- 1Department of Earth and Environmental Sciences, Ludwig-Maximilians-Universität München, Munich, Germany
- 2Department of Earth and Atmospheric Sciences, University of Houston, Houston, TX, United States
The tectonics of East Asia are notoriously complex. Consisting of an intricate patchwork of microplates and accreted terranes, even the Cenozoic tectonic history of the region remains controversial, and many differing reconstructions have been proposed. While the exact kinematics remain poorly constrained, it is generally accepted that East Asia has been characterised by a long history of subduction and downwelling. However, numerous geological observations, at a first glance, appear to lie in stark contrast to this history. For example, seismically slow anomalies in the uppermost mantle are extensive in this region and coincide spatially with widespread intraplate volcanism since the latest Paleogene, which is seemingly at odds with the cold upper mantle and downwelling flow expected from a history of subduction. Here, we propose a solution to this paradox, in which hot asthenospheric material flows through the slab window opened by the subduction of the Izanagi-Pacific ridge during the early Cenozoic, passing from the Pacific domain into East Asia. To investigate this hypothesis, we compare several independent geological observations to the asthenospheric flow predicted by a suite of recently published global mantle circulation models. The timing and location of intraplate volcanism is compared with the predicted distribution of this hot material through time, while observations linked to uplift and erosion are compared to the changes in dynamic topography that it induces. These include the widespread late Eocene–Oligocene sedimentary hiatus in far eastern China and the regional erosion of the South China Block since the Miocene inferred from Apatite Fission Track Thermochronology studies. The westward influx of hot asthenospheric material is a robust feature in the models, being predicted regardless of the implemented Cenozoic tectonic reconstruction. However, we find that a small Philippine Sea Plate that overrides a marginal “vanished ocean” during the late Cenozoic provides an optimal fit to the geological observations considered. Flow of hot asthenospheric material through gaps in subduction has the potential to significantly affect the geodynamic and geologic history of backarc and hinterland regions, and might have been a recurring phenomenon throughout Earth’s history. However, further research will be required in order to establish this.
1 Introduction
East Asia lies at a key junction between the converging Pacific (PAC), Eurasian (EUR), and Indo-Australian (IA) plates. Characterised by several microplates, marginal seas, accreted terranes, and zones of diffuse deformation, it is generally considered to be one of the most (if not the most) tectonically complex regions on Earth. In addition to this, reconstructing even the Cenozoic tectonic history of the region has been hampered by the existence of potentially numerous small plates which have been completely subducted into the mantle, or plates which have been isolated by subduction zones from global plate circuits (Hall and Spakman, 2015; Wu et al., 2016). Consequently, extracting the first-order details of the Cenozoic tectonic history of this region from geological and seismological evidence remains an ongoing endeavour, and a variety of differing reconstructions have been proposed. Nevertheless, there exists a general consensus that the long-term convergence of PAC, EUR, and IA must have been accommodated by widespread subduction and downwelling throughout East Asia.
Given this consensus, it is understandable that geodynamic studies (at least on inter-regional scales) have focussed on downwelling slabs (e.g., Zahirovic et al., 2016), or their influence at the surface in terms of dynamic subsidence and rebound (e.g., Cao et al., 2018). However, numerous observations appear to suggest that a widespread presence of hot material in the uppermost mantle is also essential in describing the Cenozoic dynamics of this region. First of all, while global tomographic models have been crucial in identifying the distribution of subducted slabs in the East Asian mantle (e.g., Wu et al., 2016), these models also consistently reveal an extensive network of seismically slow anomalies at asthenospheric depths (e.g., Li et al., 2008; Schaeffer and Lebedev, 2013; Ma J. et al., 2019). The region may therefore be tentatively interpreted as being characterised by a largely hot upper mantle in the present day. This interpretation is corroborated by studies of present-day residual (i.e., dynamic) topography [see the recent review of Hoggard et al. (2021)], which have consistently found swells of present-day dynamic uplift throughout East Asia. Given these observations, the immediate questions arise as to when, and how, this present-day state of the mantle has developed—in a region dominated by subduction and downwelling. Regarding the first of these questions, intraplate volcanism has been widespread in East Asia since the latest Paleogene (Figure 1), potentially hinting at the arrival of this material during the late Cenozoic. The global connection between Neogene intraplate volcanism and seismically slow anomalies in the uppermost mantle was recently made explicit by the work of Ball et al. (2021), within which East Asia stood out as a prominent feature in the global picture. However, the question of how this state arose on an inter-regional scale in East Asia has not yet been tackled.
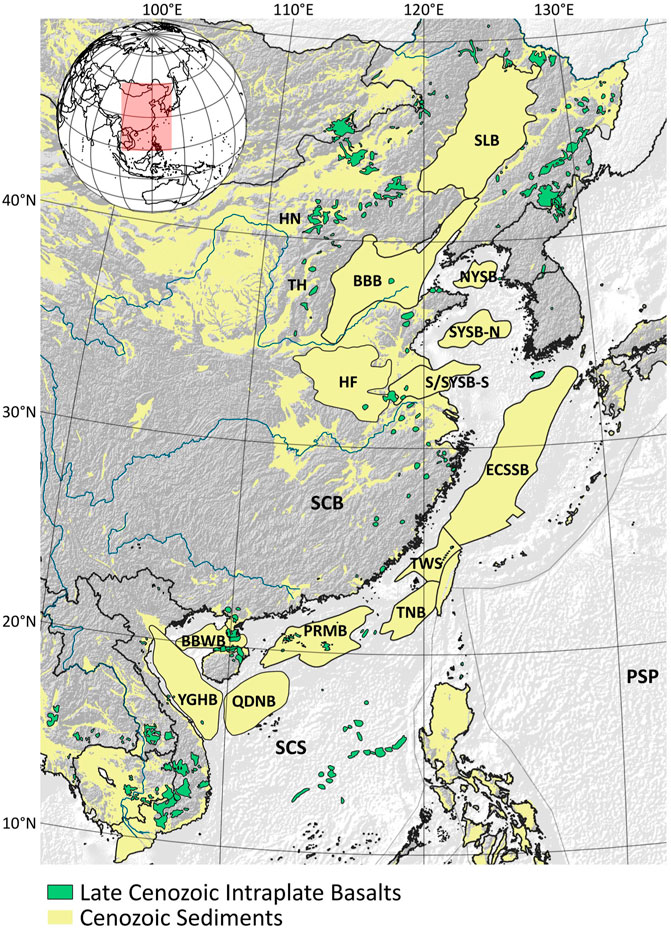
FIGURE 1. Map of the study area showing the distribution of late Cenozoic intraplate basalts considered in this study (in green) and Cenozoic sediments (in yellow), together with the outline of the main sedimentary basins mentioned in the text. Notice the scarcity of Cenozoic sediments in the South China Block. SLB, Songliao Basin; BBB, Bohai Bay Basin; NYSB, North Yellow Sea Basin; SYSB-N, South Yellow Sea Basin Northern Depression; S/SYSB-S, Subei/South Yellow Sea Basin Southern Depression; HF, Hefei Basin; ECSSB, East China Sea Shelf Basin; TWS, Taiwan Strait; TNB, Tainan Basin; PRMB, Pearl River Mouth Basin; BBWB, Beibuwan Basin; QDNB, Qiongdongnan Basin; YGHB, Yinggehai Basin; HN, Hannuoba Basalts; TH, Taihang Basalts. SCB, South China Block; SCS, South China Sea; PSP, Philippine Sea Plate.
Here, we aim to reconcile the subduction-dominated environment of East Asia with its inferred hot upper mantle in the present day. We propose and investigate a new mechanism by which hot asthenospheric material from the Pacific domain can migrate into this region through pressure- and plate-driven asthenospheric flow. To test our new hypothesis, we compile geological evidence of intraplate volcanism and past changes in dynamic topography in East Asia from published literature. These observations are then compared to the asthenospheric flow predicted by the suite of global mantle circulation models published by Lin et al. (2020). The purpose of this paper is therefore twofold. First and foremost, we present a new model for the Cenozoic geodynamic development of East Asia, and substantiate it by connecting independent mantle circulation models with a wide variety of geological observations. Secondly, the comparisons made between these models and the compiled geological evidence are used to provide new constraints on the uncertain Cenozoic tectonics of the region. A map of the relevant regions and geological features for this study is shown in Figure 1 (compiled from Steinshouer et al., 1999; Chung, 1999; Ho et al., 2003; Tang et al., 2006; Choi et al., 2006; Sun et al., 2010; Gong and Chen, 2014; Liu et al., 2001; Yan Q. et al., 2018; Zheng et al., 2019).
The remainder of our paper is structured as follows. In Section 2, we introduce our hypothesis, and the inferred Mesozoic–Cenozoic dynamics on which it is based. In Section 3, we introduce the relevant tectonic reconstructions of the study region and their implementation in the circulation models of Lin et al. (2020). In Section 4, we compile four independent geological observations in East Asia, related to both intraplate volcanism and dynamic topography, and compare these with the model predictions of Lin et al. (2020). We discuss these comparisons, and bring them together under our hypothesis, in Section 5. A discussion on the limitations of the models of Lin et al. (2020) is also included. Finally, we summarise our conclusions in Section 6.
2 Asthenospheric flow through the Izanagi-Pacific slab window
The low-viscosity asthenosphere has traditionally been regarded as a passive lubricating layer to facilitate plate motion (Chase, 1979), but mounting evidence now suggests that active pressure-driven flow plays a significant role in asthenospheric dynamics. Theoretical (Weismüller et al., 2015) and observational (White and Lovell, 1997; Hartley et al., 2011; Colli et al., 2014; Parnell-Turner et al., 2014; Chen et al., 2021) constraints have found pressure-driven flow velocities of ∼15–20 cm/year, which influence the motion of the overlying plates (e.g., Colli et al., 2014; Chen et al., 2021; Stotz et al., 2021). Flow in the asthenosphere is therefore thought to consist of a top-down, plate-driven component (i.e., Couette flow), and a component induced by pressure gradients in the asthenosphere (i.e., Poiseuille flow) (Höink and Lenardic, 2008; Höink and Lenardic, 2010; Höink et al., 2011; Höink et al., 2012). Given the aforementioned pressure-driven velocities, and the additional plate-driven component, it becomes apparent that asthenospheric material could potentially be transported over significant (e.g., ∼4,500 km) distances within relatively short (∼30 Ma) geological timescales. Combined with the late Mesozoic–Cenozoic dynamics of East Asia and the Pacific introduced below, these forms of flow provide a mechanism by which hot asthenospheric material could have migrated into East Asia during the Cenozoic.
Magnetic lineations in the western Pacific (e.g., Müller et al., 2016, and references therein) show that during the Mesozoic the Pacific plate had a conjugate plate to the northwest, termed the Izanagi plate by Woods and Davies (1982). The Mesozoic subduction of this plate has been deduced based on subduction-induced volcanism in East Asia (e.g., Charvet et al., 1994), while a break in volcanism during 56–46 Ma in Northeast Asia (Wu and Wu, 2019) reveals the subduction of the Izanagi-Pacific ridge. The slab window opened by the subduction of this ridge would have provided a pathway for Pacific asthenosphere to flow into East Asia. A detailed reconstruction of Mesozoic–Cenozoic western Pacific tectonics, however, is hindered by the fact that most of the lithosphere that was flooring the western Pacific has since been subducted, leaving large areas with unconstrained kinematics (Müller et al., 2016). The unconstrained area can in principle be filled by a single, large Izanagi plate, inducing margin-wide ridge subduction (e.g., Seton et al., 2012). However, the accretion of intra-oceanic arcs along the Eurasian margin during the Mesozoic suggests that the large Izanagi plate may in fact have been several smaller plates, with intervening intra-oceanic subduction zones providing the accreted material (see, e.g., Lin et al., 2021, and references therein). These more complex reconstructions feature even more pathways for Pacific asthenosphere to flow into Eurasia while implying the same first-order effects on asthenospheric dynamics. We therefore centre our work on the commonly implemented large Izanagi plate and leave the implications of more complex scenarios for future investigation.
While East Asia is noted for its large-scale mantle downwelling, the Pacific domain is well-known for large-scale thermal upwelling. This is evidenced by the numerous plume-fed hotspots emanating from the Pacific LLSVP (e.g., French and Romanowicz, 2015), the influence of which at the surface is revealed by dynamic uplift inferred from tomographically-constrained geoid modelling (e.g., Richards and Hager, 1984; Richards et al., 1988) and residual topography measurements (e.g., Hoggard et al., 2017). Evidence from plume-sourced basalts found in accreted complexes on the Eurasian margin shows that this state of large-scale thermal upwelling has been present in the Pacific domain since Cretaceous times (Safonova and Santosh, 2014). One may therefore infer that the Pacific asthenosphere has been fed by hot material from the lowermost mantle since at least this time. Asthenospheric material is naturally driven from high-pressure upwellings to low-pressure subduction zones by a combination of pressure-driven Poiseuille flow and plate-driven Couette flow (Höink and Lenardic, 2010; Colli et al., 2018). Combined, these two effects provide a mechanism for the long-term transport of hot asthenospheric material to the western Pacific margin during the Cretaceous. The mantle below Eurasia has probably been characterised by even lower pressures than the western Pacific, induced by large-scale mantle downwelling (e.g., Izanagi slab, Neo- and Meso-Tethys slabs) and by the trench–arc trending pressure gradient which arises naturally by slab-induced mantle flow during subduction (sometimes referred to as trench suction, see Turcotte and Schubert, 2002). During the Mesozoic, asthenospheric flow between the Pacific/Panthalassa domain and Eurasia was likely blocked by the subducting Izanagi plate. Following the subduction of the Izanagi-Pacific ridge, however, hot material may then have flowed through the opened slab window into East Asia, and been driven further into this region by downwelling slabs during the Cenozoic. Indeed, this east–west Poiseuille flow following the subduction of the Izanagi-Pacific ridge has recently been noted, and invoked as a cause of the flat-slab subduction style of the Pacific plate (Peng et al., 2021).
Our hypothesis can be summarised as follows. During the Izanagi subduction, hot asthenospheric material was built up in the westernmost Pacific through a combination of plume- and plate-driven flow. Following the subduction of the Izanagi-Pacific ridge during the early Cenozoic, this material was then driven into East Asia by pressure-driven Poiseuille flow, induced by the large-scale mantle downwelling in this region. The migration of this material into East Asia during the late Cenozoic provides a new explanation for its inferred hot upper mantle in the present day (Section 1). A cartoon illustrating this hypothesis is shown in Figure 2.
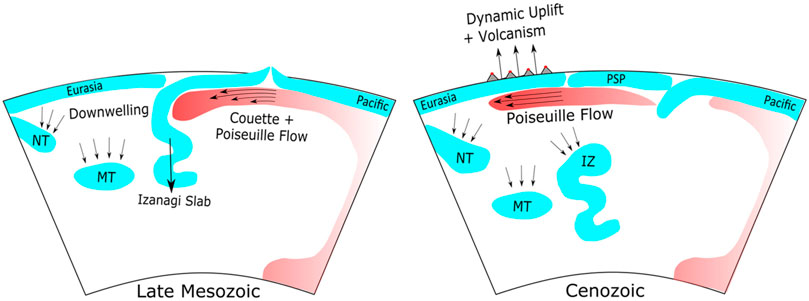
FIGURE 2. Cartoon illustrating the proposed build-up of Pacific asthenospheric material in the far western Pacific during the late Mesozoic through pressure- and plate-driven flow, and subsequent pressure-driven influx into East Asia through the Izanagi-Pacific slab window during the Cenozoic. NT, Neotethys slab; MT, Mesotethys slab; IZ, Izanagi slab; PSP, Philippine Sea Plate.
3 Global mantle circulation models
Mantle circulation models have used kinematic reconstructions of past plate motion as a key input to overcome the initial condition problem of mantle convection. This, in turn, has allowed geodynamically-derived mantle states to be compared against a variety of geophysical and geological observations (Bunge et al., 1998; Schuberth et al., 2009a; Schuberth et al., 2009b; Nerlich et al., 2016; Zahirovic et al., 2016). But the reconstructed mantle states depend critically on the input plate kinematic model (Colli et al., 2018; Lin et al., 2020) and, as discussed above, the constant loss of ocean floor means that significant uncertainties still exist in the kinematics of subduction-dominated regions. However, advances in the resolution of seismic tomography have made it possible to “image” subducted slabs in the mantle (e.g., Li et al., 2008), providing access to new sub-surface information with which to constrain past tectonics. This has led to the development of new “slab unfolding” methods (Wu et al., 2016; Wu and Suppe, 2018), which make use of this new window into past subduction. Slabs are identified as seismically fast anomalies, and are then unfolded, and projected to their implied extent at the surface. The surface distribution of the imaged slabs can then be used to improve upon surface-based reconstructions. These methods have been applied in East Asia (Wu et al., 2016), and have shed new light on the Cenozoic history of subduction in this region. Of particular importance here are the proto-South China Sea (PSCS) and the Philippine Sea Plate (PSP), as the slab unfolding method has been used to develop new fully-kinematic plate models specifically for these plates.
3.1 Plate motion models
The PSCS, which has now been completely removed from the surface, is inferred to have subducted during the opening of the present-day South China Sea (SCS). This has been argued based both on a gap in tectonic reconstructions prior to this opening (e.g., Holloway, 1982) and the observation of fast slab-like anomalies below this region in tomographic models (e.g., Hall and Spakman, 2015). However, the details of this subduction remain uncertain. Traditionally, the PSCS has been envisioned as subducting southward below Borneo (e.g., Hall, 2002; Seton et al., 2012; Zahirovic et al., 2014). Based on slab-unfolding methods, Wu and Suppe (2018) instead argue that the PSCS subducted both northward and southward during the opening of the SCS, due to both the position of the subducted PSCS slab in the mantle and its subhorizontal orientation.
Unlike the PSCS, the PSP is still present at the Earth’s surface (Figure 1). But its Cenozoic history remains uncertain due to its isolation from global plate circuits by surrounding subduction zones (see discussion in Wu et al., 2016). Due to this, vast differences exist in the proposed PSP reconstructions. A common view is that the PSP was much larger in the past, and began its current subduction along the Eurasian margin before 30 Ma (e.g., Seno and Maruyama, 1984; Xu et al., 2014; Zahirovic et al., 2014). On the other hand, based on the slab anomalies underlying the PSP in the present day, Wu et al. (2016) argue for a much smaller historic PSP, which begins subducting along the Eurasian margin at ∼16 Ma. In this reconstruction, the spatial gap produced by the smaller PSP is made up by the now fully-subducted “East Asian Sea” (EAS) slabs, which have been inferred based on the imaged slabs to have subducted southward below the PSP. Therefore, under this viewpoint, the smaller PSP overrides the southward-subducting EAS slabs as it migrates northward towards its present day position. For a more detailed review of the reasoning behind these differing reconstructions, we refer the reader to the discussion of PSCS reconstructions in Lin et al. (2020) and the discussion of the PSP and EAS slabs in Wu et al. (2016) and Zahirovic et al. (2014).
3.2 Model implementations
The study of Lin et al. (2020) investigated the mantle flow predicted by differing tectonic reconstructions in East Asia. Reconstructions were assimilated as surface velocity boundary conditions in the global mantle convection code TERRA (Bunge and Baumgardner, 1995). This allows geological information about past plate motion to enter the flow, as downwellings develop at locations of plate convergence (e.g., Bunge et al., 2002). Mantle convection was modelled by solving the conservation equations for mass, momentum, and energy in the truncated anelastic liquid approximation (Jarvis and McKenzie, 1980a). Lithospheric thickness variations were generated self-consistently by solving the conservation equations with tectonic velocities and a surface temperature TS = 300 K as boundary conditions. The computational domain was discretised on a regular grid based on the icosahedron, with ∼80 million grid points and a minimum resolution of ∼25 km. The mantle was heated from below and from within with a CMB temperature TCMB = 4,200 K and a radiogenic heating rate of 6 × 10–12 W⋅kg−1. The viscosity was assumed to be Newtonian and depends on temperature (T) and depth (d) as
where V∗ = 3.976 and E∗ = 4.610 are non-dimensional constants controlling the degree of depth and temperature dependence respectively. An increasing viscosity with depth is imposed by the factor exp[V∗d/(RE − RCMB)], in which the depth is normalised by the mantle thickness RE − RCMB. The reference viscosity is η0 = 1022 Pa⋅s and is multiplied with a radial pre-factor A(d) in order to impose a low-viscosity asthenosphere. The resulting maximum, minimum, and average radial viscosity profiles are reported in Supplementary Figure S1, together with the total radial pre-factor η0A(d). This model rheology leads to two-sided subduction, and one-sided subduction was not forced by imposing either dipping weak plate boundaries or a prescribed dipping slab thermal structure. Slab material therefore sinks vertically unless carried passively by mantle flow.
Four different tectonic reconstructions (Models 1a, 1b, 2a & 2b) were implemented, which are designed to test different end-members of the proposed reconstructions of the PSP and the PSCS introduced above. Models labelled “a” (Models 1a & 2a) implement a southward PSCS subduction, while models labelled “b” (Models 1b & 2b) implement a double-sided PSCS subduction. Models labelled “1” (Models 1a & 1b) implement a large PSP in the style of Zahirovic et al. (2014), while models labelled with a “2” (Models 2a & 2b) implement a simplified form of the slab-unfolded model of Wu et al. (2016), featuring a small PSP that overrides a single “vanished ocean” (VO) plate. In these reconstructions, the small PSP overrides the VO plate and begins its subduction along the Eurasian margin at ∼12 Ma. The naming scheme for these models is summarised in Table 1. Figure 3 shows the reconstructed plate motions at 30 Ma, highlighting their key differences. The primary aim of Lin et al. (2020) was to test the present-day mantle heterogeneity predicted under each reconstruction against seismic tomography. Model 2b led to a present-day mantle state which had the highest correlation with seismic tomography, and so is our preferred reconstruction.
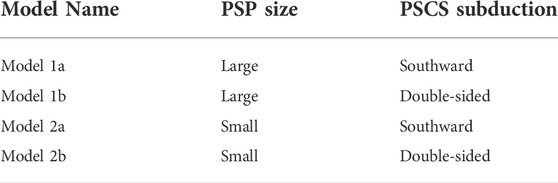
TABLE 1. Model naming scheme based on the implemented Cenozoic tectonic reconstruction following Lin et al. (2020).
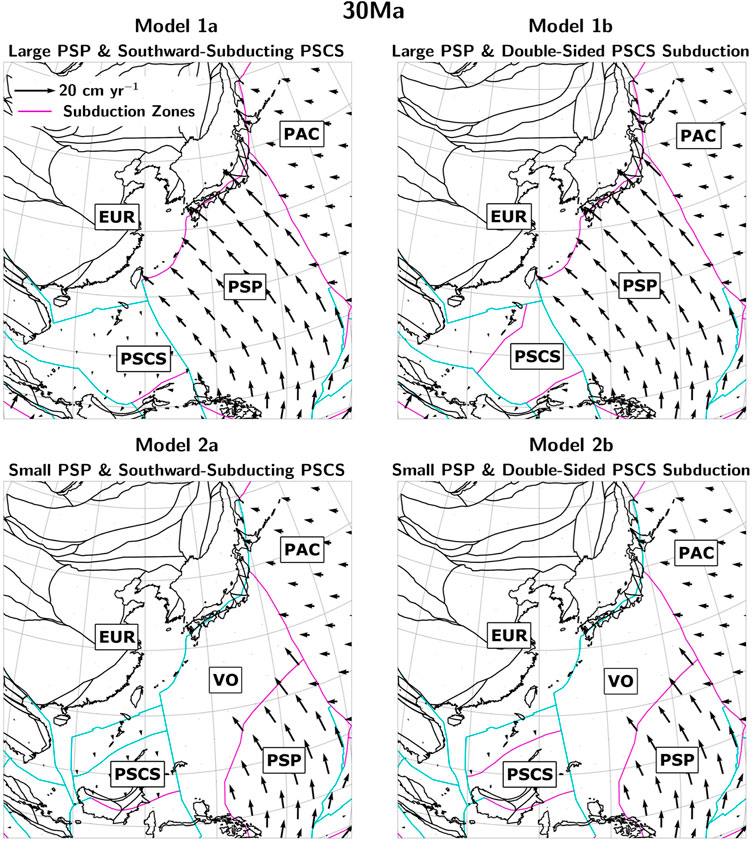
FIGURE 3. 30 Ma time-step of the four late Cenozoic tectonic reconstructions used in the mantle circulation models of Lin et al. (2020). Black arrows denote plate velocities in a global moving hotspot reference frame (Matthews et al., 2016). Purple lines show the location of subduction zones, while other plate margins are drawn in light blue. EUR, Eurasian Plate; PAC, Pacific Plate; PSP, Philippine Sea Plate; VO, Vanished Ocean Plate; PSCS, proto-South China Sea Plate.
Prior to the assimilation of these reconstructions at 30 Ma (Models 1a & 1b) and 45 Ma (Models 2a & 2b), the reconstruction of Matthews et al. (2016) is used as a surface boundary condition on velocities since 410 Ma. A reference model implements the reconstruction of Matthews et al. (2016) for the entire 410–0 Ma timespan. All models therefore implement the subduction of a large Izanagi plate during the Mesozoic and the subduction of the Izanagi-Pacific ridge at ∼55 Ma. Running these models since the Paleozoic means that an Earth-like convective planform is allowed to develop, generating vigorous plumes in the Pacific domain. Thus, a realistic hot upper mantle arises in this region during the Mesozoic. Along with the implemented Izanagi subduction and downwelling slabs in East Asia, this provides a realistic scenario in which to test our asthenospheric flow hypothesis.
The asthenospheric build-up and subsequent influx proposed in Section 2 arises under all considered reconstructions. Transects of the reference model showing the full history of this influx from 60 to 0 Ma can be found in Supplementary Figures S2–S8. Hot asthenospheric material is driven westward through the Izanagi-Pacific slab window from ∼50–30 Ma, subsequently flowing laterally below East Asia during the late Cenozoic. Figure 4 shows four snapshots from the evolution of our preferred Model 2b, which implements a double-sided subduction of the PSCS and a smaller PSP. While this influx occurs under all considered tectonic reconstructions, the differences between the plate motion models described above affect how much hot material flows below East Asia, which parts of the asthenosphere are occupied by slabs, and the induced late Cenozoic dynamic topography. For further details of these models we refer the reader to Lin et al. (2020).
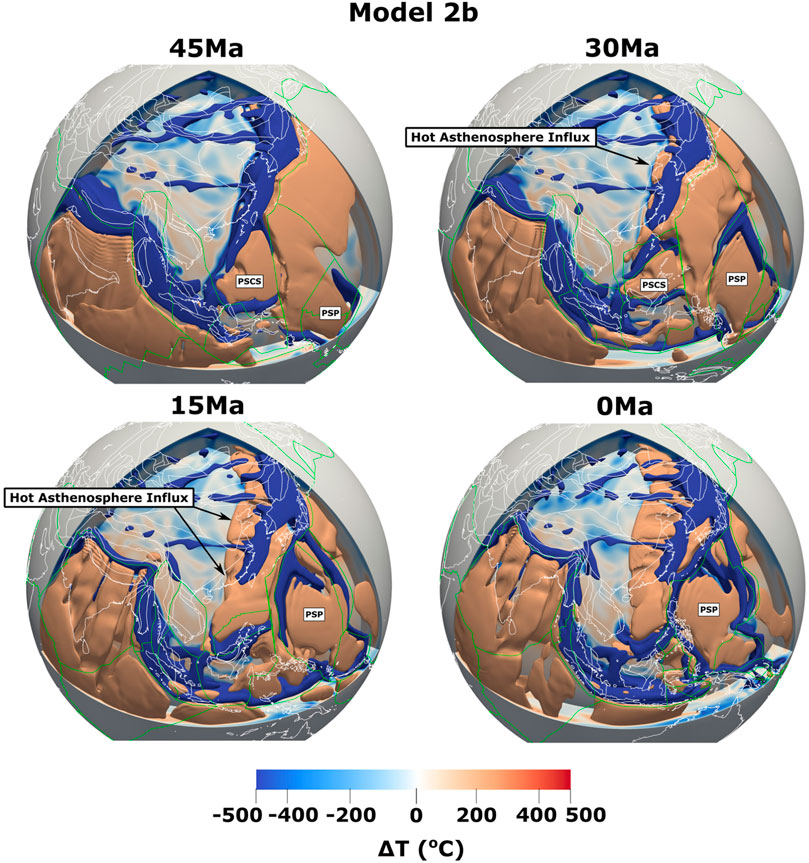
FIGURE 4. Four snapshots at 45, 30, 15, and 0 Ma from the upper mantle flow history of our preferred Model 2b (Lin et al., 2020) showing the Cenozoic influx of hot asthenospheric material through the Izanagi-Pacific slab window and its subsequent migration below East Asia. Isosurfaces of anomalous temperature (with respect to the mean radial temperature profile of the model) of −500°C and +200°C have been extracted in the upper mantle in order to highlight the downwelling slabs and influx of hot Pacific material. PSP, Philippine Sea Plate; PSCS, proto-South China Sea Plate.
4 Comparisons with the geological record
4.1 Intraplate volcanism
Intraplate basaltic magmatism has been widespread in East Asia since the latest Paleogene (Figure 1), spanning from the well-known eruptions in Indochina and Hainan (e.g., Yan et al., 2008), throughout the South China Block (SCB) (e.g., Gong and Chen, 2014), and extending far northward to the Changbai mountains on the Sino-Korean border (e.g., Liu et al., 2015). These basalts are predominantly of Ocean-Island-Basalt (OIB) type (e.g., SCS and Indochina, Yan Q. et al. (2018) and references therein; SCB, Ho et al. (2003); Tan-Lu fault zone, Chung, (1999); Changbai Mountains, Liu et al. (2015); inter-regional, Kimura et al. (2018)), have moderate to high mantle potential temperatures (∼1,320°C–1,440°C, see Kimura et al., 2018), and coincide spatially with seismically slow anomalies (Ball et al., 2021). Together these observations suggest that a source of hot, upwelling asthenospheric material is required in order to explain this recent phase of eruptions. We note that this doesn’t necessarily preclude a contribution from slab dehydration (e.g., Richard and Iwamori, 2010), at least for some of this magmatism.
In order to investigate a potential link between the late Cenozoic volcanism in East Asia and our asthenospheric flow hypothesis, we compare the timing and location of intraplate volcanic eruptions to the distribution of hot asthenospheric material from 30 to 0 Ma predicted by the models of Lin et al. (2020). We trace the distribution of temperature anomalies in the asthenosphere by defining the model lithosphere by the 1,300°C isotherm, and the model asthenosphere as the 200 km thick channel below this. In the case that a subducting slab is encountered (i.e., T < 1,300°C through the asthenosphere) a default lithosphere thickness of 100 km is taken, which produces the sharp jumps near subduction zones seen in Figure 5. To avoid the influence of “drip” artefacts left over in the asthenosphere from the subduction of the Izanagi plate (see discussion of model limitations in Section 5.4), we take the mean anomalous temperature in the model asthenosphere, as this best reflects the full lateral extent of the influx of hot material. We make use of the global database of intraplate volcanic samples compiled by Ball et al. (2021). While this is primarily a database of Neogene volcanism, many Paleogene samples are also included, meaning it is suitable for the 30–0 Ma timespan considered here. Additional eruptions in the SCB, Hainan, and South Korea are taken from Gong and Chen (2014) and Arai et al. (2001). The post-rift basaltic eruptions in the PRMB have been included from the map compiled by Sun et al. (2010).
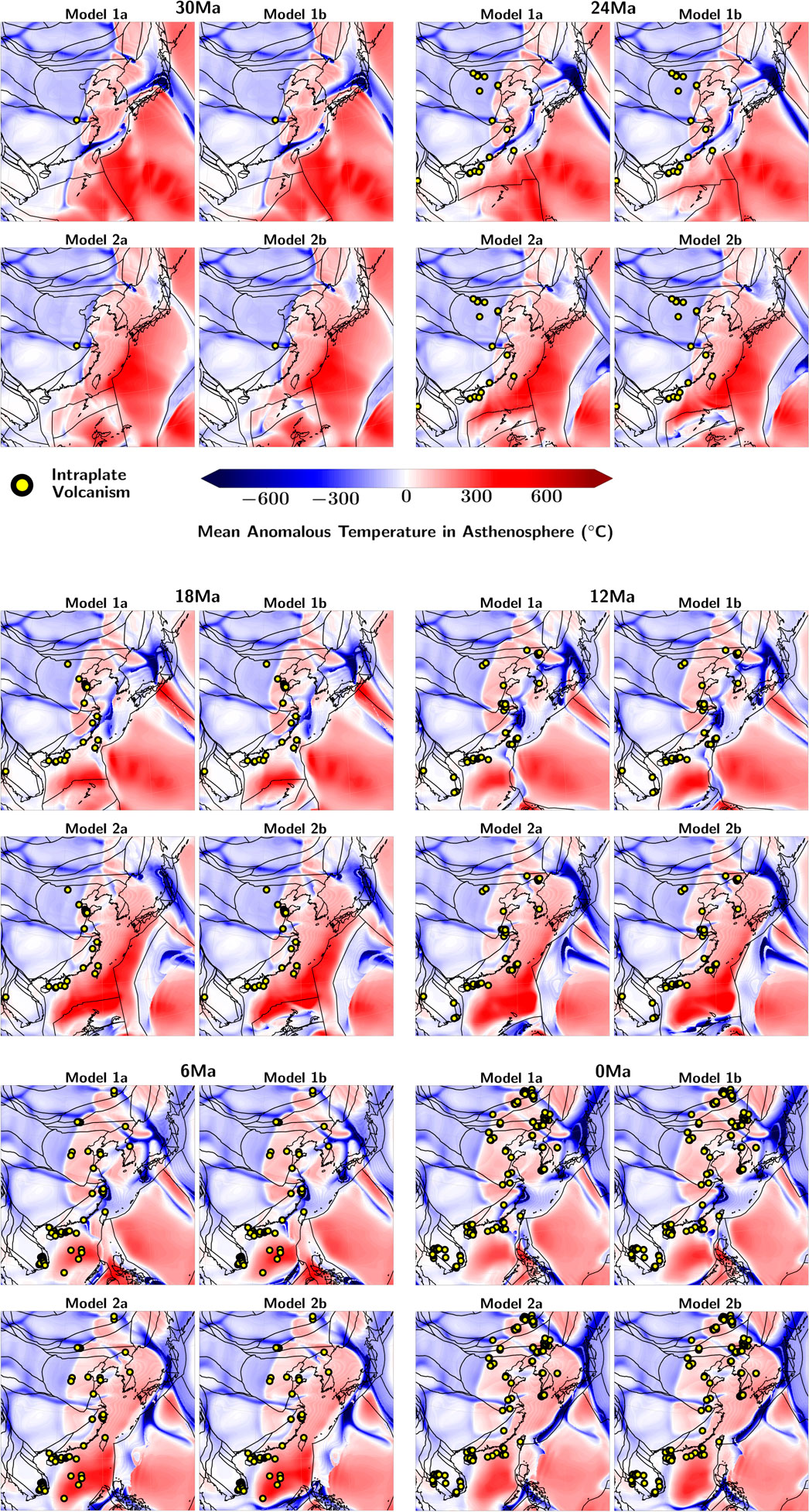
FIGURE 5. Maps of mean anomalous asthenospheric temperature predicted by the models of Lin et al. (2020) at six times from 30 to 0 Ma, with superimposed distribution of coeval intraplate volcanism (Arai et al., 2001; Sun et al., 2010; Gong and Chen, 2014; Ball et al., 2021). Note the connection between the distribution of anomalously hot material underneath the Eurasian plate and the location of intraplate volcanic eruptions, with the notable exceptions of the Hannuoba and Taihang basalts at 24–18 Ma and the eruptions in the Indochina peninsula.
Figure 5 shows the mean anomalous temperature in the asthenosphere at 6 Ma intervals from 30 to 0 Ma, overlaid with intraplate eruptions of age within ±1.5 Ma. Given that the uncertainties in dating are often on the order of ∼1 Ma although in some rare cases, were as high as ∼7–10 Ma, see database of Ball et al. (2021), and the slow pace of mantle flow, this approximation is not likely to result in any spurious connections between the modelling results and mapped eruptions. Maps for the full time-series are reported in Supplementary Figures S9–S19. We briefly note that the anomalous temperature values are systematically over-estimated in the models of Lin et al. (2020), and so we simply consider the distribution of anomalously hot material through time, rather than interpreting its values.
The predicted lateral extent of hot material beneath East Asia from 30 to 0 Ma shows a good match to the distribution of intraplate volcanism during this time (Figure 5). The volcanism in NE China is matched well by all models. Moving further south, Models 2a & 2b, which implement a small PSP, predict a hot, positively buoyant asthenosphere below SE China, Taiwan, and Penghu from 24 to 0 Ma, which is consistent with the OIB-type magmatism observed here (e.g., Chung et al., 1995; Ho et al., 2003). In contrast, Models 1a & 1b, which feature a large PSP, predict predominantly downwelling, cold material in the asthenosphere below these regions, which is inconsistent with this form of magmatism. Some of the westernmost eruptions, such as the Hannuoba and Taihang basalts (HN and TH in Figure 1), are only reached a few Myrs after the onset of magmatism (cf. 24–12 Ma snapshots of Figure 5). Others, such as the eruptions in the Indochina peninsula, are not reached in any of the considered models, although the flow is typically ≲1,000 km from these eruptions. The lateral distribution of hot asthenospheric material at the present-day time-step in Figure 5 shows that this hypothesis is also able to account for the seismically slow anomalies observed in the upper mantle throughout East Asia. Here as well the modelled asthenospheric material does not match the full westward extent of the slow anomalies observed in tomographic models (e.g., Schaeffer and Lebedev, 2013) by ∼1,000 km.
4.2 Hiatus mapping
Stratigraphic data sets hold crucial information about past mantle flow. Mapping conformable and unconformable contacts on inter-regional scales can yield a proxy for the large-scale uplift and subsidence associated with dynamic topography, which may in turn be linked to convective motion in the Earth’s mantle (Friedrich et al., 2018). This method of “hiatus mapping” has been carried out on the scale of single (Vibe et al., 2018; Carena et al., 2019) and multiple (Friedrich et al., 2018; Hayek et al., 2020, 2021) continents, and has provided important information on the influence of mantle flow on large-scale topographic changes since the Mesozoic. However, likely due to the potential tectonic influence of nearby subduction zones, East Asia has not been the focus of any hiatus mapping studies to date—although an inter-regional Cretaceous–Paleocene sedimentary hiatus in Southeast Asia has been noted and linked to mantle flow (Clements et al., 2011). In our preceding comparison, it was found that the proposed influx of hot asthenospheric material approximately matches the distribution of late Cenozoic intraplate volcanism in East Asia. Due to its positive buoyancy, this influx is also expected to induce a coeval dynamic uplift. We may therefore use the hiatus mapping concept to trace this uplift and compare it to the model predictions of Lin et al. (2020), allowing us to test our hypothesis against the sedimentary record over a large region.
In order to gauge large-scale topographic changes during the late Cenozoic, we make use of generalised stratigraphic columns for a group of sedimentary basins (both onshore and offshore) in eastern China. We map columns for the basins surrounding Hainan Island (Shi et al., 2011); the Pearl River Mouth Basin (PRMB, Shi et al., 2008); the Taiwan region (including both exposed strata on Taiwan Island and the rift basins in the Taiwan Strait (TWS), Lin et al., 2003; Shi et al., 2008; Huang et al., 2012); the East China Sea Shelf Basin (ECSSB, Shi et al., 2008; Wang et al., 2019); the Subei Basin (Zhou et al., 2019); the South Yellow Sea Basin (SYSB, Yang et al., 2020; Zhang R. et al., 2020); the Hefei Basin (HF, Jiaodong et al., 2012); the North Yellow Sea Basin (NYSB, Wang et al., 2017); the Bohai Bay Basin (BBB, Tang et al., 2019); and the Songliao Basin (SLB, Song et al., 2018). A map of these basins can be found in Figure 1.
Stratigraphic columns are mapped at the resolution of geological series (ten to a few tens of Myrs, see the definition of series as a unit of chronostratigraphy, e.g., Cohen et al., 2013; Ogg et al., 2016), given that stratigraphic columns with stages resolution were available only in a few offshore basins in eastern China. We follow the approach and terminology of Friedrich (2019), Carena et al. (2019) and Hayek et al. (2020), Hayek et al. (2021), in which, for example, “Base of Miocene” refers to the conformable/unconformable surface below this series (i.e., Oligocene sedimentation/hiatus), and a series is marked as hiatus if and only if there are no preserved sediments of this series (i.e., missing stages within a series are not marked as hiatus). In some cases, stratigraphic columns were available for individual sub-basins and so these too have been mapped where available. Stratigraphic columns are mapped as single points, approximately central in a given basin or sub-basin. While not portraying the true scale of a given basin, this approach tends to avoid issues with intraplate deformation as stratigraphic data are reconstructed back in time to their appropriate palaeolocations. In some cases, however, we still run into issues of this kind. For example, the generalised stratigraphic columns for Taiwan Island represent exposed strata which were deposited on the continental margin during the early Cenozoic. These sediments have been significantly deformed and moved from the location where they were deposited (Suppe, 1980) during the Taiwan Orogeny since ∼6.5 Ma—which is not accounted for when these points are reconstructed back in time. Due to this, the reconstructed point data will not reflect the true palaeolocations of deposition, and so this must be kept in mind when interpreting the resulting maps. Given the size of the Songliao Basin, and the lack of nearby basins, a representation of its stratigraphic column as a single point is particularly misleading (for other larger basins, stratigraphic columns for each sub-basin were available, or nearby basins filled out the surrounding space). We therefore make the choice to instead plot the generalised stratigraphic column of the Songliao Basin as a group of points—one for each sub-unit of the basin (sags and uplifts). This better represents the true area of the data used in NE China. Given that the Songliao Basin has not undergone significant deformation since the Paleocene (e.g., Song et al., 2018), the locations of the points relative to each other will have remained relatively constant throughout the late Cenozoic. This means that the reconstruction, which keeps these points fixed relative to each other, will result in a reasonable representation of their palaeolocations.
As can be seen in Figure 4, the modelled influx of hot asthenospheric material is present below far eastern China from the mid/late Eocene and flows below this region (i.e., SLB, BBB, S/SYSB, HF, ECSSB, TWS, TNB, see Figure 1) during the Oligocene. This coincides temporally with an inter-regional late Eocene–Oligocene sedimentary hiatus recorded in these basins, the exact timing of which depends somewhat on location. To investigate the potential link between this large-scale hiatus and the inflowing Pacific material, we compare our Base of Miocene hiatus map to the modelled change in dynamic topography during the Oligocene. A comparison between our Base of Pliocene hiatus map and the predicted change in dynamic topography during the Miocene can instead be found in Supplementary Figure S20, along with an associated discussion in Supplementary Text S4.
The change in dynamic topography is calculated in a Lagrangian sense. That is, we calculate this change in the reference frame of the Eurasian plate as it moves with respect to the underlying time-dependent dynamic topography signal. The modelled asthenospheric flow predicts a broad N-S trending band of uplift during the Oligocene (Figure 6), which matches the distribution of sedimentary hiatus during this time to first-order. The BBB and NYSB (highlighted as blue stars in Figure 6) both stand out as outliers amongst the sedimentary hiatus in eastern China. It is worth noting, however, that rifting continued into the late Oligocene in these basins (Qi and Yang, 2010; Wang R. et al., 2020), and so rapid syn-rift tectonic subsidence is likely to largely overwrite any dynamic topography signal in the stratigraphic record. In addition to this, while syn-rift sedimentation occurred in these basins, the late Oligocene strata are clearly eroded, and have been completely removed in some shallower parts of the basins. Indeed, this aspect of the development of the BBB has been investigated further using Apatite Fission Track Thermochronolgy (AFT), which found a rapid cooling event from 27 to 16 Ma, removing an estimated 1.5–1.8 km of sediment (Tang et al., 2019). This uplift event has been noted too in the NYSB (Wang R. et al., 2020), and while it has been attributed to tectonic compression, the stratigraphic cross-sections of the basin are also consistent with a regional uplift characteristic of dynamic topography. This uplift is thought to have continued until ∼14 Ma in the NYSB (Wang R. et al., 2020). And so, while a full sedimentary hiatus was not induced in these basins, they remain consistent with the inter-regional picture of uplift and erosion during the Oligocene and early Miocene.
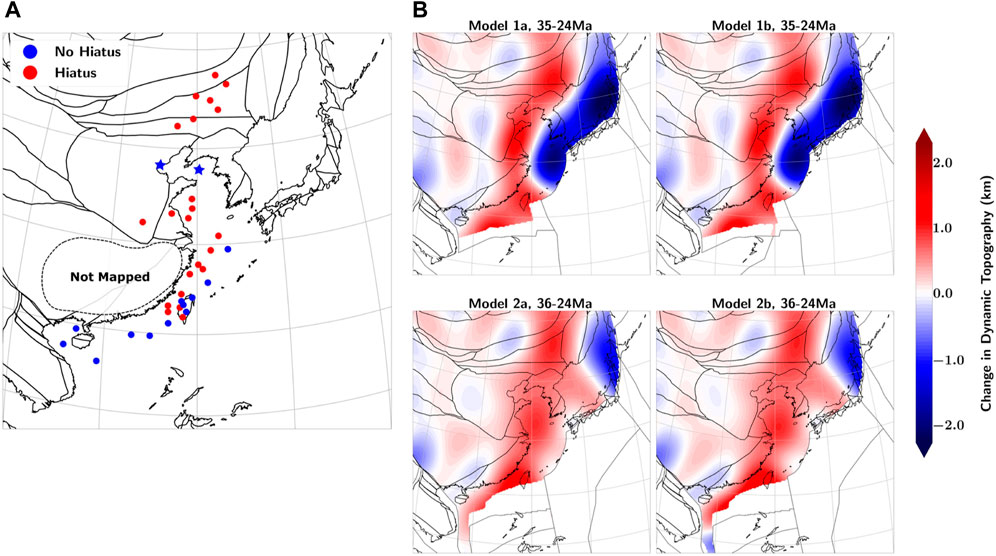
FIGURE 6. (A) Base of Miocene hiatus map for the sedimentary basins in eastern China and exposed Cenozoic strata in Taiwan. Blue stars mark the locations of Bohai Bay Basin (west) and North Yellow Sea Basin (east), which are mapped as No Hiatus but are consistent with a regional uplift event (see discussion in text). Notice that the SCB (outlined by a dashed line) is excluded from our mapping. This region is characterised by a distinct lack of Cenozoic sediments (cf. Figure 1) meaning that Cenozoic uplift events in this area can be more easily gauged via AFT thermochronology (Section 4.3). (B) Change in dynamic topography during the Oligocene predicted by the mantle circulation models of Lin et al. (2020).
Additional misfits can be found in some offshore regions, such as the eastern ECSSB and the northern margin of the SCS, in which the predicted dynamic uplift covers regions of Oligocene sedimentation. However, the early Cenozoic rifting and offshore setting of these regions means that it is somewhat less likely that a dynamic uplift will lead to a series-long sedimentary hiatus. Indeed, while a series-long hiatus has not been recorded, there nevertheless exists evidence of late Cenozoic uplift and erosion on the northern margin of the SCS (Section 5.2.3). Intense erosion has been noted too in the Taiwan region (e.g., Fuh, 2000; Shi et al., 2008), but the onset of sedimentation during the late Oligocene means that this event is not highlighted when mapping is carried out at the resolution of geological series (Figure 6).
Similarly to our comparisons with intraplate volcanism, Figure 6 shows that the downwelling induced by the large PSP of Models 1a & 1b leads to an inconsistency with the geological record. These models predict a large-scale dynamic subsidence in the ECSSB during the Oligocene, which is inconsistent with the observed sedimentary hiatus. In Models 2a & 2b, instead, the influx of hot asthenospheric material is allowed to predominate the dynamic topography signal over a larger region, leading to a consistency with the sedimentary hiatus in the ECSSB.
As can be seen in Figure 6, the SCB was excluded from our mapping. Cenozoic deposits are largely absent from this region (Figure 1), meaning that the hiatus mapping concept is not useful in gauging large-scale topographic changes during this time. Cenozoic sedimentation occurred mainly in small intermontane basins, which are not likely to be sensitive to the changes in relative sea level induced by dynamic topography. We therefore take a different approach in the SCB, which is discussed in the following section.
4.3 AFT comparisons
The SCB has garnered significant attention from thermochronological studies (e.g., Yan et al., 2009; Wang et al., 2015; Li and Zou, 2017; Su et al., 2017; Tao et al., 2019; Wang Y. et al., 2020; Qiu et al., 2020), likely due to the regional history of exhumation inferred from the widespread present-day exposure of Mesozoic and Paleozoic rocks. That this exhumation has continued into the Cenozoic is revealed by the exposure of late Cretaceous granites formed during the flat-slab subduction of the Izanagi plate (Charvet et al., 1994), indicating at least kilometre-scale erosion has occurred during the Cenozoic (Yan Y. et al., 2018). While thermochronological studies have uncovered multiple phases of heating and cooling since the beginning of the Mesozoic (Tao et al., 2019; Wang Y. et al., 2020), we focus here on the late Cenozoic cooling history of the region. This allows us to investigate the dynamic uplift expected under our hypothesis over a larger region, by filling a spatial gap in the SCB (Figure 6).
A significant rapid cooling event during the Oligocene and Miocene has been found consistently in numerous studies (see those compiled by Qiu et al., 2020), with samples covering a large (∼1,000,000 km2) region. To test whether this regional-scale erosion of the SCB during the late Cenozoic is linked to the dynamic uplift expected under our hypothesis, we compare the dynamic topography predictions of each model to the AFT-derived cooling histories published by Wang Y. et al. (2020), Yan et al. (2009), and Li and Zou, (2017). A total of 21 samples are considered, of which 9 have been reported in Figure 7 to highlight key characteristics in different regions. Clouds of “acceptable” (95% confidence limit) fits have been included to indicate the uncertainty of the AFT cooling histories. The full set of samples is included in Supplementary Figures S21–S41.
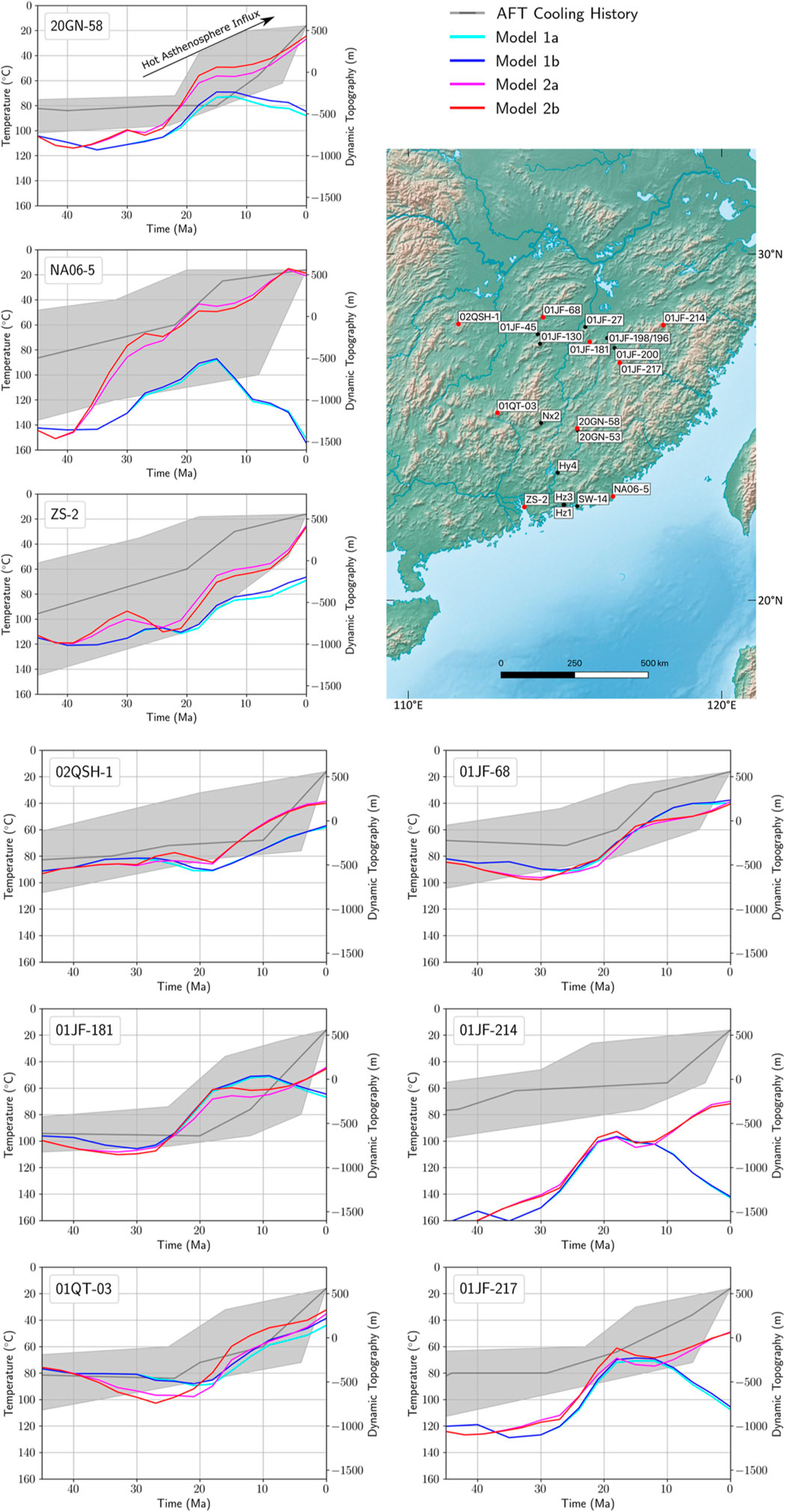
FIGURE 7. Comparison between the dynamic topography predictions of the mantle circulation models of Lin et al. (2020) and the AFT-derived cooling histories published by Wang Y. et al. (2020), Yan et al. (2009) and Li and Zou (2017). Notice the poorer fit of Models 1a & 1b, in particular for samples closer to the Ryukyu Trench. Samples included here are highlighted in red on the map of sample locations. The full set of samples is included in Supplementary Figures S13–S33.
The AFT cooling histories generally show a distinct increase in cooling rate during the early Miocene (see, e.g., samples 20GN-58 and 01JF-181 in Figure 7) or late Miocene (samples 02QSH-1 and 01JF-214). In northwesterly regions (samples 02QSH-1, 01JF-68 and 01QT-03), the model predictions of dynamic topography are broadly similar, and we find a good match between the observed cooling histories and the increase in dynamic topography induced by the inflowing asthenospheric material from ∼20 Ma. In easterly samples (NA06-5, 01JF-214, 01JF-217), the closer proximity to the Ryukyu Trench leads to significant differences in the model predictions based on their implementations of the PSP history. In line with our previous comparisons, we find that the large PSP implemented in Models 1a & 1b leads to a dynamic subsidence which is inconsistent with the observed cooling histories. In contrast, the smaller PSP implemented in Models 2a & 2b allows the influx of hot asthenospheric material to dominate the Neogene dynamic topography in this region, leading to a better prediction of the increased cooling rate during this time.
A key consideration to be made when interpreting this widespread cooling effect is that it occurs outside of the well-constrained Apatite Partial Annealing Zone (PAZ, 110°C–60°C). Generally, one cannot confidently interpret cooling histories below the lower bound of 60°C, due to the lack of fission track annealing below this temperature. However, we note that the samples considered often leave the PAZ at ∼20–10 Ma, and given their present-day exposure at surface temperatures (∼20°C), their Neogene cooling histories remain well constrained. For example, samples 20GN-58 and 01JF-181 (Figure 7) cool through the lower bound of the PAZ at ∼10 Ma, and so we may infer that ∼40°C of cooling has occurred since this time. Taking a lower bound on the geothermal gradient of ∼20°C/km, we may then estimate ∼2 km of erosion has occurred with a rate of ∼0.2 km/Ma since ∼10 Ma. We therefore find the recent regional cooling to be a robust effect in the derived cooling histories.
We note additionally that the predicted uplift is generally significantly lower than the eroded sediment thickness inferred from AFT cooling histories (Figure 7). For instance, the 2 km exhumation of samples 20GN-58 and 01JF-181 since 10 Ma, as estimated above, corresponds to only ∼250–500 m of predicted dynamic uplift. A key reason for this is that isostatic rebound has not been accounted for in these comparisons, which provides an additional component of uplift as overlying rock is eroded. Part of this disparity may also arise due to the modelled asthenospheric influx not flowing far west enough in significant amounts, in keeping with our comparison to the magmatic record (Section 4.1). Nevertheless, we find an encouraging match between the recent regional cooling and the modelled increase in dynamic topography during the Neogene.
4.4 Anomalous uplift of the Qiongdongnan Basin
Subsidence analysis may be used to make quantitative estimations of the history of dynamic topography affecting rifted sedimentary basins. Traditionally, this is achieved by estimating strain rates during rifting, for instance by inverting the backstripped subsidence (White, 1994), and using these in conjunction with lithospheric stretching models (McKenzie, 1978; Jarvis and McKenzie, 1980b) in order to forward model the post-rift tectonic subsidence. This forward modelled tectonic subsidence may then be subtracted from the subsidence gleaned from backstripping (Sclater and Christie, 1980), in order to find the anomalous (i.e., dynamic) uplift or subsidence which has affected a given basin during its post-rift phase. A refined method of this kind has recently been proposed by Zhao (2021), which sought to remove several of the underlying assumptions of earlier methods. Zhao (2021) used fault growth rates derived from seismic cross sections to allocate the total strain of a basin, allowing spatially and temporally varying strain rates to be calculated. The use of strain rates which are independent of the backstripped subsidence means that one separates the tectonic and dynamic components of subsidence from the outset. This allows for the full (i.e., including the syn-rift phase) history of anomalous subsidence to be derived, and the tectonic subsidence due to secondary rifting phases to be accounted for. This method was applied to the Qiongdongnan Basin (QDNB), which, given its location on the northern margin of the SCS (Figure 1), provides us with an additional observation with which to test our asthenospheric flow hypothesis. Zhao (2021) found that the QDNB was anomalously uplifted by ∼1 km beginning at 28.4 Ma, which decreased significantly after 11.6 Ma. Our intraplate volcanism comparisons in Figure 5 show that the influx of hot material reaches the QDNB during the Oligocene, and may therefore provide an explanation for this uplift. We therefore compare the anomalous uplift since 45 Ma with the modelled change in dynamic topography since this time. The resulting comparisons, and a map of the relevant borehole locations, is shown in Figure 8.
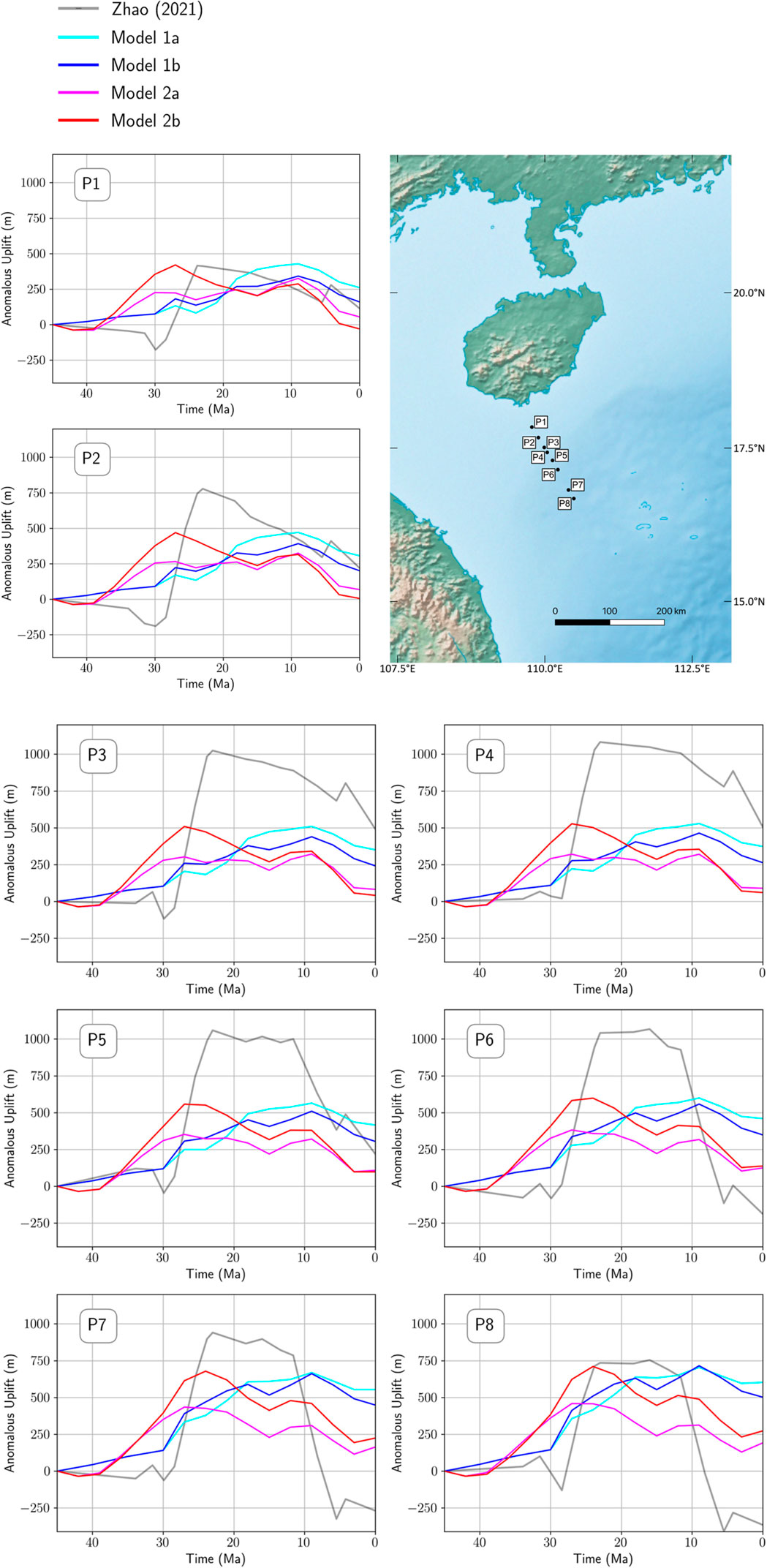
FIGURE 8. Comparison between the anomalous uplift of the QDNB from Zhao (2021) since 45 Ma with the predicted change of dynamic topography since this time predicted by the mantle circulation models of Lin et al. (2020).
To first order, we find a good match between the modelled timing of dynamic uplift and subsidence affecting the QDNB and the anomalous uplift at boreholes P1–P8. The modelled uplift tends to have an earlier onset than the results of Zhao (2021) in Models 2a & 2b, and has a particularly high amplitude in Model 2b. Given the double-sided PSCS subduction in Model 2b, the northern subduction zone leads to additional downflow in the QDNB region, pulling more asthenospheric material towards the basin from the east. The northern subduction zone additionally leads to a larger initial dynamic subsidence in the QDNB, and so the rebound from this initial state following the subduction of the PSCS provides an additional component of uplift. The inflowing hot asthenospheric material comes to predominate the dynamic topography signal in this basin in all considered models during ∼30–25 Ma (i.e., the dynamic topography signal becomes net positive). This timing aligns with the onset of uplift found by Zhao (2021), although the modelled amplitude of the uplift tends to fall short by ∼500 m (excluding P1 and P8, in which we find an excellent match to the reconstructed amplitude). As noticed in Section 4.1, the modelled influx of asthenospheric material does not flow far west enough. This is particularly apparent in the regions of Hainan and Indochina (Figure 5), which are adjacent to the QDNB. A more vigorous influx of hot material in the geodynamic models would presumably lead to a better match to the observed amplitudes.
Another difference we observe between the results of Zhao (2021) and the model predictions of Lin et al. (2020) is the spatial variability of the dynamic uplift signal: the models predict a maximum of ∼250 m of variation of the peak of uplift between the borehole locations, while subsidence analysis predicts it to be ∼500 m. This may have resulted either from a lack of small-scale structure in the modelled dynamic topography, or from underlying assumptions in the analysis of Zhao (2021). At short enough wavelengths, the viscous stresses which would otherwise induce dynamic topography are instead opposed by the strength of the lithosphere. This filtering effect is modulated by the flexural rigidity of the lithosphere and is strongly wavelength-dependent (see, e.g., Turcotte and Schubert, 2002). To account for this effect, the dynamic topography predicted by the models of Lin et al. (2020) has been expressed as a spherical harmonic expansion truncated at lmax = 40, which filters out short-scale (i.e., ≲500 km) variability. While this is a reasonable first-order approximation of lithospheric behaviour, it might filter out too much of the mantle signal, particularly in regions of young oceanic lithosphere where the flexural rigidity might be low. The analysis of Zhao (2021), on the other hand, assumes local isostasy in both the backstripping routine and the lithospheric stretching model used to forward model the tectonic subsidence. It is therefore assumed that both the sediment load and crustal thinning during rifting vary smoothly with respect to the elastic thickness of the lithosphere. If present, short-scale variability in these features leads to additional spatial variability in the derived subsidence histories (instead of being compensated via regional isostasy). The gradient in dynamic topography of ∼10 m/km between boreholes P1 and P3 at ∼25 Ma is particularly high, suggesting that the assumption of local isostasy might not be adequate.
5 Discussion
Several lines of evidence point towards a pervasive influence of hot asthenosperic material in East Asia during the late Cenozoic. These include the widespread intraplate volcanism since the latest Paleogene (e.g., Ball et al., 2021), extensive seismically slow anomalies at asthenospheric depths (e.g., Li et al., 2008; Schaeffer and Lebedev, 2013; Ma J. et al., 2019), and swells of present-day dynamic uplift (Hoggard et al., 2021). A westward influx of Pacific asthenospheric material through the Izanagi-Pacific slab window arises naturally in the global mantle circulation models of Lin et al. (2020), showing this to be a plausible new mechanism in explaining these observations. The comparisons presented in Section 4 show that the predicted influence of hot Pacific material during the late Cenozoic corresponds well with several independent observations throughout East Asia. These are linked to both intraplate volcanism and dynamic topography on inter-regional (Sections 4.1, 4.2), regional (Section 4.3), and local (Section 4.4) scales.
5.1 Intraplate volcanism in East Asia
A multitude of hypotheses have been proposed to explain the recent inter-regional phase of mafic volcanism in East Asia (Figures 1, 5) on smaller, regional scales. Of the mechanisms proposed, a hypothesised mantle plume below Hainan Island on the northern margin of the SCS is by far the most prevalent (e.g., Yan et al., 2008; Lei et al., 2009; Wang et al., 2012; Huang, 2014; Xia et al., 2016; Yan Q. et al., 2018; Zhang Y et al., 2020, etc.). The “Hainan plume” has been proposed to explain the basaltic eruptions in Leizhou, Hainan, Indochina and the SCS basin since the late Miocene (e.g., Yan et al., 2008), as well as the seismically slow anomalies observed below the SCS region in numerous tomographic models (e.g., Lei et al., 2009). Limited tomographic resolution in the lower mantle means it is not yet possible to conclusively prove the existence of a Hainan plume extending down to the Core Mantle Boundary (CMB), and while a seismically slow anomaly in the upper mantle appears to be a robust feature, similar anomalies are observed throughout East Asia, as discussed in Section 1. It is worth noting too that a plume in this region has not been resolved in global tomographic studies which have resolved plumes (e.g., French and Romanowicz, 2015; Hosseini et al., 2020), although it may be the case that tomographic resolution is not yet high enough to resolve the proposed Hainan plume. Additionally, the magmatic record in this region lacks the clear arrival of a plume head, which is evidenced at well-known plume locations by the formation of a Large Igneous Province (LIP) (Richards et al., 1989). The westward migration of a small plume into this region has been found to be a plausible geodynamic mechanism using mantle circulation models (Zhang and Li, 2018). As noted above, it remains unclear, though, whether the magmatic and tomographic evidence supports this hypothesis.
The lack of a clear plume signal is true, similarly, for the eruptions on inter-regional scales. In addition to the absence of LIPs, the eruptions don’t show the linear age progression characteristic of plume-fed hotspots, and don’t align with absolute plate motions, with contemporaneous eruptions spanning over large (∼5,000 km) distances. The pattern of these eruptions (Figure 1) is instead reminiscent of those observed in the far-field of mantle upwellings, for instance in Africa (Lees et al., 2020). An east–west younging in the onset of eruptions is expected under our hypothesis, and is indeed observed in several regions. For example, the onset of volcanism in SE China and Taiwan is earlier than in Hainan, which in turn has an earlier onset than the main phase of eruptions in Indochina. This east–west younging can also be observed in NE China, with an earlier onset of volcanism in Changbai than the more westerly regions of Halaha and Dalenuor (Liu et al., 2001). However, there are clear exceptions to this trend. For instance, the onset of volcanism in Hannuoba is earlier than the more easterly eruptions in the Jiaodong peninsula, which in turn is earlier than the eruptions in the Korean peninsula. It is worth noting, however, that the study region is interspersed with offshore areas within which the magmatic record is highly biased towards the most recent eruptions. Post-rift intraplate eruptions in, for example, the ECSSB and SYSB (e.g., Yao et al., 2020) must be mapped and dated using seismic stratigraphy and borehole data due to recent sediment cover. As such, it is less likely that such eruptions have been mapped and dated accurately in the current literature. This, more generally, leads to a bias towards onshore regions. Indeed, this bias can be clearly observed in Supplementary Figures S9–S19, with the vast majority of mapped eruptions lying within onshore regions. It may therefore be the case that a clearer east–west younging will emerge as the magmatic record in these offshore regions becomes more complete.
Another prominent line of thinking invokes the influence of the Pacific slab in sourcing the intraplate volcanism (e.g., Liu et al., 2001) and underlying seismically slow anomalies (e.g., Ma J. et al., 2019) in NE China, through deep slab dehydration (e.g., Richard and Iwamori, 2010; Kuritani et al., 2019) or the interaction of the slab with a hydrous Mantle Transition Zone (MTZ) (Yang and Faccenda, 2020). While we don’t necessarily preclude some contribution from these mechanisms, we note that they are not required under our hypothesis. Relatively high mantle potential temperatures (∼1,380°C–1,440°C) have been noted in NE China (Kimura et al., 2018), indicating that a hot mantle source is required in this region. In addition to this, geochemical analyses have found no evidence of a typical slab-derived isotopic signature in these basalts (Chen et al., 2007; Ward et al., 2021). In any case, the distribution of seismically slow anomalies and intraplate volcanism spans far into regions which are not underlain by slabs (e.g., the SCB, see Gong and Chen, 2014; He and Santosh, 2021), suggesting that these mechanisms are not sufficient on inter-regional scales.
The asthenospheric flow hypothesis presented here unifies the inter-regional observations of intraplate OIB-type magmatism into a single picture. The approximate match observed between the timing and location of these eruptions and the models of Lin et al. (2020) shows this to be a viable new mechanism in explaining these eruptions. An open question under our hypothesis is the differing eruption volumes observed between regions—for example the low-volume eruptions observed in SE China and Taiwan compared to those observed in Indochina or NE China (Figure 1). There are many factors which may explain this disparity in future studies, such as local variations in lithospheric thickness, the influence of underlying slabs, or as noted by Lin et al. (2020), local passive return flow in the mantle—which may play a role in facilitating particularly voluminous eruptions in certain regions. Nevertheless, the correspondence we have found between the distribution of Pacific asthenospheric material and the timing and location of intraplate volcanism in East Asia provides a starting point for future investigation into the local variations observed in the erupted magmas, such as volume and geochemistry.
5.2 Topographic changes linked to asthenospheric flow
5.2.1 Far Eastern China
The dynamic uplift induced under our hypothesis is consistent with the sedimentary record over a large region. The sedimentary hiatus associated with this uplift has been noted locally under a number of names (e.g., the “Puli Event/Orogeny” in Taiwan, the “Yuquan Movement” in the ECSSB, the “Sanduo Movement” in the SYSB, etc.), and has been commented on as an inter-regional effect (e.g., Wang R. et al., 2020), although its source has remained unclear. Due to the coeval uplift of the Tibetan plateau further west, and the proximity to the Pacific subduction zone to the east, this hiatus is sometimes attributed to a temporary change from an extensional to a compressional stress regime due to far-field effects either from the India-Asia collision (e.g., Song et al., 2018), or from an increase in the convergence rate at the Pacific subduction zone (e.g., Tang et al., 2019). However, the structures of the basins recording this hiatus inferred from seismic stratigraphy and borehole data (e.g., Lin et al., 2008; Qi and Yang, 2010; Cukur et al., 2011; Yang et al., 2016; Song et al., 2018; Yang et al., 2020) show no clear signs of compression in the sedimentary layers directly underlying the unconformity associated with this hiatus. Indeed, throughout the basins considered, the relevant unconformity truncates extensional or quiescent structures in the underlying layers, showing that structural deformation cannot be the root cause of the stratigraphic hiatus, and proving the need for inter-regional scale uplift. In addition to this, the scale (∼3,000,000 km2) of this uplift event is more characteristic of a dynamic uplift than a far-field compression such as that observed in, e.g., the Baikal rift system (Petit and Deverchere, 2006). Another consideration to be made is the eustatic sea-level drop (∼50–100 m) during the Oligocene, which can look indistinguishable from dynamic uplift in the stratigraphic record (i.e., large-scale erosion with no compressional deformation). Indeed, this is observed as a clear erosional/non-depositional signal in the hiatus maps of Hayek et al. (2020), Hayek et al. (2021). However, sonic velocity vs. depth measurements (Fuh, 2000) have found 1–3.5 km of eroded sediment thickness during the late Eocene–Oligocene erosion of the Taiwan region and the ECSSB. Similarly, as noted in Section 4.2, AFT-derived cooling histories suggest that 1.5–1.8 km of sediment was removed from the BBB from 27 to 16 Ma (Tang et al., 2019). And so, while the Oligocene sea-level drop likely contributed to this widespread erosion event, it is not sufficient in explaining the measured eroded sediment thickness. Taken together, the scale, lack of compression, and eroded sediment thickness indicate this to be a robust dynamic topography signal in the stratigraphic record.
5.2.2 The South China Block
Following the Oligocene erosion in far eastern China, the modelled hot asthenospheric material spreads further westward into the SCB during the Miocene. Along with the coeval intraplate volcanism in this region (Gong and Chen, 2014), this coincides temporally with a regional erosion event inferred from numerous AFT studies (e.g., Yan et al., 2009; Li and Zou, 2017; Wang Y. et al., 2020; Qiu et al., 2020). Recent reports by Stephenson et al. (2021) suggest the SCB to be dynamically uplifted by ∼1 km at present, which is consistent with the seismically slow anomalies observed below this region (e.g., He and Santosh, 2021). Together, these observations point towards a dynamic origin of the recent regional cooling. The comparisons presented in Figure 7 show that the dynamic uplift predicted under small-PSP Models 2a & 2b provides a good match to the erosion inferred from AFT studies. Our hypothesis therefore ties together a variety of past and present-day observations in the SCB, including Neogene regional erosion (Figure 7), present-day dynamic uplift (Stephenson et al., 2021), seismically slow anomalies (e.g., He and Santosh, 2021) and intraplate volcanism (e.g., Gong and Chen, 2014).
5.2.3 The northern margin of the South China Sea
To the south of the SCB, the hot asthenospheric influx predicted by the models of Lin et al. (2020) flows westward below the northern margin of the SCS from the late Eocene to the present day (Figures 4, 5). While this region is largely dominated by syn- and post-rift tectonic subsidence during the Cenozoic, uplift events have been noted in several regions. Similarly to the observations of uplift discussed above, these also hint at the east–west progression expected under our hypothesis. At the eastern end of this region, erosion was particularly intense in Taiwan and its surrounding region from the late Eocene–late Oligocene, removing an estimated 1–3.5 km of sediment (Fuh, 2000) and generating hiatuses that span back to the Cretaceous in the Tainan Basin and the adjacent Chaoshan depression in the PRMB (Shi et al., 2008). Further westward, the subsidence analysis of Zhao (2021) found that kilometre-scale dynamic uplift affected the QDNB from the Oligocene–late Miocene. While attributed by Zhao (2021) to the dynamic influence of the Hainan plume, the first-order match between this uplift and the models of Lin et al. (2020) (Figure 8) shows that this can be explained under our asthenospheric flow hypothesis. The timing of this uplift is also consistent with thermochronological results on the adjacent Hainan Island (Shi et al., 2011). Further west still, the Yinggehai Basin shows clear evidence of erosion during the early Miocene. Cross-sections of the basin’s structure (Clift and Sun, 2006) show that the ∼21–16 Ma post-rift sedimentary successions are highly thinned in much of the basin, being present mainly in its central sag. Together with the lack of compressional deformation below the associated seismic reflector, this is consistent with the dynamic uplift observed in the adjacent QDNB.
The PRMB, which lies between the Tainan Basin and Hainan Island, is currently the weak link in this picture. Subsidence analyses have been applied in this basin (Xie et al., 2006; Dong et al., 2020), finding anomalous subsidence of ∼1–2 km since the beginning of the Miocene. At a first glance, this is seemingly at odds with the expected dynamic uplift under our asthenospheric flow hypothesis. It is worth noting, however, that these methods contain numerous underlying assumptions which may have had a significant effect on the previously derived subsidence histories in this region. This is highlighted by the results of Zhao, (2021), in which a refined method found ∼1 km of Oligocene–Miocene dynamic uplift in the QDNB, a basin in which ∼1 km of Miocene dynamic subsidence was found using previous methods (Xie et al., 2006). The history of the PRMB is particularly complex, with multiple rifting phases interspersed by periods of erosion (e.g., Xie et al., 2019), followed by post-rift basaltic magmatism (e.g., Zhou et al., 2020). As such, the application of the method of Zhao, (2021) to this basin is likely to be essential in elucidating Cenozoic dynamic uplift and subsidence events in this basin. Along with the uplift events discussed above, this will lead to a more complete picture of Cenozoic dynamic topography changes on the northern margin of the SCS.
5.3 Constraints on Cenozoic tectonics
The comparisons presented in Section 4 provide new constraints on the uncertain Cenozoic tectonics of East Asia introduced in Section 3.1. The earlier onset of PSP subduction along the Eurasian margin at ∼30 Ma in Models 1a & 1b leads to predominantly downwelling, cold material in the asthenosphere below SE China and the ECSSB. This downwelling corresponds poorly with the timing of OIB-type magmatism (Figure 5) and induces large-scale dynamic subsidence which is inconsistent with the observations of inter-regional uplift in far eastern China (Figure 6) and the SCB (Figure 7). In contrast, the later onset of PSP subduction at ∼12 Ma in Models 2a & 2b allows a hot, positively buoyant asthenosphere to dominate over a larger region during the late Cenozoic. This provides a good fit to the observations of OIB-type magmatism and inter-regional erosion presented in Figures 5–7. The comparisons with the results of Zhao (2021) show that Models 1a & 1b provide a better fit to the onset of uplift in the QDNB (Figure 8). However, the peak of uplift predicted by Models 1a & 1b tends to come later than that found by Zhao (2021), with the latter generally lying between the peaks of Models 1a & 1b and Models 2a & 2b. As such, it is not possible to make a strong distinction between the PSP implementations based on this form of evidence. Based on Figures 5–7, we therefore find new support for the small PSP and existence of the EAS slabs proposed by Wu et al. (2016).
While the PSCS subduction style was the focus of the original study of Lin et al. (2020), it has a largely negligible effect on the asthenospheric flow considered here. This is highlighted by the minor differences between models which implement a single- (Models “a”) or double-sided (Models “b”) subduction of the PSCS in Figures 5–7. The location of the QDNB on the northern margin of the SCS means that we see more significant differences between the models based on their PSCS implementation in this region. However, the comparisons shown in Figure 8 indicate no clear preference for a particular reconstruction. Of Models 2a & 2b, the double-sided PSCS subduction (2b) provides a better match to the amplitude of uplift; whereas of Models 1a & 1b, the single-sided PSCS subduction (1a) provides a better match to the amplitude of uplift. And so, overall, it is not possible to make any clear distinctions between the implemented PSCS reconstructions based on the comparisons presented here.
5.4 Model limitations
Several of our comparisons in Section 4 show that the asthenospheric flow predicted by the models of Lin et al. (2020) does not flow far west enough to explain certain observations. The comparisons in Figure 5 show a ∼1,000 km shortfall in matching both the westernmost volcanic eruptions considered and the present-day distribution of seismically slow anomalies (e.g., Schaeffer and Lebedev, 2013). In addition to this, Figure 8 shows that, while the models predict well the timing of uplift and subsidence of the QDNB, the influx of hot material does not reach this basin in significant enough amounts to match the amplitude of anomalous uplift found by Zhao (2021) by ∼500 m.
One key limitation of these models which may reconcile this disparity in future studies is the implemented mantle viscosity profile. The asthenosphere features only a moderate (200x) viscosity drop with respect to the lithosphere, which is achieved with a gentle gradient from 50 to 250 km depth (Supplementary Figure S1). Due to limitations on the maximum per-gridpoint viscosity variations in the TERRA mantle convection code, this viscosity profile is a compromise between viscosity drop and channel thickness. These models therefore implement a relatively large viscosity in the asthenosphere, which is coupled with a temperature-dependent rheology without a yield stress. As a consequence, following the cessation of the subduction of a particular plate (e.g., the subduction of the Izanagi-Pacific ridge), slabs gradually break off at mid-asthenospheric depths, leaving large “drips” of cold slab material which hang from the lithosphere into the asthenosphere. This effect can be clearly observed in Figure 4, in which a large band of cold material is left hanging in the upper mantle at 45 Ma following the subduction of the Izanagi-Pacific ridge at 55 Ma. This can be observed, too, in Figure 5, in which the bands of cold material observed throughout China represent the drips left over from old subduction zones.
The band of slab material left over from the Izanagi subduction has a significant effect on the asthenospheric flow studied here. The inflowing material from the Pacific domain is forced to push through the base of this high-viscosity barrier and largely flows at depths of ∼300–500 km (Supplementary Figures S2–S8). The blockage of this material from large parts of the low viscosity asthenosphere, as well as the intervening slab material, leads to a significant slow-down in flow velocity. Consequently, the inflowing Pacific material cannot flow far west enough during the late Cenozoic. A larger viscosity drop in the asthenosphere, making it compatible with the new constraints of
Another limitation of these models is the unrealistic slab morphology induced by two-sided subduction. For instance, the stagnant Pacific slab beneath East Asia (e.g., Ma J. et al., 2019) is not reproduced (Supplementary Figures S2–S8), which could have a significant effect on the predicted dynamic topography signal during the late Cenozoic (Supplementary Text S4). A variety of methods have aimed to reproduce this slab geometry using mantle circulation models (MCMs) (e.g., Mao and Zhong, 2018; Ma P. et al., 2019; Peng et al., 2021). However, these approaches have tended to come at the expense of significant additional approximations, such as the suppression of mantle upwellings. As such, it is not yet apparent whether current models can reproduce stagnant slab morphologies within an Earth-like convective planform. A conceptually simple method of generating more realistic slab behaviour is the use of a temperature-dependent viscosity coupled with a pseudo-plastic rheology (Moresi and Solomatov, 1998). The use of this rheology in MCMs produces more concentrated deformation at subduction zones, reducing the presence of “drip” artefacts, and inducing flat-slab subduction following fast trench retreat (Bello et al., 2015). Furthermore, the weak zones which arise between plates would presumably lead to more realistic slab window geometries in the uppermost mantle, which could prove vital when using MCMs to study slab window asthenospheric flow more generally. And so, while the use of a non-linear rheology comes with additional computational difficulties (e.g., Fraters et al., 2019), the self-consistent generation of realistic slab morphologies makes this an attractive option for future studies of this kind.
In any case, MCMs in general are fundamentally limited in their ability to reconstruct past mantle flow, as the initial state from which this flow is derived is unknown. This is partially overcome in MCMs by assuming an initial state, and assimilating tectonic histories as boundary conditions at the surface (Bunge et al., 2002). The models of Lin et al. (2020) illustrate nicely the utility of this technique when reconstructions are assimilated over a significant
5.5 Implications for Southeast Asia
Our asthenospheric flow hypothesis has significant implications for the more southerly regions of Southeast Asia which were not considered here. Following the future model improvements discussed above, it becomes apparent that the influx of hot material studied here could flow into these region during the late Cenozoic. Due to its proximity to the Sunda Trench, Southeast Asia has traditionally been expected to be dominated by dynamic subsidence during the late Cenozoic (e.g., Wheeler and White, 2002). However, growing evidence implies the influence of hot material during this time, such as the dynamic uplift and basaltic magmatism in Borneo since the Neogene (Roberts et al., 2018), the Neogene uplift (Fyhn et al., 2009) and intraplate basalts (e.g., Yan Q. et al., 2018) in Indochina, and the seismically slow anomalies observed in the uppermost mantle throughout these regions (e.g., Schaeffer and Lebedev, 2013). While Roberts et al. (2018) found a Neogene–present-day swell of dynamic uplift around Borneo, and dynamic subsidence in the adjacent SCS, we note that the dynamic influence at the surface of this hot material will depend on the depth, wavelength, and lateral distribution of slabs in these regions (Colli et al., 2016). Future convection models which implement refined viscosity profiles may therefore reconcile the disparity between convection models (which often predict dynamic subsidence) and observations (which find dynamic uplift) highlighted by Roberts et al. (2018).
5.6 Further applications
While we have focussed our analysis on a single ridge subduction event during the early Cenozoic, we note that the process proposed is generic. The hypothesis rests on the idea that hot asthenospheric material can be driven from high-pressure upwellings to low-pressure subduction zones by a combination of pressure- and plate-driven flow (Höink and Lenardic, 2010; Colli et al., 2018), which may then spill over into adjacent regions following ridge subduction events and the opening of slab windows. This mechanism may therefore be more widely applicable in future studies of dynamic topography and intraplate volcanism, as similar events could have occurred throughout Earth’s history. Indeed, this process has been proposed in order to explain the intraplate volcanism (Zhou et al., 2018) and dynamic uplift (Zhou and Liu, 2019) in the western United States during the late Cenozoic, following the opening of tears in the Juan de Fuca slab and the Juan de Fuca-Pacific slab window. Furthermore, the asthenospheric build-up stage of this process may be invoked at circum-Pacific margins in the present day, and evidenced by seismically slow anomalies beneath subducting slabs in Japan (Ma J. et al., 2019; Bogiatzis et al., 2019), Cascadia (Hawley et al., 2016; Bodmer et al., 2018, 2020), and South America (Portner et al., 2017).
6 Conclusion
We have proposed and investigated a new component of the Cenozoic geodynamics of East Asia, whereby hot asthenospheric material from the Pacific domain flows through the Izanagi-Pacific slab window during the early Cenozoic. This mechanism provides a new explanation for the seismically slow anomalies (e.g., Schaeffer and Lebedev, 2013), late Cenozoic intraplate volcanism (Ball et al., 2021), and swells of present-day dynamic uplift (Hoggard et al., 2021) observed throughout this region. This effect arises naturally in the global mantle circulation models of Lin et al. (2020), which we have tested against a wide variety of geological observations. A good match was found between the predicted distribution of this hot material through time and the timing and location of intraplate volcanic eruptions in East Asia since 30 Ma. This influx predicts a broad band of dynamic uplift in eastern China during the Oligocene, which aligns spatially and temporally with an observed inter-regional, non-compressional sedimentary hiatus. Subsequently, the material spreads further into the SCB, inducing widespread dynamic uplift which coincides with a regional Neogene erosion event inferred from numerous AFT studies. The hot material flows below the northern margin of the SCS during the late Cenozoic, and matches the timing of anomalous uplift in the QDNB found by Zhao (2021) to first order, although the modelled amplitude of uplift tends to fall short by ∼500 m. The connection found between these independent geological observations and the models of Lin et al. (2020) shows the proposed mechanism to be a viable new component in the Cenozoic geodynamic development of East Asia.
The comparisons presented here have provided new constraints on the uncertain history of the PSP. Due to its ∼30 Ma onset of subduction along the Eurasian margin, the larger PSP implemented in Models 1a & 1b was found to predict large-scale mantle downwelling in eastern China during the Oligocene and Miocene. This downwelling, and the resulting dynamic subsidence, is inconsistent with observations of OIB-type magmatism and uplift during this time. In contrast, the small PSP implemented in Models 2a & 2b, which has a much later onset of subduction at ∼12 Ma, allows the influx of hot asthenospheric material to dominate over a larger region during the late Cenozoic. This provides an excellent fit to the observations of uplift and intraplate volcanism considered here. We therefore find new support for tectonic reconstructions which implement a small PSP and the consequent existence of the hypothesised “East Asian Sea” slabs.
Future improvements of the implemented viscosity profile, which will be allowed by the next generation of mantle convection codes, are likely to result in this influx of hot material flowing further westward during the late Cenozoic—making up for the ∼1,000 km shortfall found in this study. Along with the connections presented in this paper, this hypothesis therefore has the potential to explain a variety of observations of late Cenozoic dynamic uplift and magmatism in East Asia which were not considered here, such as the previously unexplained dynamic uplift and basaltic magmatism in Borneo since the Neogene (Roberts et al., 2018). Beyond the regional setting of Cenozoic East Asia, the geodynamic mechanism proposed here may be more widely applicable to subduction zone dynamics throughout Earth’s history.
Data availability statement
The original contributions presented in the study are included in the article/Supplementary Material, further inquiries can be directed to the corresponding author.
Author contributions
Data was collated from published literature by HB under the supervision of LC. All figures were produced by HB under the supervision of LC and HPB. The initial draft of manuscript was written by HB, and was reviewed and edited by LC and HB. The manuscript was restructured and further edited following suggestions from HPB.
Funding
The initial conception of this study arose from the Master’s research of HB at LMU Munich, which was funded by a scholarship from the Deutscher Akademischer Austauschdienst (DAAD). LC acknowledges funding from the State of Texas Governor’s University Research Initiative (GURI).
Acknowledgments
HB sincerely thanks Bernhard Schuberth for help with extracting temperature data from the MCMs (Figure 5), and Zhongxian Zhao for kindly providing data for the anomalous uplift of the QDNB (Figure 8). LC thanks Jonny Wu, Yi-An Lin, Yiduo Liu, and John Suppe for insightful discussions on East Asian geology, tectonics and kinematics. All authors thank Jonny Wu and a reviewer for helpful comments on the manuscript.
Conflict of interest
The authors declare that the research was conducted in the absence of any commercial or financial relationships that could be construed as a potential conflict of interest.
Publisher’s note
All claims expressed in this article are solely those of the authors and do not necessarily represent those of their affiliated organizations, or those of the publisher, the editors and the reviewers. Any product that may be evaluated in this article, or claim that may be made by its manufacturer, is not guaranteed or endorsed by the publisher.
Supplementary material
The Supplementary Material for this article can be found online at: https://www.frontiersin.org/articles/10.3389/feart.2022.889907/full#supplementary-material
References
Arai, S., Kida, M., Abe, N., and Yurimoto, H. (2001). Petrology of peridotite xenoliths in alkali basalt (11 Ma) from Boun, Korea: An insight into the upper mantle beneath the east Asian continental margin. J. Mineralogical Petrological Sci. 96, 89–99. doi:10.2465/jmps.96.89
Ball, P., White, N., Maclennan, J., and Stephenson, S. (2021). Global influence of mantle temperature and plate thickness on intraplate volcanism. Nat. Commun. 12, 2045. doi:10.1038/s41467-021-22323-9
Bauer, S., Bunge, H.-P., Drzisga, D., Ghelichkhan, S., Huber, M., Kohl, N., et al. (2020). “TerraNeo—Mantle Convection Beyond a Trillion Degrees of Freedom,” in Software for Exascale Computing - SPPEXA 2016-2019. Lecture Notes in Computational Science and Engineering. Editors H. J. Bungartz, S. Reiz, B. Uekermann, P. Neumann, and W. Nagel (Cham: Springer) 136, 569–610. doi:10.1007/978-3-030-47956-5_19
Bello, L., Coltice, N., Tackley, P. J., Müller, R. D., and Cannon, J. (2015). Assessing the role of slab rheology in coupled plate-mantle convection models. Earth Planet. Sci. Lett. 430, 191–201. doi:10.1016/j.epsl.2015.08.010
Bodmer, M., Toomey, D. R., Hooft, E. E., and Schmandt, B. (2018). Buoyant asthenosphere beneath Cascadia influences megathrust segmentation. Geophys. Res. Lett. 45, 6954–6962. doi:10.1029/2018GL078700
Bodmer, M., Toomey, D., Roering, J., and Karlstrom, L. (2020). Asthenospheric buoyancy and the origin of high-relief topography along the Cascadia forearc. Earth Planet. Sci. Lett. 531, 115965. doi:10.1016/j.epsl.2019.115965
Bogiatzis, P., Ishii, M., and Davis, T. A. (2019). The Dulmage–Mendelsohn permutation in seismic tomography. Geophys. J. Int. 218, 1157–1173. doi:10.1093/gji/ggz216
Bunge, H.-P., and Baumgardner, J. R. (1995). Mantle convection modeling on parallel virtual machines. Comput. Phys. 9, 207. doi:10.1063/1.168525
Bunge, H.-P., Hagelberg, C., and Travis, B. (2003). Mantle circulation models with variational data assimilation: Inferring past mantle flow and structure from plate motion histories and seismic tomography. Geophys. J. Int. 152, 280–301. doi:10.1046/j.1365-246x.2003.01823.x
Bunge, H.-P., Richards, M. A., Lithgow-Bertelloni, C., Baumgardner, J. R., Grand, S. P., Romanowicz, B. A., et al. (1998). Time scales and heterogeneous structure in geodynamic Earth models. Science 280, 91–95. doi:10.1126/science.280.5360.91
Bunge, H.-P., Richards, M., and Baumgardner, J. (2002). Mantle circulation models with sequential data assimilation: Inferring present–day mantle structure from plate motion histories. Philosophical Trans. R. Soc. Lond. Ser. A Math. Phys. Eng. Sci. 360, 2545–2567. doi:10.1098/rsta.2002.1080
Cao, X., Flament, N., Müller, D., and Li, S. (2018). The dynamic topography of eastern China since the latest Jurassic Period. Tectonics 37, 1274–1291. doi:10.1029/2017TC004830
Carena, S., Bunge, H.-P., and Friedrich, A. M. (2019). Analysis of geological hiatus surfaces across Africa in the Cenozoic and implications for the timescales of convectively-maintained topography. Can. J. Earth Sci. 56, 1333–1346. doi:10.1139/cjes-2018-0329
Charvet, J., Lapierre, H., and Yu, Y. (1994). Geodynamic significance of the Mesozoic volcanism of southeastern China. J. Southeast Asian Earth Sci. 9, 387–396. doi:10.1016/0743-9547(94)90050-7
Chase, C. G. (1979). Asthenospheric counterflow: A kinematic model. Geophys. J. Int. 56, 1–18. doi:10.1111/j.1365-246X.1979.tb04764.x
Chen, Y.-W., Colli, L., Bird, D. E., Wu, J., and Zhu, H. (2021). Caribbean plate tilted and actively dragged eastwards by low-viscosity asthenospheric flow. Nat. Commun. 12, 1603. doi:10.1038/s41467-021-21723-1
Chen, Y., Zhang, Y., Graham, D., Su, S., and Deng, J. (2007). Geochemistry of Cenozoic basalts and mantle xenoliths in Northeast China. Lithos 96, 108–126. doi:10.1016/j.lithos.2006.09.015
Choi, S. H., Mukasa, S. B., Kwon, S.-T., and Andronikov, A. V. (2006). Sr, Nd, Pb and Hf isotopic compositions of late Cenozoic alkali basalts in South Korea: Evidence for mixing between the two dominant asthenospheric mantle domains beneath East Asia. Chem. Geol. 232, 134–151. doi:10.1016/j.chemgeo.2006.02.014
Chung, S.-L., Jahn, B.-M., Chen, S.-J., Lee, T., and Chen, C.-H. (1995). Miocene basalts in northwestern Taiwan: Evidence for EM-type mantle sources in the continental lithosphere. Geochimica Cosmochimica Acta 59, 549–555. doi:10.1016/0016-7037(94)00360-X
Chung, S.-L. (1999). Trace element and isotope characteristics of Cenozoic basalts around the Tanlu fault with implications for the eastern plate boundary between north and South China. J. Geol. 107, 301–312. doi:10.1086/314348
Clements, B., Burgess, P. M., Hall, R., and Cottam, M. A. (2011). Subsidence and uplift by slab-related mantle dynamics: A driving mechanism for the late cretaceous and Cenozoic evolution of continental SE Asia? Geol. Soc. Lond. Spec. Publ. 355, 37–51. doi:10.1144/SP355.3
Clift, P. D., and Sun, Z. (2006). The sedimentary and tectonic evolution of the Yinggehai–Song Hong basin and the southern Hainan margin, South China Sea: Implications for Tibetan uplift and monsoon intensification. J. Geophys. Res. 111. doi:10.1029/2005JB004048
Cohen, K. M., Finney, S. C., Gibbard, P. L., and Fan, J.-X. (2013). The ICS international chronostratigraphic chart. Episodes 36, 199–204. doi:10.18814/epiiugs/2013/v36i3/002
Colli, L., Bunge, H.-P., and Schuberth, B. S. (2015). On retrodictions of global mantle flow with assimilated surface velocities. Geophys. Res. Lett. 42, 8341–8348. doi:10.1002/2015GL066001
Colli, L., Ghelichkhan, S., Bunge, H.-P., and Oeser, J. (2018). Retrodictions of Mid Paleogene mantle flow and dynamic topography in the Atlantic region from compressible high resolution adjoint mantle convection models: Sensitivity to deep mantle viscosity and tomographic input model. Gondwana Res. 53, 252–272. doi:10.1016/j.gr.2017.04.027
Colli, L., Ghelichkhan, S., and Bunge, H.-P. (2016). On the ratio of dynamic topography and gravity anomalies in a dynamic Earth. Geophys. Res. Lett. 43, 2510–2516. doi:10.1002/2016GL067929
Colli, L., Stotz, I., Bunge, H.-P., Smethurst, M., Clark, S., Iaffaldano, G., et al. (2014). Rapid South Atlantic spreading changes and coeval vertical motion in surrounding continents: Evidence for temporal changes of pressure-driven upper mantle flow. Tectonics 33, 1304–1321. doi:10.1002/2014TC003612
Cukur, D., Horozal, S., Kim, D. C., and Han, H. C. (2011). Seismic stratigraphy and structural analysis of the northern East China Sea Shelf Basin interpreted from multi-channel seismic reflection data and cross-section restoration. Mar. Petroleum Geol. 28, 1003–1022. doi:10.1016/j.marpetgeo.2011.01.002
Dong, M., Zhang, J., Brune, S., Wu, S., Fang, G., Yu, L., et al. (2020). Quantifying postrift lower crustal flow in the northern margin of the South China Sea. J. Geophys. Res. Solid Earth 125, e2019JB018910. doi:10.1029/2019JB018910
Fraters, M. R., Bangerth, W., Thieulot, C., Glerum, A., and Spakman, W. (2019). Efficient and practical Newton solvers for non-linear Stokes systems in geodynamic problems. Geophys. J. Int. 218, 873–894. doi:10.1093/gji/ggz183
French, S. W., and Romanowicz, B. (2015). Broad plumes rooted at the base of the Earth’s mantle beneath major hotspots. Nature 525, 95–99. doi:10.1038/nature14876
Friedrich, A. M., Bunge, H.-P., Rieger, S. M., Colli, L., Ghelichkhan, S., Nerlich, R., et al. (2018). Stratigraphic framework for the plume mode of mantle convection and the analysis of interregional unconformities on geological maps. Gondwana Res. 53, 159–188. doi:10.1016/j.gr.2017.06.003
Friedrich, A. M. (2019). Palaeogeological hiatus surface mapping: A tool to visualize vertical motion of the continents. Geol. Mag. 156, 308–319. doi:10.1017/S0016756818000560
Fuh, S.-C. (2000). Magnitude of Cenozoic erosion from mean sonic transit time, offshore Taiwan. Mar. Petroleum Geol. 17, 1011–1028. doi:10.1016/S0264-8172(00)00035-0
Fyhn, M. B., Boldreel, L. O., and Nielsen, L. H. (2009). Geological development of the central and south Vietnamese margin: Implications for the establishment of the South China Sea, Indochinese escape tectonics and Cenozoic volcanism. Tectonophysics 478, 184–214. doi:10.1016/j.tecto.2009.08.002
Ghelichkhan, S., and Bunge, H.-P. (2018). The adjoint equations for thermochemical compressible mantle convection: Derivation and verification by twin experiments. Proc. R. Soc. A 474, 20180329. doi:10.1098/rspa.2018.0329
Ghelichkhan, S., and Bunge, H.-P. (2016). The compressible adjoint equations in geodynamics: Derivation and numerical assessment. Int. J. Geomath. 7, 1–30. doi:10.1007/s13137-016-0080-5
Ghelichkhan, S., Bunge, H., and Oeser, J. (2021). Global mantle flow retrodictions for the early Cenozoic using an adjoint method: Evolving dynamic topographies, deep mantle structures, flow trajectories and sublithospheric stresses. Geophys. J. Int. 226, 1432–1460. doi:10.1093/gji/ggab108
Gong, J., and Chen, J. Y. (2014). Evidence of lateral asthenosphere flow beneath the South China Craton driven by both Pacific plate subduction and the India–Eurasia continental collision. Terra nova. 26, 55–63. doi:10.1111/ter.12069
Hall, R. (2002). Cenozoic geological and plate tectonic evolution of SE Asia and the SW pacific: Computer-based reconstructions, model and animations. J. Asian Earth Sci. 20, 353–431. doi:10.1016/S1367-9120(01)00069-4
Hall, R., and Spakman, W. (2015). Mantle structure and tectonic history of SE Asia. Tectonophysics 658, 14–45. doi:10.1016/j.tecto.2015.07.003
Hartley, R. A., Roberts, G. G., White, N. J., and Richardson, C. (2011). Transient convective uplift of an ancient buried landscape. Nat. Geosci. 4, 562–565. doi:10.1038/ngeo1191
Hawley, W. B., Allen, R. M., and Richards, M. A. (2016). Tomography reveals buoyant asthenosphere accumulating beneath the Juan de Fuca plate. Science 353, 1406–1408. doi:10.1126/science.aad8104
Hayek, J. N., Vilacís, B., Bunge, H.-P., Friedrich, A. M., Carena, S., Vibe, Y., et al. (2020). Continent-scale hiatus maps for the Atlantic Realm and Australia since the Upper Jurassic and links to mantle flow induced dynamic topography. Proc. R. Soc. A 476, 20200390. doi:10.1098/rspa.2020.0390
Hayek, J. N., Vilacís, B., Bunge, H.-P., Friedrich, A. M., Carena, S., Vibe, Y., et al. (2021). Correction: Continent-scale hiatus maps for the Atlantic Realm and Australia since the Upper Jurassic and links to mantle flow-induced dynamic topography. Proc. R. Soc. A 477, 20210437. doi:10.1098/rspa.2021.0437
He, C., and Santosh, M. (2021). Mantle upwelling beneath the Cathaysia Block, South China. Tectonics 40, e2020TC006447. doi:10.1029/2020TC006447
Ho, K.-s., Chen, J.-c., Lo, C.-h., and Zhao, H.-l. (2003). 40Ar–39Ar dating and geochemical characteristics of late Cenozoic basaltic rocks from the zhejiang–fujian region, SE China: Eruption ages, magma evolution and petrogenesis. Chem. Geol. 197, 287–318. doi:10.1016/S0009-2541(02)00399-6
Hoggard, M., Austermann, J., Randel, C., and Stephenson, S. (2021). Observational estimates of dynamic topography through space and time. Mantle Convect. Surf. expressions, 371–411. doi:10.1002/9781119528609.ch15
Hoggard, M. J., Winterbourne, J., Czarnota, K., and White, N. (2017). Oceanic residual depth measurements, the plate cooling model, and global dynamic topography. J. Geophys. Res. Solid Earth 122, 2328–2372. doi:10.1002/2016JB013457
Höink, T., Jellinek, A. M., and Lenardic, A. (2011). Viscous coupling at the lithosphere-asthenosphere boundary. Geochem. Geophys. Geosyst. 12. doi:10.1029/2011GC003698
Höink, T., and Lenardic, A. (2010). Long wavelength convection, Poiseuilleâ“Couette flow in the low-viscosity asthenosphere and the strength of plate margins. Geophys. J. Int. 180, 23–33. doi:10.1111/j.1365-246X.2009.04404.x
Höink, T., Lenardic, A., and Richards, M. (2012). Depth-dependent viscosity and mantle stress amplification: Implications for the role of the asthenosphere in maintaining plate tectonics. Geophys. J. Int. 191, 30–41. doi:10.1111/j.1365-246X.2012.05621.x
Höink, T., and Lenardic, A. (2008). Three-dimensional mantle convection simulations with a low-viscosity asthenosphere and the relationship between heat flow and the horizontal length scale of convection. Geophys. Res. Lett. 35. doi:10.1029/2008GL033854
Holloway, N. (1982). North Palawan Block, Philippines—Its relation to Asian mainland and role in evolution of South China sea. Am. Assoc. Pet. Geol. Bull. 66, 1355–1383. doi:10.1306/03B5A7A5-16D1-11D7-8645000102C1865D
Horbach, A., Bunge, H.-P., and Oeser, J. (2014). The adjoint method in geodynamics: Derivation from a general operator formulation and application to the initial condition problem in a high resolution mantle circulation model. Int. J. Geomath. 5, 163–194. doi:10.1007/s13137-014-0061-5
Hosseini, K., Sigloch, K., Tsekhmistrenko, M., Zaheri, A., Nissen-Meyer, T., Igel, H., et al. (2020). Global mantle structure from multifrequency tomography using P, PP and P-diffracted waves. Geophys. J. Int. 220, 96–141. doi:10.1093/gji/ggz394
Huang, C.-Y., Yen, Y., Zhao, Q., and Lin, C.-T. (2012). Cenozoic stratigraphy of Taiwan: Window into rifting, stratigraphy and paleoceanography of South China Sea. Chin. Sci. Bull. 57, 3130–3149. doi:10.1007/s11434-012-5349-y
Huang, J. (2014). P- and S-wave tomography of the Hainan and surrounding regions: Insight into the Hainan plume. Tectonophysics 633, 176–192. doi:10.1016/j.tecto.2014.07.007
Ismail-Zadeh, A., Schubert, G., Tsepelev, I., and Korotkii, A. (2004). Inverse problem of thermal convection: Numerical approach and application to mantle plume restoration. Phys. Earth Planet. Interiors 145, 99–114. doi:10.1016/j.pepi.2004.03.006
Jarvis, G. T., and McKenzie, D. P. (1980a). Convection in a compressible fluid with infinite Prandtl number. J. Fluid Mech. 96, 515–583. doi:10.1017/S002211208000225X
Jarvis, G. T., and McKenzie, D. P. (1980b). Sedimentary basin formation with finite extension rates. Earth Planet. Sci. Lett. 48, 42–52. doi:10.1016/0012-821X(80)90168-5
Jiaodong, Z., Xuanhua, C., Qiuli, L., Chengzhai, L., Bing, L., Jie, L., et al. (2012). Mesozoic “red beds” and its evolution in the Hefei Basin. Acta Geol. Sinica-English Ed. 86, 1060–1076. doi:10.1111/j.1755-6724.2012.00731.x
Kimura, J.-I., Sakuyama, T., Miyazaki, T., Vaglarov, B. S., Fukao, Y., Stern, R. J., et al. (2018). Plume-stagnant slab-lithosphere interactions: Origin of the late Cenozoic intra-plate basalts on the east Eurasia margin. Lithos 300, 227–249. doi:10.1016/j.lithos.2017.12.003
Kuritani, T., Xia, Q.-K., Kimura, J.-I., Liu, J., Shimizu, K., Ushikubo, T., et al. (2019). Buoyant hydrous mantle plume from the mantle transition zone. Sci. Rep. 9, 6549. doi:10.1038/s41598-019-43103-y
Lees, M. E., Rudge, J. F., and McKenzie, D. (2020). Gravity, topography, and melt generation rates from simple 3-D models of mantle convection. Geochem. Geophys. Geosyst. 21, e2019GC008809. doi:10.1029/2019GC008809
Lei, J., Zhao, D., Steinberger, B., Wu, B., Shen, F., Li, Z., et al. (2009). New seismic constraints on the upper mantle structure of the Hainan plume. Phys. Earth Planet. Interiors 173, 33–50. doi:10.1016/j.pepi.2008.10.013
Li, C., van der Hilst, R. D., Engdahl, E. R., and Burdick, S. (2008). A new global model for P wave speed variations in Earth’s mantle. Geochem. Geophys. Geosyst. 9 (5). doi:10.1029/2007GC001806
Li, X., and Zou, H. (2017). Late cretaceous–Cenozoic exhumation of the southeastern margin of coastal mountains, SE China, revealed by fission-track thermochronology: Implications for the topographic evolution. Solid Earth Sci. 2, 79–88. doi:10.1016/j.sesci.2017.02.001
Lin, A. T., Liu, C.-S., Lin, C.-C., Schnurle, P., Chen, G.-Y., Liao, W.-Z., et al. (2008). Tectonic features associated with the overriding of an accretionary wedge on top of a rifted continental margin: An example from Taiwan. Mar. Geol. 255, 186–203. doi:10.1016/j.margeo.2008.10.002
Lin, A., Watts, A. B., and Hesselbo, S. (2003). Cenozoic stratigraphy and subsidence history of the South China Sea margin in the Taiwan region. Basin Res. 15, 453–478. doi:10.1046/j.1365-2117.2003.00215.x
Lin, Y.-A., Colli, L., and Wu, J. (2021). NW Pacific-Panthalassa intra-oceanic subduction during Mesozoic times from mantle convection and geoid models (in press). Earth Space Sci. Open Archive ESSOAr. doi:10.1002/essoar.10506879.1
Lin, Y.-A., Colli, L., Wu, J., and Schuberth, B. S. (2020). Where are the proto-South China sea slabs? SE asian plate tectonics and mantle flow history from global mantle convection modeling. JGR. Solid Earth 125, e2020JB019758. doi:10.1029/2020JB019758
Liu, J.-q., Chen, S.-s., Guo, Z.-f., Guo, W.-f., He, H.-y., You, H.-t., et al. (2015). Geological background and geodynamic mechanism of Mt. Changbai volcanoes on the China–Korea border. Lithos 236–237, 46–73. doi:10.1016/j.lithos.2015.08.011
Liu, J., Han, J., and Fyfe, W. S. (2001). Cenozoic episodic volcanism and continental rifting in Northeast China and possible link to Japan Sea development as revealed from K–Ar geochronology. Tectonophysics 339, 385–401. doi:10.1016/S0040-1951(01)00132-9
Ma, J., Tian, Y., Zhao, D., Liu, C., and Liu, T. (2019). Mantle dynamics of Western pacific and east Asia: New insights from P wave anisotropic tomography. Geochem. Geophys. Geosyst. 20, 3628–3658. doi:10.1029/2019GC008373
Ma, P., Liu, S., Gurnis, M., and Zhang, B. (2019). Slab horizontal subduction and slab tearing beneath East Asia. Geophys. Res. Lett. 46, 5161–5169. doi:10.1029/2018GL081703
Mao, W., and Zhong, S. (2018). Slab stagnation due to a reduced viscosity layer beneath the mantle transition zone. Nat. Geosci. 11, 876–881. doi:10.1038/s41561-018-0225-2
Matthews, K. J., Maloney, K. T., Zahirovic, S., Williams, S. E., Seton, M., Mueller, R. D., et al. (2016). Global plate boundary evolution and kinematics since the late Paleozoic. Glob. Planet. Change 146, 226–250. doi:10.1016/j.gloplacha.2016.10.002
McKenzie, D. (1978). Some remarks on the development of sedimentary basins. Earth Planet. Sci. Lett. 40, 25–32. doi:10.1016/0012-821X(78)90071-7
Moresi, L., and Solomatov, V. (1998). Mantle convection with a brittle lithosphere: Thoughts on the global tectonic styles of the Earth and venus. Geophys. J. Int. 133, 669–682. doi:10.1046/j.1365-246X.1998.00521.x
Müller, R. D., Seton, M., Zahirovic, S., Williams, S. E., Matthews, K. J., Wright, N. M., et al. (2016). Ocean basin evolution and global-scale plate reorganization events since Pangea breakup. Annu. Rev. Earth Planet. Sci. 44, 107–138. doi:10.1146/annurev-earth-060115-012211
Nerlich, R., Colli, L., Ghelichkhan, S., Schuberth, B., and Bunge, H.-P. (2016). Constraining central Neo-Tethys ocean reconstructions with mantle convection models. Geophys. Res. Lett. 43, 9595–9603. doi:10.1002/2016GL070524
Ogg, J. G., Ogg, G., and Gradstein, F. M. (2016). “1. Introduction,” in A concise geologic time scale (Elsevier), 1–8.
Parnell-Turner, R., White, N. J., Henstock, T., Murton, B., Maclennan, J., Jones, S. M., et al. (2014). A continuous 55-million-year record of transient mantle plume activity beneath Iceland. Nat. Geosci. 7, 914–919. doi:10.1038/ngeo2281
Peng, D., Liu, L., Hu, J., Li, S., and Liu, Y. (2021). Formation of East Asian stagnant slabs due to a pressure-driven Cenozoic mantle wind following Mesozoic subduction. Geophys. Res. Lett. 48, e2021GL094638. doi:10.1029/2021GL094638
Petit, C., and Deverchere, J. (2006). Structure and evolution of the Baikal rift: A synthesis. Geochem. Geophys. Geosyst. 7. doi:10.1029/2006GC001265
Portner, D. E., Beck, S., Zandt, G., and Scire, A. (2017). The nature of subslab slow velocity anomalies beneath South America. Geophys. Res. Lett. 44, 4747–4755. doi:10.1002/2017GL073106
Qi, J., and Yang, Q. (2010). Cenozoic structural deformation and dynamic processes of the Bohai Bay Basin province, China. Mar. Petroleum Geol. 27, 757–771. doi:10.1016/j.marpetgeo.2009.08.012
Qiu, L., Yan, D.-P., Tang, S.-L., Chen, F., Gong, L.-X., Zhang, Y.-X., et al. (2020). Cenozoic exhumation of the neoproterozoic sanfang batholith in South China. J. Geol. Soc. 177, 412–423. doi:10.1144/jgs2019-041
Richard, G. C., and Iwamori, H. (2010). Stagnant slab, wet plumes and Cenozoic volcanism in East Asia. Phys. Earth Planet. Interiors 183, 280–287. doi:10.1016/j.pepi.2010.02.009
Richards, M. A., Duncan, R. A., and Courtillot, V. E. (1989). Flood basalts and hot-spot tracks: Plume heads and tails. Science 246, 103–107. doi:10.1126/science.246.4926.103
Richards, M. A., and Hager, B. H. (1984). Geoid anomalies in a dynamic Earth. J. Geophys. Res. 89, 5987–6002. doi:10.1029/JB089iB07p05987
Richards, M. A., Hager, B. H., and Sleep, N. H. (1988). Dynamically supported geoid highs over hotspots: Observation and theory. J. Geophys. Res. 93, 7690. doi:10.1029/JB093iB07p07690
Roberts, G. G., White, N., Hoggard, M. J., Ball, P. W., and Meenan, C. (2018). A Neogene history of mantle convective support beneath Borneo. Earth Planet. Sci. Lett. 496, 142–158. doi:10.1016/j.epsl.2018.05.043
Safonova, I. Y., and Santosh, M. (2014). Accretionary complexes in the Asia-pacific region: Tracing archives of Ocean plate stratigraphy and tracking mantle plumes. Gondwana Res. 25, 126–158. doi:10.1016/j.gr.2012.10.008
Schaeffer, A., and Lebedev, S. (2013). Global shear speed structure of the upper mantle and transition zone. Geophys. J. Int. 194, 417–449. doi:10.1093/gji/ggt095
Schuberth, B. S., Bunge, H.-P., and Ritsema, J. (2009a). Tomographic filtering of high-resolution mantle circulation models: Can seismic heterogeneity be explained by temperature alone? Geochem. Geophys. Geosyst. 10. doi:10.1029/2009GC002401
Schuberth, B. S., Bunge, H.-P., Steinle-Neumann, G., Moder, C., and Oeser, J. (2009b). Thermal versus elastic heterogeneity in high-resolution mantle circulation models with pyrolite composition: High plume excess temperatures in the lowermost mantle. Geochem. Geophys. Geosyst. 10. doi:10.1029/2008GC002235
Sclater, J. G., and Christie, P. A. (1980). Continental stretching: An explanation of the post-mid-Cretaceous subsidence of the central North Sea basin. J. Geophys. Res. 85, 3711–3739. doi:10.1029/JB085iB07p03711
Seno, T., and Maruyama, S. (1984). Paleogeographic reconstruction and origin of the Philippine Sea. Tectonophysics. 102, 53–84. doi:10.1016/0040-1951(84)90008-8
Seton, M., Müller, R. D., Zahirovic, S., Gaina, C., Torsvik, T., Shephard, G., et al. (2012). Global continental and ocean basin reconstructions since 200 Ma. Earth-Science Rev. 113, 212–270. doi:10.1016/j.earscirev.2012.03.002
Shi, X., Kohn, B., Spencer, S., Guo, X., Li, Y., Yang, X., et al. (2011). Cenozoic denudation history of southern Hainan Island, South China Sea: Constraints from low temperature thermochronology. Tectonophysics 504, 100–115. doi:10.1016/j.tecto.2011.03.007
Shi, X., Xu, H., Qiu, X., Xia, K., Yang, X., Li, Y., et al. (2008). Numerical modeling on the relationship between thermal uplift and subsequent rapid subsidence: Discussions on the evolution of the Tainan Basin. Tectonics 27. doi:10.1029/2007TC002163
Song, Y., Stepashko, A., Liu, K., He, Q., Shen, C., Shi, B., et al. (2018). Post-rift tectonic history of the Songliao Basin, NE China: Cooling events and post-rift unconformities driven by orogenic pulses from plate boundaries. J. Geophys. Res. Solid Earth 123, 2363–2395. doi:10.1002/2017JB014741
Steinshouer, D. W., Qiang, J., McCabe, P. J., and Ryder, R. T. (1999). Maps showing geology, oil and gas fields, and geologic provinces of the Asia Pacific region. Tech. Rep. U. S. Geol. Surv. Open-File Rep. 97–470. doi:10.3133/ofr97470F
Stephenson, S., Hoggard, M., and White, N. (2021). “Global continental residual topography,” in EGU general assembly conference abstracts (EGU General Assembly Conference Abstracts). doi:10.5194/egusphere-egu21-15505
Stotz, I. L., Vilacís, B., Hayek, J. N., Bunge, H.-P., and Friedrich, A. M. (2021). Yellowstone plume drives Neogene North American Plate motion change. Geophys. Res. Lett. 48, e2021GL095079. doi:10.1029/2021GL095079
Su, J., Dong, S., Zhang, Y., Li, Y., Chen, X., Li, J., et al. (2017). Apatite fission track geochronology of the southern hunan province across the Shi-hang belt: Insights into the Cenozoic dynamic topography of South China. Int. Geol. Rev. 59, 981–995. doi:10.1080/00206814.2016.1240049
Sun, Z., Zhou, D., Sun, L., Chen, C., Pang, X., Jiang, J., et al. (2010). Dynamic analysis on rifting stage of Pearl River Mouth Basin through analogue modeling. J. Earth Sci. 21, 439–454. doi:10.1007/s12583-010-0106-0
Suppe, J. (1980). A retrodeformable cross section of northern Taiwan. Proc. Geol. Soc. China. 23, 46–55.
Tang, X., Zuo, Y., Kohn, B., Li, Y., and Huang, S. (2019). Cenozoic thermal history reconstruction of the dongpu sag, Bohai Bay Basin: Insights from apatite fission-track thermochronology. Terra nova. 31, 159–168. doi:10.1111/ter.12379
Tang, Y.-J., Zhang, H.-F., and Ying, J.-F. (2006). Asthenosphere–lithospheric mantle interaction in an extensional regime: Implication from the geochemistry of Cenozoic basalts from Taihang mountains, north China Craton. Chem. Geol. 233, 309–327. doi:10.1016/j.chemgeo.2006.03.013
Tao, N., Li, Z.-X., Danišík, M., Evans, N. J., Li, R.-X., Pang, C.-J., et al. (2019). Post-250 Ma thermal evolution of the central Cathaysia Block (SE China) in response to flat-slab subduction at the proto-Western Pacific margin. Gondwana Res. 75, 1–15. doi:10.1016/j.gr.2019.03.019
Vibe, Y., Friedrich, A. M., Bunge, H.-P., and Clark, S. (2018). Correlations of oceanic spreading rates and hiatus surface area in the North Atlantic realm. Lithosphere 10, 677–684. doi:10.1130/L736.1
Vynnytska, L., and Bunge, H.-P. (2015). Restoring past mantle convection structure through fluid dynamic inverse theory: Regularisation through surface velocity boundary conditions. Int. J. Geomath. 6, 83–100. doi:10.1007/s13137-014-0060-6
Wang, B., Doust, H., and Liu, J. (2019). Geology and petroleum systems of the East China Sea Basin. Energies 12, 4088. doi:10.3390/en12214088
Wang, F., Chen, H., Batt, G. E., Lin, X., Gong, J., Gong, G., et al. (2015). Tectonothermal history of the NE Jiangshan–Shaoxing suture zone: Evidence from 40Ar/39Ar and fission-track thermochronology in the Chencai region. Precambrian Res. 264, 192–203. doi:10.1016/j.precamres.2015.04.009
Wang, R., Shi, W., Xie, X., Wang, L., Manger, W., Busbey, A. B., et al. (2017). Lower Cretaceous lacustrine successions, North Yellow Sea Basin, eastern China: Rift basin sequence stratigraphy and stacking patterns in response to magmatic activity. Mar. Petroleum Geol. 88, 531–550. doi:10.1016/j.marpetgeo.2017.09.006
Wang, R., Shi, W., Xie, X., Zhang, X., Wang, L., Manger, W., et al. (2020). Coupling of strike-slip faulting and lacustrine basin evolution: Sequence stratigraphy, structure, and sedimentation in the north Yellow Sea basin (west Bay Basin offshore north Korea), eastern China. Mar. Petroleum Geol. 120, 104548. doi:10.1016/j.marpetgeo.2020.104548
Wang, Y., Wang, Y., Li, S., Seagren, E., Zhang, Y., Zhang, P., et al. (2020). Exhumation and landscape evolution in eastern South China since the Cretaceous: New insights from fission-track thermochronology. J. Asian Earth Sci. 191, 104239. doi:10.1016/j.jseaes.2020.104239
Wang, X.-C., Li, Z.-X., Li, X.-H., Li, J., Liu, Y., Long, W.-G., et al. (2012). Temperature, pressure, and composition of the mantle source region of late Cenozoic basalts in Hainan Island, SE Asia: A consequence of a young thermal mantle plume close to subduction zones? J. Petrology 53, 177–233. doi:10.1093/petrology/egr061
Ward, J. F., Rosenbaum, G., Ubide, T., Wu, J., Caulfield, J. T., Sandiford, M., et al. (2021). Geophysical and geochemical constraints on the origin of Holocene intraplate volcanism in East Asia. Earth-Science Rev. 218, 103624. doi:10.1016/j.earscirev.2021.103624
Weismüller, J., Gmeiner, B., Ghelichkhan, S., Huber, M., John, L., Wohlmuth, B., et al. (2015). Fast asthenosphere motion in high-resolution global mantle flow models. Geophys. Res. Lett. 42, 7429–7435. doi:10.1002/2015GL063727
Wheeler, P., and White, N. (2002). Measuring dynamic topography: An analysis of Southeast Asia. Tectonics 21, 4. doi:10.1029/2001TC900023
White, N. (1994). An inverse method for determining lithospheric strain rate variation on geological timescales. Earth Planet. Sci. Lett. 122, 351–371. doi:10.1016/0012-821X(94)90008-6
White, N., and Lovell, B. (1997). Measuring the pulse of a plume with the sedimentary record. Nature 387, 888–891. doi:10.1038/43151
Woods, M. T., and Davies, G. F. (1982). Late cretaceous Genesis of the kula plate. Earth Planet. Sci. Lett. 58, 161–166. doi:10.1016/0012-821X(82)90191-1
Wu, J., Suppe, J., Lu, R., and Kanda, R. (2016). Philippine Sea and East Asian plate tectonics since 52 Ma constrained by new subducted slab reconstruction methods. J. Geophys. Res. Solid Earth 121, 4670–4741. doi:10.1002/2016JB012923
Wu, J., and Suppe, J. (2018). Proto-South China Sea plate tectonics using subducted slab constraints from tomography. J. Earth Sci. 29, 1304–1318. doi:10.1007/s12583-017-0813-x
Wu, J. T.-J., and Wu, J. (2019). Izanagi-Pacific ridge subduction revealed by a 56 to 46 Ma magmatic gap along the Northeast Asian margin. Geology 47, 953–957. doi:10.1130/G46778.1
Xia, S., Zhao, D., Sun, J., and Huang, H. (2016). Teleseismic imaging of the mantle beneath southernmost China: New insights into the Hainan plume. Gondwana Res. 36, 46–56. doi:10.1016/j.gr.2016.05.003
Xie, X., Müller, R. D., Li, S., Gong, Z., and Steinberger, B. (2006). Origin of anomalous subsidence along the northern South China Sea margin and its relationship to dynamic topography. Mar. Petroleum Geol. 23, 745–765. doi:10.1016/j.marpetgeo.2006.03.004
Xie, X., Ren, J., Pang, X., Lei, C., and Chen, H. (2019). Stratigraphic architectures and associated unconformities of Pearl River Mouth Basin during rifting and lithospheric breakup of the South China Sea. Mar. Geophys. Res. 40, 129–144. doi:10.1007/s11001-019-09378-6
Xu, J., Ben-Avraham, Z., Kelty, T., and Yu, H.-S. (2014). Origin of marginal basins of the NW Pacific and their plate tectonic reconstructions. Earth-Science Rev. 130, 154–196. doi:10.1016/j.earscirev.2013.10.002
Yan, Q., Shi, X., Metcalfe, I., Liu, S., Xu, T., Kornkanitnan, N., et al. (2018). Hainan mantle plume produced late Cenozoic basaltic rocks in Thailand, Southeast Asia. Sci. Rep. 8, 2640. doi:10.1038/s41598-018-20712-7
Yan, Y., Yao, D., Tian, Z.-X., Huang, C.-Y., Dilek, Y., Clift, P. D., et al. (2018). Tectonic topography changes in Cenozoic East Asia: A landscape erosion-sediment archive in the South China Sea. Geochem. Geophys. Geosyst. 19, 1731–1750. doi:10.1029/2017GC007356
Yan, Q., Shi, X., Wang, K., Bu, W., and Xiao, L. (2008). Major element, trace element, and Sr, Nd and Pb isotope studies of Cenozoic basalts from the South China Sea. Sci. China Ser. D-Earth. Sci. 51, 550–566. doi:10.1007/s11430-008-0026-3
Yan, Y., Hu, X., Lin, G., Xia, B., Li, X., Patel, R. C., et al. (2009). Denudation history of South China Block and sediment supply to northern margin of the South China Sea. J. Earth Sci. 20, 57–65. doi:10.1007/s12583-009-0006-3
Yang, C. S., Li, S. Z., Li, G., Yang, C. Q., Yang, Y. Q., Dai, L. M., et al. (2016). Tectonic units and proto-basin of the East China Sea Shelf basin: Correlation to mesozoic subduction of the palaeo-Pacific Plate. Geol. J. 51, 149–161. doi:10.1002/gj.2776
Yang, F., Hu, P., Zhou, X., Zhang, R., Peng, Y., Li, X., et al. (2020). The late Jurassic to early Cretaceous strike-slip faults in the Subei-South Yellow Sea Basin, eastern China: Constraints from seismic data. Tectonics 39, e2020TC006091. doi:10.1029/2020TC006091
Yang, J., and Faccenda, M. (2020). Intraplate volcanism originating from upwelling hydrous mantle transition zone. Nature 579, 88–91. doi:10.1038/s41586-020-2045-y
Yao, Z., Li, C.-F., He, G., Tao, T., Zheng, X., Zhang, T., et al. (2020). Cenozoic sill intrusion in the central and southern East China Sea Shelf basin. Mar. Petroleum Geol. 119, 104465. doi:10.1016/j.marpetgeo.2020.104465
Zahirovic, S., Matthews, K. J., Flament, N., Müller, R. D., Hill, K. C., Seton, M., et al. (2016). Tectonic evolution and deep mantle structure of the eastern Tethys since the latest Jurassic. Earth-Science Rev. 162, 293–337. doi:10.1016/j.earscirev.2016.09.005
Zahirovic, S., Seton, M., and Müller, R. (2014). The Cretaceous and Cenozoic tectonic evolution of Southeast Asia. Solid earth. 5, 227–273. doi:10.5194/se-5-227-2014
Zhang, N., and Li, Z.-X. (2018). formation of mantle “lone plumes” in the global downwelling zone—a multiscale modelling of subduction-controlled plume generation beneath the South China Sea. Tectonophysics 723, 1–13. doi:10.1016/j.tecto.2017.11.038
Zhang, R., Yang, F., Zhao, C., Zhang, J., and Qiu, E. (2020). Coupling relationship between sedimentary basin and moho morphology in the South Yellow Sea, East China. Geol. J. 55, 6544–6561. doi:10.1002/gj.3821
Zhang, Y., Yu, K., Fan, T., Yue, Y., Wang, R., Jiang, W., et al. (2020). Geochemistry and petrogenesis of Quaternary basalts from Weizhou Island, northwestern South China Sea: Evidence for the Hainan plume. Lithos 362, 105493. doi:10.1016/j.lithos.2020.105493
Zhao, Z.-X. (2021). The deep mantle upwelling beneath the northwestern South China Sea: Insights from the time-varying residual subsidence in the Qiongdongnan Basin. Geosci. Front. 12, 101246. doi:10.1016/j.gsf.2021.101246
Zheng, H., Zhong, L.-F., Kapsiotis, A., Cai, G.-Q., Wan, Z.-F., Xia, B., et al. (2019). Post-spreading basalts from the Nanyue Seamount: Implications for the involvement of crustal-and plume-type components in the Genesis of the South China Sea mantle. Minerals 9, 378. doi:10.3390/min9060378
Zhou, Q., Liu, L., and Hu, J. (2018). Western US volcanism due to intruding oceanic mantle driven by ancient Farallon slabs. Nat. Geosci. 11, 70–76. doi:10.1038/s41561-017-0035-y
Zhou, Q., and Liu, L. (2019). Topographic evolution of the Western United States since the early Miocene. Earth Planet. Sci. Lett. 514, 1–12. doi:10.1016/j.epsl.2019.02.029
Zhou, W., Zhuo, H., Wang, Y., Xu, Q., and Li, D. (2020). post-rift submarine volcanic complexes and fault activities in the Baiyun sag, Pearl River Mouth Basin: New insights into the breakup sequence of the northern South China Sea. Mar. Geol. 430, 106338. doi:10.1016/j.margeo.2020.106338
Zhou, X., Jiang, Z., Quaye, J. A., Duan, Y., Hu, C., Liu, C., et al. (2019). Ichnology and sedimentology of the trace fossil-bearing fluvial red beds from the lowermost member of the Paleocene Funing Formation in the Jinhu Depression, Subei Basin, East China. Mar. Petroleum Geol. 99, 393–415. doi:10.1016/j.marpetgeo.2018.10.032
Keywords: East Asia, asthenospheric flow, dynamic topography, intraplate volcanism, slab window, ridge subduction, mantle circulation models, plate reconstructions
Citation: Brown H, Colli L and Bunge H-P (2022) Asthenospheric flow through the Izanagi-Pacific slab window and its influence on dynamic topography and intraplate volcanism in East Asia. Front. Earth Sci. 10:889907. doi: 10.3389/feart.2022.889907
Received: 04 March 2022; Accepted: 27 June 2022;
Published: 13 September 2022.
Edited by:
Lijun Liu, University of Illinois at Urbana-Champaign, United StatesReviewed by:
Bernhard Maximilian Steinberger, GFZ German Research Centre for Geosciences, GermanyJiashun Hu, Southern University of Science and Technology, China
Copyright © 2022 Brown, Colli and Bunge. This is an open-access article distributed under the terms of the Creative Commons Attribution License (CC BY). The use, distribution or reproduction in other forums is permitted, provided the original author(s) and the copyright owner(s) are credited and that the original publication in this journal is cited, in accordance with accepted academic practice. No use, distribution or reproduction is permitted which does not comply with these terms.
*Correspondence: Hamish Brown, aGJyb3duQGdlb3BoeXNpay51bmktbXVlbmNoZW4uZGU=