- Department of Geoscience, University of Nevada Las Vegas, Las Vegas, NV, United States
The transition towards zero-CO2 energy generation, storage and transport will require a range of metals that are often considered critical and are produced as byproducts of the production of other metals. This means that the reliance of some critical elements on the production of main metal commodities, such as Cu and Ni, is a significant source of supply risk. However, how can we evaluate resource scarcity and supply risks for elements that we do not routinely analyze for and characterize in present day mined ores? Here we demonstrate a method for exploring for and assessing the byproduct critical element potential of magmatic sulfide and volcanogenic massive sulfide deposits using a LA–ICP–MS database. Our results indicate there are significant enrichments of Sb, Bi, Cd, Co, Se and Te in pentlandite (Ni sulfide), chalcopyrite (Cu sulfide) and sphalerite (Zn sulfide) within these systems, demonstrating the need for a holistic approach to critical element research with unrecovered byproducts in existing mining supply chains having the potential to solve perceived resource scarcity challenges.
Introduction
Modern day exploration for base (Cu–Pb–Zn–Ni) and precious (Au–Ag–platinum group element (PGE)) metals integrates geological, geochemical and geophysical datasets within a metallogenic framework to target geographical locations for investigation at a range of scales. A mineral deposit is defined as a concentration of useful metals, minerals, or rocks whereas an ore deposit is a mineral deposit that can be exploited for a profit (e.g., Pohl, 2020; Jowitt and McNulty, 2021b). The elevation of a mineral deposit to a mineral resource or an ore deposit requires the collection and assessment of multiple datasets by professionally qualified persons to determine the economic feasibility of the raw materials contained within the deposit (Jowitt and McNulty, 2021b). Some raw materials, such as Cu, Ni, Zn, and Fe, have well-established metallogenic frameworks (geology) and commodity markets (economics); in other words, humanity has been successful at finding and developing these needed resources in the form of main-product mining operations that operate above the mineralogical barrier (Skinner, 1976). However, there are numerous metaliferous raw materials that have become increasingly technologically important, such as In (Werner et al., 2017; Jowitt and McNulty, 2021a), which as yet do not have the market demand to justify industry investment to explore for and develop into main-product mining operations (e.g., Sykes et al., 2016). This means that the current supply and demand for these raw materials is met by the extraction of these metals as byproducts during the processing of base and precious metals (Figure 1; Nassar et al., 2015; Huston, 2021). This, combined with the potential for rapid increases in demand for these raw materials (Jowitt and McNulty, 2021a) means they have higher security of supply risk. This increased risk has led some governments and organizations to categorize these raw materials as critical metals and minerals along with other main and co-product metals and minerals that have supply chains that are at risk for a number of different reasons (e.g., McNulty and Jowitt, 2021). Examples of these by-product critical metal and minerals include Sb, Cd, Co, In, Se, and Te, which are used in technologies for low-emissions energy production such as solar panels and wind turbines, and energy storage, such as batteries (Jowitt et al., 2018; Jowitt and McNulty, 2021a). In comparison, Bi is used as an additive for fusible alloys and free machining steels, as well as in electronic products such as a non-toxic replacement for lead in solders (Krenev et al., 2015; Austrade, 2020). All of these elements are characterized as critical by various governments and agencies (McNulty and Jowitt, 2021), in part because their supply is predicated on their metal companionality with the production of base and precious main metals (Figure 1A) from which they are recovered as by- or coproducts (Figure 1B).
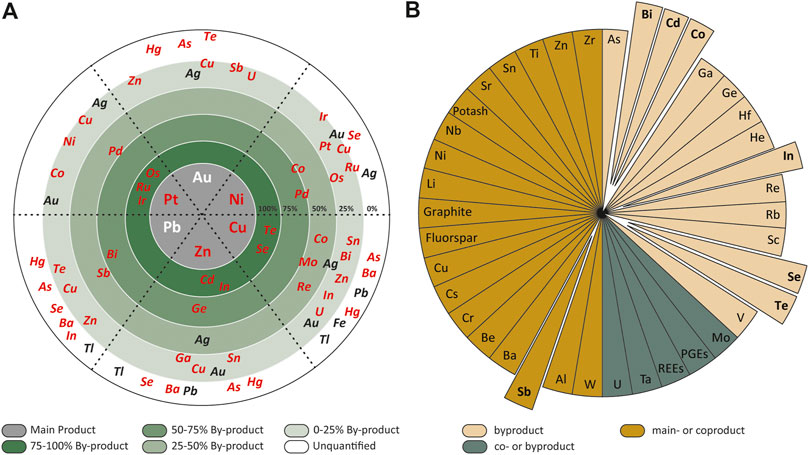
FIGURE 1. (A) The wheel of metal companionality for select base and precious metals (after Nassar et al., 2015; Jowitt et al., 2018) which shows the relationship between primary metal (the central part of the wheel) and by- or coproduct elements and their percentage of production with critical elements highlighted in red. (B) Critical elements categorized by their byproduct, coproduct and main product nature (adapted from Huston, 2021). The critical elements investigated in this study are Sb, Bi, Cd, Co, Se and Te.
Magmatic Ni-Cu-PGE sulfide deposits are accumulations of base and precious metals that form from the segregation of immiscible sulfide liquids from mafic or ultramafic silicate magmas (Naldrett, 2010). These deposits contain about one third of the world’s Ni mineral resources and are also important producers of the PGEs, Co and Cu (Mudd and Jowitt, 2014; Nassar et al., 2015). Volcanogenic massive sulfide (VMS) deposits form from metal-bearing hydrothermal fluids associated with seafloor and sub-seafloor hydrothermal convection and are major sources of Zn, Cu, Pb, Ag, and Au, as well as significant sources of other elements such as Co, Sn, and Se, amongst others (Galley et al., 2007). This study presents a method for the assessment of critical element byproduct resources in magmatic sulfide and VMS mineral deposits, two key sources of base and precious metals, as an initial step to enhance the production of these elements. This targeting of areas of enhanced recovery is a more time- and cost-effective approach to the improved extraction (and hence sustainability) of these byproduct metals than undertaking expensive and time-consuming deportment studies of the majority of mineral deposits and represents an ideal complement to downstream-up studies examining smelter and refinery data to identify high potential mineral deposit systems (McNulty et al., 2022). In addition, this approach represents a template for the targeting of specific parts of the minerals industry for enhanced byproduct critical metal and mineral recovery through deportment and mineral processing studies to understand the way these individual elements behave within the mining-smelting-refining value chain.
Our approach uses a compilation of laser ablation–inductively coupled plasma–mass spectrometry (LA–ICP–MS) data for previously published analyses of pentlandite, chalcopyrite and sphalerite. These data are used to evaluate the chalcophile Sb, Bi, Cd, Co, In, Se, and Te byproduct critical element resource potentials of a range of magmatic sulfide and VMS deposits. Base metal sulfide concentrates are typically liberated from magmatic and VMS mineral deposits using froth flotation mineral processing methods with main commodity metals extracted by a combination of pyrometallurgical and hydrometallurgical techniques (e.g., Berry et al., 2001). These techniques produce intermediates and/or byproducts that can contain significant quantities of critical elements (McNulty et al., 2022). This means that sulfide concentrates from these deposits have the potential to contain recoverable byproduct critical elements that can be estimated using LA–ICP–MS analytical data. The proxies developed during this study using this approach highlight the potential for realizing new streams of hidden byproduct critical element supplies in sulfide-rich ore systems which, could in part, meet the forecasted increases in demand for raw materials vital for non-fossil fuel energy technologies (Drexhage et al., 2017; Lee et al., 2020).
Materials and Methods
The trace element concentrations of pentlandite, chalcopyrite and sphalerite from magmatic sulfide and VMS deposits from 2,220 compiled LA–ICP–MS point analyses indicate that the sulfide ore minerals in these systems contain significant amounts of Sb, Bi, Cd, Co, In, Se and Te (Tables 1, 2; Figure 2; Supplementary Table S1). To explore for hidden byproduct critical element resources, proxy inventories of Sb, Bi, Cd, Co, In, Se, and Te were calculated based on average trace element concentrations in Ni, Cu, and Zn sulfide ore minerals for four magmatic sulfide and six VMS deposits. These ten ore deposits were selected for investigation because: a) mineral resource, ore reserves or historic production information were available for the main metal commodities (grade and tonnage data); b) several trace critical element concentrations were reported for the analyzed ore minerals from each deposit; and c) each mineral deposit had a minimum of 30 LA–ICP–MS spot analyses and at least two samples of sulfide mineralization were analyzed. Contained chalcopyrite, pentlandite and sphalerite abundances were estimated based on publicly available mineral resource, ore reserve or historic production data (Supplementary Table S2). These estimates assume that Cu, Ni, and Zn are contained in chalcopyrite (CuFeS2), pentlandite (Ni4.5Fe4.5S8) and sphalerite (Zn0.5Fe0.5S), a reasonable assumption given the dominance of these minerals within Cu, Ni, and Zn supply chains. Inferred contents of byproduct critical elements were estimated by multiplying the estimated amount of each contained mineral phase by its average trace element composition. These proxy byproduct inventories are reported in metric tonnes (t) and rounded to the nearest integer or base ten (Supplementary Table S3). Although there are inherent uncertainties in this proxy approach, specifically the assumptions that Cu, Ni and Zn grades report to one mineral phase and that the limited LA–ICP–MS data are representative of each mineral deposit, it provides an initial assessment for elements that are not routinely analyzed for and considered in resource calculations. In addition, unlike whole-rock assay data, this proxy has the benefit of excluding byproduct elements that might be trapped in gangue minerals and therefore provides an estimate of the potential abundance of byproducts that would report to base metal sulfide concentrates. It is also likely that some of the byproduct elements within the deposits being considered reside in minerals other than the sulfides considered here, for example tellurides or bismuth minerals. Although these minerals are certainly of interest for byproduct critical metal extraction, the processing approaches used in the base metal mines considered here may well not concentrate these minerals, meaning that any incorporation of these analyses might give an erroneous idea of what critical metals are present in existing metal supply chains and materials flows. The outcome here is information on the short-term critical metal potential of existing supply chains derived from the mineral deposits that form the focus of this study. It should also be noted that comparing different analytical protocols, standard reference materials, and performances of the different LA–ICP–MS systems used to generate the data considered here is beyond the scope of this paper (e.g., Wohlgemuth-Ueberwasser and Jochum 2015). However, the analytical conditions and routines used in each study from our database, along with the obtained detection limits, are summarized in the Supplementary Table S4 for data quality assurance purposes.
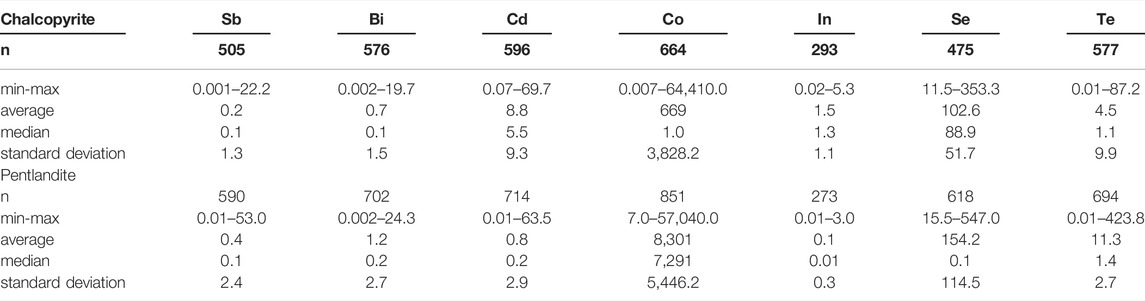
TABLE 1. Summary of select trace element concentrations (ppm) in chalcopyrite and pentlandite from 22 magmatic sulfide deposits (Supplementary Table 1; data sources therein).
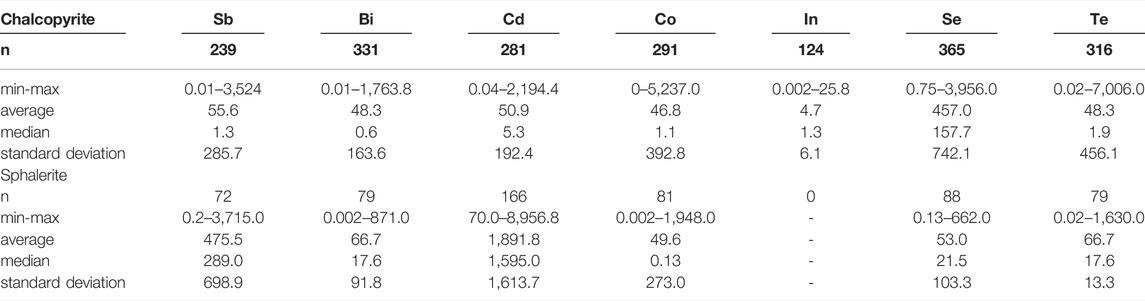
TABLE 2. Summary of select trace element concentrations (ppm) in chalcopyrite and sphalerite from 28 volcanogenic massive sulfide deposits (Supplementary Table 1; data sources therein).
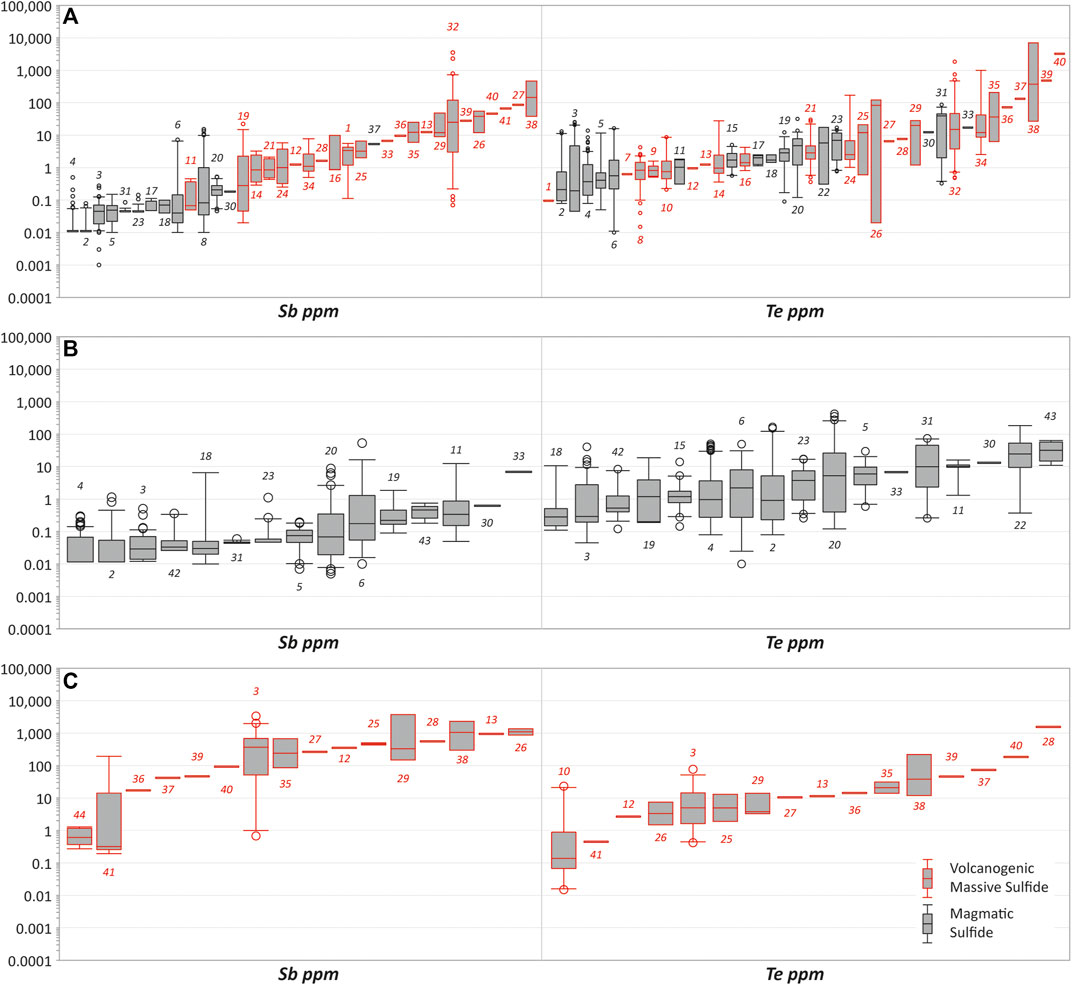
FIGURE 2. Summary of Sb and Te trace element concentrations in ore sulfides from compiled LA-ICP-MS analyses of magmatic sulfide (N = 18) and volcanogenic massive sulfide (N = 26) deposits (Supplementary Table S1). (A) Chalcopyrite. (B) Pentlandite. (C) Sphalerite. Although Sb, Bi, Cd, Co, In, Se and Te were investigated in this study here we only show Sb and Te as an example to save space. Mineral Deposits 1, Vorta; 2, Stillwater Complex; 3, Lac des Iles Complex; 4, Bushveld Complex; 5, Mirabela Complex; 6, Great Dyke; 7, Mala; 8, Sulitjelma; 9, Phoenix; 10, Bieluwutu; 11, Alexo; 12, Tash-Tau; 13, Talgan; 14, Kokkinoyla; 15, Aguablanca; 16, Three Hills; 17, Talnakh; 18, Yangliuping; 19, Duluth; 20, Sudbury Complex; 21, Apliki; 22, Jinchuan Complex; 23, Kharaelakh; 24, Phoucasa; 25, Yubileynoye; 26, Dergamysh; 27, Alexandrinskoye; 28, Oktyabrskoye; 29; Safyanovskoye; 30, Serranía de Ronda; 31, Norilsk; 32, Çayeli; 33, Beni Bousera; 34, Kutluar; 35, Uzgela; 36, Buribay; 37, Valentorskoye; 38, Yaman-Kasay; 39, Sultanovskoye; 40, Moldezhnoye; 41, Mathiatis N; 42, Voisey’s Bay; 43, Rosie Nickel Project; 44, Agrokipia B.
Results
Byproduct critical element inventory proxies based on trace element concentrations in pentlandite, chalcopyrite and sphalerite (Table 3) were calculated for magmatic sulfide (n = 4) and VMS (n = 6) deposits. These data show the variance in critical element supply potential in these deposit types as well as sulfide mineral deportment (Table 4). Magmatic sulfide deposits are considerably enriched in Co, with ∼2,260–20,910 t proxy inventories, compared to VMS deposits, which have lower Co but significant proxy inventories of Sb, Bi and Cd: up to ∼1,460 t Sb, ∼170 t Bi and ∼8,790 t Cd (Table 4). In comparison, Se and Te are present in significant quantities (by proxy) in both mineral deposit types (Table 4). For instance, Vale’s Sudbury magmatic sulfide deposits have proxy inventories of Se and Te of ∼670 t and ∼100 t, respectively, whereas the Çaylie VMS deposit potentially contains ∼120 t Se and ∼80 t Te in base metal sulfide ores. In addition, VMS deposits can also contain sulfide ore minerals that are only enriched in Se, as exemplified by the Sultijelma deposit, which has a Se proxy inventory of ∼280 t, or the Yaman-Kasay deposit that is enriched in Te relative to Se with a Te proxy inventory of ∼340 t (Table 4). These results are consistent with previous research examining the concentrations of these elements within copper anode slimes derived from both magmatic and VMS deposits (McNulty et al., 2022). Of the analysed trace elements, there is limited data for In and as such it is unclear if there is preferential enrichment of In in the evaluated magmatic and VMS deposits. This is perhaps unsurprising given the seeming preference for In to be present in systems enriched in Pb and Zn rather than Cu (e.g., Werner et al., 2017).
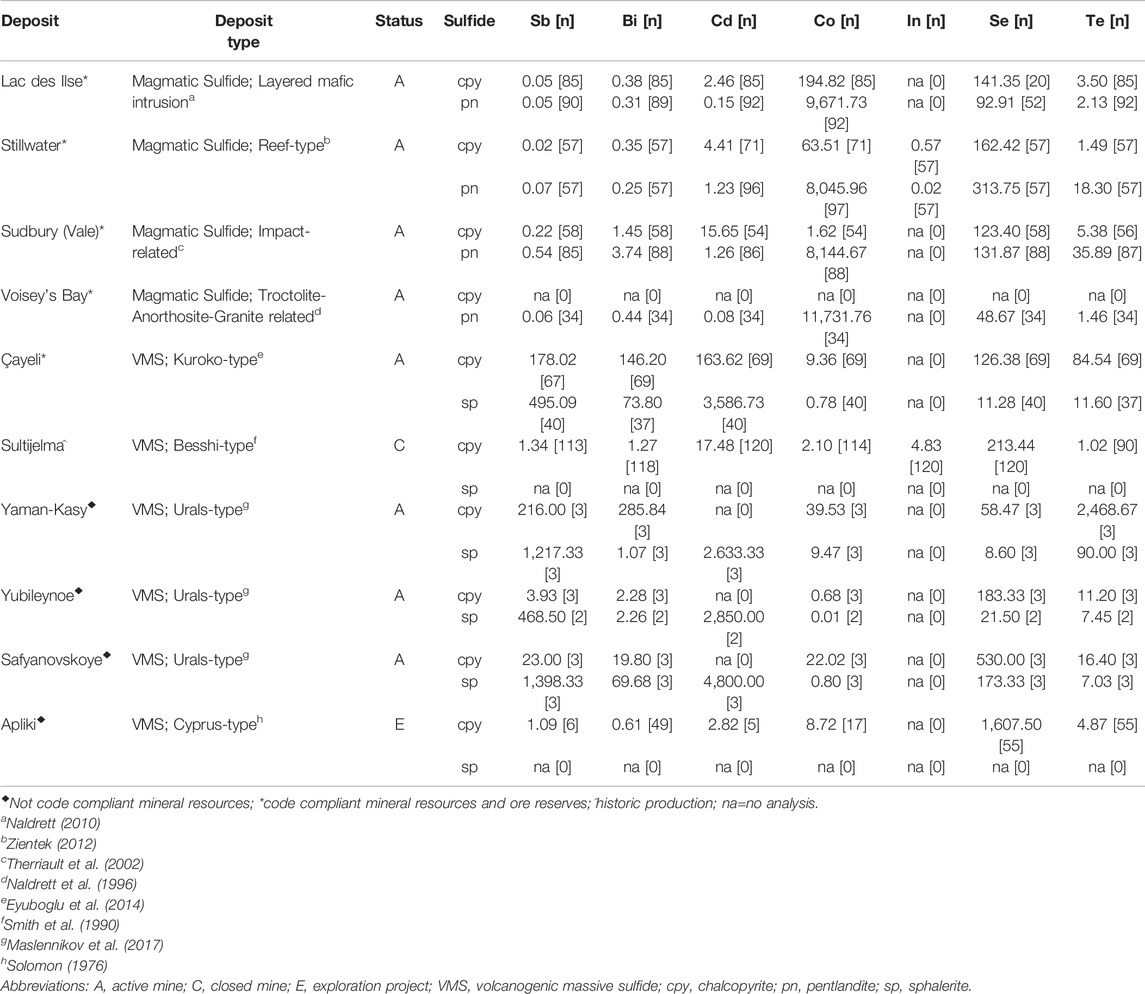
TABLE 3. Average byproduct critical element concentrations (ppm) in pentlandite, chalcopyrite and sphalerite from select magmatic sulfide and volcanogenic massive sulfide deposits.
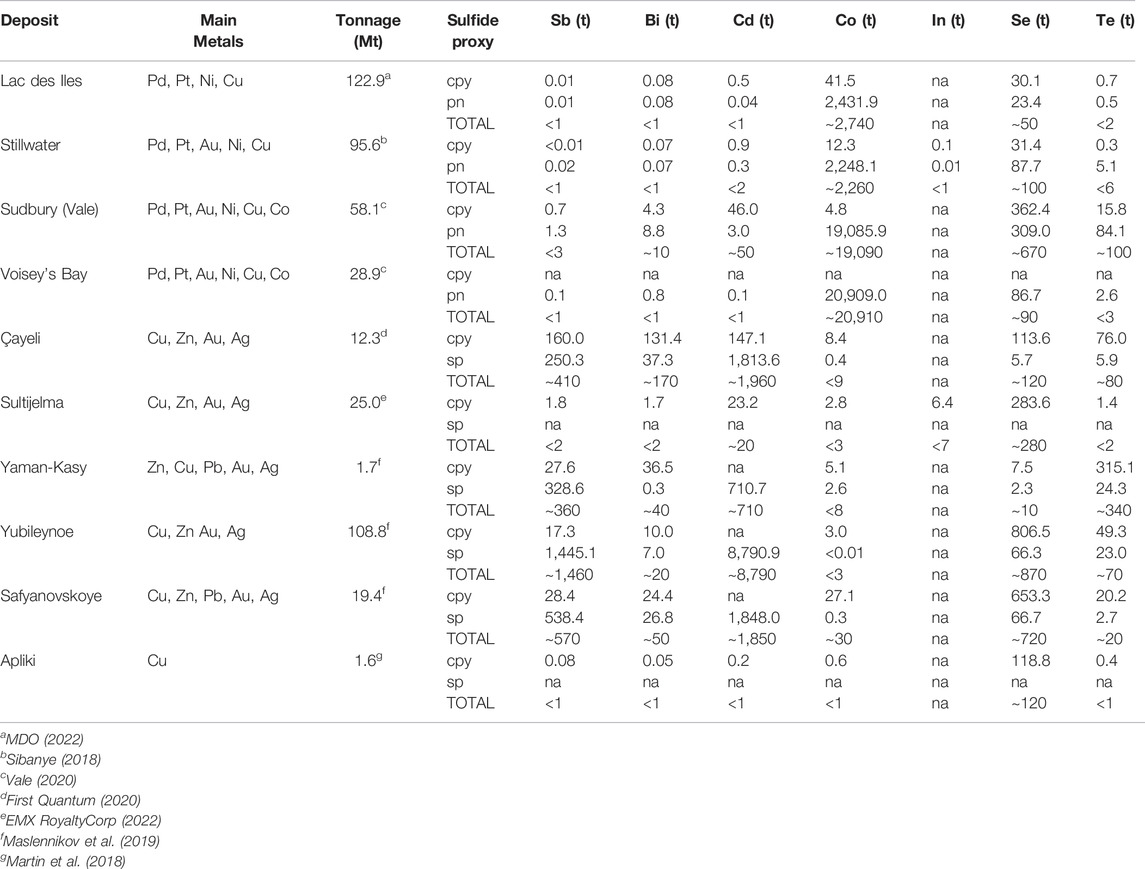
TABLE 4. Proxy byproduct critical element inventories (t) for select magmatic sulfide (n=4) and volcanogenic massive sulfide deposits (n=6).
Sulfide mineralogy also appears to be a significant factor for the deportment of these critical elements. Proxy inventories for magmatic sulfide deposits indicate that Co is significantly higher in pentlandite and when present Bi and Cd appear to preferentially be incorporated into pentlandite and chalcopyrite, respectively. In comparison Se is enriched in both chalcopyrite and pentlandite and Te, when present, appears to be preferentially incorporated into pentlandite rather than chalcopyrite. Proxy inventories for VMS deposits suggest that Sb and Cd are enriched in sphalerite compared to chalcopyrite whereas Bi and Co, when present, appear to preferentially be incorporated into chalcopyrite. Although Se and Te can be present in elevated concentrations in both chalcopyrite and sphalerite in VMS deposits, they both tend to be more enriched in chalcopyrite (e.g., Yubileynoe). All of the above relationships between inventory proxies and host minerals provide insights into potential opportunities to target specific areas of existing supply chains (e.g., chalcopyrite derived from VMS systems for Bi, Co, Se, and Te) to increase the supply (and security of supply) of these byproduct critical elements.
Although data to validate the proxy inventories are generally lacking, the Sudbury and Voisey’s Bay operations include grades for Co in their code compliant ore reserve estimates (Vale, 2020). Sudbury has 58.1 Mt of ore at 0.4% Co and Voisey’s Bay has 28.9 Mt of ore at 0.13% Co for total contained reserves of 23,240 t and 37,570 t of Co, respectively. Our Co proxy inventories for Sudbury and Voisey’s Bay are ∼19,090 t and ∼20,910 t, respectively, suggesting that the proxy approaches used here may yield conservative rather than overly optimistic estimates of contained Co (and likely other critical metals). For the Lac Des Iles and Stillwater deposits Co is not reported in the mineral resource and ore reserves estimates despite the proxies calculated in this study indicating that these magmatic sulfide deposits could contain significant Co in base metal sulfides; ∼2,740 and ∼2,220 t of Co, respectively (Table 4). All of these data demonstrate that the use of LA–ICP–MS data in the development of byproduct proxies for a variety of mineral deposits is a potentially effective way of targeting areas for enhanced byproduct production within existing or soon-to-be developed metal supply chains.
Discussion
The demand for technologies that improve energy storage and transport and deliver renewable energy solutions is increasing as a result of a worldwide movement to reduce CO2 emissions and mitigate climate change (Drexhage et al., 2017; Lee et al., 2020). Many of the raw materials required for these technologies are classified as critical (Grandell et al., 2016) and will likely require innovation in recycling (Fröhlich et al., 2017; Tabelin et al., 2021), mining (Lee et al., 2021), and the extraction of these materials from mine tailings as well as other mine waste reprocessing (Whitworth et al., 2022) in order to secure future supply. Another complementary solution to resolving these supply chain issues is to identify hidden byproduct critical element supply potentials within existing mining value chains and associated materials flows (Moats et al., 2021; McNulty et al., 2022).
Our analysis of compiled trace element LA–ICP–MS data from sulfide ore minerals provides a method to explore for byproduct critical elements supply potentials in existing supply chains. These data confirm that some magmatic sulfide deposits contain significant quantities of Co as well appreciable amounts of Se and Te. In comparison, some VMS deposits contain potentially recoverable quantities of Sb, Bi, Cd, Se, and Te. These elements are chalcophile in nature, which means they associate with sulfur and, as the above analysis shows, can be concentrated in a number of different base metal sulfide ore minerals. Table 2 outlines the range of concentrations of these critical elements in base metal sulfides. In some cases the variation in element concentration is significant and this is an important point of consideration for future work. In this study, LA–ICP–MS data were used to estimate the abundance of a suite of elements for which whole-rock assay data were not available. However, an ideal sampling and analytical procedure would utilize whole-rock assay results to target styles of mineralization with elevated critical elements of interest and then LA–ICP–MS analysis to determine the deportment of those critical elements within base metal sulfides. This integrated analytical approach could be completed on in situ ore types or on mineral processing and metal extraction products produced during mining to understand materials flows (i.e., elemental abundances in sulfide concentrates vs. mineral processing tailings) and the likelihood of recovery. In addition, the mineralogical deportment of critical element concentrations in sulfides needs to be reconciled. For instance, critical elements occurring as crystal lattice substitutions versus discrete mineral inclusions in sulfide minerals will likely have different geometallurgical behaviors, which will influence the liberation and concentration deportments of these potentially recoverable byproducts.
Recent research in critical element deportment has focused on assessing mine tailings (Araya et al., 2020) however; in general, there is a need to characterize ores in active mines. These data can also be linked (and provide input) to mineral exploration and the analysis of known mineralization. For instance, a rapid and sustained increase in the demand for Co, an element commonly used in Li-ion batteries, may perhaps warrant mineral exploration that focuses on magmatic sulfide systems that concentrate this element rather than those that are relatively Co-depleted. This compilation-based analytical approach allows the comparison of mineralization between mineral deposits with similar affinities, providing insight into the deportment and distribution of metals within mineralizing systems. This approach therefore outlines of areas for further investigation in terms of the temporal and spatial distribution and geological processes involved in the generation of deposits enriched in certain byproduct metals (Mudd et al., 2013; Holwell et al., 2019).
There is also a need to characterize extracted sulfide concentrates for elements beyond the current payable metals and penalty elements that are often defined in mine-smelter industry contracts or are deemed as environmentally dangerous (i.e., Cd; Scoullos et al., 2001; Lokanc et al., 2015). For instance, Te is rarely routinely measured in sulfide metal concentrates although other chalcophile elements that Te often naturally associates with, such as Bi and Se, are. As a result, there is very little code compliant Te data that can be used to estimate potential mineral resources (USGS, 2021) and although current Te is sourced as a byproduct of Cu, our analysis indicates that Ni and Zn sulfide metal concentrates could, in fact, contain significant recoverable quantities of this critical element, representing areas of current supply chains that remain unexamined for their Te potential. The above instance is not unique to Te, as many of the byproduct critical elements share the same fate, leading to a situation where a lack of data means there is an artificial scarcity of resource and therefore potentially a perceived rather than actual supply risk.
There are uncertainties involved in these approaches as mentioned above. Some of these can be reduced by increasing the number of LA–ICP–MS spot analysis and the number of mineralized samples analysed from a single mineral deposit. In addition, the incorporation of mass balance-type approaches where the deportment of a given metal or metal within all elements of a mineralized system, or at least within the appropriate stage of a mineralizing system, is analyzed before being compared to whole-rock concentrations to understand the deportment of selected metals within all minerals in a sample or mineralizing system (e.g., Frenzel et al., 2019). This would include the LA–ICP–MS analysis of gangue minerals that could potentially be hosts to byproduct metals within mineralizing systems. However, this approach is cost prohibitive, is time consuming, and can be problematic for a number of reasons, including the presence of small minerals that cannot effectively be analyzed using this approach given the diameter of the laser beam, a lack of suitable matrix matched standards with well-characterized byproduct contents (e.g., Yang et al., 2021), and the question of how representative sample materials are relative to an entire system. Even the use of techniques such as nano-secondary ion mass spectrometry (nano-SIMS) that analyze far smaller volumes of material cannot overcome issues such as a lack of matrix-matched standards and would also significantly increase costs. As such, an approach using previously determined or estimated (e.g., using Goldschmidt’s rules of substitution) partitioning behavior where data are missing or are unavailable may be one way of reducing but not eliminating this uncertainty, and would certainly be one potential approach to mass balancing. Despite these uncertainties, the data produced by these studies are certainly crucial for deportment studies and furthering our understanding of the materials flows of byproduct metals from mine to concentrate to smelter and refinery and the behavior of these metals during this flow (Figure 3; i.e., lost to waste, residing in anode slimes, produced as a byproduct, etc.).
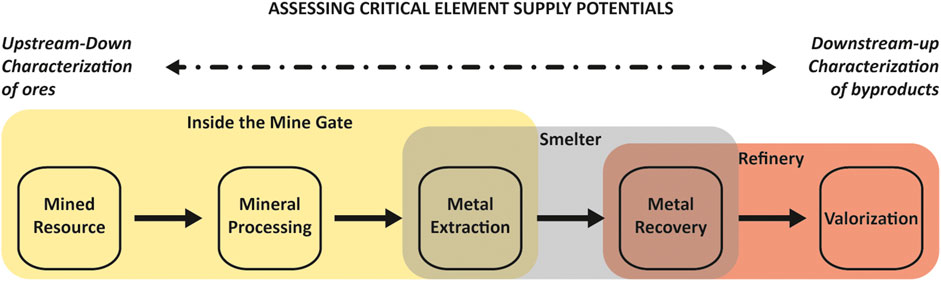
FIGURE 3. Generalized major materials flows by process in the mining-smelting-refining value chain with each process representing an opportunity to transport critical elements for downstream byproduct recovery as well as the challenge of integrating critical element deportment across multi-step, often global and multi-company value chains. The color polygons illustrate the potential, present-day silos between different operations in the value chain. Mineral processing liberates and concentrates economic metals and minerals from mined mineral resource (Lottermoser, 2010) for metal extraction, which collects metals from mineral concentrates and removes gangue minerals and metals (Jiang, 2017). Depending on the structure of the mining operation these two steps can occur inside the mine gate or both inside and outside the mine gate. Metal recovery purifies the mixture of extracted metals and compounds into a sought after chemical form (Spooren et al., 2020) with impurities, such as chalcophile critical elements, reporting to metallurgical wastes and residues that than can be recovered (i.e. valorization; Binnemans et al., 2015). Depending on the refinery both primary metal recovery and byproduct metal (i.e., Au) semi-metal (i.e., Te) or chemical compound (i.e., sulfuric acid) recovery will occur within an operation or metallurgical wastes/residuals are sold to an external operation for processing and recovery of byproducts (i.e., saleable copper anode slimes).
The approaches outlined above are a crucial step in the development of key target areas within existing supply chains for enhanced byproduct mineral and metal production and are vital for ensuring the security of supply of these commodities for the energy transition. These upstream-down studies that focus on the deportment of metals within mineral deposits are also ideal complements to downstream-up studies like McNulty et al. (2022), which examine smelter data and determine the likely source of certain byproducts and hence areas to target for enhanced critical byproduct production. All of this clearly demonstrates the value of a holistic approach to metal deportment, where upstream LA–ICP–MS studies and collations such as this study can be combined with downstream data to identify individual mineral deposit types, camps, and deposits that represent high- but as yet-unrealized sources of critical byproduct elements.
The next steps to fully utilize LA–ICP–MS analysis in assessing byproduct critical elements are: a) increase number of samples from defined main metal product ore domains; b) compare whole-rock assay results with LA–ICP–MS results on a sample by sample bases to understand mineralogical deportment of the critical elements; and c) expand this characterization from in situ ore studies to characterize critical element abundance in main product mineral processing and metal extraction produced materials.
Conclusion
LA–ICP–MS is a powerful tool for the in situ analysis of elements in sulfides, an approach that has been frequently used in ore deposit research to better understand the physiochemical conditions involved in different ore forming systems and the generation of mineral deposits. The results presented in this contribution serve as a first pass at exploring for critical element supplies associated with base metal sulfide ores with LA–ICP–MS datasets. Although the proxies developed in this study are compelling, additional datasets in combination with LA–ICP–MS analysis are needed to explore for and evaluate byproduct critical element supply potentials in existing base metal mineral deposits. Securing supply of byproduct critical metals is challenging because, unlike Cu, Ni or Zn, they are present in ores at low concentrations that often barely constitute payable byproducts at smelters, meaning that the presence of these critical metals is generally not quantified by mining operations. The integration of whole-rock assay data with trace element concentrations in sulfide ores provides a method to link critical element abundance with mineralogical deportment. The fact that there are few natural examples of economically feasible concentrations of Sb, Bi, Cd, Co, Se, and Te means that these energy critical metals need to be recovered from main metal production waste streams. This demonstrates that current approaches to evaluating and recovering these critical metals need to evolve. The realization of supply streams for byproduct critical elements requires ore characterization studies and the transfer of this orebody knowledge across global, multidisciplinary and multi-company supply chains. At this stage, proxies are vital to explore active mines for high priority starting points to initiate collaborative research to achieve short- and long-term critical element supply goals.
Data Availability Statement
The original contributions presented in the study are included in the article/Supplementary Material, further inquiries can be directed to the corresponding author.
Author Contributions
BM and SJ contributed to the conception and design of the study. BM organised the database. BM and SJ performed the analysis of the LA–ICP–MS data and developed the proxies. BM and SJ wrote sections of the manuscript and contributed to manuscript revision, read, and approved the submitted version.
Conflict of Interest
The authors declare that the research was conducted in the absence of any commercial or financial relationships that could be construed as a potential conflict of interest.
Publisher’s Note
All claims expressed in this article are solely those of the authors and do not necessarily represent those of their affiliated organizations, or those of the publisher, the editors and the reviewers. Any product that may be evaluated in this article, or claim that may be made by its manufacturer, is not guaranteed or endorsed by the publisher.
Acknowledgments
The authors would like to thank Mário Gonçalves, Guoguang Wang, and Stephanos Kilias for their constructive feedback, which improved an earlier version of this paper. Manuel Keith is also thanked for timely and efficient editorial handling.
Supplementary Material
The Supplementary Material for this article can be found online at: https://www.frontiersin.org/articles/10.3389/feart.2022.892941/full#supplementary-material.
References
Araya, N., Kraslawski, A., and Cisternas, L. A. (2020). Towards Mine Tailings Valorization: Recovery of Critical Materials from Chilean Mine Tailings. J. Clean. Prod. 263, 121555. doi:10.1016/j.jclepro.2020.121555
Austrade (2020). Australian Critical Minerals Prospectus. Available at: https://www.austrade.gov.au/australian-critical-minerals-prospectus.
Berry, J. B., Ferrada, J. J., Dole, L. R., and Moonis, A. (2001). Sustainable Recovery of By-Products in the Mining Industry.
Binnemans, K., Jones, P. T., Blanpain, B., Van Gerven, T., and Pontikes, Y. (2015). Towards Zero-Waste Valorisation of Rare-Earth-Containing Industrial Process Residues: A Critical Review. J. Clean. Prod. 99, 17–38. doi:10.1016/j.jclepro.2015.02.089
Drexhage, J. R., Arrobas, D. L. P., Hund, K. L., McCormick, M. S., and Jagabanta, N. (2017). The Growing Role of Minerals and Metals for a Low Carbon Future. Washington, DC, USA. doi:10.1596/28312
EMX Royalty Corp (2022). Sulitjelma. Available at: https://emxroyalty.com/asset-portfolio/norway/sulitjelma.
Eyuboglu, Y., Santosh, M., Yi, K., Tuysuz, N., Korkmaz, S., Akaryali, E., et al. (2014). The Eastern Black Sea-type Volcanogenic Massive Sulfide Deposits: Geochemistry, Zircon U-Pb Geochronology and an Overview of the Geodynamics of Ore Genesis. Ore Geol. Rev. 59, 29–54. doi:10.1016/j.oregeorev.2013.11.009
Frenzel, M., Bachmann, K., Carvalho, J. R. S., Relvas, J. M. R. S., Pacheco, N., and Gutzmer, J. (2019). The Geometallurgical Assessment of By-Products-Geochemical Proxies for the Complex Mineralogical Deportment of Indium at Neves-Corvo, Portugal. Min. Deposita 54, 959–982. doi:10.1007/s00126-018-0849-6
Fröhlich, P., Lorenz, T., Martin, G., Brett, B., and Bertau, M. (2017). Valuable Metals-Recovery Processes, Current Trends, and Recycling Strategies. Angew. Chem. Int. Ed. 56, 2544–2580. doi:10.1002/anie.201605417
Galley, A. G., Hannington, M. D., and Jonasson, I. R. (2007). “Volcanogenic Massive Sulphide Deposits,” in Mineral Deposits of Canada: A Synthesis of Major Deposit Types, District Metallogeny, the Evolution of Geological Province and Exploration Methods. Editor W. D. Goodfellow (St. John's Newfoundland, Canada: Geological Association of Canada, Mineral Deposits Division, Special Publication No. 5), 141–161.
Grandell, L., Lehtilä, A., Kivinen, M., Koljonen, T., Kihlman, S., and Lauri, L. S. (2016). Role of Critical Metals in the Future Markets of Clean Energy Technologies. Renew. Energy 95, 53–62. doi:10.1016/j.renene.2016.03.102
Holwell, D. A., Fiorentini, M., McDonald, I., Lu, Y., Giuliani, A., Smith, D. J., et al. (2019). A Metasomatized Lithospheric Mantle Control on the Metallogenic Signature of Post-subduction Magmatism. Nat. Commun. 10, 1. doi:10.1038/s41467-019-11065-4
Huston, D. (2021). Critical Minerals in Ores: Towards an Understanding of Critical Mineral Abundances in Mineral Deposits. Available at: https://www.youtube.com/watch?app=desktop&v=7btJ3rGc29Q.
Jiang, L. Y. (2017). “Metallurgy: Importance, Processes, and Development Status,” in Membrane-Based Separations in Metallurgy: Principles and Applications. Editor N. L. Lan Ying Jiang (Elsevier), 3–18. doi:10.1016/B978-0-12-803410-1.00001-3
Jowitt, S. M., and McNulty, B. A. (2021a). Battery and Energy Metals: Future Drivers of the Minerals Industry? Seg. Discov. 11, 11–18. doi:10.5382/2021-127.fea-01
Jowitt, S. M., and McNulty, B. A. (2021b). Geology and Mining: Mineral Resources and Reserves: Their Estimation, Use, and Abuse. Seg. Discov. 125, 27–36. doi:10.5382/Geo-and-Mining-11
Jowitt, S. M., Mudd, G. M., Werner, T. T., Weng, Z., Barkoff, D. W., and McCaffrey, D. (2018). “The Critical Metals: An Overview and Opportunities and Concerns for the Future,” in The Critical Metals: An Overview and Opportunities and Concerns for the Future (Littleton, Colorado: SEG Special PublicationSociety of Economic Geologists), 21, 25–38. doi:10.5382/sp.21.02
Krenev, V. A., Drobot, N. F., and Fomichev, S. V. (2015). Bismuth: Reserves, Applications, and the World Market. Theor. Found. Chem. Eng. 49, 532–535. doi:10.1134/S0040579515040120
Lee, J.-c., Kurniawan, K., Chung, K. W., and Kim, S. (2021). Metallurgical Process for Total Recovery of All Constituent Metals from Copper Anode Slimes: A Review of Established Technologies and Current Progress. Mater. Mat. Int. 27, 2160–2187. doi:10.1007/s12540-020-00716-7
Lee, J., Bazilian, M., Sovacool, B., Hund, K., Jowitt, S. M., Nguyen, T. P., et al. (2020). Reviewing the Material and Metal Security of Low-Carbon Energy Transitions. Renew. Sustain. Energy Rev. 124, 109789. doi:10.1016/j.rser.2020.109789
Lokanc, M., Eggert, R., and Redlinger, M. (2015). The Availability of Indium: The Present, Medium Term, and Long Term. Osti.Gov, 1–79. doi:10.2172/1327212
Lottermoser, B. G. (2010). Mine Wastes: Characterization, Treatment and Environmental Impacts. third ed. Berlin, Heideberg: Springer.
Martin, A. J., McDonald, I., MacLeod, C. J., Prichard, H. M., and McFall, K. (2018). Extreme Enrichment of Selenium in the Apliki Cyprus-type VMS Deposit, Troodos, Cyprus. Mineral. Mag. 82, 697–724. doi:10.1180/mgm.2018.81
Maslennikov, V. V., Ayupova, N. R., Safina, N. P., Tseluyko, A. S., Melekestseva, I. Y., Large, R. R., et al. (2019). Mineralogical Features of Ore Diagenites in the Urals Massive Sulfide Deposits, Russia. Minerals 9, 64–106. doi:10.3390/min9030150
Maslennikov, V. V., Maslennikova, S. P., Large, R. R., Danyushevsky, L. V., Herrington, R. J., Ayupova, N. R., et al. (2017). Chimneys in Paleozoic Massive Sulfide Mounds of the Urals VMS Deposits: Mineral and Trace Element Comparison with Modern Black, Grey, White and Clear Smokers. Ore Geol. Rev. 85, 64–106. doi:10.1016/j.oregeorev.2016.09.012
McNulty, B. A., and Jowitt, S. M. (2021). Barriers to and Uncertainties in Understanding and Quantifying Global Critical Mineral and Element Supply. iScience 24, 102809. doi:10.1016/j.isci.2021.102809
McNulty, B. A., Jowitt, S. M., and Belousov, I. (2022). The Importance of Geology in Assessing by- and Coproduct Metal Supply Potential; A Case Study of Antimony, Bismuth, Selenium, and Tellurium within the Copper Production Stream. Econ. Geol. in press. doi:10.5382/econgeo.4919
MDO (2022). Lac des Iles Mine. Available at: https://miningdataonline.com/property/55/Lac-des-Iles-Mine.aspx.
Moats, M., Alagha, L., and Awuah-Offei, K. (2021). Towards Resilient and Sustainable Supply of Critical Elements from the Copper Supply Chain: A Review. J. Clean. Prod. 307, 127207–127215. doi:10.1016/j.jclepro.2021.127207
Mudd, G. M., and Jowitt, S. M. (2014). A Detailed Assessment of Global Nickel Resource Trends and Endowments. Econ. Geol. 109, 1813–1841. doi:10.2113/econgeo.109.7.1813
Mudd, G. M., Weng, Z., Jowitt, S. M., Turnbull, I. D., and Graedel, T. E. (2013). Quantifying the Recoverable Resources of By-Product Metals: The Case of Cobalt. Ore Geol. Rev. 55, 87–98. doi:10.1016/j.oregeorev.2013.04.010
Naldrett, A. J., Keats, H., Sparkes, K., and Moore, R. (1996). Geology of the Voisey’s Bay Ni-Cu-Co Depsoits, Labrador, Canada. Explor. Min. Geol. 2, 169–179.
Naldrett, A. J. (2010). Secular Variation of Magmatic Sulfide Deposits and Their Source Magmas. Econ. Geol. 105, 669–688. doi:10.2113/gsecongeo.105.3.669
Nassar, N. T., Graedel, T. E., and Harper, E. M. (2015). By-product Metals Are Technologically Essential but Have Problematic Supply. Sci. Adv. 1, 1–10. doi:10.1126/sciadv.1400180
Pohl, W. L. (2020). Economic Geology: Principles and Practice. 2nd edition. Stutgart, Germany: Schweizerbart Science Publishers.
Quantum, First. (2020). Cayeli Reserves & Resources. Available at: https://www.first-quantum.com/English/our-operations/operating-mines/cayeli/reserves-and-resources/default.aspx.
Scoullos, M. J., Vonkeman, G. H., Thornton, I., and Makuch, Z. (2001). in Mecury - Cadmium - Lead: Handbook for Sustainable Heavy Metals Policy and Regulation. Editor M. J. Scoullos. 1st ed. (Springer)
Sibanye Stillwater (2018). Mineral Resources and Mineral Reserves. Available at: https://www.bafokengplatinum.co.za/reports/integrated-report-2019/pdf/royal-bafokeng-platinum-mmr.pdf.
Skinner, B. J. (1976). “A Second Iron Age Ahead?,” in Studies in Environmental Science. Editors P. A. Trudinger, and D. J. Swaine (Elsevier), 559–574. doi:10.1016/S0166-1116(08)71071-9
Smith, A. D., Farquhar, J. S., Smith, P., and Smith, P. (1990). Geochemistry of Ordovician Keli Group Basalts Associated with Besshi-type Cu-Zn Deposits from the Southern Trondheim and Sulitjelma Mining Districts of Norway. Mineral. Deposita 25, 15–24. doi:10.1007/bf03326379
Solomon, M. (1976). “"Volcanic" Massive Sulphide Deposits and Their Host Rocks - a Review and an Explanation,” in Handbook of Stratabound and Strataform Ore Deposits. Editor K. H. Wolf (Amsterdam, Netherlands: Elsevier Scientific Publishing Company), 21–54. doi:10.1016/b978-0-444-41406-9.50006-3
Spooren, J., Binnemans, K., Björkmalm, J., Breemersch, K., Dams, Y., Folens, K., et al. (2020). Near-zero-waste Processing of Low-Grade, Complex Primary Ores and Secondary Raw Materials in Europe: Technology Development Trends. Resour. Conservation Recycl. 160, 104919. doi:10.1016/j.resconrec.2020.104919
Sykes, J. P., Wright, J. P., and Trench, A. (2016). Discovery, Supply and Demand: From Metals of Antiquity to Critical Metals. Appl. Earth Sci. 125, 3–20. doi:10.1080/03717453.2015.1122274
Tabelin, C. B., Park, I., Phengsaart, T., Jeon, S., Villacorte-Tabelin, M., Alonzo, D., et al. (2021). Copper and Critical Metals Production from Porphyry Ores and E-Wastes: A Review of Resource Availability, Processing/recycling Challenges, Socio-Environmental Aspects, and Sustainability Issues. Resour. Conservation Recycl. 170, 105610. doi:10.1016/j.resconrec.2021.105610
Therriault, A. M., Fowler, A. D., and Grieve, R. A. F. (2002). The Sudbury Igneous Complex: A Differentiated Impact Melt Sheet. Econ. Geol. 97, 1521–1540. doi:10.2113/gsecongeo.97.7.1521
Werner, T. T., Mudd, G. M., and Jowitt, S. M. (2017). The World's By-Product and Critical Metal Resources Part III: A Global Assessment of Indium. Ore Geol. Rev. 86, 939–956. doi:10.1016/j.oregeorev.2017.01.015
Whitworth, A. J., Forbes, E., Verster, I., Jokovic, V., Awatey, B., and Parbhakar-Fox, A. (2022). Review on Advances in Mineral Processing Technologies Suitable for Critical Metal Recovery from Mining and Processing Wastes. Clean. Eng. Technol. 7, 100451. doi:10.1016/j.clet.2022.100451
Wohlgemuth-Ueberwasser, C. C., and Jochum, K. P. (2015). Capability of fs-LA-ICP-MS for Sulfide Analysis in Comparison to ns-LA-ICP-MS: Reduction of Laser Induced Matrix Effects? J. Anal. At. Spectrom. 30, 2469–2480. doi:10.1039/c5ja00251f
Yang, W., Zhao, H., Zhang, W., Luo, T., Li, M., Chen, K., et al. (2021). A Simple Method for the Preparation of Homogeneous and Stable Solid Powder Standards: Application to Sulfide Analysis by LA-ICP-MS. Spectrochim. Acta Part B At. Spectrosc. 178, 106124. doi:10.1016/j.sab.2021.106124
Keywords: byproduct proxies, critical elements, LA-ICP-MS, magmatic sulfide deposits, volcanogenic massive sulfide (VMS) deposits
Citation: McNulty BA and Jowitt SM (2022) Exploration for Byproduct Critical Element Resources: Proxy Development Using a LA–ICP–MS Database. Front. Earth Sci. 10:892941. doi: 10.3389/feart.2022.892941
Received: 09 March 2022; Accepted: 19 April 2022;
Published: 12 May 2022.
Edited by:
Manuel Keith, University of Erlangen Nuremberg, GermanyReviewed by:
Mário Gonçalves, University of Lisbon, PortugalGuoguang Wang, Nanjing University, China
Stephanos P. Kilias, National and Kapodistrian University of Athens, Greece
Copyright © 2022 McNulty and Jowitt. This is an open-access article distributed under the terms of the Creative Commons Attribution License (CC BY). The use, distribution or reproduction in other forums is permitted, provided the original author(s) and the copyright owner(s) are credited and that the original publication in this journal is cited, in accordance with accepted academic practice. No use, distribution or reproduction is permitted which does not comply with these terms.
*Correspondence: Brian A. McNulty, YnJpYW4ubWNudWx0eUB1bmx2LmVkdQ==