- 1IRD-Sorbonne University-CNRs-MNHN, UMR LOCEAN-IPSL, Paris, France
- 2HIMB, SOEST-University of Hawaii, Kaneohe, HI, United States
- 3UMR ENTROPIE (Université de La Réunion-IRD-CNRS-Ifremer-Université de la Nouvelle Calédonie)—Labex Corail, Université de La Réunion, Réunion, France
Bioerosion, resulting from microbioerosion or biogenic dissolution, macrobioerosion and grazing, is one the main processes involved in reef carbonate budget and functioning. On healthy reefs, most of the produced carbonates are preserved and accumulate. But in the context of global change, reefs are increasingly degraded as environmental factors such as ocean warming and acidification affect negatively reef accretion and positively bioerosion processes. The recent 2019 SROCC report suggests that if CO2 emissions in the atmosphere are not drastically reduced rapidly, 70%–99% of coral reefs will disappear by 2,100. However, to improve projections of coral reef evolution, it is important to better understand dynamics of bioerosion processes. Among those processes, it was shown recently that bioeroding microflora which actively colonize and dissolve experimental coral blocks, release significant amount of alkalinity in seawater both by day and at night under controlled conditions. It was also shown that this alkalinity production is enhanced under ocean acidification conditions (saturation state of aragonite comprised between 2 and 3.5) suggesting that reef carbonate accumulation will be even more limited in the future. To better understand the conditions of production of alkalinity in seawater by boring microflora and its possible consequences on reef resilience, we conducted a series of experiments with natural rubble maintained under natural or artificial light, and various saturation states of aragonite. We show here that biogenic dissolution of natural reef rubble colonized by microboring communities dominated by the chlorophyte Ostreobium sp., and thus the production of alkalinity in seawater, can occur under a large range of saturation states of aragonite, from 2 to 6.4 under daylight and that this production is directly correlated to the photosynthetic activity of microboring communities. We then discuss the possible implications of such paradoxical activities on reef resilience.
1 Introduction
Due to the exponentially rising atmospheric carbon dioxide partial pressure (pCO2) and its partial absorption by the ocean (−30%, Sabine et al., 2004), the saturation state of surface seawater (Ω) with respect to calcium carbonate minerals (CaCO3) will decrease together with seawater pH by the end of the century (−0.2 to −0.4 pH unit depending on the IPCC scenario and seasonality; Orr et al., 2005; Bindoff et al., 2019). Such decrease will greatly impact negatively major calcifying organisms and some coastal carbonate ecosystems (Guinotte and Fabry, 2008; Fabricius et al., 2011; Agostini et al., 2018) while enhancing carbonate dissolution (Andersson et al., 2007; Andersson et al., 2008; Krumins et al., 2013; Stubler and Peterson, 2016; Schönberg et al., 2017).
Cyronak et al. (2014) showed that the average pCO2 may have increased faster in coral reefs than in the atmosphere and the open ocean over the past 2 decades (−3.5-fold, i.e., + 6.6 ± 1.4 µatm.y−1) due to additional local disturbances resulting from human activities (e.g., eutrophication), thus putting these ecosystems even more at risk under ocean acidification. Notwithstanding the fact that reef ecosystems will greatly be degraded by the end of the century due to this factor but also ocean warming and local disturbances (e.g., storm impacts and rising runoffs), the latest SROCC report (Bindoff et al., 2019) highlighted the lack of information regarding the sensitivity and adaptive capacity of coral reef organisms and ecosystems to climate change impacts inducing bias in projections.
While several studies highlighted the negative effect of ocean acidification (combined or not with other factors) on growth, abundance and calcification rates of the main reef framebuilders, i.e., corals and calcifying algae under more or less controlled conditions and over short term (e.g., Langdon and Atkinson, 2005; Kuffner et al., 2008; Pandolfi et al., 2011; Comeau et al., 2014; Johnson et al., 2014), at the global reef scale the relationship between net reef community calcification and aragonite saturation state varies greatly from one area to the next (e.g., Shamberger et al., 2011; Falter et al., 2012; Shaw et al., 2012; Page et al., 2016). Such variations may be explained by local biological adaptations of calcifiers to the natural variability of their environmental conditions (Vargas et al., 2022) and/or variability of carbonate dissolution processes in both reef sediments and hard substrates (Pandolfi et al., 2011; see summary Table 2 in Tribollet et al., 2019); reef budget depending on the equilibrium between reef calcification and dissolution. Eyre et al. (2014) showed that despite possible adaptations of calcifiers, changes in reef dissolution are more rapid than changes in reef calcification under ocean acidification, enhancing net reef dissolution (see also Andersson and Gledhill 2013).
Reef dissolution comprises the thermodynamically driven carbonate dissolution (seawater chemistry with Ω < 1), the bacteria driven dissolution (release of CO2 through organic matter remineralization) and the biogenic carbonate dissolution driven mainly by boring microflora and sponges (Tribollet and Golubic, 2011; Schönberg et al., 2017). Among those processes, the spatial and temporal variability of biogenic dissolution rates due to microboring flora remains poorly known (Carreiro-Silva et al., 2005; Tribollet, 2008b; Grange et al., 2015; Schönberg et al., 2017), most probably because this process which occurs at the microscale is considered as negligible, or is ignored and/or too difficult to study. However, this process may explain an important part of the reef dissolution variability (Andersson and Gledhill 2013). Tribollet et al. (2019) showed indeed that biogenic dissolution due to boring microflora dissolve up to 20% of carbonates deposited by reef calcifiers under ambient conditions (dissolution estimated on average at 11 mmol CaCO3.m−2 of reef.d−1 in both hard and sediment substrates via microscopy techniques). They also highlighted that mature microboring communities dominated by the chlorophyte Ostreobium sp. colonizing experimental blocks of dead coral skeleton can produce consequently, significant amount of seawater alkalinity both under low controlled light intensity (during photosynthesis) and at night, at ambient saturation states (at ΩArag 3–3.5). They finally showed that this production is enhanced by 50% under ocean acidification conditions (at ΩArag = 2), confirming trends obtained for biogenic dissolution rates in dead corals measured by microscopy or buoyant weight by Tribollet et al. (2009), Reyes-Nivia et al. (2013) and Enochs et al. (2016). Meanwhile, Tribollet et al. (2019) suggested a potential negative feedback of this biogenic dissolution on ocean acidification; a feedback that could benefit to reef calcifiers and ecosystems, at least at the microscale. Thus, to better understand dynamics of the biogenic dissolution process resulting from microborers’ metabolic activity and possible implications on reef resilience, it is necessary to further investigate the relationship between the production of seawater alkalinity by microborers and the saturation state of aragonite under natural conditions and at various spatial (from substrate to ecosystem scale) and temporal scales (day, season, year).
Here we studied the capacity of natural mature microboring communities dominated by Ostreobium sp. at dissolving natural coral rubble and consequently at producing seawater alkalinity under different light regimes and natural variations of aragonite saturation states. Two experiments were carried out, one in an indoor flume under controlled light and the other one outdoor under natural light, in Hawaii (Kaneohe Bay). Seawater DIC was not artificially modified as we did not test the effects of ocean acidification on the metabolism of natural microboring communities; this being reported elsewhere (see Tribollet et al., 2009; Reyes-Nivia et al., 2013; Enochs et al., 2016; Tribollet et al., 2019).
2 Material and methods
2.1 Experimental design
Two distinct experiments were carried out at the Hawaii Institute of Marine Biology (HIMB, HI, United States) in an indoor flume and in an outdoor tank to see the possible effects of light regimes and evolving aragonite saturation state on biogenic dissolution of carbonates by boring microflora, and thus on their production of seawater alkalinity. Substrates used in this study were rubbles mainly composed of Porites lobata scraps as one colony of this massive coral was used previously to cut experimental blocks for another bioerosion study (Figure 1A; see also Tribollet et al., 2006; Tribollet et al., 2009). The rest was a mix of P. compressa, Pocillopora meandrina and Montipora capitata rubble, which are commonly found in Kaneohe Bay, Ohau, Hawaii. Those rubbles were colonized by natural communities of microborers in the shallow fringing reef near the HIMB laboratory (<1 m depth) during at least 1 year in order to work with mature communities dominated by Ostreobium sp. (Chazottes et al., 1995; Gektidis, 1999; Tribollet, 2008b; Grange et al., 2015). The metabolism of colonized rubbles was then estimated in the two different settings by following the evolution of seawater pH and total alkalinity (see description below).
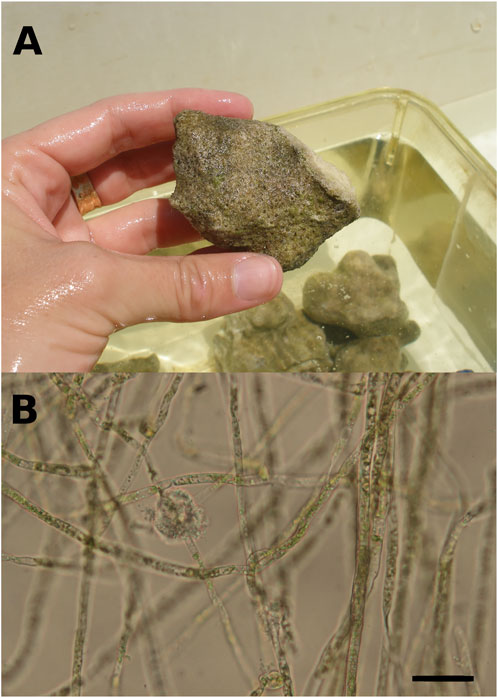
FIGURE 1. (A). Brushed coral rubble originating from a massive Porites colony, colonized by at least a 12 months-old microboring community. Greenish patches of phototrophic endoliths dominated the microboring community. (B). Filaments of the bioeroding chlorophyte Ostreobium sp. observed under light microscopy. Chloroplasts are visible inside siphons (filaments without cross-walls) of the chlorophyte. Scale bar = 20 µm.
2.1.1 Indoor flume experiment under controlled light conditions
To study the impact of microborers’ metabolism on the carbonate system under saturating light regime (Vooren, 1981; Tribollet et al., 2006) and natural seawater conditions (i.e., seawater from Kaneohe Bay with ΩArag varying between 3 and 4 during the experiment), we randomly collected natural rubble colonized by mature microboring communities near the HIMB laboratory to form a composite set of rubbles representative of those present on the natural reef flat. Rubbles were brushed gently to remove epiliths such as turfs, crustose coralline algae and serpulids, and were then affixed on a PVC plate with epoxy to obtain a planar surface area of 0.33 m2. The top surfaces of rubbles were positioned relative to light in the same way as they were on the reef. The plate was quickly (≈15 min) transported into the indoor flume facility at HIMB (see description in Falter et al., 2006) and kept in flow-through seawater (sand filtered seawater from Kaneohe Bay) during 1 day to allow acclimation. The flume was then closed and had a total volume of seawater of 1.1 m3. During 1 week, an oscillatory flow was maintained, as well as a constant temperature (25 ±1°C) and a 12:12 h light to dark photoperiod (between 7 a.m. and 7 p.m. with a constant light intensity of 350 µmol photons.m−2.s−1 delivered by two Solar simulator Arrays, Tailored lighting). The delivered light intensity was similar to that used in a previous experiment carried out at Biosphere (Tribollet et al., 2009). Seawater samples were taken every day at 7 a.m. and 7 p.m. for analysis of total alkalinity (AT) and pH on the total hydrogen ion concentration scale (pHT). After that first set of measurements, the flume was flushed to renew seawater to maintain natural reef conditions, and rubbles were brushed again to avoid any epilithic overgrowth. AT and pHT variations were then again surveyed during another week (2nd set of measurements). Slight differences in the carbonate system parameters between the initial conditions of the two sets of measurements (Table 1) were due to the time of the day the flume was filled, and therefore to the natural variation of seawater chemistry on the reef flat (1st set in the morning; 2nd set in the afternoon).
2.1.2 Outdoor incubations under natural daylight
To determine if biogenic dissolution by microborers could occur at high ΩArag values during daylight, i.e., when photosynthesis occurs and thus when carbonate dissolution is thermodynamically difficult, short incubations with another composite set of natural rubbles similar to those used in the indoor flume experiment and representative of the reef flat (Figure 1A), were performed in an outdoor tank. This time, the studied rubble planar surface area was 0.067 m2. Brushed rubbles were put in a 18 L tank with a marine seawater bilge pump (12 V, 250 rpm) to insure water motion, under natural daylight. The set of rubbles was incubated 3 times, 4 days apart between 9 a.m. and 6 p.m., allowing the microboring community to evolve naturally as in the natural reef flat. Rubbles were indeed kept in a flow-through seawater pumped on the reef flat (unfiltered) between two incubations. They were also gently brushed to remove epiliths' overgrowth, and to allow recruitement of new borers and epiliths, as well as growth of existing microborers up to their new depth of compensation (see Schneider and Le Campion-Alsumard, 1999; Tribollet, 2008a). During the experiments, mean light intensity measured at the weather station of HIMB varied between 750 and 1300 µmol photons.m−2.s−1 depending on the day of incubation. The 18 L tank with rubbles was partially submerged in flow-through seawater in an outdoor flume to maintain a constant temperature (27 ±1°C). To ensure that ΩArag would not reach very high and unrealistic values in the tank (compare to coral reef conditions), a few ml of HCl (0.1 N) were added prior each of the 3 incubations to adjust an initial ΩArag comprised between 1.8 and 2.1 (ΩArag > 1 to avoid dissolution thermodynamically driven). Incubations lasted for 5–9 h and AT and pHT were sampled every hour (see initial conditions in Table 1). AT and pHT of a control tank with just un-acidified seawater were also measured at the beginning and end of every working day to ensure that phytoplanktonic activity did not impact AT and/or dissolved inorganic carbon concentration (DIC) during rubble incubations.
2.2 Seawater chemistry analysis
After collection, saturated mercuric chloride was added to seawater samples to prevent biological activity (Dickson et al., 2007). The potentiometric determination of pHT was realized using Tris/HCl and 2-aminopyridine/HCl buffers in synthetic seawater to calibrate the Ross combination electrode (ORION 81-03) using a Thermo Scientific Orion 2 star Plus pH meter at room temperature. Temperature variation did not exceed 0.1°C during one set of measurements.
Potentiometric titration of AT was carried out using 0.01 mol.L−1 HCl in NaCl to approximate the ionic strength of seawater after filtration of each AT sample through Whatman GF/F (to remove possible suspended carbonate particles). The acid titrant concentration was determined each day of AT measurements using Certified Reference Material from A. Dickson’s laboratory (Scripps Institution of Oceanography). A manual Gran titration was performed with a precision of ± 2 µequiv.l−1.
Measured pHT and AT allowed calcultating the other CO2 chemistry parameters with the software CO2SYS (Pierrot et al., 2006). The same parameters for seawater salinity, phosphates, silicates and seawater density were used as in Tribollet et al. (2019)’s study.
2.3 Net dissolution and organic carbon metabolism calculation
Net dissolution rates (G), expressed in mmol CaCO3.m−2.h−1 were calculated according to the following Eq. 1:
where G is positive when net dissolution occurs,
Net organic carbon metabolism (NP), expressed in mmol C.m−2.h−1 was calculated according to the following Eq. 2:
Where NP is the rate of net photosynthesis during daylight (negative value, although for simplicity it will be presented as positive in the results) or the rate of dark respiration at night (positive value) and ∆DIC is the difference in DIC between two measurements (final DIC minus initial DIC). The other symbols are as defined in Eq. 1.
For the indoor flume experiment, daily net dissolution was estimated from the slope of the AT vs. time relationship. Daily excess production (the difference between gross production and daily respiration) was calculated from the slopes of the AT and DIC vs. time relationships. Other calculations are explained in the Results section.
For the outdoor experiment in a 18 L tank (seawater surface area in contact with the atmosphere estimated to be <0.1 m2), NP were estimated without taking into account potential fluxes of CO2 between air and the tank seawater. Because we did not observe much turbulence at the tank surface due to the bilge pump or wind during our incubations, we assume that CO2 diffusion was probably limited. However we cannot exclude that NP rates were slightly overestimated at the start of incubations (due to an evasion of CO2 from the tank to the atmosphere), or underestimated at the end of incubations (uptake of CO2 atmospheric by the tank seawater).
2.4 Statistical analysis
Statistical tests were performed using STATISTICA 7.1 (Statsoft) and R version 2.15.1 (R Foundation for Statistical Computing). First, an analysis of covariance (ANCOVA) was performed to compare the slopes of the AT vs. time regression lines between the two sets of measurements performed in the indoor flume (1st experiment). Regression lines were compared by studying the interaction of the categorical variable (i.e., 1st or 2nd set of measurements) with time. Beforehand, a Robust Jarque Bera test and a Levene test were used to test for normality and homogeneity of variances, respectively. Second, DIC concentration vs. AT relationships were compared between the two sets of measurements using the (S)MATR software (version 1.0 2003, http://www.bio.mq.edu.au/ecology/SMATR) designed for making comparisons among lines fitted according to the standardized major axis method (SMA). In both cases, the slopes were not significantly different between the 2 sets of measurements in the indoor flume (p > 0.05), therefore a common slope was calculated. The common slope of the DIC concentration vs. AT relationship was then compared to 0.5 using (S) MATR. Means are reported ±SE.
3 Results
Observations of rubbles showed that epiliths colonizing substrate surfaces were dominated by green short turfs. Rare crustose coralline algae and boring polychaetes (1 or 2) were observed on some rubbles, as well as rare traces of grazing. Observations under light microscopy revealed that rubbles were colonized by mature communities of microborers dominated by the chlorophyte of the genus Ostreobium (Figure 1B; Chazottes et al., 1995; Grange et al., 2015). Once epiliths were gently brushed off, rubbles showed an intense yellowish-greenish colour indicative of the presence of a majority of phototrophic endoliths inside dead coral skeletons (Figure 1A).
3.1 Variability of rubbles metabolism under constant light (indoor flume experiment)
In the indoor flume experiment ran with rubbles exposed to constant light intensity (350 µmol photons.m−2.s−1 with a 12:12 light to dark photoperiod), temperature (−25.5°C) and water flow, total alkalinity of seawater (AT) increased linearly with time (Figure 2A; R2 = 0.99 and R2 = 0.98 before and after water in the flume was renewed, respectively; p < 0.0001). The slope of AT vs. time relationship remained the same when water in the flume was renewed after a few days of experiment, resulting in a constant mean net dissolution rate of 35.5 ± 0.2 mmol CaCO3.m−2.d−1 over the course of the experiment. At night, mean CaCO3 dissolution was 2.4 ± 0.3 mmol CaCO3.m−2.h−1. A much lower CaCO3 dissolution rate of about [35.5-(2.4 × 12)]/12 = 0.5 mmol CaCO3.m−2.h−1 was recorded under light.
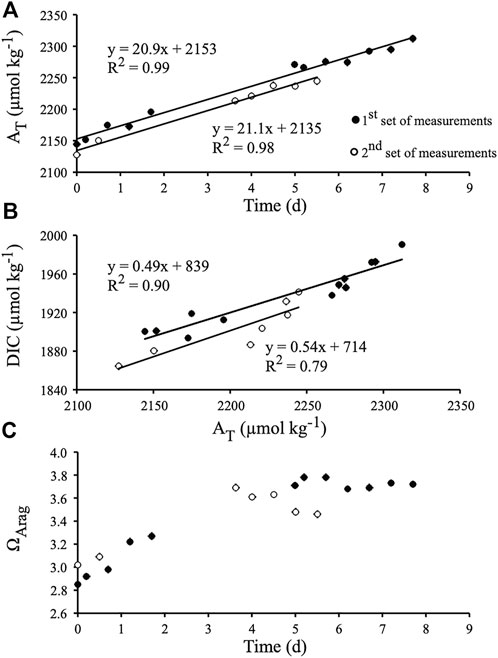
FIGURE 2. Chemical parameters recorded during the first experiment, i.e., during the indoor flume experiment under controlled light (350 µmol photons m−2 s−1, Hawaii). (A) Total alkalinity (AT) monitored over time. (B) Dissolved inorganic carbon (DIC) versus AT. (C) Evolution of the saturation state of aragonite (ΩArag) over the course of the experiment. The equation of each slope is provided as well as its determination coefficient (R2).
DIC concentration increased linearly with time as well, although data were clearly more scattered than for AT (Figure 2B; R2 = 0.91; p < 0.0001, and R2 = 0.83; p < 0.005 before and after water in the flume was renewed, respectively). The slope of the DIC vs. AT relationships (R2 = 0.90; p < 0.0001, and R2 = 0.79; p = 0.007, respectively) did not change between the two parts of the experiment, and the mean slope (0.52 ± 0.02) was not significantly different from 0.5 (p > 0.05; Figure 2B). DIC increase in the flume was therefore mainly due to CaCO3 dissolution while excess production (gross production minus respiration) remained on average close to zero. Night time respiration rate (Rn) was 1.8 ± 0.2 mmol C.m−2.h−1, thus daily respiration (R) was assumed to be 1.8 × 24 = 44 mmol C.m−2.d−1 (as daylight respiration could not be measured). Excess production, calculated from the mean slopes of the AT and DIC vs. time relationships, was 1.9 mmol C.m−2.d−1 (net release of DIC). Gross production (Pg) was therefore 42 mmol C.m−2.d−1, resulting in a Pg/R ratio of 0.96. Net photosynthesis (Pg – (12 × Rn)) was 20 mmol C.m−2 during the 12 h of light, or 1.7 mmol C.m−2.h−1. Aragonite saturation state (ΩArag) varied between 2.85 and 3.02 (ambient) at the beginning of the two series of measurements and increased afterwards due to CaCO3 dissolution (Figure 2C). ΩArag however either stabilized at about 3.7 or decreased at the end of the incubation period, suggesting that some increase in respiration rate or a decrease in photosynthesis occurred at that time. Nevertheless, these ΩArag variations did not affect the CaCO3 dissolution rate.
3.2 Variability of rubbles metabolism under natural daylight (outdoor tank)
In the outdoor experiment ran with another set of rubbles exposed to natural daylight at constant temperature (−27.5°C; Table 1), and after addition of a few ml of HCl to decrease ΩArag down to ∼2, we observed the increase of AT over time in two of the three incubations (Figure 3A). Dissolution rates were 1.4 ± 0.2 and 1.5 ± 0.2 mmol CaCO3.m−2.h−1 during incubation 1 and 2, respectively. DIC decreased in the meantime (Figure 3B), showing that DIC removal linked to photosynthesis exceeded DIC release due to CaCO3 dissolution. Net photosynthesis was 12.9 ± 1.6 and 16.4 ± 3.7 mmol C.m−2.h−1 during incubations 1 and 2, respectively. Due to both net photosynthesis and CaCO3 dissolution, ΩArag increased greatly throughout the two incubations (Figure 3C), from an initial value of 2.1 to a final value of 5.0–5.1 after 5–6 h. In the meantime, pCO2 decreased to about 150 µatm (Figure 3D). Interestingly these variations in seawater chemistry did not affect the CaCO3 dissolution rate.
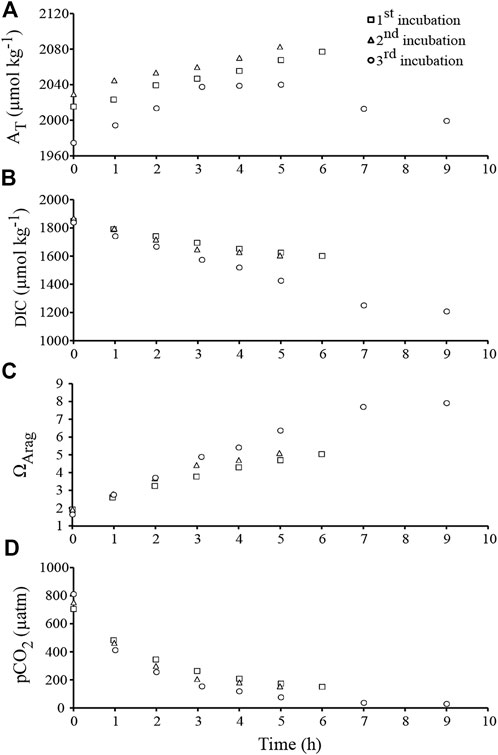
FIGURE 3. Chemical parameters recorded during the course of the second experiment, i.e., during the three outdoor incubations under natural daylight (between 9 a.m. and 6 p.m.; Hawaii). (A) Total alkalinity (AT). (B) Dissolved inorganic carbon (DIC). (C) Saturation state of aragonite (ΩArag). (D) Aqueous partial pressure of CO2 (pCO2).
In contrast, during the third incubation, the AT vs. time relationship was bell-shaped, suggesting that an initial phase of CaCO3 dissolution was followed by some CaCO3 precipitation in the afternoon (Figure 3A). While the initial value of ΩArag was very low (1.8; Figure 3C), ΩArag reached very high values at the end of the day: up to 7.9 after 9 h, corresponding to a pCO2 of about 30 µatm. CaCO3 precipitation clearly prevailed over CaCO3 dissolution for ΩArag values above 6.4 (Figure 3A, C). Since such values are unlikely to be reached in most natural environments, CaCO3 dissolution rate was calculated from the five first hours of the experiment (mean ΩArag = 4.1 ± 0.7, compared to 3.7 ± 0.4 and 3.8 ± 0.5 during the 1st and the 2nd experiment, respectively). Dissolution rate was slowing down from 2.8 to 0.2 mmol CaCO3.m−2.h−1 in the course of the experiment, and was on average 1.8 ± 0.6 mmol CaCO3.m−2.h−1. In the meantime, DIC decreased with time (Figure 3B), and net photosynthesis was 24.6 ± 2.2 mmol C.m−2.h−1.
4 Discussion
Although chasmo- and crypto-endoliths including phototrophs (see definitions in Golubic et al., 1981) are present in coral pores (Yang et al., 2019; Pernice et al., 2020), most of the biomass in dead corals or coral rubble is generally attributed to microboring flora (Wanders, 1977; Vooren 1981; Fine & Loya 2002). Our light microscopy observations of a few pieces of decalcified coral skeleton confirmed the abundance of the photrotrophic eukaryote Ostreobium sp. within the natural microboring communities studied in our rubble set exposed to colonization for over 12 months (Figure 1B). The alga Ostreobium sp. is known to dominate mature microboring communities in dead coral skeletons after more than 6 months of exposure (Chazottes et al., 1995; Le Campion-Alsumard et al., 1995; le Bris et al., 1998; Tribollet et al., 2006; Tribollet, 2008a; Grange et al., 2015). The green turfs growing on rubble surfaces were also similar to those observed on experimental coral blocks exposed to colonization during at least 6 months at 3 m depth near Coconut Island (Tribollet et al., 2006; see also Hatcher and Larkum, 1983). The presence of only a few polychaetes in rubbles was not surprising as after 6 months of exposure, the experimental blocks studied by Tribollet et al. (2006) presented only a few traces of boring polychaetes at a lagoonal site nearby HIMB laboratory. Polychaetes are generally more abundant in dead coral after several years of exposure to colonization as shown by Kiene and Hutchings (1994) and Tribollet and Golubic (2005). Moreover, Hutchings et al. (1992) pointed out that polychaetes are generally less abundant in shallow lagoon reefs than in other environments.
In our experiments, we did not observe the formation of dense epilithic biofilms at the surface of our coral debris after brushing, unlike Leggat et al. (2019). These authors suggested that corals freshly killed by marine heat waves (i.e., denuded coral skeletons) are immediately and intensely colonized by epilithic biofilms dominated by Ostreobium sp. that originate from the interior of the skeleton (thus growing outward), generating strong carbonate dissolution of coral skeletons. This is unlikely as the green alga Ostreobium sp. is a cryptic sciaphile siphonale avoiding intense light intensities to prevent photoinhibition (Fine et al., 2005; Ralph et al., 2007). This alga has developed a large repertoire of specific pigments to live in extremely low light environments such as in coral skeletons (Shashar and Stambler, 1992; Koehne et al., 1999; Iha et al., 2021). It also actively dissolves carbonates by creating galleries that perfectly fit the shape of its filaments (e.g., Chazottes et al., 1995; Carreiro-Silva et al., 2005; Tribollet et al., 2009; Wisshak et al., 2011). Although Massé et al. (2018) and Massé et al. (2020) showed that Ostreobium filaments can live freely in seawater and can be epilithic under certain conditions (see also Kobluk and Risk, 1977), they also never observed the formation of dense biofilms of Ostreobium sp. on dead corals within a few days. This is especially true since more exposure to light, if not too rapid (Fine et al., 2005), stimulates phototrophic microborers’ growth inside carbonates (Schneider and Le Campion-Alsumard 1999; Fine and Loya, 2002; Tribollet and Golubic 2005; Iha et al., 2021). Here we thus present results obtained from typical mature microboring communities that developed in naturally dead corals in reef lagoonal shallow waters.
Brushed coral rubbles under a constant light in the indoor flume (350 µmol photons.m−2.s−1) had a Pg/R ratio of about 1, suggesting much less limitation of microborers’ photosynthesis than in Tribollet et al. (2019)’s study (Pg/R ratio <1 in experimental coral blocks colonized by mature microboring communities dominated by Ostreobium sp. under 200 µmol photons.m−2.s−1). Interestingly, net carbonate dissolution measured in the indoor flume was close to biogenic dissolution measured either by buoyant weight on recently killed corals colonized mostly by Ostreobium and regularly brushed (27–42 mmol.m−2.d−1; Reyes-Nivia et al., 2013), or by microscopy techniques on 1 year or three years-old experimental coral blocks grazed by fishes (8–30 mmol.m−2 d−1; Tribollet 2008a; see Table 2 in Tribollet et al., 2019). Removal of epiliths by brushing coral rubble may have indeed simulated a moderate scrapping pressure. Grazers including scrapers and excavators, remove substrate surfaces (Clements et al., 2017) to feed on endolithic phototrophs (especially Ostreobium sp.). That way they allow more light reaching microborers and consequently, stimulate their growth inside substrates until they reach their new depth of compensation (Tribollet and Golubic, 2005).
The three incubations carried out under natural daylight showed that natural coral rubbles can release alkalinity in seawater at rates as high as 2.8–3.6 mequiv.m−2.h−1 (i.e., a CaCO3 dissolution rate of 1.4–1.8 mmol.m−2.h−1). This is the first time that CaCO3 biogenic dissolution is reported under natural daylight conditions, simultaneously with significant rates of net photosynthesis (13–25 mmol C.m−2.h−1). Assuming a two-fold increase in CaCO3 dissolution due to the use of HCl (consistent with results found during acidification experiments in New Caledonia as well as in literature; Tribollet et al., 2009; Tribollet et al., 2019; Reyes-Nivia et al., 2013), the alkalinity production by coral rubbles under ambient pCO2 would still be high (1.4–1.8 mequiv.m−2.h−1). Interestingly, net CaCO3 biogenic dissolution rate and net photosynthesis increased with time in the three outdoor incubations (up to ΩArag < 6.4), suggesting that constant brushing, combined with some environmental factors such as nutrient availability and temperature, certainly stimulated microborer growth (in depth and/or by branching) over the duration of the experiment. Rates of biogenic dissolution in coral skeletons are known to be related to the biomass of microborers under natural light cycles (Reyes-Nivia et al., 2013; Grange et al., 2015).
By taking into account all experiments, the higher was net photosynthetic activity the higher was net biogenic dissolution (Figure 4), which is a priori counter intuitive as rising pH due to photosynthesis is not thermodynamically in favour of carbonate dissolution. This further supports the hypothesis that alkalinity release is related to the metabolic activity of phototrophic microborers (Tribollet et al., 2019). Light availability and DIC speciation did probably however, influenced pathways of carbon acquisition and thus, CaCO3 dissolution by microborers. Massé et al. (2020) showed that seawater DIC (most probably CO2 according to Tribollet et al., 2009; Tribollet et al., 2019) is the main source of C involved in Ostreobium photosynthesis. However, these authors also reported several other possible sources of C that could be used depending on the DIC concentration in seawater (i.e., HCO3− or CO2 released during carbonate dissolution and organic C remineralized by Ostreobium associated bacteria). The recycling of inorganic C from dissolved CaCO3 was also shown in the boring cyanobacteria, Mastigocoleus testarum, when DIC was limiting (Guida and Garcia-Pichel, 2016).
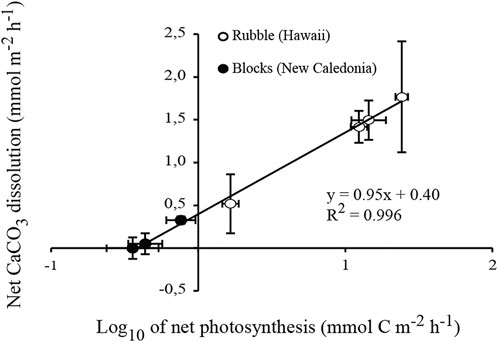
FIGURE 4. Linear relationship between net biogenic dissolution of carbonates and net photosynthesis (expressed in log10). Averaged rates quantified as part of the three experiments (present indoor and outdoor flume experiments, and ocean acidification experiment by Tribollet et al., 2019) are combined. The standard error of each mean is indicated.
In the present study, the logarithmic relationship presented Figure 4 (R2 = 0.996; p < 0.0001) shows that biogenic dissolution increased rapidly as a function of net photosynthesis at low light intensities (≤350 µmol photons.m−2.s−1), but was less dependent on net photosynthetic rates under high natural daylight intensities (740–1300 µmol photons.m−2.s−1). In the case of the outdoor incubations, it is unlikely that CO2 escape into the atmosphere resulted in over-estimation of net photosynthesis because seawater was on average under-saturated with respect to CO2 (<90%). Although we cannot exclude that non-boring phototrophic chasmo- and cryptoendoliths may have benefited from increased amounts of light (Marcelino et al., 2018; Yang et al., 2019), we suggest that most probably the decreasing pCO2 over the course of the outdoor incubations (Figure 3D) in combination with high light availability promoted the use of carbon concentrating mechanisms (CCMs) by Ostreobium filaments which dominated microboring communities. The use of CCM was suggested for the first time by Tribollet et al. (2009) in an acidification experiment, and more recently by Massé et al. (2020) depending on environmental conditions.
For four out of the five drift incubations (indoor and outdoor incubations combined), CaCO3 dissolution rates did not change over time (Figure 2A and3A) although ΩArag was clearly increasing (Figure 2C and 3C). Variations in ΩArag were driven by variations in AT and DIC, which were in turn driven by organic carbon metabolism (net photosynthesis under natural daylight conditions) and/or CaCO3 dissolution. It thus may not be appropriate to attempt to relate CaCO3 fluxes to ΩArag variations (see Andersson and Gledhill, 2013; Chauvin, 2013). Ostreobium response to variations in the parameters of the carbonate system could also be non-linear, as found for some marine calcifiers (Maier et al., 2013) and bioeroding communities dominated by boring sponges (Wisshak et al., 2012; Stubler and Peterson, 2016). Total bioerosion rates (particulate + dissolved CaCO3) for the boring clionaid sponge, Cliona orientalis, were similar under present (pCO2 = 393 ± 56 µatm) and slightly lowered pCO2 conditions (pCO2 = 339 ± 37 µatm), suggesting that the sponge was able to compensate for the less favourable conditions (Wisshak et al., 2012). In a similar manner, the somewhat higher range in pCO2 (pCO2 = 339–512 μatm; ΩArag = 2.85-3.78) during the indoor flume experiment did not affect net biogenic dissolution rates, which were also similar at ΩArag 3 and 3.5 (pCO2 = 570 and 437 µatm respectively) during the acidification experiment in New Caledonia conducted by Tribollet et al. (2019). It is therefore likely that a threshold somewhere between ΩArag 2 and 3 must be reached before promoting increased CaCO3 dissolution by microboring communities dominated by Ostreobium. Above ΩArag 3, microboring communities dominated by Ostreobium seem to be able to maintain a constant carbonate dissolution rate.
Surprisingly, elevated CO2 at the beginning of the incubations performed under natural daylight in the outdoor experiment (757 ± 31 μatm, due to the initial addition of HCl) did not cause an increase of CaCO3 dissolution early in the incubations like it was shown in controlled acidification experiments under low light intensities (Tribollet et al., 2009; Reyes-Nivia et al., 2013; Tribollet et al., 2019). It is hypothesized that high light availability promoted CO2 fixation by RUBISCO, preventing acidification of the intracellular medium of boring filaments. In contrast, during the third incubation performed under natural daylight, CaCO3 dissolution was followed by some CaCO3 precipitation when the tank seawater ΩArag reached values higher than 6.4 and pCO2 decreased down to 30 µatm (Figure 3A and 3C). Conditions where pH is above 9, Ω is very high while pCO2 is close to 13 μatm, are known to stimulate nucleation of CaCO3 in seawater (Morse and He, 1993; Wurgaft et al., 2016). It is most probable here that chemical conditions at the interface (boundary layer) between carbonate rubbles (behaving as carbonate nucleus) and seawater were even more extreme than those measured in the middle of the tank at the end of the third incubation, creating conditions for CaCO3 precipitation. We also hypothesize that under such extreme conditions, either 1) the available energy was allocated by phototrophic microborers (mainly Ostreobium sp.) to CCM to support the Calvin cycle instead of supporting other processes such as the Ca2+ ATPase dependent pumps, and/or 2) the use of HCO3− as a source of CO2 for photosynthesis via the implication of carbonic anhydrase enzymes in Ostreobium filaments (Shashar and Stambler 1992; Iha et al., 2021) may have produced significant amounts of OH− (Giordano et al., 2005) which, combined to H+ protons, could have stopped the biogenic dissolution process (this implies that Ostreobium filaments used Ca2+/H+ ATPase dependent pumps similar to those involved by the bioeroding cyanobacterium Mastigocoleus testerum; (Garcia-Pichel et al., 2010), a hypothesis supported by Krause et al. (2019) and Iha et al. (2021)), and/or 3) the number of Ca2+ pumps was limited so despite an important photosynthetic activity (providing energy), biogenic dissolution could be limited or stopped. In the case of cultured euendolithic cyanobacteria, Garcia-Pichel et al. (2010) showed that calcium release at the substrate surface resulted in a local super-saturation with respect to CaCO3 creating conditions for a micrite layer formation. More recently, Krause et al. (2019) highlighted the capacity of Ostreobium filaments to uptake Ca2+ at their apical thallus tip and to excrete it at the other end of its thallus, most probably in the coral pore spaces causing reprecipitation of CaCO3 in the form of secondary aragonite. In contrast, in the indoor flume, micritization most probably did not contribute to the difference recorded between alkalinity production in the light and in the dark during the indoor flume experiment because ΩArag was at most 3.7 and no curvature of the AT vs. time relationships was observed before ΩArag reached about 5 (Figure 3A and 3C). Nevertheless, this process could probably lower alkalinity production by microborers on some reef flats where extreme diurnal variability in carbonate chemistry is recorded (e.g. ΩArag up to 6.5 during the day at Lady Elliot reef, Great Barrier Reef; Shaw et al., 2012).
5 Conclusion and perspectives
We show here that microboring communities dominated by the phototrophic green alga Ostreobium sp. are most probably the main drivers of reef dead coral skeleton dissolution observed under a large range of saturation states in seawater, at least between 2 and 6.4. In previous studies on microboring flora, no traces of random dissolution by endolithic bacteria inside coral skeletons were observed under scanning electron microscopy (Tribollet and Golubic, 2011; Tribollet et al., 2019) although bacteria are known to be associated to Ostreobium filaments (unpublished data) and the boring cyanobacterium Solentia sp. (see Figure 10 in Le Campion-Alsumard et al., 1996). Those bacteria are preferentially located inside filaments (personal observations) or in polysaccharide envelope layers of cells (Le Campion-Alsumard et al., 1996). We suggest that phototrophic microborers are thus able to keep dissolving reef carbonate substrates under higher saturation states of aragonite than those measured in our study as long as they have enough light and available C sources, as nitrogen source is not limiting (see Massé et al., 2020; Pernice et al., 2020). We thus strongly stress the importance for more investigations to better understand the roles of the phototrophic microboring flora in reef carbonate budget and resilience as they have paradoxical activities: they are able to rise pH, ΩArag, and seawater alkalinity under daylight while they dissolve actively dead carbonate substrates enhancing reef fragility and degradation. Those roles should be studied in more or less mature endolithic communities in rubbles and dead coral substrates as for instance, the epilithic cover (Chazottes et al., 2002) and the presence of macroborers such as sponges and bivalves can greatly influence the composition of microboring flora communities and consequently, the biogenic dissolution rates (Grange et al., 2015; Schönberg et al., 2017; Fordyce et al., 2020). There is also a specific need to better understand the functional roles played by the various genotypes and phenotypes of Ostreobium as Massé et al. (2020) highlighted a large diversity in response to environmental constraints such as the type of habitat. Finally, we also suggest that microborer metabolic activity could counteract the effects of acidification at least at the local scale of very shallow reefs with long water residence time (Page et al., 2016) and in some living calcifiers such as massive corals which are heavily colonized by Ostreobium sp. compare to branching corals (Le Campion-Alsumard et al., 1995; Godinot et al., 2012; Massé et al., 2018) and crustose coralline algae (Tribollet and Payri, 2001; Reyes-Nivia et al., 2014). They could indeed benefit to their host through a potential transfer of photoassimilates (e.g. in Mediterranean subtropical corals: Fine and Loya, 2002; Sangsawang et al., 2017), photoprotection (Galindo-Martinez et al., 2022), and/or through the rise of pH and alkalinity at the microscale inside their host under various environmental conditions (Nothdurft and Webb, 2009; Garcia-Pichel et al., 2010; Reyes-Nivia et al., 2014; Krause et al., 2019; Tribollet et al., 2019 and present study).
Data availability statement
The original contributions presented in the study are included in the article/Supplementary Material, further inquiries can be directed to the corresponding author.
Author contributions
AT designed the experiments. AT and PC collected samples and carried out the analyses. AC helped with statistical analysis. All authors (AT, AC, and PC) interpreted data, discussed trends and wrote the manuscript.
Funding
This study was supported by a Marie Curie Outgoing International Fellowship obtained as part of the FP6 of EU, as well as by the project MIDACOR funded by the French Ministry of Ecology (MEEDDM Ministry action: 23-190RECHERCINCITAT, program 190-090-THUR-BSAF) and IRD (Institut de Recherche pour le Développement).
Acknowledgments
The authors would like to warmly thank the late Marlin J. Atkinson and James H. Fleming, as well as Dan Schar without whom this study could not have been carried out at the Hawaii Institute of Marine Biology on Coconut Island.
Conflict of interest
The authors declare that the research was conducted in the absence of any commercial or financial relationships that could be construed as a potential conflict of interest.
Publisher’s note
All claims expressed in this article are solely those of the authors and do not necessarily represent those of their affiliated organizations, or those of the publisher, the editors and the reviewers. Any product that may be evaluated in this article, or claim that may be made by its manufacturer, is not guaranteed or endorsed by the publisher.
References
Agostini, S., Harvey, B. P., Wada, S., Kon, K., Milazzo, M., Inaba, K., et al. (2018). Ocean acidification drives community shifts towards simplified non-calcified habitats in a subtropical−temperate transition zone. Sci. Rep. 8, 11354. doi:10.1038/s41598-018-29251-7
Andersson, A. J., Bates, N. R., and Mackenzie, F. T. (2007). Dissolution of carbonate sediments under rising pCO2 and ocean acidification: Observations from Devil’s Hole, Bermuda. Aquat. Geochem. 13, 237–264. doi:10.1007/s10498-007-9018-8
Andersson, A. J., and Gledhill, D. (2013). Ocean acidification and coral reefs: Effects on breakdown, dissolution, and net ecosystem calcification. Ann. Rev. Mar. Sci. 5, 321–348. doi:10.1146/annurev-marine-121211-172241
Andersson, A. J., Mackenzie, F. T., and Bates, N. R. (2008). Life on the margin: Implications of ocean acidification on Mg-calcite, high latitude and cold-water marine calcifiers. Mar. Ecol. Prog. Ser. 373, 265–273. doi:10.3354/meps07639
Bindoff, N. L., Cheung, W. W. L., and Kairo, J. G. (2019). IPCC SR ocean and cryosphere chapter 5: Changing ocean, marine ecosystems, and dependent communities. Geneva, Switzerland: IPCC.
Carreiro-Silva, M., McClanahan, T. R., and Kiene, W. E. (2005). The role of inorganic nutrients and herbivory in controlling microbioerosion of carbonate substratum. Coral Reefs 24, 214–221. doi:10.1007/s00338-004-0445-3
Chauvin, A. (2013). “Photosynthèse et calcification sur les récifs coralliens face au changement global et aux impacts anthropiques: Du corail hermatypique Acropora muricata à l’écosystème,” (University of Réunion). thesis.
Chazottes, V., Le Campion-Alsumard, T., and Peyrot-Clausade, M. (1995). Bioerosion rates on coral reefs: Interactions between macroborers, microborers and grazers (moorea, French polynesia). Palaeogeogr. Palaeoclimatol. Palaeoecol. 113, 189–198. doi:10.1016/0031-0182(95)00043-l
Chazottes, V., Le Campion-Alsumard, T., Peyrot-Clausade, M., and Cuet, P. (2002). The effects of eutrophication-related alterations to coral reef communities on agents and rates of bioerosion (Reunion Island, Indian Ocean). Coral Reefs 21, 375–390. doi:10.1007/s00338-002-0259-0
Clements, K. D., German, D. P., Piché, J., Tribollet, A., and Choat, J. H. (2017). Integrating ecological roles and trophic diversification on coral reefs: Multiple lines of evidence identify parrotfishes as microphages. Biol. J. Linn. Soc. 120 (4), 729–751.
Comeau, S., Edmunds, P. J., Spindel, N. B., and Carpenter, R. C. (2014). Fast coral reef calcifiers are more sensitive to ocean acidification in short-term laboratory incubations. Limnol. Oceanogr. 59, 1081–1091. doi:10.4319/lo.2014.59.3.1081
Cyronak, T., Schulz, K. G., Santos, I. R., and Eyre, B. D. (2014). Enhanced acidification of global coral reefs driven by regional biogeochemical feedbacks. Geophys. Res. Lett. 41, 5538–5546. doi:10.1002/2014GL060849
Dickson, A., Christian, J. R., and Sabine, C. L. (2007). Guide to best practices for ocean CO2 measurements. Canada: PICES Special Publication.
Dickson, A. G. (1990). Standard potential of the reaction: AgCl(s) + 1/2H2(g) = Ag(s) + HCl(aq), and and the standard acidity constant of the ion HSO4− in synthetic sea water from 273.15 to 318.15 K. J. Chem. Thermodyn. 22, 113–127. doi:10.1016/0021-9614(90)90074-z
Enochs, I. C., Manzello, D. P., Tribollet, A., Valentino, L., Kolodziej, G., Donham, E. M., et al. (2016). Elevated colonization of microborers at a volcanically acidified coral reef. PLoS ONE 11, e0159818. doi:10.1371/journal.pone.0159818
Eyre, B. D., Andersson, A. J., and Cyronak, T. (2014). Benthic coral reef calcium carbonate dissolution in an acidifying ocean. Nat. Clim. Chang. 4, 969–976. doi:10.1038/nclimate2380
Fabricius, K. E., Langdon, C., Uthicke, S., Humphrey, C., Noonan, S., De’ath, G., et al. (2011). Losers and winners in coral reefs acclimatized to elevated carbon dioxide concentrations. Nat. Clim. Chang. 1, 165–169. doi:10.1038/nclimate1122
Falter, J. L., Atkinson, M. J., Fleming, J. H., Bos, M. M., Lowe, R. J., Koseff, J. R., et al. (2006). A novel flume for simulating the effects of wave- and tide-driven water motion on the biogeochemistry of benthic reef communities. Limnol. Oceanogr. Methods 4, 68–79. doi:10.4319/lom.2006.4.68
Falter, J. L., Lowe, R. J., Atkinson, M. J., and Cuet, P. (2012). Seasonal coupling and de-coupling of net calcification rates from coral reef metabolism and carbonate chemistry at Ningaloo Reef, Western Australia. J. Geophys. Res. 117, C05003. doi:10.1029/2011JC007268
Fine, M., and Loya, Y. (2002). Endolithic algae: An alternative source of photoassimilates during coral bleaching. Proc. R. Soc. Lond. B 269, 1205–1210. doi:10.1098/rspb.2002.1983
Fine, M., Meroz-Fine, E., and Hoegh-Guldberg, O. (2005). Tolerance of endolithic algae to elevated temperature and light in the coral Montipora monasteriata from the southern Great Barrier Reef. J. Exp. Biol. 208, 75–81. doi:10.1242/jeb.01381
Fordyce, A. J., Ainsworth, T. D., and Leggat, W. (2020). Microalgae, a boring bivalve and a coral – A newly described association between two coral reef bioeroders within their coral host. Integr. Org. Biol. 2 (1), obaa035. doi:10.1093/iob/obaa035
Galindo-Martinez, C. T., Weber, M., Avila-Magňa, V., Enriquez, S., Kitano, H., Medina, M., et al. (2022). The role of the endolithic alga Ostreobium spp. during coral bleaching recovery. Sci. Rep. 12, 2977. doi:10.1038/s41598-022-07017-6
Garcia-Pichel, F., Ramírez-Reinat, E., and Gao, Q. (2010). Microbial excavation of solid carbonates powered by P-type ATPase-mediated transcellular Ca2+ transport. Proc. Natl. Acad. Sci. U. S. A. 107, 21749–21754. doi:10.1073/pnas.1011884108
Gektidis, M. (1999). Development of microbial euendolithic communities: The influence of light and time. bgsd. 45, 147–150. doi:10.37570/bgsd-1998-45-18
Giordano, M., Beardall, J., and Raven, J. A. (2005). CO2 concentrating mechanisms in algae: Mechanisms, environmental modulation, and evolution. Annu. Rev. Plant Biol. 56, 99–131. doi:10.1146/annurev.arplant.56.032604.144052
Godinot, C., Tribollet, A., Grover, R., and Ferrier-Pagès, C. (2012). Bioerosion by euendoliths decreases in phosphate-enriched skeletons of living corals. Biogeosciences 9, 2377–2384. doi:10.5194/bg-9-2377-2012
Golubic, S., Friedmann, I., and Schneider, J. (1981). The lithobiontic ecological niche, with special reference to microorganisms. J. Sediment. Petrology 51, 0475–0478.
Grange, J. S., Rybarczyk, H., and Tribollet, A. (2015). The three steps of the carbonate biogenic dissolution process by microborers in coral reefs (New Caledonia). Environ. Sci. Pollut. Res. 22, 13625–13637. doi:10.1007/s11356-014-4069-z
Guida, B. S., and Garcia-Pichel, F. (2016). Draft genome assembly of a filamentous euendolithic (true boring) cyanobacterium, Mastigocoleus testarum strain BC008. Genome Announc. 4, 015744-15. doi:10.1128/genomeA.01574-15
Guinotte, J. M., and Fabry, V. J. (2008). Ocean acidification and its potential effects on marine ecosystems. Ann. N. Y. Acad. Sci. 1134, 320–342. doi:10.1196/annals.1439.013
Hatcher, B. G., and Larkum, A. W. D. (1983). An experimental analysis of factors controlling the standing crop of the epilithic algal community on a coral reef. J. Exp. Mar. Biol. Ecol. 69, 61–84. doi:10.1016/0022-0981(83)90172-7
Hutchings, P. A., Kien, W. E., Cunningham, R. B., and Donnelly, C. (1992). Spatial and temporal patterns of non-colonial boring organisms (polychaetes, sipunculans and bivalve molluscs) in Porites at Lizard Island, Great Barrier Reef. Coral Reefs 11, 23–31. doi:10.1007/bf00291931
Iha, C., Dougan, K. E., Varela, J. A., Avila, V., Jackson, C. J., Bogaert, K. A., et al. (2021). Genomic adaptations to an endolithic lifestyle in the coral-associated alga Ostreobium. Curr. Biol. 31, 1393–1402.e5. doi:10.1016/j.cub.2021.01.018
Johnson, M. D., Price, N. N., and Smith, J. E. (2014). Contrasting effects of ocean acidification on tropical fleshy and calcareous algae. PeerJ 2, e411. doi:10.7717/peerj.411
Kiene, W. E., and Hutchings, P. A. (1994). Bioerosion experiments at lizard Island, Great barrier reef. Coral Reefs 13, 91–98. doi:10.1007/bf00300767
Kobluk, D. R., and Risk, M. J. (1977). Micritization and carbonate-grain binding by endolithic algae. Am. Assoc. Petroleum Geol. Bull. 61 (7), 1069–1082.
Koehne, B., Elli, G., Jennings, R. C., Wilhelm, C., and Trissl, H.-W. (1999). Spectroscopic and molecular characterization of a long wavelength absorbing antenna of Ostreobium sp. Biochimica Biophysica Acta - Bioenergetics 1412, 94–107. doi:10.1016/S0005-2728(99)00061-4Available at: www.elsevier.com/locate/bba.
Krause, S., Liebetrau, V., Nehrke, G., Damm, T., Büsse, S., Leipe, T., et al. (2019). Endolithic algae affect modern coral carbonate morphology and chemistry. Front. Earth Sci. (Lausanne). 7, 1–19. doi:10.3389/feart.2019.00304
Krumins, V., Gehlen, M., Arndt, S., van Cappellen, P., and Regnier, P. (2013). Dissolved inorganic carbon and alkalinity fluxes from coastal marine sediments: Model estimates for different shelf environments and sensitivity to global change. Biogeosciences 10, 371–398. doi:10.5194/bg-10-371-2013
Kuffner, I. B., Andersson, A. J., Jokiel, P. L., Rodgers, K. S., and MacKenzie, F. T. (2008). Decreased abundance of crustose coralline algae due to ocean acidification. Nat. Geosci. 1, 114–117. doi:10.1038/ngeo100
Langdon, C., and Atkinson, M. J. (2005). Effect of elevated pCO2 on photosynthesis and calcification of corals and interactions with seasonal change in temperature/irradiance and nutrient enrichment. J. Geophys. Res. 110, C09S07–16. doi:10.1029/2004JC002576
Le Bris, S., Le Campion-Alsumard, T., and Romano, J.-C. (1998). Caractéristiques du feutrage algal des récifs coralliens de Polynésie française soumis à différentes intensités de bioérosion. Oceanol. Acta 21, 695–708. doi:10.1016/s0399-1784(99)80025-5
Le Campion-Alsumard, T., Golubic, S., and Hutchings, P. (1995). Microbial endoliths in skeletons of live and dead corals: Porites lobata (Moorea, French Polynesia). Mar. Ecol. Prog. Ser. 117, 149–157. doi:10.3354/meps117149
Le Campion-Alsumard, T., Golubic, S., and Pantazidou, A. (1996). On the euendolithic genus Solentia ERCEGOVIC (Cyanophyta/Cyanobacteria). Archiv_algolstud. 83, 107–127. doi:10.1127/algol_stud/83/1996/107
Leggat, W. P., Camp, E. F., Suggett, D. J., Heron, S. F., Fordyce, A. J., Gardner, S., et al. (2019). Rapid coral decay is associated with marine heatwave mortality events on reefs. Curr. Biol. 29, 2723–2730.e4. doi:10.1016/j.cub.2019.06.077
Maier, C., Schubert, A., Berzunza Sànchez, M. M., Weinbauer, M. G., Watremez, P., and Gattuso, J. P. (2013). End of the century pCO2 levels do not impact calcification in mediterranean cold-water corals. PLoS ONE 8, e62655. doi:10.1371/journal.pone.0062655
Marcelino, V. R., van Oppen, M. J. H., and Verbruggen, H. (2018). Highly structured prokaryote communities exist within the skeleton of coral colonies. ISME J. 12, 300–303. doi:10.1038/ismej.2017.164
Massé, A., Domart-Coulon, I., Golubic, S., Duché, D., and Tribollet, A. (2018). Early skeletal colonization of the coral holobiont by the microboring Ulvophyceae Ostreobium sp. Sci. Rep. 8, 2293. doi:10.1038/s41598-018-20196-5
Massé, A., Tribollet, A., Meziane, T., Bourguet-Kondracki, M. L., Yéprémian, C., Sève, C., et al. (2020). Functional diversity of microboring Ostreobium algae isolated from corals. Environ. Microbiol. 22, 4825–4846. doi:10.1111/1462-2920.15256
Morse, J. W., and He, S. (1993). Influences of T, S and pCO2 on the pseudo-homogeneous precipitation of CaCO3 from seawater: Implications for whiting formation. Mar. Chem. 41, 291–297. doi:10.1016/0304-4203(93)90261-l
Nothdurft, L. D., and Webb, G. E. (2009). Earliest diagenesis in scleractinian coral skeletons: Implications for palaeoclimate-sensitive geochemical archives. Facies 55, 161–201. doi:10.1007/s10347-008-0167-z
Orr, J. C., Fabry, V. J., Aumont, O., Bopp, L., Doney, S. C., Feely, R. A., et al. (2005). Anthropogenic ocean acidification over the twenty-first century and its impact on calcifying organisms. Nature 437, 681–686. doi:10.1038/nature04095
Page, H. N., Andersson, A. J., Jokiel, P. L., Rodgers, K. S., Lebrato, M., Yeakel, K., et al. (2016). Differential modification of seawater carbonate chemistry by major coral reef benthic communities. Coral Reefs 35, 1311–1325. doi:10.1007/s00338-016-1490-4
Pandolfi, J. M., Connolly, S. R., Marshall, D. J., and Cohen, A. L. (2011). Projecting coral reef futures under global warming and ocean acidification. Science 333, 418–422. doi:10.1126/science.1204794Available at: http://paleodb.org/cgi-bin/bridge.pl.
Pernice, M., Raina, J. B., Rädecker, N., Cárdenas, A., Pogoreutz, C., and Voolstra, C. R. (2020). Down to the bone: The role of overlooked endolithic microbiomes in reef coral health. ISME J. 14, 325–334. doi:10.1038/s41396-019-0548-z
Pierrot, D., Lewis, E., and Wallace, D. W. R. (2006). MS Excel program developed for CO2 system calculations. Tennessee: ORNL/CDIAC-105 Carbon Dioxide Information Analysis Center.
Ralph, P. J., Larkum, A. W. D., and Kühl, M. (2007). Photobiology of endolithic microorganisms in living coral skeletons: 1. Pigmentation, spectral reflectance and variable chlorophyll fluorescence analysis of endoliths in the massive corals Cyphastrea serailia, Porites lutea and Goniastrea australensis. Mar. Biol. 152, 395–404. doi:10.1007/s00227-007-0694-0
Reyes-Nivia, C., Diaz-Pulido, G., and Dove, S. (2014). Relative roles of endolithic algae and carbonate chemistry variability in the skeletal dissolution of crustose coralline algae. Biogeosciences 11, 4615–4626. doi:10.5194/bg-11-4615-2014
Reyes-Nivia, C., Diaz-Pulido, G., Kline, D., Guldberg, O. H., and Dove, S. (2013). Ocean acidification and warming scenarios increase microbioerosion of coral skeletons. Glob. Chang. Biol. 19, 1919–1929. doi:10.1111/gcb.12158
Roy, R. N., Roy, L. N., Vogel, K. M., Porter-Moore, C., Pearson, T., Good, C. E., et al. (1993). The dissociation constants of carbonic acid in seawater at salinities 5 to 45 and temperatures 0 to 45°C. Mar. Chem. 44, 249–267. doi:10.1016/0304-4203(93)90207-5
Sabine, C. L., Feely, R. A., Gruber, N., Key, R. M., Lee, K., Bullister, J. L., et al. (2004). The oceanic sink for anthropogenic CO2. Science 305, 367–371. doi:10.1126/science.1097403
Sangsawang, L., Casareto, B. E., Ohba, H., Vu, H. M., Meekaew, A., Suzuki, T., et al. (2017). 13C and 15N assimilation and organic matter translocation by the endolithic community in the massive coral Porites lutea. R. Soc. open Sci. 4, 171201. doi:10.1098/rsos.171201
Schneider, J., and Le Campion-Alsumard, T. (1999). Construction and destruction of carbonates by marine and freshwater cyanobacteria. Eur. J. Phycol. 34 (4), 417–426. doi:10.1080/09670269910001736472
Schönberg, C. H. L., Fang, J. K. H., Carreiro-Silva, M., Tribollet, A., and Wisshak, M. (2017). Bioerosion: The other ocean acidification problem. ICES J. Mar. Sci. 74, 895–925. doi:10.1093/icesjms/fsw254
Shamberger, K. E. F., Feely, R. A., Sabine, C. L., Atkinson, M. J., DeCarlo, E. H., Mackenzie, F. T., et al. (2011). Calcification and organic production on a Hawaiian coral reef. Mar. Chem. 127, 64–75. doi:10.1016/j.marchem.2011.08.003
Shashar, N., and Stambler, N. (1992). Endolithic algae within corals - life in an extreme environment. J. Exp. Mar. Biol. Ecol. 163, 277–286. doi:10.1016/0022-0981(92)90055-f
Shaw, E. C., McNeil, B. I., and Tilbrook, B. (2012). Impacts of ocean acidification in naturally variable coral reef flat ecosystems. J. Geophys. Res. 117, C03038. doi:10.1029/2011JC007655
Stubler, A. D., and Peterson, B. J. (2016). Ocean acidification accelerates net calcium carbonate loss in a coral rubble community. Coral Reefs 35, 795–803. doi:10.1007/s00338-016-1436-x
Tribollet, A., Chauvin, A., and Cuet, P. (2019). Carbonate dissolution by reef microbial borers: A biogeological process producing alkalinity under different pCO2 conditions. Facies 65, 9. doi:10.1007/s10347-018-0548-x
Tribollet, A. (2008a). Dissolution of dead corals by euendolithic microorganisms across the northern Great Barrier Reef (Australia). Microb. Ecol. 55, 569–580. doi:10.1007/s00248-007-9302-6
Tribollet, A., Godinot, C., Atkinson, M., and Langdon, C. (2009). Effects of elevated pCO2 on dissolution of coral carbonates by microbial euendoliths. Glob. Biogeochem. Cycles 23, GB3008. doi:10.1029/2008GB003286
Tribollet, A., and Golubic, S. (2005). Cross-shelf differences in the pattern and pace of bioerosion of experimental carbonate substrates exposed for 3 years on the northern Great Barrier Reef, Australia. Coral Reefs 24, 422–434. doi:10.1007/s00338-005-0003-7
Tribollet, A., and Golubic, S. (2011). “Reef bioerosion: Agents and processes,” in Coral reefs: An ecosytem in transition. Editors Z. Dubinsky, and N. Stambler (Berlin Heidelberg New York: Springer Science + Business Media), 435–449. doi:10.1007/978-94-007-0114-4_25
Tribollet, A., Langdon, C., Golubic, S., and Atkinson, M. (2006). Endolithic microflora are major primary producers in dead carbonate substrates of Hawaiian coral reefs. J. Phycol. 42, 292–303. doi:10.1111/j.1529-8817.2006.00198.x
Tribollet, A., and Payri, C. (2001). Bioerosion of the coralline alga Hydrolithon onkodes by microborers in the coral reefs of Moorea, French Polynesia. Oceanol. Acta 24, 329–342. doi:10.1016/s0399-1784(01)01150-1
Tribollet, A. (2008b). “The boring microflora in modern coral reef ecosystems: A review of its roles,” in Current developments in bioerosion. Editors M. Wisshak, and L. Tapanila (Berlin Heidelberg New York: Springer), 67–94.
Vargas, C. A., Cuevas, L. A., Broitman, B. R., San Martin, V. A., Lagos, N. A., Gaitan-Espitia, J. G., et al. (2022). Upper environmental pCO2 drives sensitivity to ocean acidification in marine invertebrates. Nat. Clim. Chang. 12, 200–207. doi:10.1038/s41558-021-01269-2
Vooren, C. M. (1981). Photosynthetic rates of benthic algae from the deep coral reef of Curaçao. Aquat. Bot. 10, 143–159. doi:10.1016/0304-3770(81)90017-6
Wanders, J. B. W. (1977). The role of benthic algae in the shallow reef of curaçao (Netherlands antilles) III : The significance of grazing. Aquat. Bot. 3, 357–390. doi:10.1016/0304-3770(77)90040-7
Wisshak, M., Schönberg, C. H. L., Form, A., and Freiwald, A. (2012). ocean acidification accelerates reef bioerosion. PLoS ONE 7, e45124. doi:10.1371/journal.pone.0045124
Wisshak, M., Tribollet, A., Golubic, S., Jakobsen, J., and Freiwald, A. (2011). Temperate bioerosion: Ichnodiversity and biodiversity from intertidal to bathyal depths (azores). Geobiology 9, 492–520. doi:10.1111/j.1472-4669.2011.00299.x
Wurgaft, E., Steiner, Z., Luz, B., and Lazar, B. (2016). Evidence for inorganic precipitation of CaCO3 on suspended solids in the open water of the Red Sea. Mar. Chem. 186, 145–155. doi:10.1016/j.marchem.2016.09.006
Keywords: biogenic carbonate dissolution, microboring flora, euendoliths, production of seawater alkalinity, saturation state of aragonite, coral reef ecosystems
Citation: Tribollet A, Chauvin A and Cuet P (2022) Natural photosynthetic microboring communities produce alkalinity in seawater whereas aragonite saturation state rises up to five. Front. Earth Sci. 10:894501. doi: 10.3389/feart.2022.894501
Received: 11 March 2022; Accepted: 25 November 2022;
Published: 21 December 2022.
Edited by:
Ana Santos, University of Oviedo, SpainReviewed by:
Chris Langdon, University of Miami, United StatesFrancisco J. Rodriguez-Tovar, University of Graanada, Spain
Copyright © 2022 Tribollet, Chauvin and Cuet. This is an open-access article distributed under the terms of the Creative Commons Attribution License (CC BY). The use, distribution or reproduction in other forums is permitted, provided the original author(s) and the copyright owner(s) are credited and that the original publication in this journal is cited, in accordance with accepted academic practice. No use, distribution or reproduction is permitted which does not comply with these terms.
*Correspondence: Aline Tribollet, YWxpbmUudHJpYm9sbGV0QGlyZC5mcg==