- 1Dipartimento di Geoscienze, Università di Padova, Padova, Italy
- 2Centre for Earth Evolution and Dynamics, University of Oslo, Oslo, Norway
- 3GeoZentrum Nordbayern, Universität Erlangen-Nurnberg, Erlangen, Germany
- 4Dipartimento Territorio e Sistemi Agro-Forestali, Università di Padova, Legnaro, Italy
The emplacement of the Siberian Traps, the Central Atlantic Magmatic Province (CAMP) and the Wrangellia have been linked to the end-Permian, the end-Triassic mass extinctions, and to the Carnian Pluvial Episode (CPE), respectively. Exploring the timing, eruptive styles, and volatile degassing of these Large Igneous Provinces (LIPs) is crucial to understand their causal link to the catastrophic environmental crises that punctuated the Triassic. In this study we review the main characteristics of these LIPs, emphasizing common features and differences, and discussing aspects that are still in debate. Estimates of CO2 budgets and emissions from the three LIPs are based on the Nb content of little evolved basalts and highlight that early Siberian Traps and CAMP and high-Ti Wrangellia volcanics were quite CO2-rich. On the contrary, other volcanics from the three LIPs probably emitted relatively low amounts of CO2, which reinforces the possibility that thermogenic reactions between sills and sediments were additional fundamental suppliers of climate-modifying gases.
Introduction
The Triassic was a crucial period for shaping the modern world, in terms of the evolution of both the biosphere and the geosphere. While successful faunas and floras spread over Pangea, in the Panthalassa and Tethys oceans, the Triassic biosphere was devastated by two extreme extinctions at its dawn (ca. 252 Ma) and its end (ca. 201 Ma) and by a combined extinction and significant radiation episode at ca. 232 Ma, during the Carnian (e.g., Tanner et al., 2004; Song et al., 2013; Bernardi et al., 2018; Dal Corso et al., 2020, 2022; Wignall and Atkinson 2020). The three main biocrises coincided with the emplacement of three Large Igneous Provinces (LIPs), namely, the Siberian Traps, the Central Atlantic Magmatic Province (CAMP), and the Wrangellia (Figure 1). In this article, we review the main features of these LIPs, with a focus on their timing, emplacement styles, and CO2 budgets. This is followed by an in-depth discussion of their critical roles in the Triassic environmental crises.
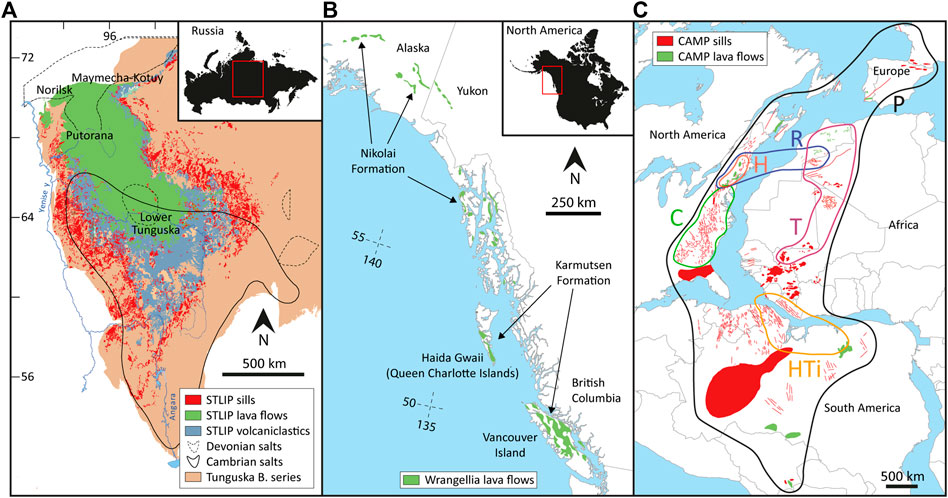
FIGURE 1. Simplified sketches of (A) the Siberian Traps (modified after Svensen et al., 2018) (B) the Wrangellia (modified after Lassiter et al., 1995), and (C) the CAMP (modified after Capriolo et al., 2020). (C) Coloured lines represent the geographic distribution of the six geochemical groups of the CAMP magmas identified by Marzoli et al. (2018): P=Prevalent, HTi=high-Ti, T=Tiourjdal, R=Recurrent, C=Carolina, H=Holyoke.
Continental Large Igneous Provinces: The Siberian Traps and the CAMP
High-precision geochronology proves that the main activity of the Siberian Traps and the CAMP (main pulses at 252.3–251.4 Ma and 201.6–201.0 Ma, respectively; for the Siberian Traps: Kamo et al., 2003; Burgess and Bowring, 2015; Burgess et al., 2017; Augland et al., 2019; for the CAMP: Blackburn et al., 2013; Davies et al., 2017, 2021; Marzoli et al., 2019) spanned the end-Permian and end-Triassic extinction intervals, respectively (251.94–251.88 Ma and 201.51–201.36 Ma; Schoene et al., 2010; Burgess et al., 2014; Wotzlaw et al., 2014). The pulsed nature of these LIPs was inferred from magnetostratigraphy and biostratigraphy (e.g., Knight et al., 2004; Panfili et al., 2019; Latyshev et al., 2020), yielding eruption rates possibly two orders of magnitude greater than the most voluminous present-day eruptions, such as those at Hawai’i (e.g., Patrick et al., 2020). The Siberian Traps and the CAMP are among the most voluminous known LIPs (both ca. 3 ± 1 million km3; Saunders and Reichow, 2009; Marzoli et al., 2018), although a precise estimate of the original and preserved magma volume remains challenging. Large parts of the Siberian Traps are intrusive, subaerial, and huge volumes of volcanics were likely removed by erosion. The best-studied part of the province is on the Siberian Craton, at a paleolatitude of ca. 50–60°N, over an area of ca. 2.5 million km2 (Figure 1A). The CAMP was emplaced over 10 million km2 straddling the equator, and has been similarly partly eroded away, as demonstrated by the sparsely preserved lava piles (Figure 1C).
The first emplacement stage of the Siberian Traps started shortly before 252.24 ± 0.12 Ma with initial pyroclastic eruptions followed by effusive activity emplaced in ca 0.30 Ma. From 251.91 ± 0.07 Ma, the extrusive activity waned and shifted to dominantly intrusive for ca. 0.40 Ma, building the vast network of sills in the Tunguska Basin. These tholeiitic magmas were produced by shallower and extensive melting of a mixed peridotitic-pyroxenitic mantle (Sobolev et al., 2011; Callegaro et al., 2021). Renewed extrusive activity is recognized from 251.48 ± 0.09 Ma, marking the beginning of the third phase of the Siberian Traps. During this phase, tholeiitic extrusive and intrusive activities were accompanied by mafic to felsic alkaline intrusions in Maymecha-Kotuy and Taimyr areas (Elkins-Tanton et al., 2007; Sobolev et al., 2009; Augland et al., 2019). Alkaline melts were produced from a deep (5.5 GPa) and volatile-rich (carbonated) mantle source (Elkins-Tanton et al., 2007). The youngest sill dated in Tunguska (251.35 ± 0.09 Ma; Burgess and Bowring, 2015), and the Guli carbonatitic complex in the Maymecha-Kotuy region (250.20 ± 0.30 Ma; Kamo et al., 2003) represent the youngest known Siberian Traps products.
The beginning of the second (intrusive) phase is considered as the deadly subinterval of the Siberian Traps (Burgess et al., 2017), since it coincides with the beginning of the extinction horizon at Meishan (251.94 ± 0.04 Ma; Burgess et al., 2014), Dongpan and Penglaitan (251.94 ± 0.03 Ma and 251.98 ± 0.03 Ma; Baresel et al., 2017), China. Even if these tholeiitic magmas were probably volatile poor (Sibik et al., 2015), the interaction between the sills and the sedimentary host-rocks (i.e., evaporites, carbonates, marlstones, and coal) likely produced large amounts of thermogenic carbon, sulfur and halocarbon species (Svensen et al., 2018; Callegaro et al., 2021; Sibik et al., 2021).
The end of the Triassic witnessed the emplacement of the CAMP mainly between 201.64 ± 0.03 Ma and 201.36 ± 0.02 Ma (Blackburn et al., 2013; Davies et al., 2017, 2021; Marzoli et al., 2019), coincident with the main end-Triassic extinction interval (201.51 ± 0.15 to 201.36 ± 0.15 Ma; Schoene et al., 2010; Wotzlaw et al., 2014). Basaltic magmas erupted as short-lived pulses (Knight et al., 2004; Marzoli et al., 2019) during this magmatic phase have been shown to be rich in CO2 and SO2 (Callegaro et al., 2014a; Capriolo et al., 2020) and possibly triggered the dramatic increase in CO2, the rise of temperature and the climatic perturbations heralding the end of the Triassic (Landwehrs et al., 2020; Lindström et al., 2021; Capriolo et al., 2022). Later erupted magmas, for example, in North America and Africa (Holyoke and Recurrent groups; Blackburn et al., 2013; Marzoli et al., 2018, 2019) appear to be less voluminous and possibly had limited environmental effects.
Similar to the Siberian Traps, CAMP magmas are mainly preserved as large sills intruding Paleozoic terrestrial sediments in South America and North-western Africa, and Triassic terrestrial sediments in North America and Europe (Marzoli et al., 2018 and references therein). The CAMP basalts mobilized large amounts of organic carbon and possibly halogens from evaporitic deposits (Heimdal et al., 2018, 2019; Capriolo et al., 2021). Available geochronological data indicate that some CAMP intrusions are older than the preserved (and dated) erupted basalts (Davies et al., 2017). However, the intrusion of sills in Amazonia and north-western Africa seems to be relatively prolonged from ca. 201.53 ± 0.07 Ma to ca. 201.35 ± 0.03 Ma (Davies et al., 2017, 2021), i.e., spanning the entire end-Triassic extinction interval (Schoene et al., 2010). However, it is still unclear whether CAMP intrusions occurred in pulses, thus emitting thermogenic volatiles at high rates, and playing a catastrophic role in the end-Triassic crisis.
Unlike the Siberian Traps, CAMP magmas are relatively uniform in composition, mainly being tholeiitic basalts. Whether such relatively high degree mantle melts originated from the deep or shallow mantle is still disputed (e.g., Ruiz-Martinez et al., 2012; Tegner et al., 2019; Boscaini et al., 2022). CAMP basalts lack geochemical signatures of a mantle plume originating from the deep mantle but show a subduction signature, which is best interpreted as reflecting the presence of recycled subducted continental rocks (sediments, mainly; Callegaro et al., 2013; Merle et al., 2014; Shellnutt et al., 2018; Marzoli et al., 2019). The absence of alkaline mafic rocks or carbonatites in the CAMP calls against a significant contribution from particularly CO2-rich metasomatized mantle portions. In contrast to the Siberian Traps, evidence for explosive volcanic activity is rare for the CAMP, whose lavas are mostly compound pahoehoe flows (e.g., El Hacimi et al., 2011) and therefore occurred as fissure eruptions like the historical Laki eruption in Iceland.
Oceanic Large Igneous Province: The Wrangellia
The Wrangellia LIP presently crops out along the north-western margin of North America (Figure 1B). It represents one of the best-preserved accreted oceanic plateaus on Earth, which contrast starkly with continental LIPs in terms of mantle processes, emplacement style and, possibly, environmental implications (Kerr, 2005).
The origin of the Wrangellia tholeiitic basalts has been attributed to deep mantle processes (i.e., mantle plume; Richards et al., 1991; Lassiter et al., 1995; Greene et al., 2009a; Greene et al., 2009b; Shellnutt et al., 2021). The early emplaced low-Ti basalts (0.4–1.2 TiO2 wt%) were probably sourced from partial melting of a rising mantle plume and show a significant contribution from the subduction-modified lithospheric mantle (Greene et al., 2009a; Greene et al., 2009b). Conversely, the later high-Ti basalts (1.4–2.4 TiO2 wt%) were sourced chiefly from the mantle plume (Greene et al., 2009a; Greene et al., 2009b).
As an oceanic plateau, the magmatic products of the Wrangellia are considerably different from those of continental LIPs like the Siberian Traps and the CAMP and include tholeiitic submarine and subaerial flows. Few sills beneath and interbedded with the lavas are also present. A total volume of at least 1 million km3 was estimated for the entire LIP (Greene et al., 2010). Pillow lavas at the base of the volcanic succession are ubiquitous over the entire province. However, they form thinner sequences (∼500 vs. ∼2,500 m) and are highly vesicular in Alaska and Yukon compared to south-western Canada (Vancouver Island), suggesting a southward deepening of the emplacement depth (Greene et al., 2010). This is consistent with subaerial pahoehoe lavas being relatively more abundant in Alaska and Yukon, where they pile up to 3,500 m (Greene et al., 2010).
The age of the Wrangellia is poorly constrained. A maximum time span of ca. 2 Ma has been inferred from magnetostratigraphic studies (Greene et al., 2010 and references therein). Valuable constraints are offered by biostratigraphy. Daonella-bearing shales unconformably underlie the lowest basalts of the volcanic sequence. Daonella would indicate a maximum Ladinian age (Smith and MacKevet, 1970; Brack et al., 2005), but Daonella is also known from younger Triassic strata (e.g., Fürsich and Wendt, 1977). The termination of Wrangellia volcanism was marked by the establishment of stable carbonate production on top of volcanic successions and was geographically diachronous. On Vancouver Island, the uppermost basalts of the Karmutsen Formation are interbedded with sediments bearing ammonoids and bivalve Halobia of the Tuvalian 1 dilleri Zone (Upper Carnian; Carlisle and Susuki, 1974). On Haida Gwaii (Queen Charlotte Islands), the volcanic sequence is overlain by the carbonate-dominated Kunga Group that yields conodonts and ammonoids of the Tuvalian 2-3 welleri Zone (Desrochers and Orchard, 1991). In south-western Yukon, the volcanism continued to at least the early Norian, evidenced by conodonts recovered from interbedded limestones in the Nikolai Formation (Israel et al., 2006). Ar/Ar and U-Pb ages for the Wrangellia magmatic rocks span between 232 and 226 Ma (Greene et al., 2010 and references therein). However, samples selected for Ar/Ar dating showed widespread alteration leading to large age uncertainties (up to 11 Ma), disturbed spectra, and excess argon. Zircons and baddeleyite selected for U-Pb dating were not chemically abraded, likely yielding ages younger than the actual crystallization ages.
The lack of high precision radioisotopic ages for the Wrangellia precludes the possibility to constrain the onset of magmatism, and to distinguish different pulses in the volcanic activity, in turn hindering reconstruction of rates of volcanic or thermogenic gas discharges. This is crucial as the Wrangellia has been tentatively linked to a period of significant climate changes and biological turnover during the Late Triassic, known as CPE (Dal Corso et al., 2020). The finding of four negative carbon isotope excursions (NCIEs) correlated with Hg spikes in sedimentary strata across the CPE suggest a volcanic origin for this event (Dal Corso et al., 2018, 2020; Lu et al., 2021; Mazaheri-Johari et al., 2021). The duration of the CPE was inferred to be of ca. 1.2 to 1.7 Ma based on magnetostratigraphic, biostratigraphic and cyclostratigraphic studies (Zhang et al., 2015; Miller et al., 2017; Bernardi et al., 2018; Dal Corso et al., 2020). Accordingly, two tuffaceous claystones intercalated with CPE-related rocks of the Jiyuan Basin (China) were dated at 233.10 ± 1.30 and 232.90 ± 2.10 Ma (LA-ICP-MS U-Pb zircon ages; Lu et al., 2021). However, although the ages of the Wrangellia and the CPE partially overlap, their relative timing remains highly debated.
Discussion and Concluding Remarks
Eruptions of the Siberian Traps, the CAMP, and the Wrangellia LIPs deeply reshaped the Triassic world. In particular, the Siberian Traps and the CAMP emplaced in continental settings as pulsed magmatic events, and both bear strong evidence of interaction between magmas and sedimentary country rocks. A clear difference between these LIPs is shown by the widespread explosive volcanism and abundant alkaline magmatism marking the early and final phases of the Siberian Traps, but unknown for the CAMP. On the other hand, the Wrangellia presents substantial differences as it was emplaced in an oceanic setting, and partially consists of subaqueous lava flows. Due to the lack of high-precision geochronology it is also impossible to clarify whether its magmatic activity was pulsed or continuous.
A recurrent aspect of the Triassic period is that multiple NCIEs were reported worldwide for the end-Permian (e.g., Wu et al., 2021) and end-Triassic mass extinction intervals (Ruhl et al., 2020), as well as for the CPE (Sun et al., 2019; Dal Corso et al., 2018, 2020). NCIEs would testify for the injection of large quantities of isotopically depleted CO2 into the atmosphere and oceans. Likely, CO2 (and other greenhouse and poisonous gases) would have been released either from the erupted magmas (Capriolo et al., 2020) or from devolatilization of the intruded host-rocks (Svensen et al., 2009; Capriolo et al., 2021), implying that LIPs-related magmatism was the main driver for catastrophic environmental changes of the Triassic. Since the magnitude of these global crises is also related to the rates at which volatiles were generated, quantifying the process of magma degassing is crucial. Direct estimates of released CO2 were obtained from fresh, non-recrystallized melt inclusions (e.g., Sobolev et al., 2009; Black et al., 2014; Sibik et al., 2015; Capriolo et al., 2020). However, these are few (and potentially not entirely representative) for the Siberian Traps and the CAMP, and completely lacking for the Wrangellia. We therefore adopted here the approach of Rosenthal et al. (2015) to estimate the initial CO2 contents of basaltic magmas from their incompatible trace element compositions. In fact, Nb and CO2 behave similarly during partial mantle melting, both strongly partitioning into the melt phase. However, at shallow depth in the crust, CO2 is degassed as magma ascends towards the surface (Capriolo et al., 2020), while Nb remains in the melt. We applied this proxy to whole-rock compositions of the three Triassic LIPs (Figure 2) filtered for basalts with MgO >7 wt% to consider only those magmas closer to the composition of mantle-derived primitive melts, as suggested by Hernandez Nava et al. (2021).
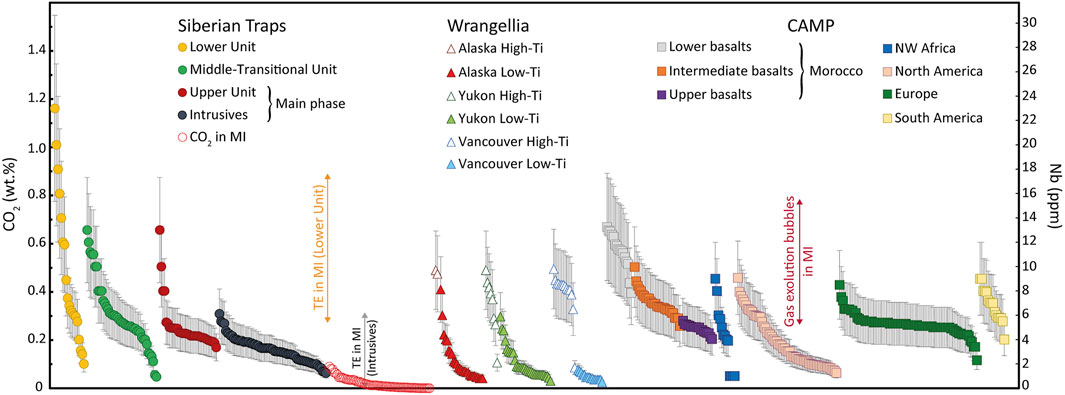
FIGURE 2. Initial maximum CO2 budgets obtained from Nb whole-rock concentrations of magmas for the Siberian Traps (circles), the Wrangellia (triangles) and the CAMP (squares). Following Rosenthal et al. (2015), we assumed CO2/Nb = 505 ± 168, which corresponds to mantle source CO2 of 75 ± 25 ppm. Data are filtered for MgO > 7wt%. Maximum and minimum MgO contents are 22.9–7.1 wt% (average 10.2 wt%) for the Siberian Traps, 17.5–7.1 wt% (average 9.8 wt%) for the Wrangellia, and 16.6–7.0 wt% (average 8.7 wt%) for the CAMP. Data are plotted in ranked order. Siberian Traps: data from GEOROC Database (http://georoc.mpch-mainz.gwdg.de/); Hawkesworth et al. (1995); Sobolev et al. (2009, 2011); Sibik et al. (2015); Callegaro et al. (2021). Wrangellia: data from Greene et al. (2009a), Greene et al. (2009b), Greene et al. (2010). CAMP: data from De Min et al. (2003); Jourdan et al. (2003); Deckart et al. (2005); Verati et al. (2005); Merle et al. (2011, 2014); Callegaro et al. (2013, 2014b); Bertrand et al. (2014); Marzoli et al. (2019). All data are reported in the Supplementary Appendix Table. CO2 concentrations measured from melt inclusions (MI) for the Siberian Traps (Black et al., 2014; Sibik et al., 2015) are reported for comparison (open circles). Yellow and grey arrows represent CO2 estimates obtained from trace element (TE) concentrations in melt inclusions following the same approach as whole-rock data. CO2 concentrations obtained from MI of the Gudchikhinsky Formation (yellow colour; Lower Unit) and of intrusives of the main phase of the Siberian Traps (grey colour; Sibik et al., 2015) are ca. 0.3–0.9 wt% and ca. 0–0.3 wt%, respectively, consistent with the results obtained from whole-rock data. Red arrow represents CO2 concentrations estimated from gas exsolution bubbles preserved in melt inclusion of CAMP magmas (Capriolo et al., 2020).
CO2 concentrations estimated for magmas of the Siberian Traps are significantly variable within the three magmatic phases previously described (Figure 2). High-Ti lava flows of the Lower Unit and the low-Ti magmas of the Middle-Transitional Unit yielded the highest calculated CO2 contents (ca. 0.1–1.2 and 0.05–0.7 wt%, respectively; Figure 2). In contrast, lavas of the Upper Unit and correlated intrusions, which characterize the second and more voluminous Siberian main phase, yielded lower CO2 contents (ca. 0.1–0.3 wt%; Figure 2). Within CAMP, the earliest erupted basalts from north-western Africa are relatively enriched in CO2 (0.4–0.7 wt%), while the slightly later and ubiquitous low-Ti magmas of the Prevalent group (Callegaro et al., 2013; Merle et al., 2014; Marzoli et al., 2018, 2019) yielded slightly lower CO2 concentrations (ca. 0.1 to 0.5 wt%; Figure 2). These results would imply that, for both the Siberian Traps and the CAMP, the highest concentrations of mantle-derived CO2 were produced from magmas of the initial phases. Interestingly, the end-Permian extinction coincides with the initial emplacement of the volatile-poor sills of the Siberian Traps, while the end-Triassic extinction coincides with the effusive activity of the CAMP, whose lavas are relatively CO2-rich (Capriolo et al., 2020). The fact that variable amounts of mantle-derived CO2 from the magmas produced similar environmental consequences suggests that additional isotopically depleted CO2 was likely released during the intrusive Siberian Traps activity. In this case, a significant part of the emitted CO2 (and possibly other gases, e.g., CH4, SO2 and halocarbons) may have been produced thermogenically from the intruded host rocks (e.g., Svensen et al., 2009, 2018; Capriolo et al., 2021) highlighting the crucial role of sedimentary basins as additional suppliers of climate-modifying gases. Noteworthy, the early terrestrial onset of the end-Permian extinction seems to suggest that mantle-derived halogens were key environmental impactors during the first phase of the Siberian Traps (Sobolev et al., 2011; Dal Corso et al., 2022).
Geochemical data for the Wrangellia are relatively scarce. However, the high-Ti basalts have the highest Nb and thus possibly the highest CO2 contents (ca. 0.3–0.5 wt%), while the low-Ti basalts were relatively Nb and thus CO2-depleted (≤0.3 wt%). This difference may imply that the overall production of CO2 increased when magmas were predominantly sourced from the mantle plume source, i.e., with minor or no contribution from the lithospheric mantle.
To summarize, in this study, we reviewed the main features of the Siberian Traps, the Wrangellia and the CAMP LIPs, which impacted the Triassic world. High-precision geochronology strongly links the Siberian Traps and the CAMP to the end-Permian and the end-Triassic mass extinctions, respectively. A similar cause-and-effect scenario between the Wrangellia and the CPE seems plausible based on paleontological, biostratigraphic and geochemical studies. However, further high-precision dating on both lava flows and intrusions is necessary to constrain the onset, evolution, and cessation of this LIP, as well as its emplacement mechanisms.
Using previously published data, we estimated maximum CO2 contents for magmas of the three Triassic LIPs. Even if these estimates are based on proxy data, our results highlight that the mantle-related CO2 budgets of the more primitive basalts seem to vary during the overall life cycle of each LIP, suggesting that different mantle sources or melting regimes came into play to produce these exceptionally voluminous volcanic events. These observations stress the fact that each LIP is a unique case-study and building paradigms that apply to all LIPs when discussing their relationship to concurrent Earth crises is not straightforward.
Author Contributions
AB wrote the review on Wrangellia, compiled data from the literature and produced the figures. SC wrote the review on the Siberian Traps. AM designed the project and wrote the review on the CAMP. YS contributed to the review on Wrangellia.
Funding
Financial support was provided by the Italian Ministry of University and Research (PRIN 20178LPCP to AM). SC acknowledges support from the Research Council of Norway (Grant 301096, Young Research Talents).
Conflict of Interest
The authors declare that the research was conducted in the absence of any commercial or financial relationships that could be construed as a potential conflict of interest.
Publisher’s Note
All claims expressed in this article are solely those of the authors and do not necessarily represent those of their affiliated organizations, or those of the publisher, the editors and the reviewers. Any product that may be evaluated in this article, or claim that may be made by its manufacturer, is not guaranteed or endorsed by the publisher.
Supplementary Material
The Supplementary Material for this article can be found online at: https://www.frontiersin.org/articles/10.3389/feart.2022.887632/full#supplementary-material
References
Augland, L. E., Ryabov, V. V., Vernikovsky, V. A., Planke, S., Polozov, A. G., and Callegaro, S. (2019). The Main Pulse of the Siberian Traps Expanded in Size and Composition. Sci. Rep. 9, 1–12. doi:10.1038/s41598-019-54023-2
Baresel, B., Bucher, H., Brosse, M., Cordey, F., Guodun, K., and Schaltegger, U. (2017). Precise Age for the Permian – Triassic Boundary in South China from High-Precision U-Pb Geochronology and Bayesian Age – Depth Modeling. Solid Earth 8, 361–378. doi:10.5194/se-8-361-2017
Bernardi, M., Gianolla, P., Petti, F. M., Mietto, P., and Benton, M. J. (2018). Dinosaur Diversification Linked with the Carnian Pluvial Episode. Nat. Commun. 9. doi:10.1038/s41467-018-03996-1
Bertrand, H., Fornari, M., Marzoli, A., García-Duarte, R., and Sempere, T. (2014). The Central Atlantic Magmatic Province Extends into Bolivia. Lithos 188, 33–43. doi:10.1016/j.lithos.2013.10.019
Black, B. A., Hauri, E. H., Elkins-Tanton, L. T., and Brown, S. M. (2014). Sulfur Isotopic Evidence for Sources of Volatiles in Siberian Traps Magmas. Earth Planet. Sci. Lett. 394, 58–69. doi:10.1016/j.epsl.2014.02.057
Blackburn, T. J., Olsen, P. E., Bowring, S. A., McLean, N. M., Kent, D. V., Puffer, J., et al. (2013). Zircon U-Pb Geochronology Links the End-Triassic Extinction with the Central Atlantic Magmatic Province. Science 340, 941–945. doi:10.1126/science.1234204
Boscaini, A., Marzoli, A., Bertrand, H., Chiaradia, M., Jourdan, F., Faccenda, M., et al. (2022). Cratonic Keels Controlled the Emplacement of the Central Atlantic Magmatic Province (CAMP). Earth Planet. Sci. Lett. 584. doi:10.1016/j.epsl.2022.117480
Brack, P., Rieber, H., Nicora, A., and Mundil, R. (2005). The Global Boundary Stratotype Section and Point (GSSP) of the Ladinian Stage (Middle Triassic) at Bagolino (Southern Alps, Northern Italy) and its Implications for the Triassic Time Scale. Episodes 28, 233–244. doi:10.18814/epiiugs/2005/v28i4/001
Burgess, S. D., and Bowring, S. A. (2015). High-precision Geochronology Confirms Voluminous Magmatism before, during, and after Earth’s Most Severe Extinction. Sci. Adv. 1, 1–14. doi:10.1126/sciadv.1500470
Burgess, S. D., Bowring, S. A., and Shen, S. (2014). High-precision Timeline for Earth’s Most Severe Extinction. Proc. Natl. Acad. Sci. U. S. A. 111, 3316–3321. doi:10.1073/pnas.140419611110.1073/pnas.1317692111
Burgess, S. D., Muirhead, J. D., and Bowring, S. A. (2017). Initial Pulse of Siberian Traps Sills as the Trigger of the End-Permian Mass Extinction. Nat. Commun. 8, 1–4. doi:10.1038/s41467-017-00083-9
Callegaro, S., Baker, D. R., de Min, A., Marzoli, A., Geraki, K., Bertrand, H., et al. (2014a). Microanalyses Link Sulfur from Large Igneous Provinces and Mesozoic Mass Extinctions. Geology 42, 895–898. doi:10.1130/G35983.1
Callegaro, S., Marzoli, A., Bertrand, H., Chiaradia, M., Reisberg, L., Meyzen, C., et al. (2013). Upper and Lower Crust Recycling in the Source of CAMP Basaltic Dykes from southeastern North America. Earth Planet. Sci. Lett. 376, 186–199. doi:10.1016/j.epsl.2013.06.023
Callegaro, S., Rapaille, C., Marzoli, A., Bertrand, H., Chiaradia, M., Reisberg, L., et al. (2014b). Enriched Mantle Source for the Central Atlantic Magmatic Province: New Supporting Evidence from Southwestern Europe. Lithos 188, 15–32. doi:10.1016/j.lithos.2013.10.021
Callegaro, S., Svensen, H. H., Neumann, E. R., Polozov, A. G., Jerram, D. A., Deegan, F. M., et al. (2021). Geochemistry of Deep Tunguska Basin Sills, Siberian Traps: Correlations and Potential Implications for the End-Permian Environmental Crisis. Contrib. Mineral. Petrol. 176, 1–30. doi:10.1007/s00410-021-01807-3
Capriolo, M., Marzoli, A., Aradi, L. E., Ackerson, M. R., Bartoli, O., Callegaro, S., et al. (2021). Massive Methane Fluxing from Magma–Sediment Interaction in the End-Triassic Central Atlantic Magmatic Province. Nat. Commun. 12, 1–9. doi:10.1038/s41467-021-25510-w
Capriolo, M., Marzoli, A., Aradi, L. E., Callegaro, S., Dal Corso, J., Newton, R. J., et al. (2020). Deep CO2 in the End-Triassic Central Atlantic Magmatic Province. Nat. Commun. 11, 1–11. doi:10.1038/s41467-020-15325-6
Capriolo, M., Mills, B. J. W., Newton, R. J., Dal Corso, J., Dunhill, A. M., Wignall, P. B., et al. (2022). Anthropogenic-scale CO2 Degassing from the Central Atlantic Magmatic Province as a Driver of the End-Triassic Mass Extinction. Glob. Planet. Change 209. doi:10.1016/j.gloplacha.2021.103731
Carlisle, D., and Suzuki, T. (1974). Emergent basalt and Submergent Carbonate-Clastic Sequences Including the Upper Triassic Dilleri and Welleri Zones on Vancouver Island. Can. J. Earth Sci. 11, 254–279. doi:10.1139/e74-023
Dal Corso, J., Bernardi, M., Sun, Y., Song, H., Seyfullah, L. J., Preto, N., et al. (2020). Extinction and Dawn of the Modern World in the Carnian (Late Triassic). Sci. Adv. 6, 1–13. doi:10.1126/sciadv.aba0099
Dal Corso, J., Callegaro, S., Sun, Y., Hilton, J., Grasby, S. E., Joachimski, M. M., et al. (2022). Environmental Crises at the Permian–Triassic Mass Extinction. Nat. Rev. Earth Environ.. doi:10.1038/s43017-021-00259-4
Dal Corso, J., Gianolla, P., Rigo, M., Franceschi, M., Roghi, G., Mietto, P., et al. (2018). Multiple Negative Carbon-Isotope Excursions during the Carnian Pluvial Episode (Late Triassic). Earth-science Rev. 185, 732–750. doi:10.1016/j.earscirev.2018.07.004
Davies, J. H. F. L., Marzoli, A., Bertrand, H., Youbi, N., Ernesto, M., Greber, N. D., et al. (2021). Zircon Petrochronology in Large Igneous Provinces Reveals Upper Crustal Contamination Processes: New U–Pb Ages, Hf and O Isotopes, and Trace Elements from the Central Atlantic Magmatic Province (CAMP). Contrib. Mineral. Petrol. 176, 1–24. doi:10.1007/s00410-020-01765-2
Davies, J. H. F. L., Marzoli, A., Bertrand, H., Youbi, N., Ernesto, M., and Schaltegger, U. (2017). End-Triassic Mass Extinction Started by Intrusive CAMP Activity. Nat. Commun. 8, 1–8. doi:10.1038/ncomms15596
De Min, A., Piccirillo, E. M., Marzoli, A., Bellieni, G., Renne, P. R., Ernesto, M., et al. (2003). The Central Atlantic Magmatic Province (CAMP) in Brazil: Petrology, Geochemistry, 40Ar/39Ar Ages, Paleomagnetism and Geodynamic Implications. Geophys. Monogr. Ser. 136, 91–128. doi:10.1029/136GM06
Deckart, K., Bertrand, H., and Liégeois, J. P. (2005). Geochemistry and Sr, Nd, Pb Isotopic Composition of the Central Atlantic Magmatic Province (CAMP) in Guyana and Guinea. Lithos 82, 289–314. doi:10.1016/j.lithos.2004.09.023
Desrochers, A., and Orchard, M. J. (1991). “The Kunga Group (Late Triassic-Early Jurassic), Queen Charlotte Islands, British Columbia: Stratigraphic Revisions and Carbonate Sedimentology,” in Evolution and Hydrocarbon Potential of the Queen Charlotte Basin, British Columbia. Editor G. W. Woodsworth (Ottawa: Can. Geol. Surv.), 163–172.
El Hachimi, H., Youbi, N., Madeira, J., Bensalah, M. K., Martins, L., Mata, J., et al. (2011). Morphology, Internal Architecture and Emplacement Mechanisms of Lava Flows from the Central Atlantic Magmatic Province (CAMP) of Argana Basin (Morocco). Geol. Soc. Spec. Publ. 357, 167–193. doi:10.1144/SP357.9
Elkins-Tanton, L. T., Draper, D. S., Agee, C. B., Jewell, J., Thorpe, A., and Hess, P. C. (2007). The Last Lavas Erupted during the Main Phase of the Siberian Flood Volcanic Province: Results from Experimental Petrology. Contrib. Mineral. Petrol. 153, 191–209. doi:10.1007/s00410-006-0140-1
Fürsich, F. T., and Wendt, J. (1977). Biostratinomy and Palaeoecology of the Cassian Formation (Triassic) in the Southern Alps. Palaeogeogr. Palaeoclimatol. Palaeoecol. 22, 257–323.
Greene, A. R., Scoates, J. S., Weis, D., and Israel, S. (2009a). Geochemistry of Triassic Flood Basalts from the Yukon (Canada) Segment of the Accreted Wrangellia Oceanic Plateau. Lithos 110, 1–19. doi:10.1016/j.lithos.2008.11.010
Greene, A. R., Scoates, J. S., Weis, D., Katvala, E. C., Israel, S., and Nixon, G. T. (2010). The Architecture of Oceanic Plateaus Revealed by the Volcanic Stratigraphy of the Accreted Wrangellia Oceanic Plateau. Geosphere 6, 47–73. doi:10.1130/GES00212.1
Greene, A. R., Scoates, J. S., Weis, D., Nixon, G. T., and Kieffer, B. (2009b). Melting History and Magmatic Evolution of Basalts and Picrites from the Accreted Wrangellia Oceanic Plateau, Vancouver Island, Canada. J. Petrol. 50, 467–505. doi:10.1093/petrology/egp008
Hawkesworth, C. J., Lightfoot, P. C., Fedorenko, V. A., Blake, S., Naldrett, A. J., Doherty, W., et al. (1995). Magma Differentiation and Mineralisation in the Siberian continental Flood Basalts. Lithos 34, 61–88. doi:10.1016/0024-4937(95)90011-x
Heimdal, T. H., Callegaro, S., Svensen, H. H., Jones, M. T., Pereira, E., and Planke, S. (2019). Evidence for Magma–Evaporite Interactions during the Emplacement of the Central Atlantic Magmatic Province (CAMP) in Brazil. Earth Planet. Sci. Lett. 506, 476–492. doi:10.1016/j.epsl.2018.11.018
Heimdal, T. H., Svensen, H. H., Ramezani, J., Iyer, K., Pereira, E., Rodrigues, R., et al. (2018). Large-scale Sill Emplacement in Brazil as a Trigger for the End-Triassic Crisis. Sci. Rep. 8, 1–12. doi:10.1038/s41598-017-18629-8
Hernandez Nava, A., Black, B. A., Gibson, S. A., Bodnar, R. J., Renne, P. R., and Vanderkluysen, L. (2021). Reconciling Early Deccan Traps CO2 Outgassing and Pre-KPB Global Climate. Proc. Natl. Acad. Sci. U. S. A. 118, 1–9. doi:10.1073/pnas.2007797118
Israel, S., Tizzard, A., and Major, J. (2006). “Bedrock Geology of the Duke River Area, Parts of NTS 115G/2, 3, 4, 6 and 7, Southwestern Yukon,” in Yukon Exploration and Geology 2005. Editors D. S. Emond, G. D. Bradshaw, L. L. Lewis, and L. H. Weston (Whitehorse: Yukon Geological Survey), 139–154.
Jourdan, F., Marzoli, A., Bertrand, H., Cosca, M., and Fontignie, D. (2003). The Northernmost CAMP: 40Ar/39Ar Age, Petrology and Sr-Nd-Pb Isotope Geochemistry of the Kerforne Dike, Brittany, France. Geophys. Monogr. Ser. 136, 209–226. doi:10.1029/136GM011
Kamo, S. L., Czamanske, G. K., Amelin, Y., Fedorenko, V. A., Davis, D. W., and Trofimov, V. R. (2003). Rapid Eruption of Siberian Flood-Volcanic Rocks and Evidence for Coincidence with the Permian-Triassic Boundary and Mass Extinction at 251 Ma. Earth Planet. Sci. Lett. 214, 75–91. doi:10.1016/S0012-821X(03)00347-9
Kerr, A. C. (2005). Oceanic LIPs: The Kiss of Death. Elements 1, 289–292. doi:10.2113/gselements.1.5.289
Knight, K. B., Nomade, S., Renne, P. R., Marzoli, A., Bertrand, H., and Youbi, N. (2004). The Central Atlantic Magmatic Province at the Triassic-Jurassic Boundary: Paleomagnetic and 40Ar/39Ar Evidence from Morocco for Brief, Episodic Volcanism. Earth Planet. Sci. Lett. 228, 143–160. doi:10.1016/j.epsl.2004.09.022
Landwehrs, J. P., Feulner, G., Hofmann, M., and Petri, S. (2020). Climatic Fluctuations Modelled for Carbon and Sulfur Emissions from End-Triassic Volcanism. Earth Planet. Sci. Lett. 537, 116174. doi:10.1016/j.epsl.2020.116174
Lassiter, J. C., DePaolo, D. J., and Mahoney, J. J. (1995). Geochemistry of the Wrangellia Flood basalt Province: Implications for the Role of continental and Oceanic Lithosphere in Flood basalt Genesis. J. Petrol. 36, 983–1009. doi:10.1093/petrology/36.4.983
Latyshev, A. V., Rad’ko, V. A., Veselovskiy, R. V., Fetisova, A. M., and Pavlov, V. E. (2020). Correlation of the Permian-Triassic Ore-Bearing Intrusions of the Norilsk Region with the Volcanic Sequence of the Siberian Traps Based on Paleomagnetic Data. Econ. Geol. 115, 1173–1193. doi:10.5382/ECONGEO.4746
Lindström, S., Callegaro, S., Davies, J., Tegner, C., van de Schootbrugge, B., Pedersen, G. K., et al. (2021). Tracing Volcanic Emissions from the Central Atlantic Magmatic Province in the Sedimentary Record. Earth-science Rev. 212. doi:10.1016/j.earscirev.2020.103444
Lu, J., Zhang, P., Corso, J. D., Yang, M., Wignall, P. B., Greene, S. E., et al. (2021). Volcanically Driven Lacustrine Ecosystem Changes during the Carnian Pluvial Episode (Late Triassic). Proc. Natl. Acad. Sci. U. S. A. 118, 1–8. doi:10.1073/pnas.2109895118
Marzoli, A., Bertrand, H., Youbi, N., Callegaro, S., Merle, R., Reisberg, L., et al. (2019). The Central Atlantic Magmatic Province (CAMP) in Morocco. J. Petrol. 60, 945–996. doi:10.1093/petrology/egz021
Marzoli, A., Callegaro, S., Dal Corso, J., Davies, J. H. F. L., Chiaradia, M., Youbi, N., et al. (2018). “The Central Atlantic Magmatic Province (CAMP): A Review,” in The Late Triassic World. Editor L. H. Tanner (Springer International Publishing), 91–125. doi:10.1007/978-3-319-68009-5_4
Mazaheri-Johari, M., Gianolla, P., Mather, T. A., Frieling, J., Chu, D., and Dal Corso, J. (2021). Mercury Deposition in Western Tethys during the Carnian Pluvial Episode (Late Triassic). Sci. Rep. 11, 1–10. doi:10.1038/s41598-021-96890-8
Merle, R., Marzoli, A., Bertrand, H., Reisberg, L., Verati, C., Zimmermann, C., et al. (2011). 40Ar/39Ar Ages and Sr–Nd–Pb–Os Geochemistry of CAMP Tholeiites from Western Maranhão basin (NE Brazil). Lithos 122, 137–151. doi:10.1016/j.lithos.2010.12.010
Merle, R., Marzoli, A., Reisberg, L., Bertrand, H., Nemchin, A., Chiaradia, M., et al. (2014). Sr, Nd, Pb and Os Isotope Systematics of CAMP Tholeiites from Eastern North America (ENA): Evidence of a Subduction-Enriched Mantle Source. J. Petrol. 55, 133–180. doi:10.1093/petrology/egt063
Miller, C. S., Peterse, F., Silva, A.-C., Baranyi, V., Reichart, G. J., and Kürschner, W. M. (2017). Astronomical Age Constraints and Extinction Mechanisms of the Late Triassic Carnian Crisis. Sci. Rep. 7, 1–7. doi:10.1038/s41598-017-02817-7
Panfili, G., Cirilli, S., Dal Corso, J., Bertrand, H., Medina, F., Youbi, N., et al. (2019). New Biostratigraphic Constraints Show Rapid Emplacement of the Central Atlantic Magmatic Province (CAMP) during the End-Triassic Mass Extinction Interval. Glob. Planet. Change 172, 60–68. doi:10.1016/j.gloplacha.2018.09.009
Patrick, M. R., Houghton, B. F., Anderson, K. R., Poland, M. P., Montgomery-Brown, E., Johanson, I., et al. (2020). The Cascading Origin of the 2018 Kīlauea Eruption and Implications for Future Forecasting. Nat. Commun. 11, 1–13. doi:10.1038/s41467-020-19190-1
Richards, M. A., Jones, D. L., Duncan, R. A., and DePaolo, D. J. (1991). A Mantle Plume Initiation Model for the Wrangellia Flood basalt and Other Oceanic Plateaus. Science 254, 263–267. doi:10.1126/science.254.5029.263
Rosenthal, A., Hauri, E. H., and Hirschmann, M. M. (2015). Experimental Determination of C, F, and H Partitioning between Mantle Minerals and Carbonated basalt, CO2/Ba and CO2/Nb Systematics of Partial Melting, and the CO2 Contents of Basaltic Source Regions. Earth Planet. Sci. Lett. 412, 77–87. doi:10.1016/j.epsl.2014.11.044
Ruhl, M., Hesselbo, S. P., Al-Suwaidi, A., Jenkyns, H. C., Damborenea, S. E., Manceñido, M. O., et al. (2020). On the Onset of Central Atlantic Magmatic Province (CAMP) Volcanism and Environmental and Carbon-Cycle Change at the Triassic–Jurassic Transition (Neuquén Basin, Argentina). Earth-science Rev. 208, 103229. doi:10.1016/j.earscirev.2020.103229
Ruiz-Martínez, V. C., Torsvik, T. H., van Hinsbergen, D. J. J., and Gaina, C. (2012). Earth at 200 Ma: Global Palaeogeography Refined from CAMP Palaeomagnetic Data. Earth Planet. Sci. Lett. 331–332, 67–79. doi:10.1016/j.epsl.2012.03.008
Saunders, A., and Reichow, M. (2009). The Siberian Traps and the End-Permian Mass Extinction: A Critical Review. Chin. Sci. Bull. 54, 20–37. doi:10.1007/s11434-008-0543-7
Schoene, B., Guex, J., Bartolini, A., Schaltegger, U., and Blackburn, T. J. (2010). Correlating the End-Triassic Mass Extinction and Flood basalt Volcanism at the 100 Ka Level. Geology 38, 387–390. doi:10.1130/G30683.1
Shellnutt, J. G., Dostal, J., and Lee, T. Y. (2021). Linking the Wrangellia Flood Basalts to the Galápagos Hotspot. Sci. Rep. 11. doi:10.1038/s41598-021-88098-7
Shellnutt, J. G., Dostal, J., and Yeh, M.-W. (2018). Mantle Source Heterogeneity of the Early Jurassic basalt of Eastern North America. Int. J. Earth Sci. 107, 1033–1058. doi:10.1007/s00531-017-1519-0
Sibik, S., Edmonds, M., Maclennan, J., and Svensen, H. (2015). Magmas Erupted during the Main Pulse of Siberian Traps Volcanism Were Volatile-Poor. J. Petrol. 56, 2089–2116. doi:10.1093/petrology/egv064
Sibik, S., Edmonds, M., Villemant, B., Svensen, H. H., Polozov, A. G., and Planke, S. (2021). Halogen Enrichment of Siberian Traps Magmas during Interaction with Evaporites. Front. Earth Sci. 9, 1–14. doi:10.3389/feart.2021.741447
Smith, J. G., and MacKevett, E. M. (1970). The Skolai Group in the McCarthy B-4, C-4, C-5 Quadrangles, Wrangell Mountains, Alaska. Bull. U.S. Geol. Surv. 1274-Q, Q1–Q26.
Sobolev, A. V., Krivolutskaya, N. A., and Kuzmin, D. V. (2009). Petrology of the Parental Melts and Mantle Sources of Siberian Trap Magmatism. Petrology 17, 253–286. doi:10.1134/S0869591109030047
Sobolev, S. V., Sobolev, A. V., Kuzmin, D. V., Krivolutskaya, N. A., Petrunin, A. G., Arndt, N. T., et al. (2011). Linking Mantle Plumes, Large Igneous Provinces and Environmental Catastrophes. Nature 477, 312–316. doi:10.1038/nature10385
Song, H., Wignall, P. B., Tong, J., and Yin, H. (2013). Two Pulses of Extinction during the Permian-Triassic Crisis. Nat. Geosci. 6, 52–56. doi:10.1038/ngeo1649
Sun, Y. D., Richoz, S., Krystyn, L., Zhang, Z. T., and Joachimski, M. M. (2019). Perturbations in the Carbon Cycle during the Carnian Humid Episode: Carbonate Carbon Isotope Records from Southwestern China and Northern Oman. J. Geol. Soc. Lond. 176, 167–177. doi:10.1144/jgs2017-170
Svensen, H. H., Frolov, S., Akhmanov, G. G., Polozov, A. G., Jerram, D. A., Shiganova, O. V., et al. (2018). Sills and Gas Generation in the Siberian Traps. Philos. Trans. R. Soc. A. 376. doi:10.1098/rsta.2017.0080
Svensen, H., Planke, S., Polozov, A. G., Schmidbauer, N., Corfu, F., Podladchikov, Y. Y., et al. (2009). Siberian Gas Venting and the End-Permian Environmental Crisis. Earth Planet. Sci. Lett. 277, 490–500. doi:10.1016/j.epsl.2008.11.015
Tanner, L. H., Lucas, S. G., and Chapman, M. G. (2004). Assessing the Record and Causes of Late Triassic Extinctions. Earth-science Rev. 65, 103–139. doi:10.1016/S0012-8252(03)00082-5
Tegner, C., Michelis, S. A. T., McDonald, I., Brown, E. L., Youbi, N., Callegaro, S., et al. (2019). Mantle Dynamics of the Central Atlantic Magmatic Province (CAMP): Constraints from Platinum Group, Gold and Lithophile Elements in Flood Basalts of Morocco. J. Petrol. 60, 1621–1652. doi:10.1093/petrology/egz041
Verati, C., Bertrand, H., and Féraud, G. (2005). The Farthest Record of the Central Atlantic Magmatic Province into West Africa Craton: Precise 40Ar/39Ar Dating and Geochemistry of Taoudenni basin Intrusives (Northern Mali). Earth Planet. Sci. Lett. 235, 391–407. doi:10.1016/j.epsl.2005.04.012
Wignall, P. B., and Atkinson, J. W. (2020). A Two-phase End-Triassic Mass Extinction. Earth-science Rev. 208, 103282. doi:10.1016/j.earscirev.2020.103282
Wotzlaw, J.-F., Guex, J., Bartolini, A., Gallet, Y., Krystyn, L., Mcroberts, C. A., et al. (2014). Towards Accurate Numerical Calibration of the Late Triassic: High-Precision U-Pb Geochronology Constraints on the Duration of the Rhaetian. Geology 42, 571–574. doi:10.1130/G35612.1
Wu, Y., Chu, D., Tong, J., Song, H., Dal Corso, J., Wignall, P. B., et al. (2021). Six-fold Increase of Atmospheric pCO2 during the Permian–Triassic Mass Extinction. Nat. Commun. 12. doi:10.1038/s41467-021-22298-7
Zhang, Y., Li, M., Ogg, J. G., Montgomery, P., Huang, C., Chen, Z., et al. (2015). Cycle-calibrated Magnetostratigraphy of Middle Carnian from South China: Implications for Late Triassic Time Scale and Termination of the Yangtze Platform. Palaeogeogr. Palaeoclimatol. Palaeoecol. 436, 135–166. doi:10.1016/j.palaeo.2015.05.033
Keywords: triassic, siberian traps, central atlantic magmatic province (CAMP), carnian crisis, wrangellia LIP, large igneous province, mass extinction
Citation: Boscaini A, Callegaro S, Sun Y and Marzoli A (2022) Late Permian to Late Triassic Large Igneous Provinces: Timing, Eruptive Style and Paleoenvironmental Perturbations. Front. Earth Sci. 10:887632. doi: 10.3389/feart.2022.887632
Received: 01 March 2022; Accepted: 23 March 2022;
Published: 12 April 2022.
Edited by:
Hossein Azizi, University of Kurdistan, IranReviewed by:
Federico Lucci, University of Bari Aldo Moro, ItalyOrhan Karsli, Karadeniz Technical University, Turkey
Copyright © 2022 Boscaini, Callegaro, Sun and Marzoli. This is an open-access article distributed under the terms of the Creative Commons Attribution License (CC BY). The use, distribution or reproduction in other forums is permitted, provided the original author(s) and the copyright owner(s) are credited and that the original publication in this journal is cited, in accordance with accepted academic practice. No use, distribution or reproduction is permitted which does not comply with these terms.
*Correspondence: Andrea Marzoli, YW5kcmVhLm1hcnpvbGlAdW5pcGQuaXQ=