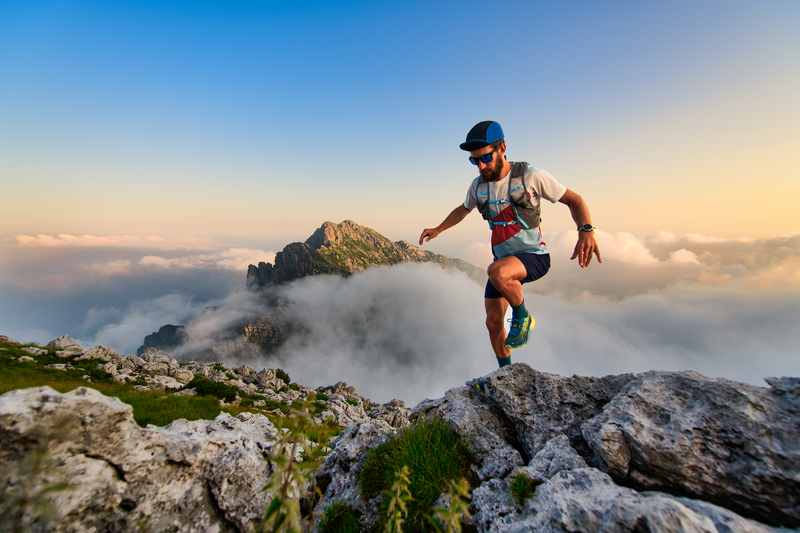
95% of researchers rate our articles as excellent or good
Learn more about the work of our research integrity team to safeguard the quality of each article we publish.
Find out more
ORIGINAL RESEARCH article
Front. Earth Sci. , 16 June 2022
Sec. Quaternary Science, Geomorphology and Paleoenvironment
Volume 10 - 2022 | https://doi.org/10.3389/feart.2022.895419
This article is part of the Research Topic Modern Geomorphic Processes: From Source to Sink and From Survey to Models View all 8 articles
In this article, we explore the potential for sea-level change and rocky coastal evolution reconstruction hidden in one of the most intriguing rocky coastal landforms in the Baltic Sea region—rauks. Those unique limestone sea stacks are preserved along the coasts of a number of Swedish islands, with Gotland and Fårö as primary locations. We contributed to the long-standing debate on their origin and attempted to investigate the modern geomorphological processes operating on rauks using novel approaches in rock coast studies, including Schmidt hammer rock tests (SHRT)—to characterize zonation in the degree of coastal landforms weathering; traversing micro-erosion meters (TMEM)—to calculate shore platform downwearing rates; and terrestrial laser scanning (TLS) to monitor coastal abrasion and detect sea-level markers (notches) and describe their size and shapes. Our study shows the dominance of mesoscale changes in the shore platform morphology (erosion scars, spalling, and block erosion) over the microscale downwearing carried out by abrasion. We argue that the preservation of rauks along the modern coast of Baltic islands is a result of a favorable sequence of events including the rapid land uplift and their lithological resistance, significantly higher than observed on surrounding platforms and cliffs formed in different types of limestone. Our findings prove that the microrelief of rauks in the form of well-preserved notches records the sea-level changes from at least the late Ancylus Lake period. Finally, we incorporate the results of our observations into the classic notions of rauk formation and highlight the effect of cutting off sea stacks from the operation of littoral processes as a consequence of land uplift and dominance of subaerial weathering over the wave action.
Until today, we have only a limited understanding of the rocky coastal landscapes that have developed along the Baltic Sea—the shallowest, youngest, and also the largest brackish inland sea in the world, with the basin eroded by the Scandinavian Ice Sheet and characterized by one of the lowest salinity and highest pollution levels among the world marine basins. Over the Holocene, the Baltic Sea has undergone pronounced salinity variations caused by eustatic sea-level rise and rapid glacioisostatic uplift, switching on and off the connection with the Atlantic Ocean (e.g., Björck, 1995; Berglund et al., 2005). The rocky coastal scenery in the Baltic region abounds with a mosaic of picturesque landforms, such as the cliffs of Rügen, Bornholm, and Saaremaa islands or thousands of rocky skerries in the Stockholm and Åland archipelagos. However, from the perspective of the rocky coastal geomorphology the most spectacular landforms are rauks/raukar (rauk—singular)—unique limestone stacks, often with fairylike shapes, located mainly in Gotland, Fårö, and Oland islands in the central part of the Baltic—were subject to only basic scientific characterization (Figure 1). Rauks are present in sites where strata of Silurian limestone and marls that dominate local geology are replaced by massif reef limestones (Figure 2). Fields of stacks consist of reef limestone with a dominance of stromatoporoids (sea sponges) and other resistant corals (Manten, 1971; Sandström, 1998).
FIGURE 1. Study area. (A) Location of Gotland and sister island Färo in central Baltic Sea. (B) Map of Gotland and Färo islands with the location of major rauk fields and study areas; (C) rauk field in Lergrav; (D) rauks in Langhammars; and (E) Asundens rauks.
FIGURE 2. (A) Simplified map of Gotland’s geology. Dark grey dots mark the location of reef facies in limestones that compose rauk fields. 1—study site in Langhammars, 2—study site in Lergrav, 3—study site in Asundens; (B) emergence of coasts of Gotland delimited by highest shorelines of Ancylus Lake, Littorina Sea, and modern coast.
The first scientific notice about rauks was probably made by the famous Carl Linnaeus, the father of modern taxonomy, who visited Gotland in 1741 and described them as stone giants (stenjättar). Surprisingly, although rauks’ natural beauty has already inspired artists (e.g., Ingmar Bergman) and was depicted on national banknotes of Sweden as one of their natural wonders (200 Swedish krona banknote), the debate on their origin and preservation is still ongoing (Linnaeus, 1745; Lindström, 1886; Munthe, 1921; Hadding, 1941; Manten, 1971; Forsberg, 1995; Strzelecki et al., 2020).
In general, it is assumed that rauks exposure started at the turn of the Pleistocene, once the Gotland started to emerge from the Baltic Sea (lake-sea stage cycle). Coastal action removed the stratified sediments that originally mantled the homogenous reef limestone. The latter, being more resistant, has survived as isolated pillars, in coastal geomorphology known as the “sea stacks.” Such a mode of evolution makes the rauks formation peculiar. This is because the classic model of sea stack development assumes the fundamental role of the fracture-guided dissection and the resultant separation of more massive rocky compartments.
Traditionally, sea stacks are interpreted as remnants of the past cliffed coastlines that have been formed once the rocky headlands were cut off from the mainland, through erosion of sections of the coastline with weaker lithology or pre-existing structural features such as faults, joints, and fractures (Shepard and Kuhn, 1983; Trenhaile et al., 1998; Limber and Murray, 2014). Before they become small islands separated from the retreating cliffs, they may constitute the legs of the rocky arches, walls of the tunnels, or columns in the coastal caves. Only a few studies attempted to capture the timing of the modern sea stack formation and collapse and suggest these are rather short-lived landforms, spanning the time from merely several years up to a few decades (Trenhaile et al., 1998). Interestingly, some of the world’s most recognizable sea stack groups are cut in limestone (e.g., the Twelve Apostles, Australia; the stacks of La Jolla and Big Sur, United States; and the Dorset coast, United Kingdom), as is the case of the Gotlandic rauks. According to Bezore et al. (2016), this lithological control may be related to the specific compressive strength (60–170 MPa), making limestone an almost ideal rock type for sea stack formation. The formation of a number of limestone arches, stacks, or coastal caves may also result from the sea transgression on karstic landscapes and the development of a network of sinkholes, tunnels, and cave systems. One of the classic reports on the geomorphology of the coastal stacks, published by Trenhaile et al. (1998) and based on the observations at the Hopewell Rocks, Canada, was focused on the microrelief features that may develop upon stack walls—the coastal notches. Notches are elongated undercuttings, ranging from a few centimeters to several meters deep, carved predominantly by sea erosion enforced by chemical and biological weathering in coastal rocks (Higgins, 1980). Lorscheid et al. (2017) suggested that notch development is controlled by four principal processes: 1) bioerosion operating predominantly on the lower, submerged notch sections; 2) tidally-induced wetting and drying cycles; 3) hyperkarst which shapes subaerial sections of the notch; 4) and mechanical abrasion with the highest range of influence as waves impact both submerged and subaerial parts of the notch. Usually notches develop close to the local mean sea level, so particularly in micro-tidal environments, they may be considered one of the most accurate sea-level indicators (e.g., Pirazzoli, 1986; Kershaw and Guo, 2001; Trenhaile, 2015; Sisma-Ventura et al., 2017). Once the notch is elevated above the sea level (active zone of erosion) either due to the land uplift or sea-level fall, it can persist for a long time and serve as a visible mark of the former shoreline position. Pirazzoli and Evelpidou (2013) emphasized that notches are a coastal geomorphic archive of relative sea level which should be used with care and rather as an assisting feature than the main sea-level change indicator. To date, the majority of sea-level reconstruction studies have explored the potential of coastal notches in the Mediterranean (e.g., Antonioli et al., 2015), Caribbean (e.g., Lorscheid et al., 2017), and other tropical locations (e.g., Rovere et al., 2016). However, as the surface of Gotland’s rauks is also marked by a number of notches of different shapes and sizes, we decided to explore their potential, for the first time, to trace the record of Holocene relative sea-level changes.
Within the context described earlier, this work investigates the geomorphology of rauk fields in northern Gotland and Fårö islands. Our investigative strategy involved the characterization of modern subaerial processes operating on rauk surfaces as well as extracting information about the past sea levels and associated climatic changes from rauk microrelief (coastal notches).
The specific research questions for this study are:
• Were the fluctuations in sea-level changes recorded in rauk coast morphology?
• What is the degree of weathering of modern and uplifted rauk surfaces?
• What processes control the modern erosion of rauk walls and the downwearing of surrounding shore platforms?
• What do the findings tell us about the vulnerability of rauk coasts to coastal and other subaerial processes?
Gotland and Fårö islands are located ca. 80–120 km east of the Swedish mainland (Figure 1) and are characterized by the Baltic semi-continental climate, with cold winters and mild summers. The location on the sea makes the climate milder than on the mainland but also windier. The coastal geomorphology is shaped predominantly by the wave action (with a mean wave height of ca. 1.6 m) as the local sea is almost tideless (Cruslock et al., 2010). According to Boelhouwers et al. (2020), the annual average of 53 frost days, 21 freeze days, and 70 snow days over the last 20 years has been measured at the local meteorological stations. The bedrock of Gotland and its sister island Fårö is comprised Silurian limestones (famous Silurian Klint), marls, and sandstones dipping to the south-east and creating cuesta-like cliffs along the northwestern coast. Those limestone cliffs stand on the flat bedrock platforms extending up to 200 m into the sea, where the steep step leads to deeper waters. To date, three major conceptual theories were presented suggesting that Gotland cliffs are either an inherited, preglacial feature (Lindström, 1886); or a preglacial feature reactivated during and after the glaciation (Rudberg, 1967); or entirely postglacial origin (Forsberg, 1995). A closer look into the Quaternary history of Sweden suggests that the region where present Gotland is located overcame several glacial-interglacial cycles, which had to interfere with bedrock topography through glacial abrasion, quarrying/plucking, and consecutive deposition of glacial sediments. However, the traces of earlier glaciations were erased by the Last Glacial Maximum, which left a rather thin layer of tills and exposed flat and even bedrock surfaces (alvars). The end of glaciation began a history of overlapping lacustrine and marine stages in the Baltic Basin. From 16 ka BP to ca. 12 ka BP (Houmark-Nielsen and Kjær, 2003), meltwaters from decaying ice sheet and rivers from the south and eastern side of the basin filled the Baltic Ice Lake (BIL). At the end of the Pleistocene, once the Scandinavian Ice Sheet (SIS) retreated from the central Baltic, Gotland started the emergence from the BIL. Ice sheet retreat reconstructions by Stroeven et al. (2016) suggest that the ice margin left northern Gotland around 14 ka BP. The system of beach ridges was formed (Mörner & Philip, 1974), which may be found only in the highest, central parts of present-day Gotland (between 83–56 m a.s.l). After the catastrophic drainage of the BIL (ca. 11.9 ka BP), when the water level dropped by ca. 25 m in just 1–2 years, the Baltic gained a connection with the Atlantic Ocean, and the inflow of marine water started. Yoldia Sea stage over the last few hundred years and resulting in another 17 m sea-level regression (Svensson, 1989). The next stage of Baltic Sea isolation from the Atlantic Ocean started in the Early Holocene (ca. 10.7 k BP) when, in response to the collapse of SIS, a significant land uplift occurred. In 300–400 years, the transgressing freshwater Ancylus Lake (ca. 11 m sea-level rise) reached its maximum size. The highest levels of Ancylus Lake are recorded in the beach ridge sequence located ca. 45 m a.s.l. in northern Gotland (Figures 2 A,B). The Ancylus Lake stage ended once the Baltic regained the connection with the Atlantic at ca. 8 ka BP (Björck 1995). However, the majority of rauk fields are preserved along the coastal slopes that were formed during the Littorina Sea (8–4 ka cal BP), which, according to some authors, was a stage characterized by two major transgressions, one around 7.3 ka cal BP and the second around 6 ka cal BP when sea level reached ca. 22–20 m above present sea level (Barliaev, 2017). After the last transgressive phase, the sea level fell rapidly ca. 10 m and around 3.9 ka cal. BP started a gradual fall from ca. 11–10 m a.s.l. to the present. Since around 4 ka BP, when the narrowing of the Danish Straits hampered Atlantic waters influx, the salinity started to decline and the Baltic Sea we know from the present was established. Even without the active ice sheet in the region during the Holocene, the legacy of glaciation was still active in the form of glacio-isostatic adjustment, leading to the uplift of northern Gotland during the Littorina Sea ca. 2.3 mm per year, ca. 2.1 mm per year in the last 4,000 years (Barliaev, 2017) and in the present day slowed down to ca. 1.5 mm per year (Ågren and Svensson, 2011). In general, the Baltic Sea has one of the best-studied Holocene sea-level change histories among world seas. It is, however, important to notice that among over 1,000 accepted Baltic sea-level data points, there is no trace of information derived from coastal notches eroded in rocky coasts (Rosentau et al., 2021).
The majority of the Gotlandic coasts are generally flattish, with a number of shingle and sand beaches, yet the western and north-western parts of the island abound with impressive cliff lines, some of which attain the height of up to 40 m (Rudberg, 1967). In the northeastern, eastern, and southern sectors, the coastal cliffs are present too, but they are much more scattered and limited to single localities (Rudberg, 1967; Eliason et al., 2010). The outline of both the Gotland’s and Fårö’s shorelines is characterized by alternating embayments and headlands, with the former developed along the major fracture zones and/or weaker lithologies (e.g., marlstones and calcarenites) and the latter within the more resistant strata (e.g., Eliason et al., 2010; Boelhouwers et al., 2020).
Rauks—being the remnants of the former coastal cliffs—are developed almost exclusively within the massive, highly homogenous reef limestones and, thus, their distribution is strictly associated with the presence of bioherm/biostrome facies (Figure 2A) (e.g., Svantesson, 2008; Eliason et al., 2010). The majority of rauk fields are present along the present-day shoreline. The most scenic localities include Digerhuvud and Langhammars in the north-western part of Fårö as well as Lergrav, Kyllaj, and Holmhällar in the eastern Gotland. Locally, however, rauks are present in the inland settings—limestone stacks in Boge, north-eastern Gotland, situated hundreds of meters away from the coast, are a fine example (Erlström et al., 2009). The elaborate description of rauks’ distribution and morphology is provided in a historical work of Munthe (1921).
The study was conducted within two representative rauk fields—Langhammars on the Fårö island as well as Lergrav in the north of Gotland. It was supported by the investigation at Asundens, yet here it was limited to the SHRT studies (Figure 1, Figure 4). The reasons standing behind the selection of these particular sites were threefold. First, they involve rauk fields which consist of a large number of sea stacks at various distances from the present-day shoreline and hence allow for the morphometric measurements of notches and SHRT studies on the relevant number of forms. Second, these localities are well-known among the researchers which give the opportunity for comparative studies in the future. Third, the selected rauk fields are very popular tourist destinations—the new data provided in this study may help to enhance the attractiveness of these landforms as a tourist product.
While in all three localities, the sea-stacks have developed upon homogenous reef limestone, they belong to two different stratigraphical units. The rauks of Langhammars are underlain by the so-called Hӧgklint Beds (Manten, 1971), often referred to as the Tofta Formation (e.g., Eriksson and Calner, 2008) or the Kopparsvik Formation (Erlström et al., 2009). Stratified sediments are predominant, and these, in general, include limestones and marly limestones, mantling grey and greyish-white rauk-forming reef limestones (Manten, 1971). The latter two localities are underlined by the Slite Beds, which occupy the majority of the northern Gotland (Manten, 1971). Here as well, the stratified marls and limestones dominate, with SW-NE striking belts of reef limestone giving rise to prominent rauks. From the geomorphological standpoint, the Langhammars locality, situated on the northernmost part of the peninsula of the Fårö island, is by far one of the most spectacular rauk fields of the Gotland archipelago. After Digerhuvud locality, some 4 km to the south, it is the second-largest rauk field on the Fårö island, extending at a distance of some 200 m (Svantesson 2008). It consists of approximately 70–80 sea stacks rising from the shore platform (Eliason et al., 2010). The rauks in Langhammars attain considerable dimensions, with the highest individuals up to ca. 10 m, typically, however, ca. 3–5 m. Fairly similar topography typifies the Asundens rauk field, situated in the eastern part of the former islet, now connected with Gotland by a narrow tombolo. The group of sea stacks, some of which remain in the water, extends to approximately 200 m. Even more extensive is the rauk field at Lergrav, occupying the S-N-trending belt ca. 300 m long. There, however, the geomorphic expression is quite different since none of the stacks is attached to the present-day shoreline anymore. In fact, the rauk field begins some 70 m from the seashore and the ruiniform relief (sensu Migoń et al., 2017) continues upslope, to the sinuous cliff line crowning the forested plateau. The vast majority of rauks here rise to the height of 2–6 m, with only one stack attaining almost 10 m. Yet, this is the Lergrav locality where the most spectacular limestone arch in the Gotland archipelago has developed (Figure 1, Figure 4).
Notches are present in the majority of modern and uplifted rauks in all investigated study sites. In a number of rauks, both seaward and landward notches were developed. However, there is a clear difference in the stage of their development—landward notches are sporadic, less pronounced, and shallower than the seaward notches. This morphological difference is most probably associated with two factors: 1) difference in the period of exposure to wave action and 2) weaker wave energy operating on the landward side of rauk. Seaward notches were formed for a longer time period, as they were exposed to the operation of waves when the landward rauk was still in the cliff wall. Once the rauk was fully exposed from the weaker stratified limestones, the waves could operate around the stack and the landward notch could be formed by the returning waves in a swash zone, characterized by lower energy. A similar difference in notch morphology was also detected by Trenhaile et al. (1998) in their investigations of Hopewell Rocks (Canada), where seaward sides of stacks were prone to more rapid undercutting and more frequent collapse events than the landward sides.
We analyzed geometries of notches detected on the surface of Lergrav and Langhammar rauks (Figure 3). We assumed that the sequence of notches preserved at different parts of the sea stack reflects the cumulative sea-level changes that occurred over time when rauk was in contact with sea waters. We considered that in the tideless Baltic Sea, the dominant process responsible for coastal notch development was most probably the wave abrasion supported by weathering in wetting-and-drying or freezing-and-thawing cycles. In cooler phases of Holocene, when sea-ice cover could maintain on the Baltic Sea over prolonged periods, cutting of notches may be also reinforced by ice foot development and/or ice-pushed boulder movement (Philip, 1990). It is important to emphasize that the majority of uplifted notches observed in the field were easily recognized by their polished surfaces which were associated with wave action. Similar wave-cut and smoothed surfaces were observed at modern rauks and cliffs shaped by waves.
FIGURE 3. Measurements of coastal notches preserved in rauk surfaces. (A) Example of rauk scanned using TLS; (B) parameters of notch measurements applied in this study (after Carobene, 2015). (A) notch upper limit; (B) maximum retreat point; (C) the lower limit of the notch; AB notch roof; BC notch base; (B) the height of point B over the notch floor; bw—base width; d—notch depth; h—notch height; r—roof height; (C,D) rauk field in Langhammars with series of modern and uplifted notches persevered at different elevations; (E) rauk field in Lergrav with well-preserved paleo-notches.
While the presence of horizontal discontinuities in the rock mass may also result in the development of notches and recesses at similar altitudinal levels, as it typically is in the case of the layered sedimentary structures (e.g., Migoń and Duszyński, 2022), such an alternative has been excluded from further considerations in this particular study. This is because the rauk-forming reef limestone is unstratified and highly homogenous, with very irregular and generally indistinct jointing (Manten, 1971). Thus, it was assumed the repeatable pattern of notches and indentations could not have been controlled by the internal rock structure. It should be stressed that no significant differences in rauks’ lithology have been detected in the course of the study.
We decided to use the terrestrial laser scanning (Riegl VZ-4000 laser scanner) for notch detection as suggested by Schneiderwind et al. (2017), who, using examples from the Greek coasts, proved that TLS provides the required precision and resolution to enable detailed analysis of notch morphometry.
We surveyed the geometry of the modern and palaeo-notches preserved in the surface of 18 rauks in Langhammars and 23 rauks in Lergrav study sites. At each site, we scanned rauk field from multiple directions in order to detect both seaward and landward notches. In the majority of observed rauks, the seaward notches were much more clearly visible and well-developed than the sporadic landward counterparts. That is why we did not analyzed morphometric parameters of landward notches. Scanning data were processed in Remote Sensing Lab in Torun, which resulted in the analysis of 88 seaward notch profiles in Langhammars rauk field and 86 in Lergrav. TLS data were used to extract the elevation of modern and palaeo-notches above the present sea level. As there were no tide gauges in the vicinity of studied sites, we have determined the sea level from hourly observations of water levels carried out during the fieldwork. From the scans, we determined the mean high and low water and calculated the arithmetic average (mean tide level—MTL). Using TLS data, we defined the shape and size of notches and their altimetric correlation with the past sea levels when marine action formed them. In the next step, we classified the notch shapes following recommendations for wave-cut notches classification developed by Wziatek et al. (2011) through their observation of formation mechanisms of the Algarve rocky coast in Southern Portugal. In the lab, we also followed the notch measurement approach previously applied by Carobene (2015) along the limestone coasts of the Tyrrhenian and Ligurian Seas (Figure 3). The Genovese scientist described notches using notch upper limit; maximum retreat point; lower limit of the notch; notch roof; notch base; the height of maximum retreat point over the notch floor; notch base width; notch depth; notch height; and roof height. Those numerical parameters precisely described the shape, size, and variability of studied notches and should allow comparison with notch forms from other regions and coastal environments.
In the field, we observed that groups of notches are concentrating on different levels. Visual inspection of scans obtained from TLS confirmed our conviction that there is a spatial pattern in their altimetric distribution across rauk fields (Figure 6). But in order to test whether notches detected in rauks’ surfaces in the field and TLS scans are not only grouped in a particular altimetric pattern but also morphometrically differ from each other, we applied the k-means clustering method in Statistica 13. K-means clustering was carried out with the option of selecting observations in order to maximize the clustering distance. We used the following notch morphometric parameters applied to describe notch geometries by Carobene (2015) in our analysis: height of the notch base and roof edge in meters above sea level, notch depth, the width of notch base, notch roof height, and base height. In general, the base of a notch is treated as the best and most precise marker of mean palaeo sea level because its width is closely linked to the local tidal range (Rovere et al., 2016; Lorscheid et al., 2017; Antonioli et al., 2018). The results of the grouping by the k-means method were confirmed by Ward’s method. On the basis of the performed statistical tests supplementing the field research, the detected notches were divided into particular levels. Five statistically significant levels of notches were found in Langhammars (Levels 1–5 in Table 1) and three in Lergrav (Levels 6–8 in Table 1). The normality of the distribution was checked in the extracted levels with the Shapiro–Wilk test. Then, the nonparametric Kruskal–Wallis test was applied, which allowed comparisons to be made between the extracted levels.
TABLE 1. Morphometric characteristics of rauk notches from Levels 1–5 (Langhammars) and Levels 6–8 (Lergrav). For notch parameters used in calculations, see Figure 3.
We have applied an electronic N-Type Silver Schmidt Hammer manufactured by Proceq to detect changes in rock surface resistance of rauk notches present at different elevations and distances from the sea at three sites: Langhammars, Lergrav, and Asundens (Figures 4A–C). The Schmidt hammer measures the rebound of a spring-loaded mass as it impacts on a rock surface, providing an arbitrary measure of rock resistance on a scale with a value range of 10–100 (R-value). The difference in surface resistance detected by the Schmidt hammer can be treated as a measure of the degree of weathering of the tested rock surface which also implies an indirect measure of time; the weathering processes could operate on a given surface (Goudie 2006). In our case, we compared the surface resistance of rauk notches along the profiles starting at the notch closest to the present shoreline through notches preserved in uplifted rauks located at different elevations. We hypothesized that the longer the notch was exposed to subaerial weathering (time since cutting off the wave action and refreshing the rock resistance through abrasion), the lower the resistance of their surfaces will be detected by SHRT. Prior to this study, we ran a pilot study Lergrav (Strzelecki et al., 2020), where we were able to test notches at six levels and carried out the final measurement at the surface of the top of the plateau (alvar) edge above the rauk field (from ca. 4 m a.s.l. to 27 m a.s.l.). In Langhammars, we tested notches at five different levels (from 0 m a.s.l. to ca. 10 m a.s.l.). In addition to the two main sites in Langhammars and Lergrav, we tested notches in Asundens rauk field. Each test comprised 25 measurements made at points randomly selected from a ca. 0.10 × 0.10 m area. The statistical study by Niedzielski et al. (2009) suggested that this number of readings provides an appropriate accuracy of SHRT in the majority of lithologies. Measurements were conducted on sunny and warm days when the rock surfaces were dry. We followed the approach to display the results of SHRT presented by Ericson (2004) and summed up the calculated R-value using box plots with the shape of the distribution, median, and variability of detected resistance of notch surfaces (Figure 4D).
FIGURE 4. The results of Schmidt hammer rock tests across the rauk fields at (A) Lergrav, (B) Langhammars, and (C) Asundens. (D) Box plots showing the variability of rock hardness across the test sites. The exact location of the tested surfaces at each rauk field is shown at (A–C).
As noted by Gómez-Pujol et al. (2006), Fårö island is one of the few places along the Swedish coast where shore platforms are presented in a modern coastal zone. We plied traversing micro-erosion meter (TMEM) to determine the recent rates of shore platform downwearing in Langhammars site, where the well-developed platform (approx. 70 m wide, with 20 m submerged even during the low tide) is located at the sea-level ca. 400 m from the rauk field (Figure 5). The tool used in this study was designed and manufactured by Albatros Marine Technologies (Palma de Mallorca, Spain). TMEMs measure erosion caused by mechanical, chemical, and biological processes with a high precision positioning system and have been successfully applied to study rock surface down-wearing rates in various coastal environments all around the world (Stephenson and Finlayson, 2009; Stephenson et al., 2019; Yuan et al., 2022). TMEM records the elevation of the rock surface relative to bolts installed on the shore platform. The repeating of measurements allows for detecting surface downwearing or swelling over the years. At least, 10 readings were collected from each TMEM station, generating reasonable estimates of rates of surface change (Trenhaile and Lakhan, 2011).
FIGURE 5. Exposed shore platform nearby Langhammars rauk field, where an attempt to monitor platform downwearing rates was carried out in years 2019–2021. (A) Almost horizontal shore platform with a small inward slope following the dip of limestone strata. Orange dots mark the location of TMEM stations. (B) Shore platform during the stormy conditions when the entire surface is inundated by approaching waves; (C) remnants of rauks and rock blocks eroded directly from the platform spread across the platform; (D) erosion scar formed between 2016–2019, eroded block/slab was not located in the surroundings, suggesting further disintegration and transport to deeper waters by waves; (E) freshly exposed rock surface through spalling of platform; (F) smoothed and polished by pebble movement platform in an abrasion strip close to the high tide; (G) smoothed/abraded section of platform covered by ca.0.4–0.5 m thick gravel-dominated beach sediments after storm season. The same platform was sediment-free season before. (H) Rugged surface with full biological coverage near low tide.
Analysis of TLS data allowed us to ground-truth field-based observations of well-developed coastal notches formed in the surface of Langhammars and Lergrav rauks (Figure 6). In our analyses, we focus on well-developed and clearly visible seaward notches. The landward notches detected in a number of rauks were less clearly visible and much shallower than the seaward notches. The results of the statistical analyses prove that there are statistically significant (p < 0.05) differences between the analysed notch levels (Table 1).
FIGURE 6. Levels of uplifted paleo-notches in Langhammars (levels 1–5) and Lergrav (Levels 6–8) detected by TLS. The relative sea-level curve in the background after Barliaev (2017). Note two-phased Littorina Sea transgression that could have a significant effect on wave-cutting of bigger notches in rauks emerging from Baltic between 6,000—8,000 years ago. Deeper notches start to form ca. 4,000 yrs ago, when the modern Baltic Sea was established, and the rate of land uplift slowed down allowing wave action to operate longer on the same rock surface.
Edges of coastal notch bases in Lergrav were detected at three elevation ranges: lower 11–18 m a.s.l. (Level 6, Table 1); medium 18–22 m a.s.l. (Level 7); and upper 22–27 m a.s.l. (Level 8). Notches from the highest level had relatively symmetrical U-shape profiles with an r/b ratio of 1.3 and were characterized by a mean depth of 0.25 m and a height of 1.24 m (shape ratio d/h of 0.23). It is important to note that without large notches formed through the connection of smaller notches, the average notch height is. 1 m. This group of notches was the most numerous (48 notches detected); 27 notches of Level 7 had a similarly symmetrical r/b ratio of 1.32 as in the upper level. The majority of notches were rather shallow (mean depth of 0.28 m) and their height ranged between 0.6 and 3.9 m. However, field inspection of the highest notches at this level induced us to describe them as multi-notch formed after the connection of a sequence of smaller notches. In this group, we also detected a couple of two-step notches, where a higher step resembles a surf notch distinguished by Moura et al. (2006) and Wziatek et al. (2011).
Third, the lowest level of notches in the Lergrav rauk field was characterized by a similar notch incision as in Level 7 (mean notch depth of 0.28 m) and r/b ratio of 1. In this level, we found the highest multi-notch form among investigated rauks, with a notch roof located 6 m over the base. Such a large feature could only be formed through multiphase erosion linking smaller notches into one wide smooth hollow, most probably over multiple sea-level transgressions into the rauk field.
In Lergrav, the statistically significant differences between the analyzed notch levels occur for the parameters: height of notch base edge m a.s.l., height of notch roof edge m a.s.l., and height of notch base edge m above rauk base, Height of notch deepest point m a.s.l., r—roof height, b—base height, h—notch height. At the same time, the statistically significant differences did not occur for parameters: d—notch depth and bw—base width. The lack of statistically significant differences between d and bw parameters may be due to the short time in which the notches were formed, which relates to the rate of glacioisostatic cutting off the stack from the sea.
Slightly below the elevation level of Lergrav notches from Level 6, we detected a group of 25 notches in the highest parts of Langhammars rauk field (Level 5: 9–12 m a.s.l.), which were slightly shallower than the lowest notches of Lergrav site (mean depth of 0.21 m and mean height of 1.23 m) but kept symmetrical U-shape profiles with an r/b ratio of around 1.3. Notches located between 7 and 9 m a.s.l. (Level 4) had very similar geometries with a mean depth of 0.22 m and height of 1.34 m but had more asymmetrical U-shaped profiles with an r/b ratio of 0.97. Interestingly, this was the first notch level where overhanged notches dominated those with more sloping profiles (Figure 6). Notches of Level 3 (elevation range 4–6 m a.s.l.) were the deepest notches among the detected population (mean depth of 0.74 m) and returned to the more symmetrical U-shaped profiles (r/b ratio of 1.2). Another characteristic feature of this notch level was that most of the notches were higher than 2 m, and on the contrary to Levels 4–8, we did not detect smaller forms which usually range between 0.25—0.8 m. Level 2 notches in Langhammars maintained the symmetrical U-shaped profiles (r/b ratio of 1.22) and was dominated by ca. 1.15 m high notches with depths ranging between 0.12 and 0.47 m. At this level, we also found a couple of examples of two-step notches with upper step similar in shape to surf notches described from Portuguese rocky coasts (Wziatek et al., 2011). The lowest notch level in Langhammars (Level 1), where a couple of notches are still in the range of large storm waves (notch base edges elevation between 0.75 and 1.75 m a.s.l.) was the second group of notches characterized by asymmetrical U-shaped profiles (r/b ratio of 0.81). This group was, however, the smallest among the tested notch levels (we detected only 5 notches at this level). In Langhammars rauks, the statistically significant differences occur for all analyzed parameters, and only the analyzed d/h and r/b ratios did not show significant statistical differences among notches.
Our pilot SHRT tests across notches of the Lergrav rauk field clearly showed the seaward increase in their rock surface resistance (Figure 4), suggesting that the longer time rauks were exposed to the operation of subaerial processes due to the land uplift and cut-off refreshing of rock surface by wave abrasion, the more weathered surface was detected in notches (Strzelecki et al., 2020). Notches in rauks closest to present shore (ca. 4 m a.s.l.) and cut off from the sea in the last 1,000–2000 years, we detected mean rock surface resistance (R-value) of 51 whereas the notches located at 20 m a.s.l. were characterized by significantly lower resistance with mean R-value of 40 (Figure 4D). We ran a similar test across a Langhammars rauk field, where we could reach the modern notch still under the influence of wave action and four levels with uplifted notches that emerged from the Baltic Sea in the last 4,000 years. Similarly, to Lergrav rauks, Langhammars notches located closer to the sea level had less weathered surfaces. SHRT in modern and wave-cut notch at the modern shoreline, with very smooth and wave polished surface indicated mean R-value of 59, whereas highest tested notch at ca. 10 m a.s.l. had rugged, weathered, and lichen-covered surface characterized by mean R-values of 49. The final SHRT campaign carried out in Asundens caught a similar tendency as in the first two rauk fields, with a weakening of notch surface with elevation and distance from the modern shore. The results demonstrated that notches exposed for a longer period of time to subaerial processes (e.g., freeze-thaw cycles) are characterized by lower resistance.
We observed a clear zonation in the dominance of processes shaping morphology of modern shore platform in Langhammars site (Figure 5A). At the seaward edge of the platform (ca. 50 m from the shoreline), which ends abruptly with a ca. 0.6 m step, most of the rugged platform surface is clean of gravel or sand but fully covered with blue-green algae (Figures 5B, H). Below the erosional step, on the surface of the submerged part of the platform, numerous blocks (pieces of broken platform or remnants of collapsed rauks) are present, also covered with algae (Figure 5C). In the next 30 m of the platform between the step and abrasion zone close to the pebble storm ridge, the majority of erosional marks and steps formed after the detachment and displacement of platform fragments were found (Figure 5D, E). Numerous pools located in this section of the platform were filled with beach pebbles. The movement of pebbles and gravel by waves polishes the pool edges and smooths the bottoms of the pools, resembling the potholes (eversion kettles). The landward edge of the platform is buried in storm ridges composed of coarse gravels and pebbles. The surface of the platform between the shoreline and ca. 10 m seaward is very smooth and even due to the intensive abrasion. The sediment coverage and movement along the platform differ from season to season e.g., the platform which in 2019 and 2020 was clean of sediments, in 2021 was covered with over. 0.5 m layer of gravel-dominated sediments (Figures 5F, G). In most of the sites in Gotland, our TMEM observations carried out between 2019–2021, resulted in rather moderate success, as a number of stations were lost either by coverage of shore platform by thick (ca. 0.5 m) layer of gravels or by tilting pins by rock blocks/boulders, which moved across the platform during winter storm season. Similarly, to Swantesson et al. (2006), who monitored modern rates of platform lowering in Fårö island in the years 1999–2001, our limited observations from Langhammars showed very scattered values of platform change (maximum annual lowering of 0.287 mm and maximum swelling of 0.289 mm) and are rather inconclusive. Apart from measurements of rock surface change conducted on shore platforms, we also tested the rates of micro-erosion on uplifted rock surfaces (base of rauks) located further inland and cut-off influence of wave action and exposed to weathering processes only. Between 2019 and 2021, we captured a lowering of 0.472 mm and a swelling of 0.227 mm. In general, the TMEM observations of shore platform changes were inconclusive, and it is difficult to use their results to explain the erosion of limestone strata around the rauks. Field observations suggest that the key process transforming the relief of shore platforms in Langhammars was block erosion and dragging of blocks across platform leading to erosional scars (Tyszkowski et al., 20221). In nearby Digerhuvud (largest rauk field in the region), Forsberg (2001) carried out calculations of the rate of shore platform breakdown across an experimental test site (25 × 25 m) and suggested corrosion as the main process responsible for loosening small fragments of bedrock. He obtained a mean value of 0.9 mm of shore platform downwearing and concluded that modern platform started to form at the end of the Littorina period (ca. 4 ka cal BP) and reached the balance between the rate of downwearing (through erosion and weathering) and shore displacement.
The geomorphological investigations carried out in this study allowed us to capture a number of mechanisms operating on the surface of modern and uplifted rauk coasts.
We confirmed that the development of shore platforms formed in the extension of modern rauk fields is dominated by mesoscale processes including spalling and breakdown of platform fragments as indicated in previous studies (Forsberg, 2001; Gómez-Pujol et al., 2006; Swantesson et al., 2006; Cruslock et al., 2010). The almost undetectable micro-erosion of shore platform surface may be attributed to low efficiency of bioerosional processes or simply low salinity of Baltic waters which is also unfavorable for erosion. On the other hand, in the abrasion zone, where we saw the smoothest and clearly abraded surfaces, we were not able to confirm the visual observations with the quantitative data from TMEM monitoring, as we lost access to all stations covered by a thick layer of pebbles and gravels deposited during winter storms (Figures 5F, G). The new information obtained during our field observations is certainly the relatively large accumulation of broken rock blocks in the middle and seaward zones of shore platforms (Figure 5C), often located close to mounds or higher areas on the shore platform surface (Figures 5A, H). Closer inspection of those landform assemblages (platform mound—rock block accumulations) leads us to believe that larger blocks present in the intertidal zone are remnants of collapsed rauks, and the visible elevations on the surface of the platform are remnants of rauk bases (rauk stumps). In the number of sites, where rauk fields are present in a modern coastal zone and directly exposed to storm waves, only a few stacks are still preserved and most of them are already removed from the platform surface (Figure 8). Forsberg (2001) noticed that rauk can fully disappear from the coastal zone in 82 years (comparison of images taken in 1918 and 2000). This corresponds to observations by Trenhaile et al. (1998) of stacks in Hopewell Rocks (Canada), which may separate from the cliff, evolve into well-undercut mushroom-shape forms, and collapse in ca. 100–250 year cycle. The mechanism that can preserve isolated stacks from the wave abrasion and consequent collapse is the glacioisostatic uplift. Insight into the results of SHRT carried out on the surfaces of uplifted rauks notches (Figure 4D) shows that with time since the separation from the sea, the rauk rock surface weakens due to the weathering. Both the low mean R-values and the dispersion of individual SH readings well testify that the notches located further inland (time of exposure from wave action) are weakened, possibly in the course of protracted weathering. Interestingly, even in the highest notches, cut off from the sea several thousand years ago, the marine character (wave polished surface) is not erased from rauk morphology. Currently, the notches in all studied locations are well-preserved in the rauk surfaces and resistant to severe degradation. Boelhouwers et al. (2020) investigated the long-term frost weathering of limestone clasts in beach ridges spread around rocky headlands, where rauk fields are located. They observed the increase in clast weathering and break down process in beach ridges formed in the last 2000 years. The reaction of the rauk surface to frost weathering is, in our opinion, slower, as the significant drop in the degree of rock surface weathering picked up in SHRT was observed on rauks separated from the sea ca. 4,000 yrs ago. However, this difference in the sensitivity to weathering between limestone clasts building local beaches and rauk surfaces may also confirm the general higher resistance of hard coralline reefs building rauks in contrast to weaker stratified limestones and marls that were most probably the main source of beach material.
The comparison of rauk notches analyzed in this study with notches formed in lower latitudes (Mediterranean and Caribbean) showed a number of morphological differences. First of all, rauk notches are much shallower and broader which contrasts with deep and narrow notches in tropical seas, notches cut predominantly by bioerosion and chemical weathering. The size of notches is related to exposure to wave action with larger forms found along coasts influenced by intensive (prolonged) wave impacts (Pirazzoli, 1986; Moura et al., 2006; Wziatek et al., 2011), therefore, we classify rauk notches into two main groups—dominant broad abrasion-type notches and secondary hook-shape surf notches, where apart from mechanical abrasion operation, the notches were shaped by wave quarrying and interaction with sea spray. This explains a fairly wide range in notch heights captured at similar elevations, suggesting the dominant role of exposure to the most powerful waves. Rovere et al. (2016) reviewing relative sea-level indicators noticed that abrasion notches have a large indicative range reaching from the storm swash wave height to the breaking depth of significant waves. The shallow depth of rauk notches may be an indicator of a rather short duration of time when waves could operate on the given section of rauk surface or, in other words, constitute the record of rapid uplift of shore, cutting off rauk field from the direct operation of waves. We hypothesize that although the wide range of rauk notch widths only provide a rough indication of a mean sea level at the time of formation but may provide insight into the intensity of wave climate (storminess, sea-ice cover, etc.). To some extent, the formation of notch sequences in the surface of rauks fits the model of notch development proposed by Cooper et al. (2007), who put forward that the notch sequences form when sea level rise is outpaced by the coastal uplift rate and that individual notch is formed when stable climate facilitated sustained erosion. Although in their case, the focus was on a typical tidal notch, where the main driver of notch development was bioerosion sustained by stable climate conditions. Cooper et al. (2007) proposed that instability in climatic conditions may terminate notch development and its preservation depends on the rate of the uplift. The cycle is repeated during another phase of climate stability. In the case of rauks, the termination of notch formation also occurred once the land uplift outpaced the sea-level rise, but during the phase of notch development, it was the duration of intensive wave action that mattered, not the stability of the climate. We even risk saying that the more unstable (stormy) the Baltic was during the past, the higher the possibility of notch formation and, as a consequence, form preservation. Here, it is important to mention that preservation of sea stack in a coastal landscape through uplift is only one of the possible scenarios. The second one, described by Bezore et al. (2016), maybe the drowning of stacks due to the rapid rise of the sea level, which happened along the Twelve Apostles coast in Australia.
The elevation range of Level 8 notches suggests the Early Holocene timing of their formation when the sea level experienced a rapid drop. The majority of highest notches in Lergrav are shallow, suggesting a short period of wave operation. The height of notch base edges excludes the possibility of another reworking of notch surface by marine action in younger phases of Holocene and directs our interpretations toward the end of the Ancylus Lake period when sea-level experience a massive, over 20 m drop (e.g., Rosentau et al., 2020). Notches of Level 7 are examples of repeated remodeling by wave action associated with the two-phased Littorina Sea transgression that peaked around 7.4 cal ka BP and 6.1 cal ka BP (Barliaev 2017). The first phase of this quick Littorina Sea transgression was found at a number of sites around the Baltic. A study of isolation basins in SE Sweden provided Yu et al. (2007) with evidence for a rapid sea-level rise ca. 7.6 cal ka BP when the Baltic rose by at least 4.5 m when flooding induced by meltwater pulse occurred. North-east to our study site, in the eastern Gulf of Finland, Rosentau et al. (2013) detected the culmination of the transgression record at ca. 7.3 cal ka. BP. Similar information was more recently extracted from coastal environments of western Hiiumaa island (Rosentau et al., 2020). In Gotland, Apel et al. (2018) found a decline in human activity between 7.6 and 6.0 cal ka BP and also associated it with sea transgression. In our opinion, the second phase of Littorina transgression around 6.1 cal ka BP explains the increased appearance of merged notches (double notches) and a significant increase in average notch height. We also detected an increase in average notch depths in Level 7 and Level 6 which could be caused by remodeling of rauk surfaces during the first and second phases of Littorina transgression. To some extent, the high notches observed in rauk surfaces resemble a smoothed version of double notches described along the Mediterranean coasts. Antonioli et al. (2006), who studied the mechanism responsible for the formation of double marine notches along the Italian rocky coast, associated their compound morphology with isostatic movement during one sea highstand, with a lower and larger notch formed during the first phase of a highstand and upper notch developing in the final stage of highstand, when isostatic movement slowed down or even diminished.
Notches of Langhammars (Levels 1–5) were eroded during the subsequent steady sea-level fall from ca. 6 cal ka BP. The slowed-down rate of sea-level fall allowed longer operation of waves on rauk walls and the formation of higher and deeper notches. This is particularly a case of notches formed in the last 4,000 years so when modern Baltic originated.
It seems the results demonstrated in this study have yet another implication, of special significance if the patterns of landform evolution are concerned. It was 1745 when Carl Linnaeus noted that rauk fields “(…) had been formerly a limestone mountain, the roots of which had been ground, cut, and formed by the heaving waves of the sea, till it finally left these stones in their present form” (p. 218, translation after Eliason et al., 2010). A hundred years later, Sir Rodrick Murchison presented a similar point of view regarding the origin of sea stacks of Gotland, stating that they are “(…) dismantled portions of former hard coralline reefs (…)” (Murchison 1946a, p. 20) and “(…) probably worn by the powerful action of water (…)” (Murchison 1946b, p. 359). Although the general concepts of these early naturalists have remained tenable to the date, no unequivocal evidence of the crucial morphogenetic role of sea abrasion in the emergence of rauks has ever been presented. Thus, various researchers have only hypothesized that the less resistant material (stratified limestone abounding with lines of discontinuities) has already been washed away, and the present-day rauks are remnants of highly homogenous cores of Silurian reefs (e.g. Svantesson, 2008; Erlström et al., 2009; Johansson, 2017), altogether forming a ruiniform morphology (Migoń et al., 2017).
The identification of notches and indentations cut in rauks at repeatable altitudes, somehow overlooked till the present day, seems to serve as the only proof of sea abrasion gradually exposing these impressive landforms (Figure 8). While the less resistant material mantling the rauks must have been removed, leaving no traces of its former presence, the action of sea waves has only “sculpted” the reef limestone, providing a clear record of the type of erosional agent as well as the interplay between transgressing sea and isostatic uplift. Presumably, if the studied rauks had remained attached to the seashore for longer, they would have been destroyed, similarly as it has happened at Digerhuvud, Gamlehamn, Holmhällar (Figure 7), or Hammarshanghällar, as shown by Rudberg (1967). Hence, isostatic uplift protected sea stacks from further erosional action and likely enables their longevity (Figure 8).
FIGURE 7. Examples of ruined rauk coasts with stacks (rauks) undercut by waves and collapse on the shore platforms: (A) largest collapsed rauk field in Digerhuvud, Fårö; (B) disintegrated rauks and rock blocks chipped of limestone cliffs in Gamlehamn, Fårö; (C) “stumps” of collapsed rauks in Holmhällar, Gotland.
FIGURE 8. The conceptual model of rauk field development along the coast of Gotland. (A) The Gotland region is overridden by an ice sheet during the last glaciation. Limestone bedrock is exposed to subglacial erosion. (B) Glacially modified bedrock is exposed to ice. Weaker limestones are cracked and shattered by glacial exaration and/or shattered by periglacial/proglacial conditions and prepared for subsequent abrasion by waves. (C) Baltic Sea in a complex cycle of transgressions and regressions erodes and removes weaker limestone strata from around the rauk. Abrasion forms sea stacks and produces the notches. (D) Glacioisostatic uplift separates sea stacks from the sea, making them residual landforms, no longer subject to wave abrasion. At present, the rauks are subject to limited physical weathering (e.g., frost action and chemical weathering), yet their microrelief maintains a marine legacy.
The present study comprises one of the first efforts to investigate in detail the processes controlling present-day development of rauk coast geomorphology in Gotland, Sweden, and is the first attempt to use the wave-cut notches preserved in rauk surface to improve our understanding of the impact of Holocene relative sea-level changes on Baltic rocky coast environments.
As a result, the present findings led us to the following conclusions:
1) Major rauk fields of northern Gotland—Lergrav and Asundens sites and Färo–Langhammars site—are well-preserved in the coastal landscape due to the glacioisostatic uplift, which cut off sea stacks from the abrasive action of waves. In other locations such as Digerhuvud or Hammarshanghällar, where rauk fields are situated closer to the present shoreline and are exposed to the operation of modern coastal processes, longer stacks are often cut by waves and collapse on the shore platforms.
2) Wave abrasion is a dominant process shaping the surface of rauks and controlling the resistance of rock surface in wave-cut notches. Schmidt hammer rock tests show that the notches separated from the operation of waves by land uplift are vulnerable to surface weathering. In all SHRT profiles, we detected a landward trend of increased rock surface weathering of rauk notches.
3) Morphometry and shape characteristics of well-preserved notches in Lergrav and Langhammars indicate the potential relationship between the rate of sea-level fall and notch size. Lower and shallower notches were typical in rauks, emerging from the sea during phases of rapid sea-level fall—at the turn of Anyclus Lake. Higher and deeper notches are much more common in rauks shaped during the monotonous sea-level fall observed during the last 4,000 years when land uplift slowed down. The largest notches in rauks resemble double-notch forms described previously along the Mediterranean coast and were formed predominantly in rauks that experience the operation of waves during repeated sea transgressions (two-phase Littorina Sea transgression).
4) Measurements of modern rates of shore platform downwearing using the TMEM showed very limited efficiency of abrasion. Major changes in the platform morphology were caused by the removal of fragments of the shore platform leaving signs of erosional scars.
5) We propose a conceptual model of rauk coast evolution and preservation in the modern coastal landscape which relies on the interplay between efficiency of wave abrasion exposing resistant rauks from weaker limestone strata and rates and trends of sea-level changes. We agree with classic notions on post-glacial formation of rauk fields, although postulate the importance of glacial preconditioning in the form of erosion and disintegration of limestone surrounding resistant reefs that could, later on, facilitate wave erosion and removal of shattered material from around the rauks. Continued land uplift separated rauks from erosive wave power and secured the preservation of relict rauks in the landscape. Preserved rauks are only smoothly reshaped by weathering processes (freezing-thawing) which so far did not manage to erase the coastal legacy of their morphology.
The present findings constitute a significant improvement in our understanding of the rauk coast (sea stack) development in the micro-tidal and brackish Baltic Sea and highlight the unique potential of modern and relict rauk fields for further geoheritage and geodiversity studies, revealing the richness of Baltic Sea rocky coast environments.
The original contributions presented in the study are included in the article/Supplementary Material; further inquiries can be directed to the corresponding author.
MCS, FD, ST, and LZ participated in the fieldwork. MCS and FD analyzed SHRT and TMEM observations and conducted geomorphological mapping. ST and LZ carried out TLS and analyzed the rauk notches' morphometric properties. All authors prepared figures. MCS and FD wrote the draft manuscript with equal input from ST and LZ.
This is the contribution to the National Science Centre in Poland project ‘RAUK- forgotten witness of Holocene sea-level changes and development of Baltic rocky coasts’ (UMO-2016/21/D/ST10/01976). Investigations of rauk coasts led by MCS are a contribution to the IAG Rock Coasts Working Group.
The authors declare that the research was conducted in the absence of any commercial or financial relationships that could be construed as a potential conflict of interest.
All claims expressed in this article are solely those of the authors and do not necessarily represent those of their affiliated organizations, or those of the publisher, the editors, and the reviewers. Any product that may be evaluated in this article, or claim that may be made by its manufacturer, is not guaranteed or endorsed by the publisher.
Our appreciation is extended to Anki from the STF Bunge Camping side for providing accommodation and information about rauk coasts during our stays in Gotland. We are also grateful to Sebastian and Krzysztof from the NAVIGA company for providing logistical support during fieldwork. The authors gratefully acknowledge the cooperation of the librarian staff of Stockholm University and Karlstad University, Sweden, and their help in a literature search. Critical remarks and comments by four reviewers also helped improve the manuscript.
1Tyszkowski, S., Zbucki, L., Kaczmarek, H., Duszyński, F., Strzelecki, M. C. (2022). Terrestrial Laser Scanning for the Detection of Coastal Changes along Rauk Coasts of Gotland, Baltic Sea. manuscript in preparation.
Ågren, J., and Svensson, R. (2011). The Height System RH 2000 and the Land Uplift Model NKG2005LU. Map. Image Sci. 3, 4–12.
Antonioli, F., Ferranti, L., and Kershaw, S. (2006). A Glacial Isostatic Adjustment Origin for Double MIS 5.5 and Holocene Marine Notches in the Coastline of Italy. Quat. Int. 145-146, 19–29. doi:10.1016/j.quaint.2005.07.004
Antonioli, F., Ferranti, L., Stocchi, P., Deiana, G., Lo Presti, V., Furlani, S., et al. (2018). Morphometry and Elevation of the Last Interglacial Tidal Notches in Tectonically Stable Coasts of the Mediterranean Sea. Earth-Science Rev. 185, 600–623.
Antonioli, F., Lo Presti, V., Rovere, A., Ferranti, L., Anzidei, M., Furlani, S., et al. (2015). Tidal Notches in Mediterranean Sea: A Comprehensive Analysis. Quat. Sci. Rev. 119, 66–84. doi:10.1016/j.quascirev.2015.03.016
Apel, J., Wallin, P., Storå, J., and Possnert, G. (2018). Early Holocene Human Population Events on the Island of Gotland in the Baltic Sea (9200-3800 Cal. BP). Quat. Int. 465, 276–286. doi:10.1016/j.quaint.2017.03.044
Barliaev, A. (2017). Paleogrography and Shore Displacement of Eastern Gotland between 9.5 and 2.8 Ka Cal BP (Stockholm: Stockholm University), 69. MSc thesis NKA193/2017.
Berglund, B. E., Sandgren, P., Barnekow, L., Hannon, G., Jiang, H., Skog, G., et al. (2005). Early Holocene History of the Baltic Sea, as Reflected in Coastal Sediments in Blekinge, Southeastern Sweden. Quat. Int. 130, 111–139. doi:10.1016/j.quaint.2004.04.036
Bezore, R., Kennedy, D. M., and Ierodiaconou, D. (2016). The Drowned Apostles: The Longevity of Sea Stacks over Eustatic Cycles. J. Coast. Res. 75, 592–596. doi:10.2112/si75-119.1
Boelhouwers, J., Andersson, C., Berg, R., Asad Kandastar, R., Sjöman, A., and Vainionpää Lindgren, E. (2020). Long-term Frost Weathering Rates of Limestone Beach Clasts, Fårö Island, Central Baltic Sea. Geogr. Ann. Ser. A, Phys. Geogr. 102, 12–32. doi:10.1080/04353676.2019.1704488
Carobene, L. (2015). Marine Notches and Sea-Cave Bioerosional Grooves in Microtidal Areas: Examples from the Tyrrhenian and Ligurian Coasts-Italy. J. Coast. Res. 313 (3), 536–556. doi:10.2112/jcoastres-d-14-00068.1
Cooper, F. J., Roberts, G. P., and Underwood, C. J. (2007). A Comparison of 103-105year Uplift Rates on the South Alkyonides Fault, Central Greece: Holocene Climate Stability and the Formation of Coastal Notches. Geophys. Res. Lett. 34, L14310. doi:10.1029/2007GL030673
Cruslock, E. M., Naylor, L. A., Foote, Y. L., and Swantesson, J. O. H. (2010). Geomorphologic Equifinality: A Comparison between Shore Platforms in Höga Kusten and Fårö, Sweden and the Vale of Glamorgan, South Wales, UK. Geomorphology 114, 78–88. doi:10.1016/j.geomorph.2009.02.019
Eliason, S., Bassett, M. G., and Willman, S. (2010). Geotourism Highlights of Gotland. Tallin: NGO GEOGuide Baltoscandia, 5–41.
Ericson, K. (2004). Geomorphological Surfaces of Different Age and Origin in Granite Landscapes: an Evaluation of the Schmidt Hammer Test. Earth Surf. Process. Landforms 29, 495–509. doi:10.1002/esp.1048
Eriksson, M. J., and Calner, M. (2008). A Sequence Stratigraphical Model for the Late Ludfordian (Silurian) of Gotland, Sweden: Implications for Timing between Changes in Sea Level, Palaeoecology, and the Global Carbon Cycle. Facies 54, 253–276. doi:10.1007/s10347-007-0128-y
Erlström, M., Persson, L., Sivhed, U., and Wickström, L. (2009). Beskrivning till Regional Berggrundskarta Över Gotlands Län. Uppsala: Sveriges Geologiska Undersökning, 60. K221.
Forsberg, P. (2001). Bjärget - Geomorfologisk Beskrivning Av Raukstråket Och Kustplattformen Vid Digerhuvuds Naturreservat, Fårö, 26. Karlstad, Sweden: Karlstad University Studies, 39.
Forsberg, P. (1995). Geomorfologiska Studier Av Kustplattformen Utmed Gotlands Nordvästra Klintkust, 101. Sweden: Univ. of Stockholm, Dep. of Phys. Geography. Forskningsrapport.
Gómez-Pujol, L., Cruslock, E. M., Fornos, J. J., and Swantesson, J. O. H. (2006). Unravelling Factors that Control Shore Platforms and Cliffs in Microtidal Coasts: The Case of Mallorcan, Catalonian and Swedish Coasts. Z. für Geomorphol. N. F. Suppl. 144, 117–135.
Goudie, A. S. (2006). The Schmidt Hammer in Geomorphological Research. Prog. Phys. Geogr. Earth Environ. 30, 703–718. doi:10.1177/0309133306071954
Hadding, A. (1941). The Pre-quaternary Sedimentary Rocks of Sweden, 37. Lund: C.W.K. Gleerup, 1–137. 6. Reef limestones. Lunds Univ. l r s s k r . N.F., Avd.2.10
Higgins, C. G. (1980). Nips, Notches, and the Solution of Coastal Limestone: an Overview of the Problem with Examples from Greece. Estuar. Coast. Mar. Sci. 10, 15–30. doi:10.1016/s0302-3524(80)80046-6
Houmark-Nielsen, M., and Henrik Kjaer, K. (2003). Southwest Scandinavia, 40-15 Kyr BP: Palaeogeography and Environmental Change. J. Quat. Sci. 18, 769–786. doi:10.1002/jqs.802
Johansson, A. E. (2017). Geomorfologisk Klassificering Och Kartering Av Fårö Kust, Gotland. Kulturgeografiska Institutionen. Uppsatser: Uppsala universitet, 44.
Kershaw, S., and Guo, L. (2001). Marine Notches in Coastal Cliffs: Indicators of Relative Sea-Level Change, Perachora Peninsula, Central Greece. Mar. Geol. 179, 213–228. doi:10.1016/s0025-3227(01)00218-3
Limber, P. W., and Murray, A. B. (2014). Sea Stack Formation and the Role of Abrasion on Beach-Mantled Headlands. Earth Surf. Process. Landforms 40, 559–568. doi:10.1002/esp.3667
Lindström, G. (1886). Om Postglaciala Sänkningar Af Gotland. G. F. I S F. 102, 251–281. doi:10.1080/11035898609444170
Linnaeus, C. (1745). Ölandska Och Gotländska Resa Pa Riksens Högloflige Ständers Befallning Förrättad Ahr 1741. . Stockholm: Natur och Kultur, 187. Partly reprinted as: Gotländska Resa flrrattad 1741.
Lorscheid, T., Felis, T., Stocchi, P., Obert, J. C., Scholz, D., and Rovere, A. (2017). Tides in the Last Interglacial: Insights from Notch Geometry and Palaeo Tidal Models in Bonaire, Netherland Antilles. Sci. Rep. 7, 16241. doi:10.1038/s41598-017-16285-6
Migoń, P., Duszyński, F., and Goudie, A. (2017). Rock Cities and Ruiniform Relief: Forms – Processes – Terminology. Earth Sci. Rev. 171, 78–104.
Migoń, P., and Duszyński, F. (2022). “Ruiniform Relief,” in Treatise on Geomorphology. Editor J. J. F. Shroder (Elsevier, Academic Press), 3, 408–431. doi:10.1016/j.earscirev.2017.05.012
Moura, D., Albardeiro, L., Veiga-Pires, C., Boski, T., and Tigano, E. (2006). Morphological Features and Processes in the Central Algarve Rocky Coast (South Portugal). Geomorphology 81 (3-4), 345–360. doi:10.1016/j.geomorph.2006.04.014
Munthe, H. (1921). Sveriges Raukar Jamte Exempel Pa Pseudoraukar. Sveriges Geol. Undersokn., Ser.C 303, 1–39.
Murchison, R. I. (1946a). On the Silurian and Associated Rocks in Dalecarlia, and on the Succession from Lower to Upper Silurian in Smoland, Öland and Gothland, and in Scania. Quart. J. Geol. Soc. Lond. 3, 1–46.
Murchison, R. I. (1946b). On the Superficial Detritus of Sweden, and on the Probable Causes Which Have Affected the Surface of the Rocks in the Central and Southern Portions of the Kingdom. Quart. J. Geol. Soc. Lond. 2, 349–381.
Niedzielski, T., Migoń, P., and Placek, A. (2009). A Minimum Sample Size Required from Schmidt Hammer Measurements. Earth Surf. Process. Landforms 34, 1713–1725. doi:10.1002/esp.1851
Philip, A. L. (1990). Ice-Pushed Boulders on the Shores of Gotland, Sweden. J. Coast. Res. 6, 661–676.
Pirazzoli, P. A., and Evelpidou, N. (2013). Tidal Notches: A Sea-Level Indicator of Uncertain Archival Trustworthiness. Palaeogeogr. Palaeoclimatol. Palaeoecol. 369, 377–384. doi:10.1016/j.palaeo.2012.11.004
Pirazzoli, P. A. (1986). “Marine Notches,” in Sea-Level Research. Editor O, van de Plassche (Dordrecht, Netherlands: Springer), 361–400. doi:10.1007/978-94-009-4215-8_12
Rosentau, A., Klemann, V., Bennike, O., Steffen, H., Wehr, J., Latinović, M., et al. (2021). A Holocene Relative Sea-Level Database for the Baltic Sea. Quat. Sci. Rev. 266, 107071. doi:10.1016/j.quascirev.2021.107071
Rosentau, A., Muru, M., Kriiska, A., Subetto, D. A., Vassiljev, J., Hang, T., et al. (2013). Stone Age Settlement and Holocene Shore Displacement in the Narva‐Luga Klint Bay Area, Eastern Gulf of Finland. Boreas 42, 912–931.
Rosentau, A., Nirgi, T., Muru, M., Bjursäter, S., Hang, T., Preusser, F., et al. (2020). Holocene Relative Shore Level Changes and Stone Age Hunter‐gatherers in Hiiumaa Island, Eastern Baltic Sea. Boreas 49, 783–798. doi:10.1111/bor.12452
Rovere, A., Raymo, M. E., Vacchi, M., Lorscheid, T., Stocchi, P., Gómez-Pujol, L., et al. (2016). The analysis of last interglacial (MIS 5e) relative sea-level indicators: Reconstructing Sea-level in a warmer world. Earth-Science Rev. 159, 404–427. doi:10.1016/j.earscirev.2016.06.006
Rudberg, S. (1967). “The Cliff Coast of Gotland and the Rate of Cliff Retreat,” in Geografiska Annaler. Series A, Physical Geography, 49, 283–298. doi:10.2307/520895
Sandström, O. (1998). Sediments and Stromatoporoid Morphotypes in Ludfordian (Upper Silurian) Reefal Sea Stacks on Gotland, Sweden. GFF 1204, 365–371. doi:10.1080/11035899801204365
Schneiderwind, S., Boulton, S. J., Papanikolaou, I., and Reicherter, K. (2017). Innovative Tidal Notch Detection Using TLS and Fuzzy Logic: Implications for Palaeo-Shorelines from Compressional (Crete) and Extensional (Gulf of Corinth) Tectonic Settings. Geomorphology 283, 189–200. doi:10.1016/j.geomorph.2017.01.028
Shepard, F. P., and Kuhn, G. G. (1983). History of Sea Arches and Remnant Stacks of La Jolla, California, and Their Bearing on Similar Features Elsewhere. Mar. Geol. 51, 139–161. doi:10.1016/0025-3227(83)90094-4
Sisma-Ventura, G., Sivan, D., Shtienberg, G., Bialik, O. M., Filin, S., and Greenbaum, N. (2017). Last Interglacial Sea Level High-Stand Deduced from Well-Preserved Abrasive Notches Exposed on the Galilee Coast of Northern Israel. Palaeogeography, Palaeoclimatology, Palaeoecology, 470, 1–10. doi:10.1016/j.palaeo.2017.01.008
Stephenson, W. J., and Finlayson, B. L. (2009). Measuring Erosion with the Micro-erosion Meter-Contributions to Understanding Landform Evolution. Earth-Science Rev. 95 (1-2), 53–62. doi:10.1016/j.earscirev.2009.03.006
Stephenson, W. J., Kirk, R. M., and Hemmingsen, M. A. (2019). Forty Three Years of Micro-erosion Meter Monitoring of Erosion Rates on Shore Platforms at Kaikōura Peninsula, South Island, New Zealand. Geomorphology 344, 1–9. doi:10.1016/j.geomorph.2019.07.012
Stroeven, A. P., Hättestrand, C., Kleman, J., Heyman, J., Fabel, D., Fredin, O., et al. (2016). Deglaciation of Fennoscandia. Quat. Sci. Rev. 147, 91–121. doi:10.1016/j.quascirev.2015.09.016
Strzelecki, M. C., Duszyński, F., Tyszkowski, S., Zbucki, Ł., and Kasprzak, M. (2020). Rauk -Forgotten Witness of Holocene Sea-Level Change and Development of Baltic Rocky Coastal Zone: A Pilot Geomorphological Study in Lergrav Raukar Field. J. Coast. Res. Special Issue 95, 659–663. doi:10.2112/si95-128.1
Svantesson, S-I. (2008). Beskrivning till Jordartskartan Gotland. Uppsala: Sveriges Geologiska Undersökning. SGU K4.
Svensson, N. O. (1989). Late Weichselian and Early Holocene Shore Displacement in the Central Baltic, Based on Stratigraphical and Morphological Records from Eastern Småland and Gotland, Sweden. Lundqua thesis.25
Swantesson, O. H. J., Gómez-Pujol, L., Cruslock, E. M., Fornós, J. J., and Balaguer, P. (2006). Processes and Patterns of Erosion and Downwearing on Micro-tidal Rock Coasts in Sweden and the Western Mediterranean. Z. für Geomorphol. N. F. Suppl. 144, 117–135.
Trenhaile, A. S. (2015). Coastal Notches: Their Morphology, Formation, and Function. Earth-Science Rev. 150, 285–304. doi:10.1016/j.earscirev.2015.08.003
Trenhaile, A. S., and Lakhan, V. C. (2011). Transverse Micro-erosion Meter Measurements Determining Minimum Sample Size. Geomorphology 134, 431–439. doi:10.1016/j.geomorph.2011.07.018
Trenhaile, A. S., Pepper, D. A., Trenhaile, R. W., and Dalimonte, M. (1998). Stacks and Notches at Hopewell Rocks, New Brunswick, Canada. Earth Surf. Process. Landforms 23, 975–988. doi:10.1002/(sici)1096-9837(1998110)23:11<975::aid-esp916>3.0.co;2-k
Wziatek, D., Vousdoukas, M. V., and Terefenko, P. (2011). Wave-cut Notches along the Algarve Coast, S. Portugal: Characteristics and Formation Mechanisms. J. Coast. Res. SI 64, 855–859.
Yu, S.-Y., Berglund, B. E., Sandgren, P., and Lambeck, K. (2007). Evidence for a Rapid Sea-Level Rise 7600 Years Ago. Geology 35, 891–894. doi:10.1130/g23859a.1
Keywords: rauk, rocky coast geomorphology, limestone, wave-cut notch, weathering, sea-level change, Baltic sea, Gotland
Citation: Strzelecki MC, Duszyński F, Tyszkowski S and Zbucki Ł (2022) Limestone Sea Stacks (Rauks) Record Past Sea Levels and Rocky Coast Evolution in the Baltic Sea (Gotland and Fårö Islands, Sweden). Front. Earth Sci. 10:895419. doi: 10.3389/feart.2022.895419
Received: 13 March 2022; Accepted: 11 May 2022;
Published: 16 June 2022.
Edited by:
Waldemar Kociuba, Maria Curie-Skłodowska University, PolandReviewed by:
Jan Rodzik, Maria Curie-Skłodowska University, PolandCopyright © 2022 Strzelecki, Duszyński, Tyszkowski and Zbucki. This is an open-access article distributed under the terms of the Creative Commons Attribution License (CC BY). The use, distribution or reproduction in other forums is permitted, provided the original author(s) and the copyright owner(s) are credited and that the original publication in this journal is cited, in accordance with accepted academic practice. No use, distribution or reproduction is permitted which does not comply with these terms.
*Correspondence: Mateusz C. Strzelecki, bWF0ZXVzei5zdHJ6ZWxlY2tpQHV3ci5lZHUucGw=
Disclaimer: All claims expressed in this article are solely those of the authors and do not necessarily represent those of their affiliated organizations, or those of the publisher, the editors and the reviewers. Any product that may be evaluated in this article or claim that may be made by its manufacturer is not guaranteed or endorsed by the publisher.
Research integrity at Frontiers
Learn more about the work of our research integrity team to safeguard the quality of each article we publish.