Source and Transport. Mar. Geol. 277, 48–60. doi:10.1016/j.margeo.2010.08.010
Matenco, L., Andriessen, P., and the Network, S. S. (2013). Quantifying the Mass Transfer from Mountain Ranges to Deposition in Sedimentary Basins: Source to Sink Studies in the Danube Basin-Black Sea System. Glob. Planet. Change 103, 1–18. doi:10.1016/j.gloplacha.2013.01.003
Meng, M., Liang, J., Lu, J. a., Zhang, W., Kuang, Z., Fang, Y., et al. (2021). Quaternary Deep-Water Sedimentary Characteristics and Their Relationship with the Gas Hydrate Accumulations in the Qiongdongnan Basin, Northwest South China Sea. Deep Sea Res. Part I Oceanogr. Res. Pap. 177, 103628. doi:10.1016/j.dsr.2021.103628
Mitchum, R. M., and Wach, G. D. (2002). Niger Delta Pleistocene Leveed-Channel Fans: Models for Offshore Reservoirs [M].
Moridis, G. J., Collett, T. S., Boswell, R., Hancock, S., Rutqvist, J., Santamarina, C., et al. (2013). Gas Hydrates as a Potential Energy Source: State of Knowledge and Challenges. Advanced Biofuels and Bioproducts. 1. 977–1033. doi:10.1007/978-1-4614-3348-4_37
Morris, W., and Busby-Spera, C. (1990). A Submarine-Fan Valley-Levee Complex in the Upper Cretaceous Rosario Formation: Implication for Turbidite Facies Models. GSA Bull. 102, 900–914. doi:10.1130/0016-7606(1990)102<0900:asfvlc>2.3.co;2
Romans, B. W., Castelltort, S., Covault, J. A., Fildani, A., and Walsh, J. P. (2016). Environmental Signal Propagation in Sedimentary Systems across Timescales. Earth-Science Rev. 153, 7–29. doi:10.1016/j.earscirev.2015.07.012
Saller, A. H., Noah, J. T., Ruzuar, A. P., and Schneider, R. (2004). Linked Lowstand Delta to Basin-Floor Fan Deposition, Offshore Indonesia: An Analog for Deep-Water Reservoir Systems. Bulletin 88, 21–46. doi:10.1306/09030303003
Santra, M., Flemings, P. B., Scott, E., and Meazell, P. K. (2020). Evolution of Gas Hydrate-Bearing Deep-Water Channel-Levee System in Abyssal Gulf of Mexico: Levee Growth and Deformation. Bulletin 104 (9), 1921–1944. doi:10.1306/04251918177
Scholz, N. A., Riedel, M., Bahk, J.-J., Yoo, D.-G., and Ryu, B.-J. (2012). Mass Transport Deposits and Gas Hydrate Occurrences in the Ulleung Basin, East Sea - Part 1: Mapping Sedimentation Patterns Using Seismic Coherency. Mar. Petroleum Geol. 35 (1), 91–104. doi:10.1016/j.marpetgeo.2012.03.004
Sha, Z., Guo, Y., Yang, M., Liang, J., and Wang, L. (2009). Relation between Sedimentation and Gas Hydrate Reservoirs in the Northern Slope of South china Sea. Mar. Geol. Quat. Geol. 29 (5), 89–98.
Sloan, E. D. (2003). Fundamental Principles and Applications of Natural Gas Hydrates. Nature 426, 353–359. doi:10.1038/nature02135
Su, M., Wu, C., Chen, H., Li, D., Jiang, T., Xie, X., et al. (2019). Late Miocene Provenance Evolution at the Head of Central Canyon in the Qiongdongnan Basin, Northern South China Sea. Mar. Petroleum Geol. 110, 787–796. doi:10.1016/j.marpetgeo.2019.07.053
Su, M., Xie, X., Xie, Y., Wang, Z., Zhang, C., Jiang, T., et al. (2014). The Segmentations and the Significances of the Central Canyon System in the Qiongdongnan Basin, Northern South china Sea. J. Asian Earth Sci., 79(pt.A), 552–563.doi:10.1016/j.jseaes.2012.12.038
Sun, Z., Zhong, Z., Zhou, D., Qiu, X., and Wu, S. (2003). Deformation Mechanism of Red River Fault Zone during Cenozoic and Experimental Evidences Related to Yinggehai Basin Formation. J. Trop. Oceanogr. 22, 1–9. (In Chinese with English abstract)
Uchida, T., and Tsuji, T. (2004). Petrophysical Properties of Natural Gas Hydrates-Bearing Sands and Their Sedimentology in the Nankai Trough. Resour. Geol. 54 (1), 79–87. doi:10.1111/j.1751-3928.2004.tb00189.x
Waite, W. F., Jang, J., Collett, T. S., and Kumar, P., (2019). Downhole Physical Property-Based Description of a Gas Hydrate Petroleum System in NGHP-02 Area C: A Channel, Levee, Fan Complex in the Krishna-Godavari Basin Offshore Eastern India. Mar. Petroleum Geol. 108, 272–295. doi:10.1016/j.marpetgeo.2018.05.021
Walsh, J. P., Wiberg, P. L., Aalto, R., Nittrouer, C. A., and Kuehl, S. A. (2016). Source-to-sink Research: Economy of the Earth's Surface and its Strata. Earth-Science Rev. 153, 1–6. doi:10.1016/j.earscirev.2015.11.010
Wang, D., Wang, Y., Yao, G., Wu, S., and Qin, Z. (2015). Deep-water Sediment Cycles in the Qiongdongnan Basin. Chin. Sci. Bull. 60 (10), 933–943. (In Chinese with English Abstract. doi:10.1360/n972014-00477
Wang, S., Zhang, N., Chen, H., Li, L., and Yan, W. (2014). The Surface Sediment Types and Their Rare Earth Element Characteristics from the Continental Shelf of the Northern South China Sea. Cont. Shelf Res. 88, 185–202. doi:10.1016/j.csr.2014.08.005
Wang, Y., Xu, Q., Li, D., Han, J., Lv, M., Wang, Y., et al. (2011). Late Miocene Red River Submarine Fan, Northwestern South China Sea. Chin. Sci. Bull. 56, 781–787. (In Chinese with English abstract). doi:10.1007/s11434-011-4441-z
Wang, Y., Zhang, D., Zhao, P., Yang, C., Huang, C., and Su, C. (2016). A New Consideration on the Genetic Mechanism of the Central Canyon in the Qiongdonnan Basin, the Northern South China Sea. Haiyang Xuebao 38 (11), 97–104. (In Chinese with English abstract). doi:10.3969/j.issn.0253-4193.2016.11.009
Wang, Z. (2012). Important Deepwater Hydrocarbon Reservoirs: The Central Canyon System in the Qiongdongnan Basin. Acta Sedimentol. Sin. 30 (4), 646–653. (In Chinese with English abstract). doi:10.14027/j.cnki.cjxb.2012.04.002
Weimer, P., Slatt, R. M., Bouroullec, R., Fillon, R., and Tari, G., (2006). Introduction to the Petroleum Geology of Deepwater Setting[M]. American Association of Petroleum Geologists.
Wynn, R., Kenyon, N. H., and Masson, D. G., (2002). Characterization and Recognition of Deep-Water Channel-Lobe Transition Zones. AAPG Bull. 86, 1441–1462. doi:10.1306/61eedcc4-173e-11d7-8645000102c1865d
Xie, H., Zhou, D., Shi, H., Li, Y., and Kong, D., (2021). Comparative Study on the Cenozoic Tectonic and Sedimentary Evolution in the Deep-Water Areas of the Zhujiang River Estuary Basin and the Qiongdongnan Basin. Haiyang Xuebao 43 (3), 48–61. (In Chinese with English abstract). doi:10.12284/hyxb2021055
Xie, X., Müller, R. D., Li, S., Gong, Z., and Steinberger, B. (2006). Origin of Anomalous Subsidence along the Northern South China Sea Margin and its Relationship to Dynamic Topography. Mar. Petroleum Geol. 23 (7), 745–765. doi:10.1016/j.marpetgeo.2006.03.004
Xie, Y. (2020). Sedimentary Characteristics and Hydrocarbon Exploration Potential of the Upstream of the Central Canyon in the Yinggehai and Qiongdongnan Basin. Bull. Geol. Sci. Technol. 39 (5), 69–78. (In Chinese with English abstract). doi:10.19509/j.cnli.dzkq.2020.0601
Xu, F., Chen, S., Cao, Y., Chen, M., Li, A., and Xiao, S. (2010). Geochemical Records and Geological Significance of the Continental Shelf Sediments in the Northern South China Sea since 4400a. Acta Sedimentol. Sin. 28 (6), 1198–1205. doi:10.14027/j.cnki.cjxb.2010.06.007
Xu, Q., Li, D., Zhu, W., and Wang, Y. (2020). SHRIMP U-Pb Ages of Detrital Zircons: Discussions on Provenance Control and the Red River Capture Event. Sediment. Geol. Tethyan Geol. 40 (3), 20–30. (In Chinese with English abstract). doi:10.19826/j.cnki.1009-3850.2020.07009
Yan, H., Tian, X., Xu, F., Hu, B., and Liu, Z. (2016). Sediment Provenance of Offshore Mud Area of the Eastern Hainan Island in South China Seas in the Mid-holocene. Haiyang Xuebao 38 (7), 97–106. (In Chinese with English abstract). doi:10.3969/j.jssn.0253-4193.2016.07.009
Yu, K., and Chen, T. (2009). Beach Sediments from Northern South China Sea Suggest High and Oscillating Sea Levels during the Late Holocene. Earth Sci. Front. 16 (6), 138–145. doi:10.1016/s1872-5791(08)60110-4
Yu, X., and Zhang, Z. (2005). Characteristics of Neogene Depositional Systems on the Northern continentalSlope of the South China Sea and Their Relationships with Gas Hydrate. Geol. China 32 (3), 470–476. (In Chinese with English abstract).
Yuan, S., Cao, F., and Wu, S. (2010a). Architecture and Origin of Deepwater Sinuous Channel on the Slope of Nortern South China Sea. Acta Sedimentol. Sin. 28 (1), 68–75.
Yuan, S., Wu, S., Thomas, L., Yao, G., Lv, F., Cao, F., et al. (2009). Fine-grained Pleistocene Deepwater Turbidite Channel System on the Slope of Qiongdongnan Basin, Northern South china Sea. Mar. Petroleum Geol. 26 (8), 1441–1451. doi:10.1016/j.marpetgeo.2009.03.007
Yuan, S., Wu, S., and Yao, G. (2010b). The Controlling Factors Analysis of Qiongdongnan Slope Deepwater Channels and its Significance to the Hydrocarbon Exploration. Mar. Geol. Quat. Geol. 30 (2), 61–66. doi:10.3724/sp.j.1140.2010.02061
Zhang, D., Zhang, Y., Shao, L., Liu, X., Wang, Y., He, X., et al. (2017). Sedimentary Provenance in the Central Canyon of Qiongdongnan Basin in the Northern South China Sea. Nat. Gas. Geosci. 28 (10), 1574–1581. (In Chinese with English abstract). doi:10.11764/j.issn.1672-1926.2017.08.012
Zhao, Z., Sun, Z., Wang, Z., Sun, Z., and Zhang, C. (2015). The High Resolution Sedimentary Filling in Qiongdongnan Basin, Northern South China Sea. Mar. Geol. 361, 11–24. doi:10.1016/j.margeo.2015.01.002
Keywords: channel system, Quaternary, sedimentary characteristic, Qiongdongnan Basin, gas hydrates
Citation: Meng M, Liang J, Kuang Z, Ren J, He Y, Deng W and Gong Y (2022) Distribution Characteristics of Quaternary Channel Systems and Their Controlling Factors in the Qiongdongnan Basin, South China Sea. Front. Earth Sci. 10:902517. doi: 10.3389/feart.2022.902517
Received: 23 March 2022; Accepted: 05 May 2022;
Published: 02 June 2022.
Edited by:
Jinan Guan, Guangzhou Institute of Energy Conversion (CAS), ChinaReviewed by:
Junjie Liu, Guangzhou Institute of Geochemistry (CAS), ChinaXiaoqun Yang, Institute of Geology and Geophysics (CAS), China
Copyright © 2022 Meng, Liang, Kuang, Ren, He, Deng and Gong. This is an open-access article distributed under the terms of the Creative Commons Attribution License (CC BY). The use, distribution or reproduction in other forums is permitted, provided the original author(s) and the copyright owner(s) are credited and that the original publication in this journal is cited, in accordance with accepted academic practice. No use, distribution or reproduction is permitted which does not comply with these terms.
*Correspondence: Miaomiao Meng, MTg4MTEzMDk5ODFAMTI2LmNvbQ==; Yulin He, aGV5dWxpbjIwMDkxMEAxNjMuY29t