- 1College of Resources and Environment, Zhongkai University of Agriculture and Engineering, Guangzhou, China
- 2State Environmental Protection Key Laboratory of Water Environmental Simulation and Pollution Control, South China Institute of Environmental Sciences, Ministry of Ecology and Environment, Guangzhou, China
- 3Guangdong Research Center for Environmental Pollution Prevention and Control of Agricultural Producing Areas, Guangzhou, China
- 4College of Life Science and Technology, Jinan University, Guangzhou, China
- 5Northwest Institute of Eco-Environment and Resources, Chinese Academy of Sciences, Lanzhou, China
- 6School of Environment, Jinan University, Guangzhou, China
Geochemical techniques have been widely applied to study the generation and migration of hydrocarbons in sedimentary basins over the last several decades. Diagnostic biomarkers and stable carbon and hydrogen isotopes (such as δ13C and D) are generally used to identify the sources and thermal maturity of hydrocarbons and to reveal the accumulation process and mechanism of oil and gas reservoirs. However, some questions, such as secondary migration processes and pathways of oil and gas, and the relationship between groundwater flow and hydrocarbon transport, remain unclear and challenging. The low abundance and chemical inertness properties allow noble gases to serve as robust tools for tracing subsurface fluid flow. Additionally, they can be used for identifying and quantifying the role of the concomitant groundwater related to the generation and migration of hydrocarbons. This paper reviews the previous modeling work on using noble gases to study the fluid flow, flow paths, and gas/oil-water interactions in hydrocarbon systems. Noble gases from various sources can be readily identified due to their distinct isotopic and elemental signatures. Atmosphere-derived noble gases can be used to evaluate the amount of involved aquifer water associated with the hydrocarbon system and determine the groundwater migration paths and flow rates. Radiogenic noble gases accumulate over time, providing information about the subsurface fluid residence time. Questions concerning the specific trapping sites and mechanisms that affect heavy noble gas adsorption into organic sediments are still unresolved. Investigating the hydrocarbon generation, migration, and subsurface crustal fluid interactions in the hydrocarbon reservoirs can improve our understanding of noble gases as useful tracers in the subsurface environment and provide valuable geological evidence for the exploration and production of petroleum sources.
Introduction
Oil and gas have served as useful energy sources for the rising demand for energy worldwide (Burruss and Laughrey, 2010; Darrah et al., 2014; Wen et al., 2015). In recent years, the exploration targets progressively extend to the deep reservoirs (>4,500 m), the deep-sea reservoirs (>300 m), and the unconventional oil and gas reservoirs. The unconventional reservoirs include shale oil and gas reservoirs, tight oil and gas reservoirs, and coalbed methane reservoirs (Jia, 2020; Jin et al., 2021). Meanwhile, the academic community has widely recognized the application of geochemical techniques to study the generation and migration of hydrocarbons in sedimentary basins over the last several decades. For example, the analysis of biomarkers and stable carbon and hydrogen isotopes (such as δ13C and D) provides information about the types of source rock and thermal maturity, allowing further investigations on burial and thermal histories of sedimentary basins (Schoell, 1984; Zhu et al., 2007; Dai et al., 2012; Cheng et al., 2015; Volk and George, 2019). Besides, combining TOC and porosity data with burial and thermal history, the geological and geochemical models for two sets of shales were established to estimate free gas contents in organic-rich shales in the Weiyuan area of Sichuan Basin, China (Zhou et al., 2014). However, the multiple sources, complicated migration, accumulation processes, pathways, and loss of prior accumulated oil and gases complicated our understanding of oil and gas reservoirs. For example, it is difficult to determine the thermal maturities, oil types, and sources of the light oils/condensates because they barely contain high molecular weight compounds and the available geochemical information generally are limited (Peng and Jia, 2021). Therefore, efficient and robust methods and technologies are urgently required in the confront of hydrocarbon exploration and development (Larter et al., 1996; Fetter et al., 2019).
Noble gases have proven to be powerful tools to understand many geological processes better (e.g., liquid-gas interactions or gas migration) in the subsurface hydrocarbon systems due to their properties of low abundance and chemical inertness (Ozima and Podosek, 2002; Porcelli et al., 2002; Hunt et al., 2012). Noble gases consist of helium (He), neon (Ne), argon (Ar), krypton (Kr), and xenon (Xe). Noble gas production from natural radioactivity significantly modifies their isotopic signatures and provides fluid source and age information (Sano and Wakita, 1985; Smith, 1985; Kennedy et al., 1990). Similarly, small amounts of mantle-derived noble gases or atmosphere-derived noble gases in equilibrium with groundwater are readily distinguished due to their unique isotopic signatures. In addition, noble gases are unaffected by inorganic or organic chemical reactions. They are only sensitive to physical processes, such as multi-phase interaction (mixing, diffusion, and phase partitioning). These properties permit noble gases as ideal tracers for investigating the origin and evolution of subsurface fluids in oil and gas systems (Pinti and Marty, 1995; Hilton, 1996; Prinzhofer and Battani, 2003; Hunt et al., 2012; Roulleau et al., 2016; Karolytė et al., 2021; Liu et al., 2021).
This paper reviews the previous modeling work on the use of noble gases to study the fluid flow, flow paths, and gas/oil-water interactions in hydrocarbon systems. The investigation of hydrocarbon generation, migration, and subsurface crustal fluid interactions in the hydrocarbon reservoirs by using noble gases as tracers in subsurface environments contributes to the exploration and production of petroleum reservoirs.
Description of Noble Gases
Properties of Each Noble Gas Element
•He
Helium has two stable isotopes: 3He and 4He. 3He is the primordial helium isotope originating during planetary formation and is primarily associated with tectonic and volcanic activities during geological evolution (O’Nions and Oxburgh, 1988). In contrast, 4He is produced from the radioactive decay of U and Th in the convective mantle and crust. Atmospheric 3He and 4He can escape to space. 4He can be replenished from the crust.
Due to several orders of magnitude differences in 3He/4He ratios in the crust and mantle, 3He/4He ratios are used to distinguish various crustal and mantle sources. Samples from mid-ocean ridge basalts (MORB) have uniform 3He/4He ratios of ∼8Ra (where Ra is the air 3He/4He ratio of 1.4 × 10–6) (Graham, 2002), whereas the 3He/4He ratio in the crust is ∼0.02Ra (Graham, 2002). In addition, some ocean island basalts (OIB) samples from Hawaii and Iceland are characterized by significantly high abundance in 3He with the 3He/4He ratios as high as 30Ra (Graham, 2002), suggesting the presence of mantle plumes or thermal upwelling from the deep Earth. Measured 4He/20Ne ratios in samples relative to the air ratio (0.032) serve as a sensitive tracer of the degree of atmospheric contamination (Sano et al., 1985; Kipfer et al., 2002; Barry et al., 2013; Wen et al., 2018). If the measured 4He/20Ne ratios are significantly higher than the air ratio, we can assume that the air contamination in samples is negligible (Figure 1).
•Ne
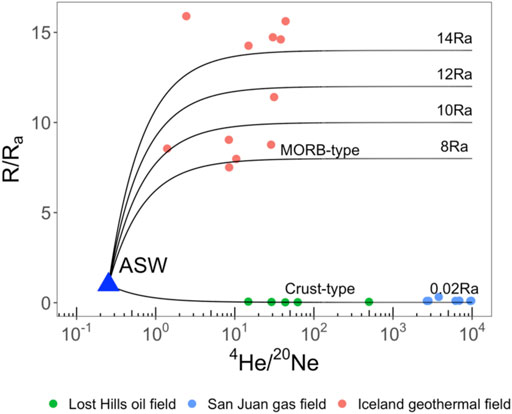
FIGURE 1. R/Ra ratio as a function of 4He/20Ne ratio for collected fluid samples. All data can be explained as a mixture of three components (Air, Crust, and Mantle). R is the measured 3He/4He ratio, and Ra is the 3He/4He ratio of air (1.4 × 10–6) (Mamyrin, 1970; Clarke et al., 1976). ASW represents the endmember of noble gases derived from Air Saturated Water. See details in Section “Three Different Sources.” Data sources: Lost Hills oil field (Barry et al., 2018), San Juan gas field (Zhou et al., 2005), and Iceland geothermal field (Byrne et al., 2021).
Neon has three stable isotopes: 20Ne, 21Ne, and 22Ne. 20Ne is produced by carbon-burning during stellar nucleosynthesis and was trapped by the Earth during accretion (Clayton, 2007), resulting in large amounts of 20Ne in the air. By contrast, the occurrence of 20Ne in the crust and mantle is negligible. 21Ne and 22Ne are both nucleogenic, but 22Ne has a relatively low abundance compared to 21Ne. Similar to He, atmospheric, mantle and crustal Ne component endmembers have been readily defined (20Ne/22Neair = 9.80, 21Ne/22Neair = 0.029, 20Ne/22Nemntl = 12.5, 21Ne/22Nemntl = 0.06, 20Ne/22Necrust = 0.30, 21Ne/22Necrust = 0.52) (Ballentine, 1997; Ballentine and Burnard, 2002). Therefore, it is possible to resolve the contribution of the atmospheric, mantle, and crustal Ne components (Figure 2).
•Ar
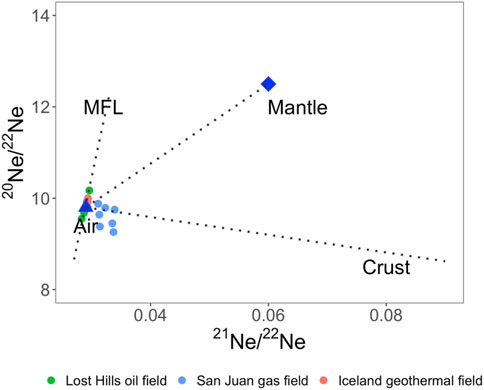
FIGURE 2. Neon three-isotope plot for selected noble gas studies in different subsurface systems. MFL represents a mass-dependent fractionation line, which is used for constraining mass-fractionation-related processes. Data sources: Lost Hills oil field (Barry et al., 2018), San Juan gas field (Zhou et al., 2005), and Iceland geothermal field (Byrne et al., 2021).
Argon has three stable isotopes: 40Ar, 38Ar, and 36Ar. 40Ar is produced by the radioactive decay of 40K in the crust, while 38Ar production is dominated by the α-decay of 35Cl and 37Cl in the crust. 36Ar production in the crust is relatively small compared to that in the air. The 40Ar/36Ar in the air is 298.6 (Lee et al., 2006). The non-atmosphere-derived excess 40Ar (40Ar*) can be calculated based on the atmosphere-derived 40Ar/36Ar ratio of 298.6 (Ballentine and Burnard, 2002) (Figure 3).
•Kr and Xe
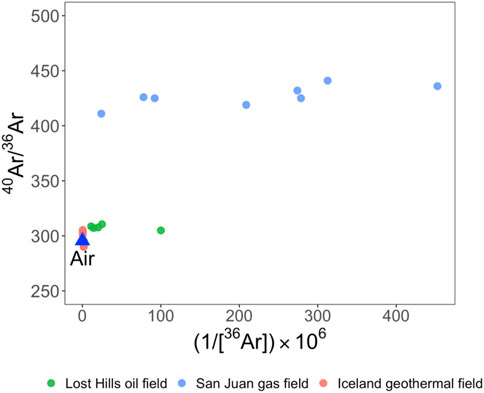
FIGURE 3. 40Ar/36Ar ratios vs. 1/36Ar. 40Ar/36Ar ratios in gas samples collected from San Juan Basin are significantly higher than the air value of 298.6 (Lee et al., 2006), suggesting the addition of a radiogenic Ar component. Data sources: Lost Hills oil field (Barry et al., 2018), San Juan gas field (Zhou et al., 2005), and Iceland geothermal field (Byrne et al., 2021).
Krypton and xenon have six and nine stable isotopes, respectively: 78Kr, 80Kr, 82Kr, 83Kr, 84Kr, 86Kr, 124Xe, 126Xe, 128Xe, 129Xe, 130Xe, 131Xe, 132Xe, 134Xe, and 136Xe. Among them, 84Kr and 132Xe are the most abundant isotopes in each group, accounting for 57.00% and 26.89% of total Kr and Xe abundances in the air, respectively.
Three Different Sources
Noble gases in crustal fluids are derived from three sources: the atmosphere, crust, and mantle (Figure 4). The distinct isotopic and elemental signatures enable noble gases from various sources to be readily identified (Ozima and Podosek, 2002; Porcelli et al., 2002; Prinzhofer, 2013).
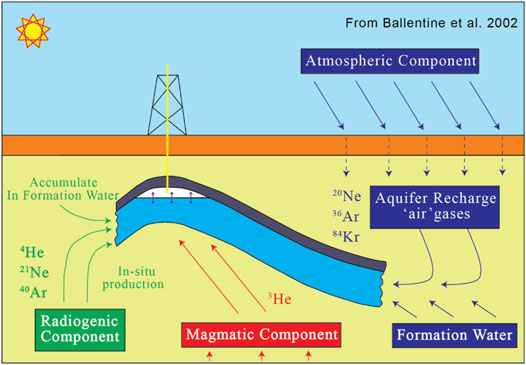
FIGURE 4. A schematic conceptual model showing three distinct sources of noble gas isotopes modified in hydrocarbon systems, redrawn from Ballentine et al. (2002).
Three main terrestrial reservoirs for noble gases are discussed as follows.
Air Saturated Water
Atmosphere-derived noble gases (e.g., 20Ne, 36Ar, and 84Kr) are introduced into the subsurface reservoirs by recharge water, which was previously in solubility equilibrium with the atmosphere (Air Saturated Water, ASW). Notably, these isotopes have no significant radiogenic production in the subsurface.
ASW noble gases are fractionated due to the noble gas solubility differences in the water. When the temperature is below 350 K, noble gas solubility in water generally increases with the mass, except that He and Ne have similar solubilities (Crovetto et al., 1982). The concentration of noble gases in the groundwater is associated with several factors, including partial pressure of the noble gases in the atmosphere, the temperature at which equilibration occurs, groundwater salinity, and recharge elevation (Kipfer et al., 2002). Given these quantities, ASW noble gas values can be readily calculated using Henry’s law. However, it should be noted that the values predicted by Henry’s law equilibration are commonly lower than empirical measurement data. This phenomenon is referred as gas surplus “excess air,” which most probably results from re-equilibration of entrapped air with water (Heaton and Vogel, 1981; Kipfer et al., 2002).
Among ASW noble gases, neon is most affected by “excess air” compared to heavier noble gases. Atmospheric noble gas isotopic ratios in water are not significantly affected by the equilibrium partitioning. They are the same as those isotopic ratios in the air because the isotopes of a given noble gas have the same properties with regards to solubility.
The ASW noble gas elemental and isotope compositions in crustal fluids are critical for understanding the interactions among various crustal subsurface fluids (groundwater, geothermal fluid, oil, and gas). For example, the depletion of atmospheric noble gases caused by subsurface boiling and steam separation processes during fluid ascent has been found in many tectonically active areas, such as Los Humeros Geothermal Field (LHGF), Mexico (Pinti et al., 2017), and Atlantis II Deep, Red Sea (Winckler et al., 2000). This indicates the occurrence of a thermal event, and it is possible to reconstruct the thermal histories in those active regions (Ma et al., 2009).
In a hydrocarbon system, groundwater and hydrocarbons were concomitantly existed and synchronously evolved (Cheng et al., 2017, 2019, 2022). Since the hydrocarbon phase is initially free of atmospheric noble gases, the measured atmospheric noble gases in oil and gas reservoirs are a result of interactions between groundwater and the hydrocarbon system (Bosch and Mazor, 1988; Hiyagon and Kennedy, 1992; Pinti and Marty, 1995; Zhou et al., 2005; Byrne et al., 2018a)). Consequently, the measured atmospheric noble gases can be used to evaluate the amount of involved aquifer water associated with the hydrocarbon system (Zhou et al., 2005; Barry et al., 2016), as well as to determine the groundwater migration paths and flow rates (Heilweil et al., 2012).
Furthermore, atmospheric noble gas ratios (e.g., 20Ne/36Ar) can also be used to identify the extent of air contamination (Barry et al., 2016). If atmospheric noble gas ratios in samples are close to those in the air, noble gas compositions are significantly affected by air contributions. Air contamination can occur during sampling, delivery, or storage procedures. Samples contaminated by air cannot be used to discuss the initial system situation (Ballentine et al., 2002; Barry et al., 2016).
Crust
The crust contains approximately 40% of the Earth’s radioactive elements (Vinogradov, 1988; Rudnick and Fountain, 1995). It serves as an important reservoir where radiogenic noble gases can be generated in addition to the atmosphere. The three most important radiogenic isotopes in the crust are 4He*, 21Ne*, and 40Ar*, where the crustal noble gases are denoted with a “*” notation. 4He*, 21Ne*, and 40Ar* have different origins. 4He* is produced by spontaneous α-decay of 235U, 238U, and 232Th. 40Ar* is generated due to the electronic capture of 40K (Ballentine and Burnard, 2002; Ozima and Podosek, 2002). 21Ne* is mainly produced by reactions between α particles (derived from U-Th decay) and O, Mg, and F nuclei in crustal rocks (Wetherill, 1954).
Crustal noble gases are initially produced within the minerals/rocks and then subsequently introduced into various subsurface crustal fluid systems. The crustal radiogenic noble gas concentrations and elemental ratios in fluids are dependent on several factors, including the contents of U, Th, and K in the source and/or host rocks, production rates and release properties of different noble gases, and degree of mixing and interaction among various crustal fluids (Zartman et al., 1961; Torgersen et al., 1989; Ballentine and O’nions, 1994; Ballentine et al., 1994; Pinti and Marty, 1995; Castro et al., 1998).
For instance, some researchers have found that 4He* is easier released than 40Ar* at low temperatures (Mamyrin and Tolstikhin, 1984; Elliot et al., 1993; Ballentine and Burnard, 2002), resulting in the higher 4He*/40Ar* ratios in low-temperature bacterial gases compared to those in high-temperature thermogenic gases. Therefore, the ratios of crustal radiogenic isotopes (e.g., 4He*/21Ne*, 4He*/40Ar*, and 21Ne*/40Ar*) are robust tools to trace the release temperatures (Torgersen and Clarke, 1985; Torgersen et al., 1989; Baxter et al., 2002).
Furthermore, radiogenic noble gas isotopes in hydrocarbon systems can be used to quantify the concentrations of 4He* in the active water phase, allowing the estimation of the subsurface fluid residence time (Zhou et al., 2005; Zhou and Ballentine, 2006; Schlegel et al., 2011; Barry et al., 2017).
In addition, the studies on the 4He/heat flux ratio in the crust reservoirs have been used to reconstruct the past thermal events and tectonic activities that occurred in regions during geological timescales (Stute et al., 1992; Castro and Goblet, 2005; Castro et al., 2007).
Mantle
The Earth’s mantle is another important reservoir for noble gas generation, accumulation, and transportation. The primordial mantle-derived noble gases were originally trapped during the early accretion of the Earth (Ozima and Podosek, 2002) and are characterized by distinct isotopic signatures. As described previously, helium is an ideal tracer of mantle contribution due to its low abundance in the air [5.24 × 10–6 cm3STP (4He)/cm3] and distinct crustal and mantle 3He/4He ratios. However, mantle-derived helium in samples with low 3He/4He values (0.1Ra > 3He/4He > 0.02Ra) cannot be quantified when local Li concentrations are high (small amounts of 3He can be produced associated with neutron interactions with Li) or there are cosmogenic He contributions. In these cases, high 20Ne/22Ne ratios, 21Ne/22Ne ratios, and 40Ar/36Ar ratios should also be considered to discriminate mantle contributions.
Mantle volatiles (e.g., 3He and CO2) can be used to investigate the geological evolution of subsurface fluid systems as they migrate through deep-seated faults and fractures and can be subsequently trapped in the shallow crust for substantial geological periods (Sherwood Lollar et al., 1997; Gilfillan et al., 2008; Zhou et al., 2012). For example, Sherwood Lollar et al. (1997) proposed that magmatic fluids have CO2/3He ratios ranging between 109 and 1010. CO2/3He ratios higher than this range would suggest negligible mantle contributions in those reservoirs. On the other hand, values below this range may be attributed to the partial loss of mantle CO2 and dilution possibly by CH4/N2. Three potential endmembers (mantle (M), limestone (L), and sediment (S)) contributing to the CO2 inventory proposed by Sano and Marty (1995) can distinguish the origin of CO2 from different components (Barry et al., 2013; Karolytė et al., 2019) (Figure 5).
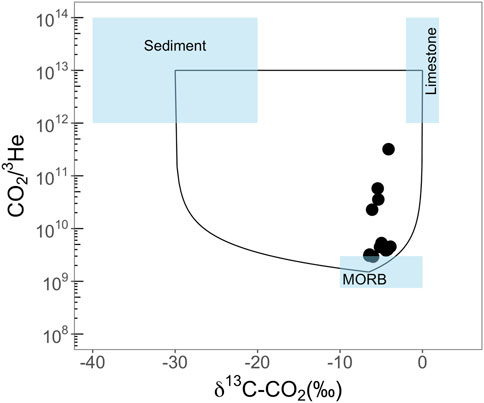
FIGURE 5. A plot of CO2/3He vs. δ13C(CO2). The endmember compositions are δ13C = −6.5‰ for mantle (M), 0‰ for limestone (L), and −30‰ for sediment (S) (relative to VPDB), and CO2/3He = 2 × 109 for M, 2 × 1013 for L and S, respectively (Sano and Marty, 1995). A 3-component mixing model between M–L–S can explain all data in the Rungwe Volcanic Province (Barry et al., 2013).
Methodology
Sample Collection
Because the abundances of noble gases are extremely low in the natural environment, any contact with air will significantly affect the measurement results. Therefore, careful sampling work is fundamental for subsequent successful analyses. To avoid air contamination, helium leak-tight and 50 cm long refrigeration grade 10 mm outer diameter internally polished copper tubes have been widely used for sample collection in noble gas communities (Kennedy et al., 1990; Ballentine et al., 1991; Zhou et al., 2005; Gilfillan et al., 2008). Stainless steel clamps are used to hold the copper tube during sampling. During the sampling procedure, well gases were allowed to flush through copper tubes for 10 min prior to sampling (Zhang et al., 2019; Li et al., 2020).
Sample Analysis
Noble gas abundances and isotopic ratios are generally determined using a noble gas mass spectrometer. In the laboratory, the sample copper tubes were mounted on an all-metal UHV (Ultra High Vacuum) system designed specifically to extract, purify, and separate noble gases. Most reactive gases (hydrocarbons, H2S, CO2, and CO, etc.) in the samples are decomposed and removed from the system using a combination of a titanium sponge and getters with no effect on the noble gases. After the removal of reactive gases, the mixture of noble gases (He, Ne, Ar, Kr, and Xe) was separated using a series of cryogenic traps prior to the inlet into the noble gas mass spec for analysis (Karolytė et al., 2019; Li et al., 2020, 2021; Byrne et al., 2021). A known amount of air standard and a procedural blank are analyzed following the same procedures as for the sample measurement prior to each sample run. The noble gas elemental abundances for each sample are calculated by normalizing to those of air standards after blank correction.
The Application of Noble Gases in Hydrocarbon Systems
Hydrocarbon systems are complex multiphase subsurface environments, consisting of a unit of active source-rock, all related hydrocarbon accumulations, and the geological features and processes that are necessary for the hydrocarbon accumulations to exist (Byrne et al., 2018b). Noble gas geochemical analyses provide a useful technique for constraining physical behavior occurring within hydrocarbon systems. This can be used to better characterize the subsurface fluid dynamics, such as processes during hydrocarbon generation, migration, and accumulation, oil/gas-water interactions, and residence time of fluids.
Determining the Genetic Fingerprint of Natural Gas Using Noble Gas Geochemistry
Four parameters, including C2+ concentration, carbon and hydrogen isotope variations in methane (δ13C(CH4), δD (CH4)), and carbon isotope variation in ethane δ13C(C2H6), are generally used for assessing the characteristics of natural gases from different sources (Schoell, 1983, 1988). Natural gases in a gas reservoir can be classified as biogenic gas with a δ13C(C1) less than −60‰ and thermogenic gas with a δ13C(C1) greater than −50‰ (Schoell, 1983, 1988). As thermal evolution proceeds, natural gases become more enriched in δ13C and a low amount of heavier hydrocarbons (C2+) (Schoell, 1983; Jenden et al., 1993; Hunt et al., 2012). When the organic matter reaches the high to over-mature stage, the natural gas is characterized by almost entirely methane (C1) with heavier hydrocarbons (C2+) less than 1% (Schoell, 1980, 1983; Tissot and Welte, 1984). However, traditional stable isotopes (C, H) can be affected by biological activities, chemical alterations, or redox reactions that may occur in the subsurface sometimes, which would complicate the investigation of subsurface hydrocarbon systems (Turner, 1982; Byrne et al., 2021). Noble gas isotopes serve as an additional constraint to complement existing techniques thanks to their chemical inertness. They are adequate to distinguish between different gas sources and identify possible physical processes over geological time (Hunt et al., 2012).
For example, Prinzhofer (2013) had shown that the two gas “families” had been differentiated by analyzing carbon isotope and noble gas isotope data. The 4He/40Ar* ratios are higher for biological gases because 4He is expelled more easily than 40Ar from the parent mineral lattice at low temperatures. As for ASW-derived noble gases, the higher 136Xe concentration in biological gases (lower 1/136Xe) suggests that biological gas underwent more interaction with the nearby aquifer water than thermogenic gas.
Noble Gases as Sensitive Tracers for Identifying Physical Processes Within Hydrocarbon Systems
Noble gases are sensitive to physical mechanisms within a hydrocarbon system during geological timescales. Groundwater has been considered important for hydrocarbon generation, migration, and accumulation. For example, groundwater can bring microbes into a previously sterile subsurface environment to generate biogenic hydrocarbons. In addition, groundwater can dissolve the hydrocarbons and carry them to trapping structures (Zhou et al., 2005; Schlegel et al., 2011).
To determine the extent of hydrocarbon and groundwater interactions, three possible processes that may take place during hydrocarbon-groundwater exchange have been summarized (Barry et al., 2016): (i) total degassing model, which assumes all noble gases have been lost from the water phase; (ii) equilibrium model, which means groundwater and hydrocarbon phase reached equilibrium at reservoir P, T, and salinity; and (iii) open and closed system gas-stripping models. There is no gas loss in the closed system model, while noble gases are accumulated in the reservoir due to groundwater gas stripping processes.
When the temperature is lower than 350 K, the noble gas solubility in water is positively correlated with the increase of noble gas masses (Crovetto et al., 1982). Variations in the noble gas elemental and isotopic ratios can explain the history of multi-component interactions (i.e., water–gas–oil) within a given hydrocarbon system (Byrne et al., 2018b).
Barry et al. (2016) showed that in a closed gas-stripping system, (20Ne/36Ar)Sample/(20Ne/36Ar)ASW values in the gas phase would start from above the ASW line and then keep going down towards the line until reaching the line (20Ne/36Ar)Sample = (20Ne/36Ar)ASW. In contrast, heavier noble gases (Kr, Xe) would start from below the ASW line and then keep going up towards the line until reaching the line (i/36Ar)Sample = (i/36Ar)ASW. However, in an open gas-stripping system, noble gas partitioning processes would continue after noble gas ratios in the gas phase are equal to their ASW values. As a result, gases are characterized by relatively lower 20Ne/36Ar ratios and higher 84Kr/36Ar and 132Xe/36Ar concerning their ASW ratios (Figure 6).
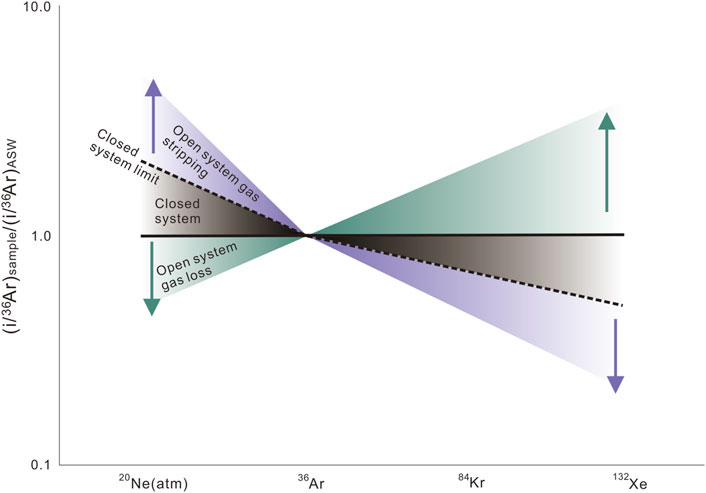
FIGURE 6. (i/36Ar)sample vs. (i/36Ar)ASW plot for 20Ne (atm), 84Kr, and 132Xe showing the expected noble gas ratios under different theoretical conditions (modified from Bosch and Mazor, (1988); Karolytė, (2019)).
To determine possible processes responsible for the water-oil/gas partition, an open system Rayleigh fractionation model below is most frequently used to interpret the noble gas data in many hydrocarbon systems. Rayleigh fractionation can be formulated by
where ([A]/[B])water is the noble gas elemental ratio in the water phase, ([A]/[B])o is the initial A/B ratio in the liquid (water) phase, P is the fraction of B remaining in the water phase, and α is the fractionation coefficient.
Two physical processes may occur in the hydrocarbon systems and can describe the partitioning of the noble gases between water and oil/gas phases in the subsurface. One is solubility-controlled fractionation; the other is diffusion-controlled fractionation.
The solubility-controlled fractionation coefficient is given for a gas/liquid system. α is defined as:
Dimensionless Henry’s constants Kd were derived from empiric equations (Crovetto et al., 1982). Fugacity coefficients Φ and liquid activity coefficients γ were calculated following.
Smith & Kennedy, (1983) and Ballentine et al. (2002).
The difference in diffusion properties in noble gases can cause preferential partitioning of noble gases from groundwater into the gas phase, as documented by Zhou et al. (2005). The mass-dependent noble gas fractionation process can generate the observed kinetic isotopic fractionation.
Assuming a gas i with mass Mi diffuses through a gas g with an average molecular mass Mg, the reduced mass Mi* is then expressed as follows (Marty, 1984; Jähne et al., 1987; Ballentine et al., 2002)
where Mi and Mg denote the mass of isotopes i and g, the average mass of the medium of the boundary layer where diffusion occurs. The value of Mg approaches infinity because of the large hydrogen bonds in the water phase (Zhou et al., 2005). Therefore, the reduced mass Mi* can be equal to Mi (Jähne et al., 1987; Lippmann et al., 2003). As the diffusion coefficients are proportional to the inverse of the square root of their reduced mass, the diffusion-controlled fractionation coefficient α is defined by:
where A and B denote noble gas isotopes, and MA and MB represent their respective masses.
For example, the isotopic fractionation of 20Ne/22Ne and 38Ar/36Ar ratios in the San Juan Basin suggested diffusion behavior caused by noble gas concentration gradients created during gas production (Zhou et al., 2005). An open system Rayleigh fractionation degassing model can interpret the relationship between water-derived 20Ne/36Ar and crustal 4He/40Ar* (Figure 7). However, the measured 20Ne concentrations are significantly lower than the values predicted by a Rayleigh fractionation-degassing model, suggesting gas that interacted with groundwater has possibly been diluted by desorbed coalbed methane. Combined with the local gas production histories, the amount of water associated with gas production can be derived at each well (Ballentine et al., 1991; Zhou et al., 2005).
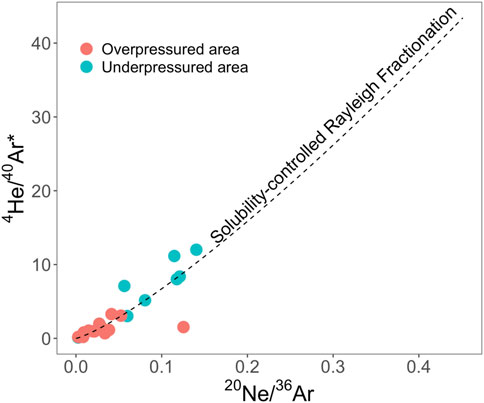
FIGURE 7. A plot of atmosphere-derived 20Ne/36Ar vs. crustal radiogenic 4He/40Ar*. The dashed line is a solubility-controlled Rayleigh fractionation modeling line for all gas samples in San Juan Basin (Zhou et al., 2005).
Quantitative Estimates of Gas-Water Ratio
As discussed previously, groundwater plays a significant role in establishing the noble gas systematics of hydrocarbon systems. Knowledge of the connected water volumes and the extent of water-gas exchange can help better understand subsurface fluid transport pathways and the subsequent involvement of water with reservoir processes (Robinson and Gluyas, 1992; Aplin et al., 1993; Ballentine et al., 1996). Additionally, groundwater behavior also has significant implications for hydrocarbon resource prediction.
When hydrocarbons interact with air-saturated formation water in the subsurface environment, noble gases are easily migrated into the hydrocarbon reservoirs due to the relatively low solubilities in the water phase. It is assumed that hydrocarbons are initially devoid of any atmospherically derived noble gases, which can be introduced into the hydrocarbon reservoir during gas-water exchange processes (Bosch and Mazor, 1988). Therefore, ASW noble gases can serve as useful tracers for assessing the extent of hydrocarbon–water interaction (e.g., volumetric gas/water (G/W) ratio). This helps to better understand hydrocarbon production, accumulation, migration pathways, and reservoir conditions (Bosch and Mazor, 1988; Ballentine et al., 1991; Barry et al., 2016, 2017).
With knowledge of the initial noble gas composition of the air-saturated water (determined by groundwater recharge conditions) and measured noble gas concentrations in the hydrocarbon phase, it is possible to constrain the processes (i.e., open vs. closed system) and quantify the extent of hydrocarbon-water interaction, and thus predict a volumetric gas/water (G/W) ratio. Barry et al. (2017) had suggested the relatively lower Vg/Vw from noble gas estimates than static geological forecasts occurs because the “geological” assessment of gas–water volumetric ratios only consider gas and water volumes in the local reservoir without the consideration of any water or gas present in deeper structures, migration pathways or source rock. Therefore, gas water ratio calculations using noble gases can provide more effective tools for constraining all formation water encountered in the hydrocarbon system, not only the reservoir interval.
Fluid Residence Time Determination
Knowledge of groundwater ages is vital for subsurface resources (such as hydrocarbon and groundwater) investigation and exploration. The accumulation of crustal radiogenic components (e.g., 4He, 21Ne, 40Ar) in the groundwater can provide useful information for groundwater-dating studies (Zhou and Ballentine, 2006; Schlegel et al., 2011; Wen et al., 2015; Byrne et al., 2018b). For example, 4He dating results in the Illinois Basin showed that older groundwater ages were associated with thermogenic methane. In comparison, younger ages were associated with microbially generated methane, suggesting that noble gas dating tools can constrain the onset and extent of microbial methane generation (Schlegel et al., 2011).
Fluid residence time can be estimated based on resolved radiogenic noble gases (e.g., 4He, 21Ne, 40Ar) that accumulate in subsurface fluids due to radioactive decay of uranium, thorium, and potassium over geologic timescales (Torgersen and Clarke, 1985; Castro et al., 1998; Ballentine and Burnard, 2002; Barry et al., 2017). Due to the low solubilities in the groundwater, noble gases will preferentially partition into the gas phase during water-gas interactions. Water-gas interactions also result in the migration of accumulated crustal noble gases and other dissolved atmospheric noble gases (e.g., 36Ar) from the water to the gas phase (Ballentine et al., 1991; Zhou and Ballentine, 2006). Therefore, crustal and atmosphere-derived noble gases allow us to estimate the initial crustal noble gas concentrations in the water phase prior to the phase separation and, thus, to acquire residence times for the associated water phase (Zhou et al., 2005; Zhou and Ballentine, 2006; Schlegel et al., 2011).
Radiogenic residence time in the fluid is calculated for two scenarios: (1) It is assumed that only in-situ radiogenic noble gases (4He, 21Ne*, 40Ar*) have accumulated in the reservoir, called a “closed” system. (2) In addition to in-situ production, an external crustal noble gas flux (upper crust + lower crust) has been introduced into the study area on a geological timescale. In this “open system,” it is assumed that the external crustal source is uniform across the sedimentary basin. Therefore, the amount of crustal radiogenic components in the groundwater system can be expressed as:
“Closed” system
“Open” system
where [Ci]H2O, [Ci]in situ production, and [Ci]external flux are the concentrations of the isotope i accumulated in the water, produced in situ and external flux (cm3STPg−1 H2O), respectively.
The in-situ production of isotope i can be expressed as:
where ρaquifer is the density of aquifer (g/cm3), Λ is release efficiency from mineral to water, which is assumed to be 1 (Torgersen, 1980; Torgersen and Clarke, 1985), φ is rock porosity, t is groundwater residence time (years), and Pi is the crustal production rate of isotope i in the reservoir rock (cm3STPg−1 yr−1).
As the radiogenic 4He, 21Ne*, and 40Ar* are produced by radioactive decay of uranium, thorium, and potassium over geologic timescales (Ballentine and Burnard, 2002), the production rate Pi can be calculated using the following equations (Craig and Lupton, 1976; Torgersen, 1980; Ballentine and Burnard, 2002)
where P4, P21, and P40 denote the production rates of 4He, 21Ne*, and 40Ar* in the rock, respectively. [U], [Th], and [K] are the U, Th, and K concentrations in the rock in ppm, respectively. [O] and [Mg] are O and Mg contents in the rock.
[Ci]external flux can be calculated by
where Fi represents the average external crustal radiogenic isotope flux (4He, 21Ne*, 40Ar*), ρcrust denotes the density of the crust, H denotes the thickness of crust (cm), and h denotes the thickness of aquifer (cm).
It has been shown that in most sedimentary basins, such as the Michigan Basin and San Juan Basin, the addition of external crust flux into the reservoir is present and must be considered in the calculation of groundwater ages (Zhou and Ballentine, 2006; Schlegel et al., 2011; Wen et al., 2015; Byrne et al., 2018a). Several orders of magnitude differences in these two sets of radiogenic ages strongly suggest that in-situ production is negligible compared to external flux additions. The significantly younger residence time than reservoir formation age indicates no significant preservation of formation water.
Heavy Noble Gas Enrichment
Oil and gas fields in contact with ancient basinal brine often show excesses of 84Kr/36Ar and 130Xe/36Ar relative to those values found in ASW. 130Xe/36Ar enrichment factors up to ∼200 relative to those ASW values have been observed in various systems, such as the Elk Hills oil field, California and Delaware Basins, and SE New Mexico (Torgersen and Kennedy, 1999; Kennedy et al., 2002; Holland et al., 2013).
Two possible mechanisms may be responsible for these observed heavy noble gas enrichments. The first is gas-oil interaction, and the second is the release of heavy noble gases initially trapped on source rocks. Bosch and Mazor (1988), Kharaka and Specht (1988), and Barry et al. (2016) have shown that the interactions between oil and gas can result in the relative abundance pattern of heavy noble gases enrichment. Heavy noble gas elemental fractionation patterns are much more extreme than light noble gas elemental fractionation patterns during the interaction with oil. However, it is still elusive to constrain the influence of oil on heavy noble gases. The second reason for heavy noble gas enrichments can be potentially attributed to the addition of sedimentary components from the source rock during geological evolution. Similar Kr-Xe enrichments have been observed in gas reservoirs in the North Sea (M. C. van Soest et al., 2000), natural gas samples from the Sleipner Vest gas field (Barry et al., 2016), and coalbed gases from the San Juan Basin (Zhou et al., 2005). They suggested that atmosphere-derived heavy noble gases (Kr and Xe) can be initially adsorbed and trapped in organic-rich sedimentary lithologies (e.g., shales and cherts), then escape into the fluid system and mixed with the hydrocarbon phases, providing an additional source of these noble gases. These “additional” components have an isotopic composition indistinguishable from the air but lead to a relative gas abundance pattern highly enriched in the heavy noble gases (Kr and Xe) (Torgersen and Kennedy, 1999; Kennedy et al., 2002). Questions concerning the specific trapping sites and mechanisms that affect heavy noble gas adsorption are still unresolved (Torgersen and Kennedy, 1999).
Conclusion
The application of noble gases in understanding hydrocarbon reservoirs has been increasing in recent years alongside improved measurement techniques, allowing the measurement of more noble gas isotopes with better accuracy and precision. However, difficulties in interpreting noble gas results still hinder the adoption of noble gas analysis as a routine geochemical analytical tool in hydrocarbon systems. For example, the noble gas-derived gas/water ratios are lower than static geological estimates. This is because the entire history of hydrocarbon-water interaction within the system has been considered during calculation rather than only considering the accumulation itself. Besides, the mechanisms that can absorb heavy noble gases into organic-rich sediment are yet to be resolved. Their explanation would benefit the investigation of adsorption versus free gas stored in porosity. Despite some limitations, noble gas isotope characterization has the obvious advantage that complications arising from chemical reactions can be confidently neglected and the assumption of ideal gas behavior is likely to be much closer to reality than for other gas species (Byrne et al., 2021). Noble gas isotopic composition and abundance can be used to place constraints on fluid sources, their mass balance, phase interactions, and the mechanisms of transport required to bring the fluid to the site of sampling. We therefore suggest that the use of noble gases may provide a robust tool for future research into subsurface hydrocarbon systems.
This paper summarizes noble gases studies on constraining origin, migration, and accumulation processes of crustal fluids in the hydrocarbon systems, providing further insights into underground fluid dynamics. The combination of noble gases and stable carbon isotope approaches can effectively differentiate distinct genetic groups of natural gases. Noble gas elemental and isotopic compositions can be used to construct physical models to describe the interaction between natural gas, oil, and groundwater, improving our understanding of the role of groundwater in different systems. These models can also be applied to estimate groundwater radiogenic noble gas concentrations that can be used to date hydrocarbon-associated water as well as the volume of water associated with hydrocarbon generation. An improved understanding of the applicability of noble gases as effective tracers in subsurface environments will aid scientific and regulatory evaluation of natural systems (e.g., hydrocarbon and groundwater) and subsurface exploration and development.
Author Contributions
YL, HFH, and CC conceived the study. HYH performed data interpretation. HFH provided funding and supervision. YL wrote the manuscript with assistance and editing from all co-authors.
Funding
This research has been funded by the China Postdoctoral Science Foundation (Grant No. 2021M701411) and Central Public-Interest Scientific Institution Basal Research Fund (Grant No. PM-zx703-202204-138).
Conflict of Interest
The authors declare that the research was conducted in the absence of any commercial or financial relationships that could be construed as a potential conflict of interest.
Publisher’s Note
All claims expressed in this article are solely those of the authors and do not necessarily represent those of their affiliated organizations, or those of the publisher, the editors and the reviewers. Any product that may be evaluated in this article, or claim that may be made by its manufacturer, is not guaranteed or endorsed by the publisher.
Acknowledgments
We thank three reviewers for their constructive comments on the manuscript. We thank Paul Mclachlan and Mounir Takriti for language correction.
References
Aplin, A. C., Warren, E. A., Grant, S. M., and Robinson, A. G. (1993). Mechanisms of Quartz Cementation in North Sea Reservoir Sandstones: Constraints from Fluid Compositions. Chapter 2: CONSTRAINTS ON DIAGENETIC PROCESSES.
Ballentine, C. J., Burgess, R., and Marty, B. (2002). 13. Tracing Fluid Origin, Transport and Interaction in the Crust. Rev. Mineral. Geochem. 47, 539–614. doi:10.2138/rmg.2002.47.1310.1515/9781501509056-015
Ballentine, C. J., and Burnard, P. G. (2002). 12. Production, Release and Transport of Noble Gases in the Continental Crust. Rev. Mineral. Geochem. 47, 481–538. doi:10.1515/9781501509056-014
Ballentine, C. J., Mazurek, M., and Gautschi, A. (1994). Thermal Constraints on Crustal Rare Gas Release and Migration: Evidence from Alpine Fluid Inclusions. Geochimica Cosmochimica Acta 58, 4333–4348. doi:10.1016/0016-7037(94)90337-9
Ballentine, C. J., O'Nions, R. K., and Coleman, M. L. (1996). A Magnus Opus: Helium, Neon, and Argon Isotopes in a North Sea Oilfield. Geochimica Cosmochimica Acta 60, 831–849. doi:10.1016/0016-7037(95)00439-4
Ballentine, C. J., O'Nions, R. K., Oxburgh, E. R., Horvath, F., and Deak, J. (1991). Rare Gas Constraints on Hydrocarbon Accumulation, Crustal Degassing and Groundwater Flow in the Pannonian Basin. Earth Planet. Sci. Lett. 105, 229–246. doi:10.1016/0012-821x(91)90133-3
Ballentine, C. J., and O’nions, R. K. (1994). The Use of Natural He, Ne and Ar Isotopes to Study Hydrocarbon-Related Fluid Provenance, Migration and Mass Balance in Sedimentary Basins. Geol. Soc. Lond. Spec. Publ. 78, 347–361. doi:10.1144/gsl.sp.1994.078.01.23
Ballentine, C. J. (1997). Resolving the Mantle He/Ne and Crustal 21Ne/22Ne in Well Gases. Earth Planet. Sci. Lett. 152, 233–249. doi:10.1016/S0012-821X(97)00142-8
Barry, P. H., Hilton, D. R., Fischer, T. P., de Moor, J. M., Mangasini, F., and Ramirez, C. (2013). Helium and Carbon Isotope Systematics of Cold “Mazuku” CO2 Vents and Hydrothermal Gases and Fluids from Rungwe Volcanic Province, Southern Tanzania. Chem. Geol. 339, 141–156. doi:10.1016/j.chemgeo.2012.07.003
Barry, P. H., Kulongoski, J. T., Landon, M. K., Tyne, R. L., Gillespie, J. M., Stephens, M. J., et al. (2018). Tracing Enhanced Oil Recovery Signatures in Casing Gases from the Lost Hills Oil Field Using Noble Gases. Earth Planet. Sci. Lett. 496, 57–67. doi:10.1016/j.epsl.2018.05.028
Barry, P. H., Lawson, M., Meurer, W. P., Danabalan, D., Byrne, D. J., Mabry, J. C., et al. (2017). Determining Fluid Migration and Isolation Times in Multiphase Crustal Domains Using Noble Gases. Geology 45, 775–778. doi:10.1130/G38900.1
Barry, P. H., Lawson, M., Meurer, W. P., Warr, O., Mabry, J. C., Byrne, D. J., et al. (2016). Noble Gases Solubility Models of Hydrocarbon Charge Mechanism in the Sleipner Vest Gas Field. Geochimica Cosmochimica Acta 194, 291–309. doi:10.1016/j.gca.2016.08.021
Baxter, E. F., DePaolo, D. J., and Renne, P. R. (2002). Spatially Correlated Anomalous 40Ar/39Ar “Age” Variations in Biotites about a Lithologic Contact Near Simplon Pass, Switzerland: a Mechanistic Explanation for Excess Ar. Geochimica Cosmochimica Acta 66, 1067–1083. doi:10.1016/S0016-7037(01)00828-6
Bosch, A., and Mazor, E. (1988). Natural Gas Association with Water and Oil as Depicted by Atmospheric Noble Gases: Case Studies from the Southeastern Mediterranean Coastal Plain. Earth Planet. Sci. Lett. 87, 338–346. doi:10.1016/0012-821X(88)90021-0
Burruss, R. C., and Laughrey, C. D. (2010). Carbon and Hydrogen Isotopic Reversals in Deep Basin Gas: Evidence for Limits to the Stability of Hydrocarbons. Org. Geochem. 41, 1285–1296. doi:10.1016/j.orggeochem.2010.09.008
Byrne, D. J., Barry, P. H., Lawson, M., and Ballentine, C. J. (2018a). Determining Gas Expulsion vs Retention during Hydrocarbon Generation in the Eagle Ford Shale Using Noble Gases. Geochimica Cosmochimica Acta 241, 240–254. doi:10.1016/j.gca.2018.08.042
Byrne, D. J., Barry, P. H., Lawson, M., and Ballentine, C. J. (2018b). Noble Gases in Conventional and Unconventional Petroleum Systems. Geol. Soc. Lond. Spec. Publ. 468, 127–149. doi:10.1144/sp468.5
Byrne, D. J., Broadley, M. W., Halldórsson, S. A., Ranta, E., Ricci, A., Tyne, R. L., et al. (2021). The Use of Noble Gas Isotopes to Trace Subsurface Boiling Temperatures in Icelandic Geothermal Systems. Earth Planet. Sci. Lett. 560, 116805. doi:10.1016/j.epsl.2021.116805
Castro, M. C., and Goblet, P. (2005). Calculation of Ground Water Ages-A Comparative Analysis. Ground Water 43, 368–380. doi:10.1111/j.1745-6584.2005.0046.x
Castro, M. C., Hall, C. M., Patriarche, D., Goblet, P., and Ellis, B. R. (2007). A New Noble Gas Paleoclimate Record in Texas - Basic Assumptions Revisited. Earth Planet. Sci. Lett. 257, 170–187. doi:10.1016/j.epsl.2007.02.030
Castro, M. C., Jambon, A., de Marsily, G., and Schlosser, P. (1998). Noble Gases as Natural Tracers of Water Circulation in the Paris Basin: 1. Measurements and Discussion of Their Origin and Mechanisms of Vertical Transport in the Basin. Water Resour. Res. 34, 2443–2466. doi:10.1029/98WR01956
Cheng, P., Tian, H., Xiao, X., Gai, H., Li, T., and Wang, X. (2017). Water Distribution in Overmature Organic-Rich Shales: Implications from Water Adsorption Experiments. Energy fuels. 31, 13120–13132. doi:10.1021/acs.energyfuels.7b01531
Cheng, P., Xiao, X. M., Gai, H. F., Li, T. F., Zhang, Y. Z., Huang, B. J., et al. (2015). Characteristics and Origin of Carbon Isotopes of N-Alkanes in Crude Oils from the Western Pearl River Mouth Basin, South China Sea. Mar. Petroleum Geol. 67, 217–229. doi:10.1016/j.marpetgeo.2015.05.028
Cheng, P., Xiao, X., Tian, H., Gai, H., Zhou, Q., Li, T., et al. (2022). Differences in the Distribution and Occurrence Phases of Pore Water in Various Nanopores of Marine-Terrestrial Transitional Shales in the Yangquan Area of the Northeast Qinshui Basin, China. Mar. Petroleum Geol. 137, 105510. doi:10.1016/j.marpetgeo.2021.105510
Cheng, P., Xiao, X., Wang, X., Sun, J., and Wei, Q. (2019). Evolution of Water Content in Organic-Rich Shales with Increasing Maturity and its Controlling Factors: Implications from a Pyrolysis Experiment on a Water-Saturated Shale Core Sample. Mar. Petroleum Geol. 109, 291–303. doi:10.1016/j.marpetgeo.2019.06.023
Clarke, W. B., Jenkins, W. J., and Top, Z. (1976). Determination of Tritium by Mass Spectrometric Measurement of 3He. Int. J. Appl. Radiat. Isotopes 27, 515–522. doi:10.1016/0020-708X(76)90082-X
Clayton, R. N. (2007). Isotopes: From Earth to the Solar System. Annu. Rev. Earth Planet. Sci. 35, 1–19. doi:10.1146/annurev.earth.35.092006.145059
Craig, H., and Lupton, J. E. (1976). Primordial Neon, Helium, and Hydrogen in Oceanic Basalts. Earth Planet. Sci. Lett. 31, 369–385. doi:10.1016/0012-821X(76)90118-7
Crovetto, R., Fernández‐Prini, R., and Japas, M. L. (1982). Solubilities of Inert Gases and Methane in H2O and in D2O in the Temperature Range of 300 to 600 K. J. Chem. Phys. 76, 1077–1086. doi:10.1063/1.443074
Dai, J., Ni, Y., and Zou, C. (2012). Stable Carbon and Hydrogen Isotopes of Natural Gases Sourced from the Xujiahe Formation in the Sichuan Basin, China. Org. Geochem. 43, 103–111. doi:10.1016/j.orggeochem.2011.10.006
Darrah, T. H., Vengosh, A., Jackson, R. B., Warner, N. R., and Poreda, R. J. (2014). Noble Gases Identify the Mechanisms of Fugitive Gas Contamination in Drinking-Water Wells Overlying the Marcellus and Barnett Shales. Proc. Natl. Acad. Sci. U.S.A. 111, 14076–14081. doi:10.1073/pnas.1322107111
Elliot, T., Ballentine, C. J., O'Nions, R. K., and Ricchiuto, T. (1993). Carbon, Helium, Neon and Argon Isotopes in a Po Basin (Northern Italy) Natural Gas Field. Chem. Geol. 106, 429–440. doi:10.1016/0009-2541(93)90042-H
Fetter, N., Blichert-Toft, J., Télouk, P., and Albarède, F. (2019). Extraction of Pb and Zn from Crude Oil for High-Precision Isotopic Analysis by MC-ICP-MS. Chem. Geol. 511, 112–122. doi:10.1016/j.chemgeo.2019.02.021
Gilfillan, S. M. V., Ballentine, C. J., Holland, G., Blagburn, D., Lollar, B. S., Stevens, S., et al. (2008). The Noble Gas Geochemistry of Natural CO2 Gas Reservoirs from the Colorado Plateau and Rocky Mountain Provinces, USA. Geochimica Cosmochimica Acta 72, 1174–1198. doi:10.1016/j.gca.2007.10.009
Graham, D. W. (2002). Noble Gas Isotope Geochemistry of Mid-ocean Ridge and Ocean Island Basalts: Characterization of Mantle Source Reservoirs. Rev. Mineralogy Geochem. 47, 247–317. doi:10.2138/rmg.2002.47.8
Heaton, T. H. E., and Vogel, J. C. (1981). "Excess Air" in Groundwater. J. Hydrology 50, 201–216. doi:10.1016/0022-1694(81)90070-6
Heilweil, V. M., Healy, R. W., and Harris, R. N. (2012). Noble Gases and Coupled Heat/fluid Flow Modeling for Evaluating Hydrogeologic Conditions of Volcanic Island Aquifers. J. Hydrology 464-465 (465), 309–327. doi:10.1016/j.jhydrol.2012.07.019
Hilton, D. R. (1996). The Helium and Carbon Isotope Systematics of a Continental Geothermal System: Results from Monitoring Studies at Long Valley Caldera (California, U.S.A.). Chem. Geol. 127, 269–295. doi:10.1016/0009-2541(95)00134-4
Hiyagon, H., and Kennedy, B. M. (1992). Noble Gases in CH4-Rich Gas Fields, Alberta, Canada. Geochimica Cosmochimica Acta 56, 1569–1589. doi:10.1016/0016-7037(92)90226-9
Holland, G., Lollar, B. S., Li, L., Lacrampe-Couloume, G., Slater, G. F., and Ballentine, C. J. (2013). Deep Fracture Fluids Isolated in the Crust since the Precambrian Era. Nature 497, 357–360. doi:10.1038/nature12127
Hunt, A. G., Darrah, T. H., and Poreda, R. J. (2012). Determining the Source and Genetic Fingerprint of Natural Gases Using Noble Gas Geochemistry: A Northern Appalachian Basin Case Study. Bulletin 96, 1785–1811. doi:10.1306/03161211093
Jähne, B., Heinz, G., and Dietrich, W. (1987). Measurement of the Diffusion Coefficients of Sparingly Soluble Gases in Water. J. Geophys. Res. 92, 10767–10776. doi:10.1029/JC092iC10p10767
Jenden, P. D., Drazan, D. J., and Kaplan, I. R. (1993). Mixing of Thermogenic Natural Gases in Northern Appalachian Basin. AAPG Bull. 77, 980–998. doi:10.1306/bdff8dbc-1718-11d7-8645000102c1865d
Jia, C. (2020). Development Challenges and Future Scientific and Technological Researches in China’s Petroleum Industry Upstream. Acta Pet. Sin. 41, 1445
Jin, Z., Wang, G., Liu, G., Gao, B., Liu, Q., Wang, H., et al. (2021). Research Progress and Key Scientific Issues of Continental Shale Oil in China. Acta Pet. Sin. 42, 821.
Karolytė, R., Barry, P. H., Hunt, A. G., Kulongoski, J. T., Tyne, R. L., Davis, T. A., et al. (2021). Noble Gas Signatures Constrain Oil-Field Water as the Carrier Phase of Hydrocarbons Occurring in Shallow Aquifers in the San Joaquin Basin, USA. Chem. Geol. 584, 120491. doi:10.1016/j.chemgeo.2021.120491
Karolytė, R., Johnson, G., Györe, D., Serno, S., Flude, S., Stuart, F. M., et al. (2019). Tracing the Migration of Mantle CO2 in Gas Fields and Mineral Water Springs in South-East Australia Using Noble Gas and Stable Isotopes. Geochimica Cosmochimica Acta 259, 109–128. doi:10.1016/j.gca.2019.06.002
Karolytė, R. (2019). Migration and Retention of CO₂ and Methane in the Otway Basin and South-East Australia: an Integrated Geochemical and Structural Analysis. Available at: https://era.ed.ac.uk/handle/1842/35538 (Accessed April 6, 2022).
Kennedy, B. M., Hiyagon, H., and Reynolds, J. H. (1990). Crustal Neon: a Striking Uniformity. Earth Planet. Sci. Lett. 98, 277–286. doi:10.1016/0012-821x(90)90030-2
Kennedy, B. M., Torgersen, T., and van Soest, M. C. (2002). Multiple Atmospheric Noble Gas Components in Hydrocarbon Reservoirs. Geochimica Cosmochimica Acta 66, 2807–2822. doi:10.1016/S0016-7037(02)00883-9
Kharaka, Y. K., and Specht, D. J. (1988). The Solubility of Noble Gases in Crude Oil at 25-100°C. Appl. Geochem. 3, 137–144. doi:10.1016/0883-2927(88)90001-7
Kipfer, R., Aeschbach-Hertig, W., Peeters, F., and Stute, M. (2002). 14. Noble Gases in Lakes and Ground Waters. Rev. Mineral. Geochem. 47, 615–700. doi:10.2138/rmg.2002.47.1410.1515/9781501509056-016
Larter, S. R., Bowler, B. F. J., Li, M., Chen, M., Brincat, D., Bennett, B., et al. (1996). Molecular Indicators of Secondary Oil Migration Distances. Nature 383, 593–597. doi:10.1038/383593a0
Lee, J.-Y., Marti, K., Severinghaus, J. P., Kawamura, K., Yoo, H.-S., Lee, J. B., et al. (2006). A Redetermination of the Isotopic Abundances of Atmospheric Ar. Geochimica Cosmochimica Acta 70, 4507–4512. doi:10.1016/j.gca.2006.06.1563
Li, Y., Qin, S., Wang, Y., Holland, G., and Zhou, Z. (2020). Tracing Interaction between Hydrocarbon and Groundwater Systems with Isotope Signatures Preserved in the Anyue Gas Field, Central Sichuan Basin, China. Geochimica Cosmochimica Acta 274, 261–285. doi:10.1016/j.gca.2020.01.039
Li, Y., Tootell, D., Holland, G., and Zhou, Z. (2021). Performance of the NGX High‐Resolution Multiple Collector Noble Gas Mass Spectrometer. Geochem Geophys Geosyst 22, e2021GC009997. doi:10.1029/2021GC009997
Lippmann, J., Stute, M., Torgersen, T., Moser, D. P., Hall, J. A., Lin, L., et al. (2003). Dating Ultra-deep Mine Waters with Noble Gases and 36Cl, Witwatersrand Basin, South Africa. Geochimica Cosmochimica Acta 67, 4597–4619. doi:10.1016/S0016-7037(03)00414-9
Liu, R., Wen, T., Amalberti, J., Zheng, J., Hao, F., and Jiang, D. (2021). The Dichotomy in Noble Gas Signatures Linked to Tectonic Deformation in Wufeng-Longmaxi Shale, Sichuan Basin. Chem. Geol. 581, 120412. doi:10.1016/j.chemgeo.2021.120412
Ma, L., Castro, M. C., and Hall, C. M. (2009). Atmospheric Noble Gas Signatures in Deep Michigan Basin Brines as Indicators of a Past Thermal Event. Earth Planet. Sci. Lett. 277, 137–147. doi:10.1016/j.epsl.2008.10.015
Mamyrin, B. A. (1970). Determination of the Isotopic Composition of Atmospheric Helium. Geochem. Int. 7, 498
Marty, B. (1984). On the Noble Gas Isotopic Fractionation in Naturally Occurring Gases. Geochem. J. 18, 157–162. doi:10.2343/geochemj.18.157
O’Nions, R. K., and Oxburgh, E. R. (1988). Helium, Volatile Fluxes and the Development of Continental Crust. Earth Planet. Sci. Lett. 90, 331–347. doi:10.1016/0012-821X(88)90134-3
Ozima, M., and Podosek, F. A. (2002). Noble Gas Geochemistry. Cambridge: Cambridge University Press.
Peng, P., and Jia, C. (2021). Evolution of Deep Source Rock and Resource Potential of Primary Light Oil and Condensate. Acta Pet. Sin. 42, 1543.
Pinti, D. L., Castro, M. C., Lopez-Hernandez, A., Han, G., Shouakar- Stash, O., Hall, C. M., et al. (2017). Fluid Circulation and Reservoir Conditions of the Los Humeros Geothermal Field (LHGF), Mexico, as Revealed by a Noble Gas Survey. J. Volcanol. Geotherm. Res. 333-334 (334), 104–115. doi:10.1016/j.jvolgeores.2017.01.015
Pinti, D. L., and Marty, B. (1995). Noble Gases in Crude Oils from the Paris Basin, France: Implications for the Origin of Fluids and Constraints on Oil-Water-Gas Interactions. Geochimica Cosmochimica Acta 59, 3389–3404. doi:10.1016/0016-7037(95)00213-J
Porcelli, D., Ballentine, C. J., and Wieler, R. (2002). An Overview of Noble Gas Geochemistry and Cosmochemistry. Rev. Mineralogy Geochem. 47, 1–19. doi:10.2138/rmg.2002.47.1
Prinzhofer, A., and Battani, A. (2003). Gas Isotopes Tracing: an Important Tool for Hydrocarbons Exploration. Oil Gas Sci. Technol. - Rev. IFP 58, 299–311. doi:10.2516/ogst:2003018
Prinzhofer, A. (2013). “Noble Gases in Oil and Gas Accumulations,” in The Noble Gases as Geochemical Tracers. Editor P. Burnard (Berlin, Heidelberg: Springer Berlin Heidelberg), 225–247. doi:10.1007/978-3-642-28836-4_9
Robinson, A., and Gluyas, J. (1992). Model Calculations of Loss of Porosity in Sandstones as a Result of Compaction and Quartz Cementation. Mar. Petroleum Geol. 9, 319–323. doi:10.1016/0264-8172(92)90080-X
Roulleau, E., Tardani, D., Sano, Y., Takahata, N., Vinet, N., Bravo, F., et al. (2016). New Insight from Noble Gas and Stable Isotopes of Geothermal/hydrothermal Fluids at Caviahue-Copahue Volcanic Complex: Boiling Steam Separation and Water-Rock Interaction at Shallow Depth. J. Volcanol. Geotherm. Res. 328, 70–83. doi:10.1016/j.jvolgeores.2016.10.007
Rudnick, R. L., and Fountain, D. M. (1995). Nature and Composition of the Continental Crust: A Lower Crustal Perspective. Rev. Geophys. 33, 267–309. doi:10.1029/95RG01302
Sano, Y., and Marty, B. (1995). Origin of Carbon in Fumarolic Gas from Island Arcs. Chem. Geol. 119, 265–274. doi:10.1016/0009-2541(94)00097-R
Sano, Y., Urabe, A., Wakita, H., Chiba, H., and Sakai, H. (1985). Chemical and Isotopic Compositions of Gases in Geothermal Fluids in Iceland. Geochem. J. 19, 135–148. doi:10.2343/geochemj.19.135
Sano, Y., and Wakita, H. (1985). Geographical Distribution of3He/4He Ratios in Japan: Implications for Arc Tectonics and Incipient Magmatism. J. Geophys. Res. 90, 8729–8741. doi:10.1029/JB090iB10p08729
Schlegel, M. E., Zhou, Z., McIntosh, J. C., Ballentine, C. J., and Person, M. A. (2011). Constraining the Timing of Microbial Methane Generation in an Organic-Rich Shale Using Noble Gases, Illinois Basin, USA. Chem. Geol. 287, 27–40. doi:10.1016/j.chemgeo.2011.04.019
Schoell, M. (1983). Genetic Characterization of Natural Gases. Am. Assoc. Petroleum Geol. Bull. 67, 2225–2238. doi:10.1306/03b5b4c5-16d1-11d7-8645000102c1865d
Schoell, M. (1988). Multiple Origins of Methane in the Earth. Chem. Geol. 71, 1–10. doi:10.1016/0009-2541(88)90101-5
Schoell, M. (1984). Recent Advances in Petroleum Isotope Geochemistry. Org. Geochem. 6, 645–663. doi:10.1016/0146-6380(84)90086-X
Schoell, M. (1980). The Hydrogen and Carbon Isotopic Composition of Methane from Natural Gases of Various Origins. Geochimica Cosmochimica Acta 44, 649–661. doi:10.1016/0016-7037(80)90155-6
Sherwood Lollar, B., Ballentine, C. J., and Onions, R. K. (1997). The Fate of Mantle-Derived Carbon in a Continental Sedimentary Basin: Integration of Relationships and Stable Isotope Signatures. Geochimica Cosmochimica Acta 61, 2295–2307. doi:10.1016/S0016-7037(97)00083-5
Smith, S. P., and Kennedy, B. M. (1983). The Solubility of Noble Gases in Water and in NaCl Brine. Geochimica Cosmochimica Acta 47, 503–515. doi:10.1016/0016-7037(83)90273-9
Smith, S. P. (1985). Noble Gas Solubility in Water at High Temperature. EOS, Trans. Am. Geophys Union 66, 397. doi:10.1029/eo066i037p00648
Stute, M., Schlosser, P., Clark, J. F., and Broecker, W. S. (1992). Paleotemperatures in the Southwestern United States Derived from Noble Gases in Ground Water. Science 256, 1000–1003. doi:10.1126/science.256.5059.1000
Tissot, B. P., and Welte, D. H. (1984). Petroleum Formation and Occurance. Berlin: Springer-Verlag. 49–215.
Torgersen, T., and Clarke, W. B. (1985). Helium Accumulation in Groundwater, I: An Evaluation of Sources and the Continental Flux of Crustal 4He in the Great Artesian Basin, Australia. Geochimica Cosmochimica Acta 49, 1211–1218. doi:10.1016/0016-7037(85)90011-0
Torgersen, T. (1980). Controls on Pore-Fluid Concentration of 4He and 222Rn and the Calculation of 4He/222Rn Ages. J. Geochem. Explor. 13, 57–75. doi:10.1016/0375-6742(80)90021-7
Torgersen, T., and Kennedy, B. M. (1999). Air-Xe Enrichments in Elk Hills Oil Field Gases: Role of Water in Migration and Storage. Earth Planet. Sci. Lett. 167, 239–253. doi:10.1016/S0012-821X(99)00021-7
Torgersen, T., Kennedy, B. M., Hiyagon, H., Chiou, K. Y., Reynolds, J. H., and Clarke, W. B. (1989). Argon Accumulation and the Crustal Degassing Flux of40Ar in the Great Artesian Basin, Australia. Earth Planet. Sci. Lett. 92, 43–56. doi:10.1016/0012-821X(89)90019-8
Turner, J. V. (1982). Kinetic Fractionation of Carbon-13 during Calcium Carbonate Precipitation. Geochimica Cosmochimica Acta 46, 1183–1191. doi:10.1016/0016-7037(82)90004-7
van Soest, M. C., Torgersen, T., and Kennedy, B. M. (2000). The Statfjord and Snorre Fields, Norwegian North Sea Oil Province. EOS, Trans. Am. Geophys. Union 81, 442.
Vinogradov, A. P. (1988). Regularities in Distributions of Chemical Elements inside Earth’s Crust. Sel. Pap. Problems Geochem. Cosmochem. 1 (1).
Volk, H., and George, S. C. (2019). Using Petroleum Inclusions to Trace Petroleum Systems - A Review. Org. Geochem. 129, 99–123. doi:10.1016/j.orggeochem.2019.01.012
Wen, T., Castro, M. C., Ellis, B. R., Hall, C. M., and Lohmann, K. C. (2015). Assessing Compositional Variability and Migration of Natural Gas in the Antrim Shale in the Michigan Basin Using Noble Gas Geochemistry. Chem. Geol. 417, 356–370. doi:10.1016/j.chemgeo.2015.10.029
Wen, T., Niu, X., Gonzales, M., Zheng, G., Li, Z., and Brantley, S. L. (2018). Big Groundwater Data Sets Reveal Possible Rare Contamination amid Otherwise Improved Water Quality for Some Analytes in a Region of Marcellus Shale Development. Environ. Sci. Technol. 52, 7149–7159. doi:10.1021/acs.est.8b01123
Wetherill, G. W. (1954). Variations in the Isotopic Abundances of Neon and Argon Extracted from Radioactive Minerals. Phys. Rev. 96, 679–683. doi:10.1103/PhysRev.96.679
Winckler, G., Kipfer, R., Aeschbach–Hertig, W., Botz, R., Schmidt, M., Schuler, S., et al. (2000). Sub Sea Floor Boiling of Red Sea Brines: New Indication from Noble Gas Data. Geochimica Cosmochimica Acta 64, 1567–1575. doi:10.1016/S0016-7037(99)00441-X
Zartman, R. E., Wasserburg, G. J., and Reynolds, J. H. (1961). Helium, Argon, and Carbon in Some Natural Gases. J. Geophys. Res. 66, 277–306. doi:10.1029/JZ066i001p00277
Zhang, W., Li, Y., Zhao, F., Han, W., Li, Y., Wang, Y., et al. (2019). Using Noble Gases to Trace Groundwater Evolution and Assess Helium Accumulation in Weihe Basin, Central China. Geochimica Cosmochimica Acta 251, 229–246. doi:10.1016/j.gca.2019.02.024
Zhou, Q., Xiao, X., Tian, H., and Pan, L. (2014). Modeling Free Gas Content of the Lower Paleozoic Shales in the Weiyuan Area of the Sichuan Basin, China. Mar. Petroleum Geol. 56, 87–96. doi:10.1016/j.marpetgeo.2014.04.001
Zhou, Z., and Ballentine, C. J. (2006). 4He Dating of Groundwater Associated with Hydrocarbon Reservoirs. Chem. Geol. 226, 309–327. doi:10.1016/j.chemgeo.2005.09.030
Zhou, Z., Ballentine, C. J., Kipfer, R., Schoell, M., and Thibodeaux, S. (2005). Noble Gas Tracing of Groundwater/coalbed Methane Interaction in the San Juan Basin, USA. Geochimica Cosmochimica Acta 69, 5413–5428. doi:10.1016/j.gca.2005.06.027
Zhou, Z., Ballentine, C. J., Schoell, M., and Stevens, S. H. (2012). Identifying and Quantifying Natural CO2 Sequestration Processes over Geological Timescales: The Jackson Dome CO2 Deposit, USA. Geochimica Cosmochimica Acta 86, 257–275. doi:10.1016/j.gca.2012.02.028
Keywords: hydrocarbon migration, noble gases, groundwater, fluid interaction, subsurface
Citation: Li Y, Cao C, Hu H and Huang H (2022) The Use of Noble Gases to Constrain Subsurface Fluid Dynamics in the Hydrocarbon Systems. Front. Earth Sci. 10:895312. doi: 10.3389/feart.2022.895312
Received: 13 March 2022; Accepted: 06 May 2022;
Published: 15 June 2022.
Edited by:
Peng Cheng, Guangzhou Institute of Geochemistry (CAS), ChinaReviewed by:
Liao Shili, Ministry of Natural Resources, ChinaChengsheng Chen, Guangzhou Institute of Geochemistry (CAS), China
Naizhong Zhang, Tokyo Institute of Technology, Japan
Copyright © 2022 Li, Cao, Hu and Huang. This is an open-access article distributed under the terms of the Creative Commons Attribution License (CC BY). The use, distribution or reproduction in other forums is permitted, provided the original author(s) and the copyright owner(s) are credited and that the original publication in this journal is cited, in accordance with accepted academic practice. No use, distribution or reproduction is permitted which does not comply with these terms.
*Correspondence: Huanfang Huang, aHVhbmdodWFuZmFuZ0BzY2llcy5vcmc=