- 1School of Earth Sciences and Engineering, Nanjing University, Nanjing, China
- 2Guangzhou Marine Geological Survey Guangzhou, China
Geochemical profiles in pore water of marine sediments have been considered as, important indicators of gas hydrate occurrence. In the gas hydrate area around the world, the decrease of sulfate, calcium, and magnesium concentrations with depth mainly results directly or indirectly from the anaerobic oxidation of methane (AOM). The ubiquitous abnormally high concentration gradients of iodide in the research area reflect the large methane-generating potential of the area. Thus, we explore the feasibility of using gradients of sulfate, iodide, and authigenic carbonate precipitation as indicators for gas hydrate in the regional exploration of gas hydrate formation. We test the criterion in the gas hydrate zone in the South China Sea (SCS) for the recognition of gas hydrate by using the gradients of sulfate, calcium plus magnesium, and iodide. Contour maps of pore-water gradients from expeditions in the study area are used to correlate the key gradients to underlying gas hydrate occurrence. The results show that the largest potential gas hydrate indicated by the contour maps of the indicators are well consistent with the discovery of GMGS1 and GMGS3 expedition. It implies the possible applicability of this geochemical method in gas hydrate exploration. Also, we identify a promising area in the South China Sea for future gas hydrate investigations. It is the first collective application of the gradients of sulfate, calcium plus magnesium, and iodide to a gas hydrate terrane, especially to a large area of the SCS. We believe that the result of this research will benefit the future exploration of gas hydrate and will arouse a lot of interest from other researchers.
Highlights
1. We link sulfate, calcium, magnesium, and iodide pore-water gradients to the occurrence of underlying gas hydrates.
2. We support this hypothesis using data from recognized gas hydrate occurrences globally.
3. We identify a promising area in the South China Sea for future gas hydrate investigations.
1 Introduction
The gas hydrate is an ice-like substance consisting of water and gas (most is CH4) that forms under conditions of low temperature, high pressure, and adequate gas concentration (Sloan, 1990). An accepted estimation of gas hydrate resources is about 3,000 trillion cubic meters (TCM), which is enormous when compared with the conventional gas resources (∼404 TCM) and shale gas (204-456 TCM) (Chong et al., 2016). Due to its considerable reserves and clean combustion products, gas hydrate may become a promising energy resource (Collett, 2002; Hesse, 2003; Makogon et al., 2007; Boswell and Collett, 2011; Chong et al., 2016). The methane contained within gas hydrates is a kind of potential greenhouse gas, 20 times more effective as a greenhouse gas than carbon dioxide (CO2) (Mitchell, 1989; Ruppel, 2011). Therefore, any release of methane from gas hydrate decomposing due to temperature or pressure changes can cause a positive feedback cycle for atmospheric warming, thus impacting climate change (Borowski et al., 1996; Haq, 2000; Wallmann et al., 2014; Brown et al., 2016; Reay et al., 2018). Accordingly, the abundance, nature, and distribution of gas hydrate are of great importance and have been arousing a lot of research interests of many researchers.
Geophysical methods are widely applied to the study of gas hydrate distribution and abundance. Bottom-simulating reflectors (BSRs) are often coincident with gas hydrate occurrences at depth because dissociating gas hydrate produces in situ gaseous methane that causes an acoustic impedance contrast that generates its strong seismic reflector (Miller et al., 1991; Hornbach et al., 2012). However, a BSR is not necessarily associated with, or completely consistent with, the presence of gas hydrate (Kvenvolden et al., 1993; Le et al., 2015; Dumke et al., 2016). Thus, we try to improve the setup of regional gas hydrate exploration by employing some geochemical indicators. Geochemical methods have already been tried to confirm potential gas hydrate-bearing areas through the geochemical studies of pore water in sediment (Borowski et al., 1996; Dickens, 2001; Bhatnagar et al., 2008; Wu et al., 2013; Pogodaeva et al., 2020). One of the most commonly used geochemical tools for hydrate recognition in drill cores is the presence of freshening chloride anomalies coupled with δ18O increase in pore water (Hesse, 2003; Luo et al., 2014). Nevertheless, the cores need to penetrate through the hydrate occurrence zone (often hundreds of meters below the seafloor) for the recognition of these anomalies, which is an economic disadvantage for large-scale exploration projects. Alternatively, the profiles of other pore water species (e.g., SO42-, I-) that record early diagenetic reactions in shallow sediments can serve as a powerful tool in gas hydrate exploration (Schulz, 2006). Some reactions associated with methane could be employed to indicate the existence of gas hydrate because a large amount of methane may occur in the gas hydrate occurrence zone. Within the sulfate reduction zone, normally, sulfate is consumed by organoclastic sulfate reduction (OSR) (Berner, 1980; Gieskes et al., 1981):
However, in the sulfate-methane transition zone (SMTZ, or SMI for sulfate–methane interface) where methane upwells from deep sediments, sulfate supplied from seawater will be exhausted by the anaerobic oxidation of methane (AOM) reaction (Barnes and Goldberg, 1976),
Substantial depletion of sulfate through AOM causes the SMTZ to become shallower (Blair and Aller, 1995; Borowski et al., 1996; Dickens, 2001). Some researchers accordingly proposed that the linearity of sulfate gradients and the shallow depth of SMTZ could be used to indicate the existence of gas hydrate-bearing areas (Borowski et al., 1999; Kim et al., 2020). What's more, the calcium concentration of pore water is also sensitive to the AOM process (Rodriguez et al., 2000). It declines with depth due to the enhanced authigenic carbonate precipitation. At the SMTZ where intense AOM reactions occur, the sharp increase in pore water alkalinity may cause the rapid decrease in calcium concentration (Raiswell, 1988; Boetius et al., 2000; Rodriguez et al., 2000). Besides, concentrated iodine anomaly is also commonly observed in gas hydrate-bearing areas (Fehn et al., 2003; Fehn et al., 2006; Muramatsu et al., 2007). The abnormally high concentration of iodide in pore water is related to the decomposition of massive sedimentary organic matter, which represents a large potential gas source. As a result, the concentrated iodide concentration of pore water could also reflect the possible occurrence of gas hydrate. Based on previous research we mentioned above, we try to explore the feasibility of using gradients of sulfate, iodide, and authigenic carbonate precipitation as indicators for gas hydrate in the regional exploration of gas hydrate formation.
Many gas hydrate-bearing areas around the world have been identified and investigated including the Blake Ridge (Paull et al., 1996; Paull and Matsumoto, 2000), Hydrate Ridge (Milkov et al., 2003; Torres et al., 2004), Gulf of Mexico (Boswell et al., 2009), Nankai Trough (Kastner et al., 1993) and Ulleung Basin (Park et al., 2008). In China, several geophysical and geochemical explorations of gas hydrate have been carried out on the northern slope of the South China Sea (SCS) since the 1990s (Zhang et al., 2002; Wu et al., 2004; Jiang et al., 2008; Wu et al., 2011; Zhang et al., 2012; Zhang et al., 2015; Jin et al., 2020; Wang et al., 2021). Numerous data from piston cores and drill sites have been accumulated, which allows us to make a regional comparison of hydrate distribution. The existence of gas hydrate in the northern SCS has been confirmed by geological, geophysical, and geochemical evidence including the occurrence of well-developed BSRs, methane-derived carbonate and shallow SMTZ depth (Liu et al., 2006; Song et al., 2007; Wang et al., 2011; Wu et al., 2011; Li et al., 2012; Li et al., 2015; Zhang et al., 2015; Chen et al., 2016; Jin et al., 2020). During the Sino-German Cooperative Project in early 2004, methane seepages in the SCS were verified for the first time by the discovery of Jiulong Methane Reef (Han et al., 2008). Three years later, natural gas hydrate samples were first recovered in a gas hydrate drilling expedition (GMGS1) which was initiated by Guangzhou Marine Geological Survey in the Shenhu area, northern SCS (Zhang et al., 2007; Wu et al., 2011). In 2013, GMGS2 was conducted in the east of Pearl River Mouth Basin of SCS, and massive gas hydrates were successfully recovered (Zhang et al., 2015). Recently, several visible gas hydrates were recovered in the Shenhu area again during the expedition GMGS3, which further constrain the gas hydrate reserves of the SCS (Yang et al., 2015). In 2016, GMGS4 was conducted in Shenhu area, and the new gas hydrates were found (Yang et al., 2017). In 2018, GMGS5 was conducted in QiongDongNan area of SCS, and massive gas hydrates were sampled (Ye et al., 2019).
Based on previous gas hydrate research, we correlate comprehensive geochemical indicators, the gradients of sulfate, calcium plus magnesium, and iodide, to the occurrence of underlying gas hydrate in marine sediments. The approach is based on the influence of the occurrence of gas hydrate on the geochemical characteristics of shallow pore water and was tested by correlating the collective gradients to known areas of gas hydrate. This result is compared with the hydrate detection results of GMGS1 and GMGS3. Based on this geochemical criterion, we also predict another promising area for future gas hydrate exploration in the northern SCS through the geographic information system. We believe that this research will benefit the future exploration of gas hydrate and will arouse a lot of interest from other researchers.
2 Geological setting and methods
The South China Sea, tectonically controlled by interactions of Eurasian Plate, Pacific Plate, and Indo-Australian Plate, is one of the largest marginal basins in the western Pacific Ocean (Li, et al., 2012; Wang et al., 2013). A series of sedimentary basins (e.g., the Taixinan Basin, the Pearl River Mouth Basin, and the Qiongdongnan Basin) in the northern SCS are filled with Mesozoic and Cenozoic sediments, whose largest thickness is over 10 km. The thick sediments provide ideal methane sources for gas hydrate formation (Lüdmann and Wong, 1999). Moreover, the northern slope of the SCS has a water depth of 200∼3400 m and a bottom water temperature of 2∼5 °C, these temperature and pressure conditions are suitable for gas hydrate formation (Yao, 2001).
The study region, the Shenhu area, is situated in the middle of the northern slope of SCS (between the Xisha Trough and the Dongsha Islands) (Figure 1). In this area, quantities of deepwater sedimentary fan have developed since Neogene, with average sedimentation rates of 110, 38, 27 m Ma-1 for Pleistocene, Pliocene, Miocene respectively (Wu et al., 2011). A series of high-angle faults and vertical fracture systems cut the sedimentary section since Pliocene. A series of central diapiric areas such as gas chimneys are well developed in the Shenhu area, providing favorable geological environments and structures for gas hydrate formation (Lüdmann et al., 2001; Wu et al., 2004). High-resolution seismic investigations show that most of BSRs are 150-350 m below the seafloor (Wu et al., 2013). In order to detect the occurrences and determine the distribution of gas hydrates, some surveys have been carried out by Guangzhou Marine Geological Survey since the 1990s. Hundreds of piston cores were recovered by research vessels of the Guangzhou Marine Geological Survey in recent years.
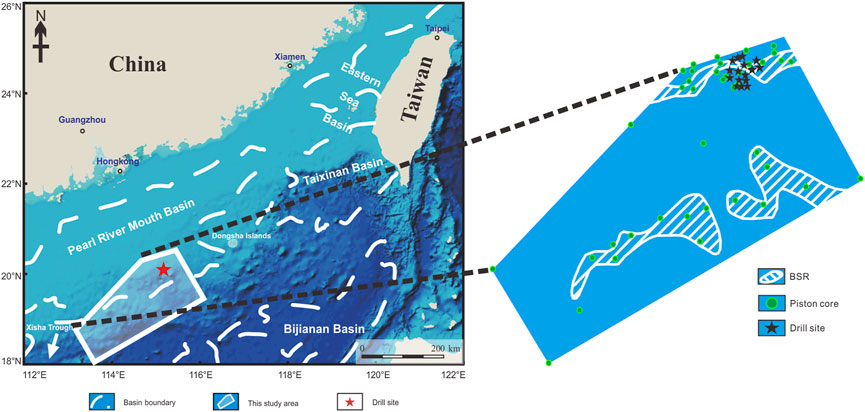
FIGURE 1. Major basins of the South China Sea and the location of the study area (Modified from Ye et al., 2016). The right part exhibits the detailed situation of the research region, including the information of piston cores we used in the article.
All the geochemical data were measured at the state key laboratory for mineral deposits research, Nanjing University. The anions and cations of pore water except iodide were measured by an ion chromatography (Metrohm 790-1, Metrosep A Supp 4-250/Metrosep C 2-150). Anions were eluted by 1.8 mM Na2CO3 + 1.7 mM NaHCO3. For cation system, ions were eluted by 4 mM dihydroxysuccinic acid + 0.75 mM pyridinedicarboxylic acid. In both systems, the flow rate of eluent was set at 1.0 mL/min, and the relative standard deviation of the measurement results was less than 3%. Iodide was measured using an inductive couple plasma mass spectrometry (ICP-MS) (Element II, ThermoFisher), and the analytical precisions were estimated to be <2%. Samples for iodide measurement was prepared by diluting in 1‰ aqua ammonia with 10 ppb of Rh as an internal standard.
The gradients of indicators are calculated by least-squares fitting, generating a correlation coefficient (r2) used to access the linearity of geochemical profiles. Detailed data of core sites are shown in Table 1. The r2 value ranges from 0-1, which represents a strong correlation at value 1, a decoupling correlation at value 0. Both the gradient and corresponding r2 values are mapped over the study area using Surfer® software.
3 Results
We analyzed 246 pore water samples from 23 piston cores in Shenhu area (Figure 1). Due to the space limitation and since the gradient redox is what we mainly discuss, in this paper we only show the gradient and r2 calculated from sulfate, iodide and calcium plus magnesium concentration profiles (Table 1).
The downward concentration gradients of sulfate, calcium plus magnesium ions and iodide vary from -0.2 to -3.72, from 0.94 to -3.15 and from 0.13 to 11.3, respectively.
HS428PC has the steepest decreasing gradient of sulfate and the steepest increasing gradient of iodide. HS251PC has the lowest sulfate decreasing gradient and HS60PC has the lowest iodide increasing gradient. For calcium plus magnesium, the downward gradient values exhibit both negative and positive. The highest downward increasing gradient of calcium plus magnesium occurs in HS08-6PC and the highest downward decreasing gradient is in HS296PC. Almost all the sites with relatively high absolute gradient values have the r2 values close to 1.
The absolute values of sulfate and iodide downward gradient have a positive relationship, which means that when sulfate decreasing trend is steeper, the iodide has a steeper increasing trend. The calcium plus magnesium concentration gradient is more complicated, but in general, the absolute values exhibit a positive relationship with sulfate and iodide.
The sites with highest absolute gradient values of sulfate, iodide or authigenic carbonate intensity [i.e., the depletion of (Ca2+) + (Mg2+)] are invariably distributed in the northeast region of the study area (in red circles from Figure 2A, Figure 3A and Figure 4A) where the r2 values are also high. The absolute gradient values gradually decrease from the northeast corner to the rest of the study area, with most of study area showing low absolute values except the northeast region. In addition, it has to be noticed that high r2 values also exist in other regions except for the northeast area.
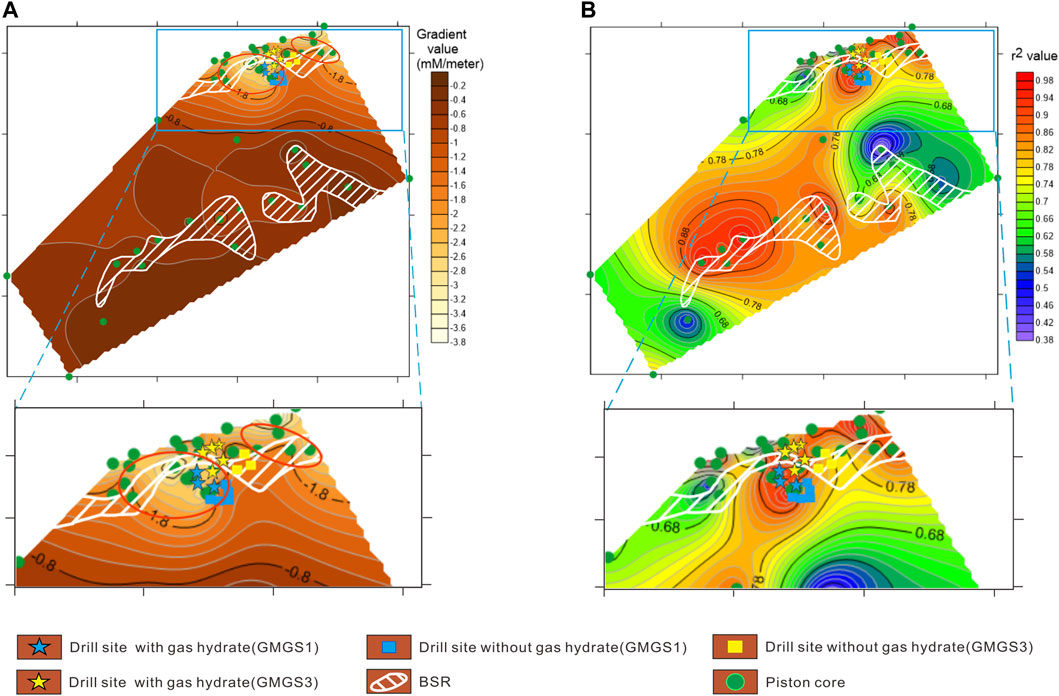
FIGURE 2. Contour maps of sulfate gradient in units of mM/meter (A) and corresponding r2 values (B) in the research area. Note the location of cores identified by green-filled circles and BSR outlines (white, from GMGS reports). The stars represent the drill sites, in which gas hydrates were recovered (blue stars represent GMGS1, yellow stars represent GMGS3). The squares represent the drill sites which has no gas hydrates recovered. In 6A, the lighter color indicates a larger gradient, which indicates a higher possibility of gas hydrate existence. In 6B, warmer colors (red) indicate a sulfate gradient that is closer to linearity, usually representing a larger portion of sulfate depletion due to AOM. Based on the gradient and r2, the most promising area is inferred which are marked by red circles in 6A.
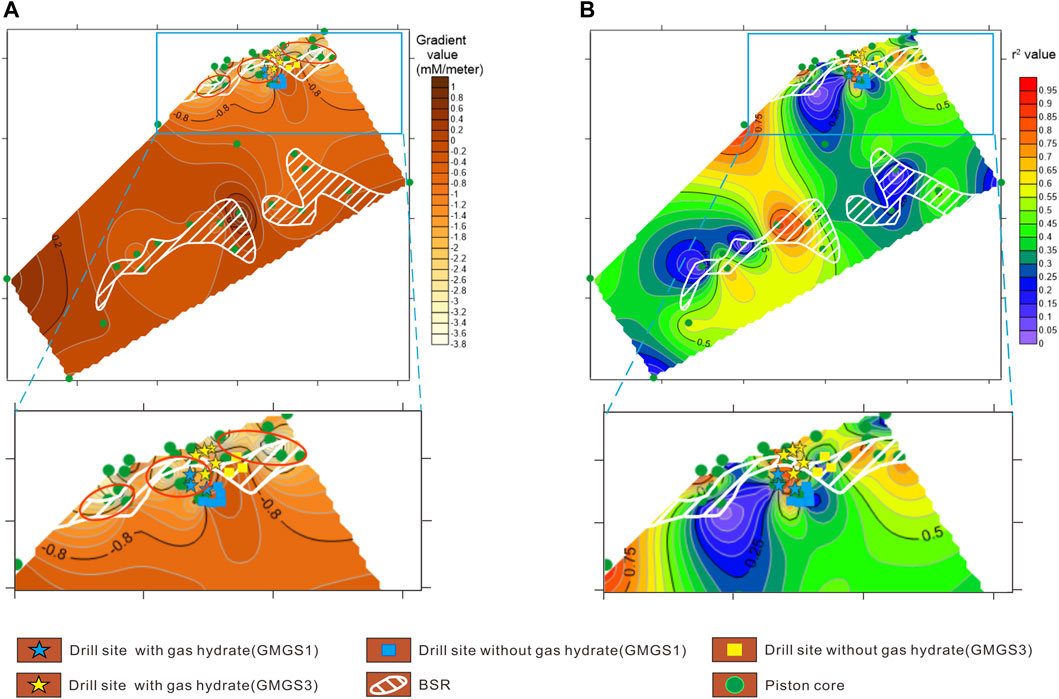
FIGURE 3. Contour maps of gradients of authigenic carbonate precipitation intensity in units of mM/meter (A) and corresponding r2 values (B) in the research area. Note the location of cores identified by green-filled circles and BSR outlines (white, from GMGS reports). The stars represent the drill site, in which gas hydrates were recovered (blue stars represent GMGS1, yellow stars represent GMGS3). The squares represent the drill site that has no gas hydrates recovered. In 7A, the lighter color indicates a larger gradient, which indicates a higher possibility of gas hydrate existence. In 7B, warmer colors (red) indicate that the corresponding gradient is closer to linearity. Based on the gradient and r2, the most promising area is inferred which are marked by red circles in 7A.
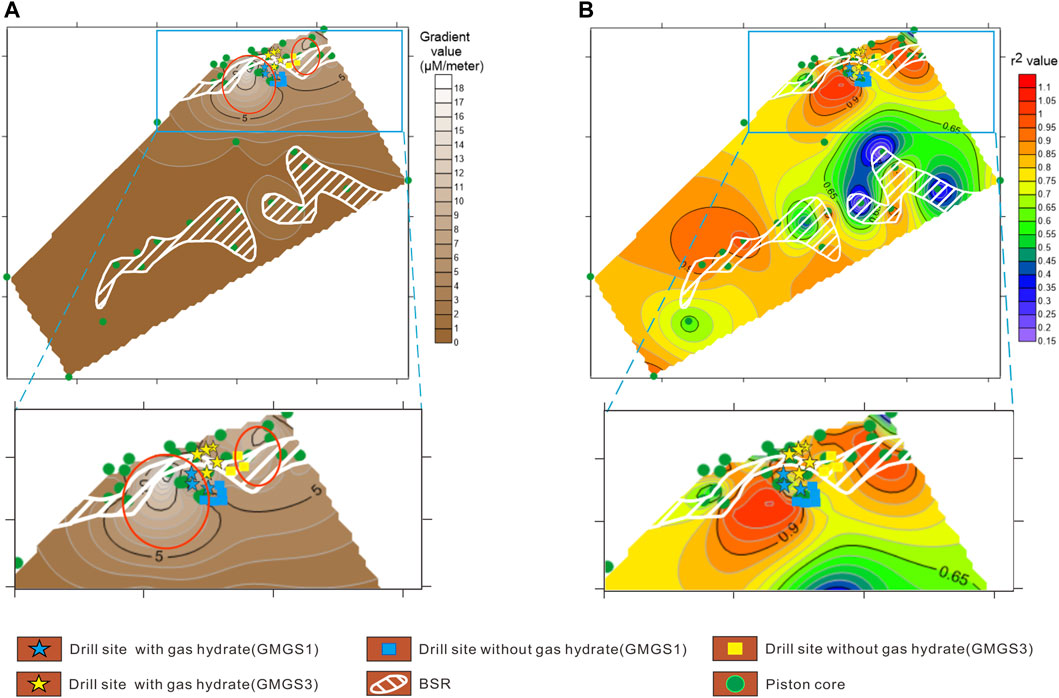
FIGURE 4. Contour maps of iodine gradient in units of μM/meter (A) and corresponding r2 values (B) in the research area. Note the location of cores identified by green-filled circles and BSR outlines (white, from GMGS reports). The stars represent the drill site, in which gas hydrates were recovered (blue stars represent GMGS1, yellow stars represent GMGS3). The squares represent the drill site that has no gas hydrates recovered. In 8A, the lighter color indicates the larger gradient, which indicates a higher possibility of gas hydrate existence. In 8B, warmer colors (red) indicate that the corresponding gradient is closer to linearity. Based on the gradient and r2, the most promising area is inferred which are marked by red circles in 8A.
4 Discussion
4.1 Geochemical Anomalies Associated With Gas Hydrate
4.1.1 AOM Process
In the gas hydrate-bearing areas, AOM is usually strong due to the enrichment of methane. Methane flux from below causes a significant portion of the interstitial sulfate pool to be consumed through AOM and deplete sulfate more rapidly than OSR alone (Borowski et al., 1996; Borowski et al., 2000). Under the correct condition, intense AOM can result in the linear depletion of sulfate, while OSR leads to a convex curve of the sulfate gradient. (Borowski et al., 1996; Niewöhner et al., 1998; Dickens, 2001; Joye et al., 2004). Thus, under the condition where AOM is intense due to the upward methane flux from below in the methane seepage area of the gas hydrate zone, the curve of sulfate gradient is impacted mainly by AOM rather than OSR. As shown in Figure 5A, the rapid consumption of sulfate with depth is common in gas hydrate-bearing sites all over the world. Despite the general downtrend, there are some differences in sulfate gradients among different regions due to the various tectonic and sedimentary environments. For the same region, larger methane flux usually leads to steeper sulfate gradients, as clearly shown in Figure 5A. For example, in Black Ridge, the methane flux of Site 995 is larger than Site 991, and the sulfate gradient of Site 995 is steeper. A similar situation occurs in Hydrate Ridge where the sulfate gradient of Site 1,251 is steeper than that of Site 1,244 perhaps due to the larger methane flux of Site 1,251.
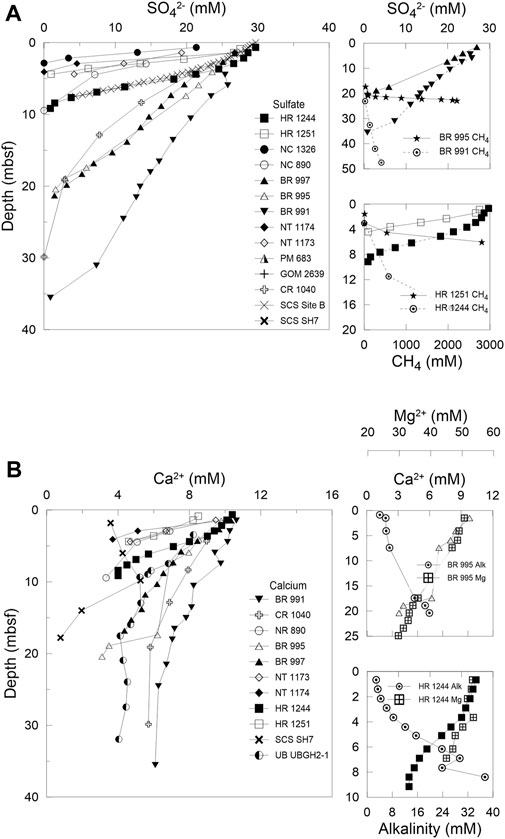
FIGURE 5. Profiles of sulfate (A) and calcium (B) with depth in gas hydrate-bearing areas worldwide. Data are selected from Hydrate Ridge (HR) (Milkov et al., 2003; Torres et al., 2004), Blake Ridge (BR) (Borowski et al., 1996; Rodriguez et al., 2000; Dickens, 2001), Nankai Trough (NT) (Kastner et al., 1993; Newberry et al., 2004), Peru Margin (PM) (Kastner et al., 1990; Kvenvolden and Kastner, 1990), North Cascadia (NC) (Lu et al., 2008), Gulf of Mexico (GOM) (Aharon and Fu, 2000), Costa Rica (CR) (Teichert et al., 2009), South China Sea (SCS) (Wu et al., 2011; Ye et al., 2016) and Ulleung Basin (UB) (Kim et al., 2007; Kim et al., 2011).
Increased consumption of sulfate through AOM also can result in increases in alkalinity near the SMTZ, producing a diagenetic environment beneficial to the precipitation of dissolved calcium and magnesium to form authigenic carbonate cement:
Concentrations of calcium and magnesium ions usually decrease with depth (Raiswell, 1988; Blair and Aller, 1995; Rodriguez et al., 2000; Tong et al., 2013). As the result of authigenic carbonate precipitation, Ca2+ exhibits a similar downward trend to that of sulfate in these gas hydrate-bearing sites (Figure 5B). The concentrations of calcium and magnesium ions decrease as the alkalinity rises with depth. The alkalinity often reaches its maximum in the SMTZ, as exemplified in both Blake Ridge and Hydrate Ridge (Figure 5B). It shows that AOM has a great effect on calcium and magnesium ions. Consequently, strong authigenic carbonate precipitation could occur in the gas hydrate-bearing areas.
4.1.2 Decomposition of Organic Matter
The gas source that is necessary for hydrate formation, thermogenic or biogenic, ultimately originates from the decomposition of organic matter (Kvenvolden and McMenamin, 1980; Kvenvolden, 1998; Clennell et al., 1999; Wallmann et al., 2006). Biophilic elements such as iodine are also released in the process of organic matter decomposition, contributing dissolved iodide (I-) to pore waters:
Where κ represents the molar fraction of iodide in organic matter.
Within sulfate reduction zone, the organic matters are reduced by sulfate, and also release the iodide:
Where κ also represents the molar fraction of iodide in organic matter. High iodide gradient implies a high organic matter decomposition rate, no matter in gas source region below SMTZ or sulfate reduction zone, which will cause a high reaction rate of both methanogenesis and OSR. Therefore, high methane flux can still be indicated by high iodide gradient although the OSR rate can be high. In addition, the iodide migrates from gas source can elevate the iodide gradient to several magnitude high (e.g., HS428PC relative to 60PC), which is much more significant than the iodide released from OSR.
As a result, iodide exhibits abnormally high concentration in gas hydrate-bearing areas (Kastner et al., 1993; Martin et al., 1993; Egeberg and Dickens, 1999; Fehn et al., 2003; Fehn et al., 2006; Yang et al., 2010). As shown in Figure 6 in gas hydrate-bearing areas, the iodide concentration of pore water ([I-]porewater) increases to several hundred times that of the seawater value ([I-]seawater). There is also a regional variation in iodide gradient because the types and activity of organic matter that have a regional difference could affect the process of decomposition. The organic matter of large activity is liable to decomposition.It is noteworthy that the abnormally high concentration of iodide is not only supplied by the decomposition of local organic matter but may also be influenced by other sources (Fehn et al., 2006; Lu et al., 2008). Fehn et al. (2006) proposed that a large fraction of the iodine is derived from another source that was located to the east (40 km) of Hydrate Ridge through the iodine isotopic dating of pore water. Because of the similar diffusion coefficients between iodide and methane, external fluids from organic matter decomposition simultaneously transport iodine and methane to this site, which promotes the gas hydrate formation by offering a major fraction of methane in the Oregon Hydrate Ridge. To conclude, the high concentration of iodide would reflect massive organic matter decomposition, no matter from internal or external sources, which is helpful to the formation of gas hydrate.
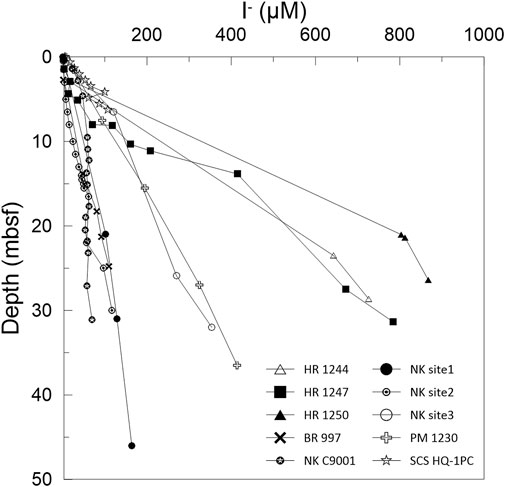
FIGURE 6. Profile of iodide concentration with depth in pore water worldwide. Data are selected from Nankai Trough (NK) (Fehn et al., 2003; Muramatsu et al., 2007), Hydrate Ridge (HR) (Torres et al., 2004; Fehn et al., 2005; Lu et al., 2008), Blake Ridge (BR) (Egeberg and Dickens, 1999), South China Sea (SCS) (Yang et al., 2010) and Peru Margin (PM) (Martin et al., 1993).
4.2 Definition of Anomaly Indicators
In gas hydrate-bearing areas, the upward methane flux usually exists due to the more likely methane seepage, which may lead to steep sulfate gradients and carbonate precipitation. What’s more, the enrichment of iodide may also indicate the existence of the regional methane source because the iodine and methane may be derived from the same source. Accordingly, we use three pore-water geochemical indicators – sulfate gradients, calcium plus magnesium gradients (a proxy for authigenic carbonate formation), and iodide gradient to recognize potential gas hydrate occurrence in underlying sediments. In each case, the higher the gradient, the greater the possibility of gas hydrate formation (Figure 7).
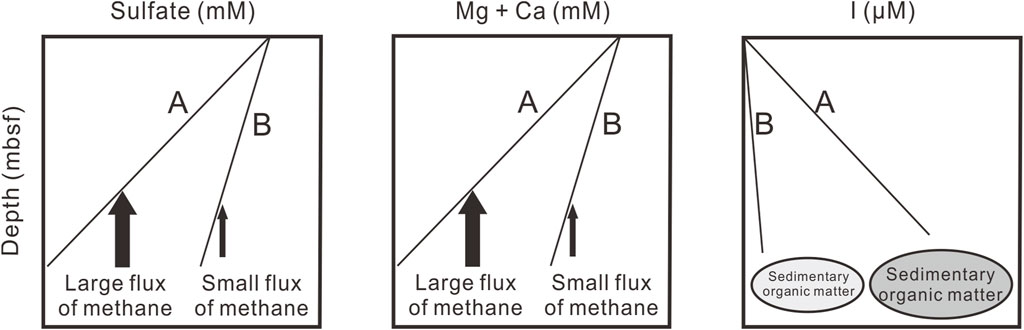
FIGURE 7. The definition of the gradients of sulfate, Mg2++Ca2+, and iodide. For all these indicators, the gradient of line A is larger than that of line B.
As mentioned above, magnesium and calcium are sensitive to the flux of methane, and iodine reflects the potentiality of the gas source. The indicator proposed here has several advantages over the SMTZ depth proxy. One major shortcoming of using the SMTZ depth as the indicator for hydrate recognition is that the SMTZ depth is liable to be altered by environmental factors such as irrigation, bioturbation, and submarine slide displacement (Fossing et al., 2000; Zabel and Schulz, 2001; Croguennec et al., 2016). As shown in Figure 8, the SMTZ of Site A is shallower than that of Site B, which nevertheless contains an interfered part. In this case, using the SMTZ depth as the indicator for hydrate recognition may result in erroneous results. However, by using the gradient of sulfate as the indicator, the correct conclusion can be obtained.
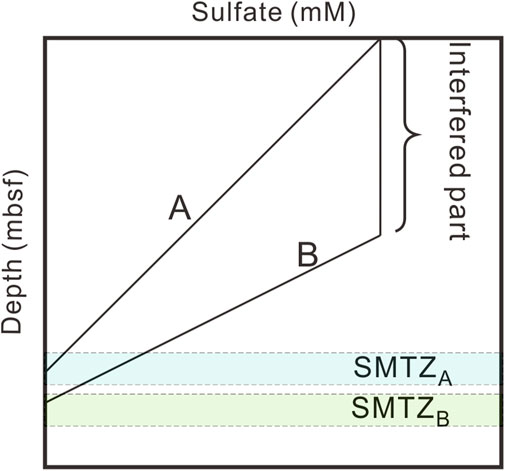
FIGURE 8. Alteration of depth to the sulfate-methane transition zone (SMTZ) by various processes such as irrigation, bioturbation, and submarine sediment slides. Note that the SMTZ depth of A is shallower than that of B and possesses a different gradient.
4.3 The Contour Maps of three Indicators and Corresponding r2 Value in the Shenhu Area
We have accumulated sizeable piston-coring data in the study area during the past decade, which provides us with an ideal chance to test the robustness of this new criterion. The gradients of the three indicators (i.e., sulfate, Mg2++Ca2+, and iodine) of all the core sites in the research area are presented in the form of contour maps. For each indicator, the r2 values are also given in the corresponding contour map to characterize the shape and linearity of the concentration profile and further assess its relationship to AOM and underlying gas hydrate. The distribution of BSRs on contour maps is acquired from Guangzhou Marine Geological Survey reports.
4.3.1 Sulfate Gradient
Mapping results of sulfate gradients are shown in Figure 2A. In Figure 2A, the lighter the color is, the larger the sulfate gradient is (see Figure 7 for the definition), which corresponds to a larger possibility of hydrate existence. The lightest colors can be found mainly in the northeast of the study area and are roughly divided into two parts. Both two parts (red circles) are the most promising areas for gas hydrate occurrence. The yellow stars and blue stars in Figure 2 are drill sites where gas hydrate samples have been collected in GMGS1 and GMGS3 expedition (yellow represents GMGS1, blue represents GMGS3), including the first gas hydrate sample of China, recovered during the GMGS1 expedition, 2007 (Wu et al., 2013). The yellow squares and blue squares in Figure 2 represent drill sites that have no gas hydrate samples recovered. It is shown in Figure 2A that the stars locate mostly in one of the red circles, while squares locate mainly out of the red circles. It indicates that the gradient of the sulfate is related to the occurrence of gas hydrate to some extent. Corresponding r2 values of stars are also high (close to 1) in the contour map of r2 (Figure 2B). The other red circle is the promising area predicted by this criterion for gas hydrate occurrence and it needs to be tested by future exploration.
4.3.2 Authigenic Carbonate Precipitation Intensity
The contour map of the intensity of authigenic carbonate precipitation exhibits a similar result to that of sulfate gradients (Figure 3A). It also suggests that the northeastern part of the study area, which however could be roughly divided into three small parts, is the most promising area of gas hydrate occurrence. The stars are located in the middle red circle, which is coherent with the mapping result of the criterion. Also, the other two red circles are promising areas for gas hydrate occurrence. In Figure 3B, the corresponding r2 values in the red circles are high (close to 1), which ensures the reliability of the gradient estimation.
4.3.3 Iodide Gradient
As for iodide, in the contour map (Figure 4), the lighter (white area) the color is, the larger the gradient is. In Figure 4A, two promising areas (red circles) for gas hydrate occurrence are identified. For the third time, the stars are located in one of the red circles, which is again coherent with the mapping result of the criterion. The other circle is a promising area for the existence of gas hydrate. The r2 values in the red circles are also close to 1, which again supports the reliability of the gradient values.
4.4 The Correlation of Anomaly Indicators
Through the correlation diagrams of authigenic carbonate precipitation intensity, sulfate, and iodine gradients of the study area, there is some correlation among them (Figure 9). It suggests that all of them are controlled by similar factors, i.e., the decomposition of organic matter and methane production. On the one hand, the organic matter fermentation and disintegration (both biological and thermal decomposition) could release methane and iodide, so the increase in methane and iodide are coupled to some degree. On the other hand, an increased flux of methane would strengthen the AOM process, which fuels the sulfate reduction and the precipitation of calcium and magnesium. Thus, we propose that these three indicators could be used together as powerful tools in the exploration of gas hydrates.
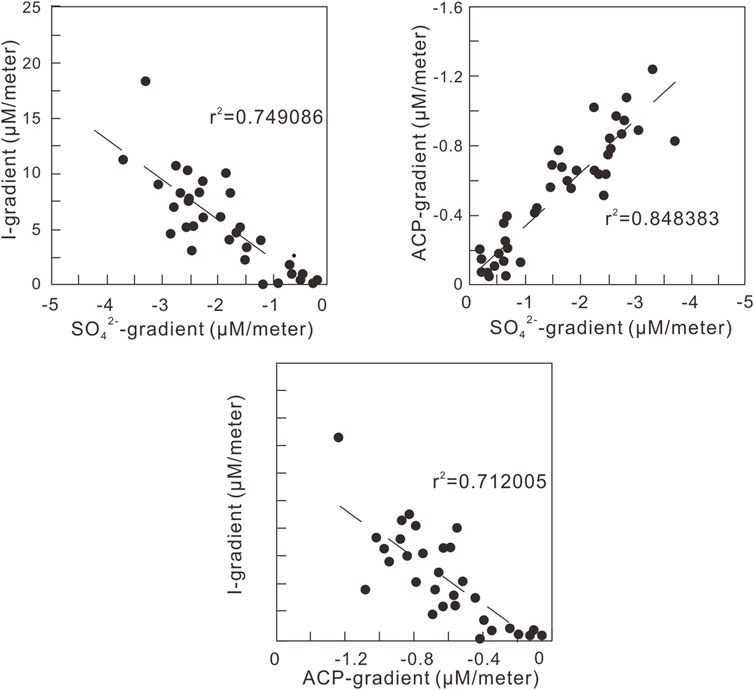
FIGURE 9. Correlation of authigenic carbonate precipitation intensity (denoted as ACP), sulfate, and iodide gradients in the study area. The relation of these three gradients shows a good correlation (r2 is 0.75, 0.85, 0.71 respectively). It indicates that the gradients of sulfate, Ca + Mg, and iodide is influenced by the same factors (AOM, methane flux, and the decomposition of organic matter)
5 Conclusion
We investigated the concentration profiles of sulfate, calcium, magnesium and iodide in pore water from worldwide main gas hydrate-bearing sites. Each kind of ion has a similar trend in different sites, but shows different downward gradients.
The gradients of sulfate concentration, calcium plus magnesium concentration and authigenic carbonate precipitation intensity can indicate the rate of AOM, which is mainly influenced by the flux of methane. Iodide concentration is strongly affected by the decomposition of organic matter from gas source, and can indicate the methane flux from below or other sources. Thus, the higher the values of these indicators are, the more likely gas hydrate exists in the deep. Also, in some cases where irrigation, bioturbation or submarine slide displacement occurs, the depth of SMTZ is not accurate to indicate the flux of methane, and the sulfate gradient can be a more accurate one.
According to the response of pore water composition in shallow sediment to the high flux of methane in gas hydrate-bearing areas, we proposed that the gradients of sulfate, authigenic carbonate precipitation, and iodide together could be comprehensively employed as the indicators in the exploration of gas hydrate. These indicators were applied to the Shenhu area, SCS, and the results were presented in the form of contour maps. Satisfactory, the most promising area for hydrate occurrence predicted in the three contour maps is consistent with the discovery of GMGS1 and GMGS3 expeditions, which indicates the availability of this criterion. According to the criterion, a new promising area for the existence of gas hydrate was identified, though it needs to be tested by future exploration. We believe that this new geochemical criterion will benefit the exploration of gas hydrate in the future.
Data Availability Statement
The raw data supporting the conclusions of this article will be made available by the authors, without undue reservation.
Author Contributions
TY provided the main idea and financial support. XA wrote the main part of the manuscript. RZ checked the English and finished the manuscript. YL finished the experiments in the manuscript. PS gave some important suggestions about manuscript revision.
Funding
This study was supported by National Natural Science Foundation of China (41873011) and National Key Scientific Project organized by the China Geological Survey (Grant No. GZH201200305-06-03).
Conflict of Interest
The authors declare that the research was conducted in the absence of any commercial or financial relationships that could be construed as a potential conflict of interest.
Publisher’s Note
All claims expressed in this article are solely those of the authors and do not necessarily represent those of their affiliated organizations, or those of the publisher, the editors and the reviewers. Any product that may be evaluated in this article, or claim that may be made by its manufacturer, is not guaranteed or endorsed by the publisher.
References
Aharon, P., and Fu, B. (2000). Microbial Sulfate Reduction Rates and Sulfur and Oxygen Isotope Fractionations at Oil and Gas Seeps in Deepwater Gulf of Mexico. Geochimica Cosmochimica Acta 64 (2), 233–246. doi:10.1016/s0016-7037(99)00292-6
Barnes, R. O., and Goldberg, E. D. (1976). Methane Production and Consumption in Anoxic Marine Sediments. Geol 4 (5), 297–300. doi:10.1130/0091-7613(1976)4<297:mpacia>2.0.co;2
Berner, R. A. (1980). Early Diagenesis: A Theoretical Approach. New Jersey, United States: Princeton University Press.
Bhatnagar, G., Chapman, W. G., Dickens, G. R., Dugan, B., and Hirasaki, G. J. (2008). Sulfate‐methane Transition as a Proxy for Average Methane Hydrate Saturation in Marine Sediments. Geophys. Res. Lett. 35 (3), L03611. doi:10.1029/2007gl032500
Blair, N. E., and Aller, R. C. (1995). Anaerobic Methane Oxidation on the Amazon Shelf. Geochimica Cosmochimica Acta 59 (18), 3707–3715. doi:10.1016/0016-7037(95)00277-7
Boetius, A., Ravenschlag, K., Schubert, C. J., Rickert, D., Widdel, F., Gieseke, A., et al. (2000). A Marine Microbial Consortium Apparently Mediating Anaerobic Oxidation of Methane. Nature 407 (6804), 623–626. doi:10.1038/35036572
Borowski, W. S., Hoehler, T. M., Alperin, M. J., Rodriguez, N. M., and Paull, C. K. (2000). Significance of Anaerobic Methane Oxidation in Methane-Rich Sediments Overlying the Blake Ridge Gas Hydrates. Proc. ocean Drill. program, Sci. results 164, 87–99. doi:10.2973/odp.proc.sr.164.214.2000
Borowski, W. S., Paull, C. K., and Ussler, W. (1999). Global and Local Variations of Interstitial Sulfate Gradients in Deep-Water, Continental Margin Sediments: Sensitivity to Underlying Methane and Gas Hydrates. Mar. Geol. 159 (1), 131–154. doi:10.1016/s0025-3227(99)00004-3
Borowski, W. S., Paull, C. K., and Ussler, W. (1996). Marine Pore-Water Sulfate Profiles Indicate In Situ Methane Flux from Underlying Gas Hydrate. Geol 24 (7), 655–658. doi:10.1130/0091-7613(1996)024<0655:mpwspi>2.3.co;2
Boswell, R., and Collett, T. S. (2011). Current Perspectives on Gas Hydrate Resources. Energy Environ. Sci. 4 (4), 1206–1215. doi:10.1039/c0ee00203h
Boswell, R., Shelander, D., Lee, M., Latham, T., Collett, T., Guerin, G., et al. (2009). Occurrence of Gas Hydrate in Oligocene Frio Sand: Alaminos Canyon Block 818: Northern Gulf of Mexico. Mar. Petroleum Geol. 26 (8), 1499–1512. doi:10.1016/j.marpetgeo.2009.03.005
Brown, P. J., Sanders, R., McDonagh, E., Henson, S., Best, A. I., Poulton, A. J., et al. (2016). “Impacts and Effects of Ocean Warming on Carbon Management Including Methane Hydrates,” in Explaining Ocean Warming, Causes, Scale, Effects and Consequence. Editors D. Laffoley, and J. M. Baster (IUCN), 380–384. doi:10.2305/IUCN.CH.2016.08.en
Chen, D., Wang, X., Völker, D., Wu, S., Wang, L., Li, W., et al. (2016). Three Dimensional Seismic Studies of Deep-Water Hazard-Related Features on the Northern Slope of South China Sea. Mar. Petroleum Geol. 77, 1125–1139. doi:10.1016/j.marpetgeo.2016.08.012
Chong, Z. R., Yang, S. H. B., Babu, P., Linga, P., and Li, X.-S. (2016). Review of Natural Gas Hydrates as an Energy Resource: Prospects and Challenges. Appl. Energy 162, 1633–1652. doi:10.1016/j.apenergy.2014.12.061
Clennell, M. B., Hovland, M., Booth, J. S., Henry, P., and Winters, W. J. (1999). Formation of Natural Gas Hydrates in Marine Sediments: 1. Conceptual Model of Gas Hydrate Growth Conditioned by Host Sediment Properties. J. Geophys. Res. 104 (B10), 22985–23003. doi:10.1029/1999jb900175
Collett, T. S. (2002). Energy Resource Potential of Natural Gas Hydrates. AAPG Bull. 86 (11), 1971–1992. doi:10.1306/61eeddd2-173e-11d7-8645000102c1865d
Croguennec, C., Ruffine, L., Dennielou, B., Baudin, F., Caprais, J.-C., Guyader, V., et al. (2016). Evidence and Age Estimation of Mass Wasting at the Distal Lobe of the Congo Deep-Sea Fan. Deep Sea Res. Part II Top. Stud. Oceanogr. 142, 50–63. doi:10.1016/j.dsr2.2016.12.013
Dickens, G. R. (2001). Sulfate Profiles and Barium Fronts in Sediment on the Blake Ridge: Present and Past Methane Fluxes through a Large Gas Hydrate Reservoir. Geochimica Cosmochimica Acta 65 (4), 529–543. doi:10.1016/s0016-7037(00)00556-1
Dumke, I., Burwicz, E. B., Berndt, C., Klaeschen, D., Feseker, T., Geissler, W. H., et al. (2016). Gas Hydrate Distribution and Hydrocarbon Maturation North of the Knipovich Ridge, Western Svalbard Margin. J. Geophys. Res. Solid Earth 121, 1405–1424. doi:10.1002/2015jb012083
Egeberg, P. K., and Dickens, G. R. (1999). Thermodynamic and Pore Water Halogen Constraints on Gas Hydrate Distribution at ODP Site 997 (Blake Ridge). Chem. Geol. 153 (1), 53–79. doi:10.1016/s0009-2541(98)00152-1
Fehn, U., Lu, Z., and Tomaru, H. (2005). Data from. 129 I/I Ratios and Halogen Concentrations in Pore Waters of the Hydrate Ridge: Relevance for the Origin of Gas Hydrates in ODP Leg 204. Proceedings of the Ocean Drilling Program: Scientific Results 204
Fehn, U., Lu, Z., and Tomaru, H. (2006). Data Report: 129I/I Ratios and Halogen Concentrations in Pore Water of Hydrate Ridge and Their Relevance for the Origin of Gas Hydrates: A Progress Report. Proc. Ocean Drill. Program Sci. Results 204. doi:10.2973/odp.proc.sr.204.107.2006
Fehn, U., Snyder, G. T., Matsumoto, R., Muramatsu, Y., and Tomaru, H. (2003). Iodine Dating of Pore Waters Associated with Gas Hydrates in the Nankai Area, Japan. Geol 31 (6), 521–524. doi:10.1130/0091-7613(2003)031<0521:idopwa>2.0.co;2
Fossing, H., Ferdelman, T. G., and Berg, P. (2000). Sulfate Reduction and Methane Oxidation in Continental Margin Sediments Influenced by Irrigation (South-East Atlantic off Namibia). Geochimica Cosmochimica Acta 64 (5), 897–910. doi:10.1016/s0016-7037(99)00349-x
Gieskes, J. M., Warme, J. E., Douglas, R. G., and Winterer, E. L. (1981). “Deep-Sea Drilling Interstitial Water Studies: Implications for Chemical Alteration of the Oceanic Crust, Layers I and II,” in The Deep Sea Drilling Project: A Decade of Progress (United States: SEPM Society for Sedimentary Geology). doi:10.2110/pec.81.32.0149
Han, X., Suess, E., Huang, Y., Wu, N., Bohrmann, G., Su, X., et al. (2008). Jiulong Methane Reef: Microbial Mediation of Seep Carbonates in the South China Sea. Mar. Geol. 249 (3), 243–256. doi:10.1016/j.margeo.2007.11.012
Haq, B. U. (2000). “Climatic Impact of Natural Gas Hydrate,” in Natural Gas Hydrate (Berlin, Germany: Springer), 137–148.
Hesse, R. (2003). Pore Water Anomalies of Submarine Gas-Hydrate Zones as Tool to Assess Hydrate Abundance and Distribution in the Subsurface: What Have We Learned in the Past Decade? Earth-Science Rev. 61 (1), 149–179. doi:10.1016/s0012-8252(02)00117-4
Hornbach, M. J., Bangs, N. L., and Berndt, C. (2012). Detecting Hydrate and Fluid Flow from Bottom Simulating Reflector Depth Anomalies. Geology 40 (3), 227–230. doi:10.1130/g32635.1
Jiang, S.-Y., Yang, T., Ge, L., Yang, J.-H., Wu, N.-Y., Liu, J., et al. (2008). "Geochemical Anomaly of Pore Waters and Implications for Gas Hydrate Occurence in the South China Sea", in: Proceedings of the 6th International Conference on Gas Hydrates (ICGH 2008). British Columbia, CANADA, July 6–10, 2008.
Jin, J., Wang, X., Guo, Y., Li, J., Li, Y., Zhang, X., et al. (2020). Geological Controls on the Occurrence of Recently Formed Highly Concentrated Gas Hydrate Accumulations in the Shenhu Area, South China Sea. Mar. Petroleum Geol. 116, 104294. doi:10.1016/j.marpetgeo.2020.104294
Joye, S. B., Boetius, A., Orcutt, B. N., Montoya, J. P., Schulz, H. N., Erickson, M. J., et al. (2004). The Anaerobic Oxidation of Methane and Sulfate Reduction in Sediments from Gulf of Mexico Cold Seeps. Chem. Geol. 205 (3), 219–238. doi:10.1016/j.chemgeo.2003.12.019
Kamath, V. A. (1990). Clatrate Hydrates of Natural gases. By E. Dendy Sloan, Jr., Marcel Dekker, lnc., New York, 1990, 664 pp.,$125.00(U.S. and Canada),$150.00(other countries). AIChE J. 36 (12), 1931–1932. doi:10.1002/aic.690361223
Kastner, M., Elderfield, H., Jenkins, W. J., Gieskes, J. M., Gamo, T., Hill, I., et al. (1993). Geochemical and isotopic evidence for fluid flow in the western Nankai subduction zone, Japan. Proc. Ocean Drill. Program Sci. Results 131, 397–413. doi:10.2973/odp.proc.sr.131.143.1993
Kastner, M., Elderfield, H., Martin, J. B., Suess, E., Kvenvolden, K. A., and Garrison, R. E. (1990). Diagenesis and interstitial-water chemistry at the Peruvian continental margin—major constituents and strontium isotopes. Proc. Ocean Drill. Program Sci. Results 112, 413–440.
Kim, G. Y., Yi, B. Y., Yoo, D. G., Ryu, B. J., and Riedel, M. (2011). Evidence of gas hydrate from downhole logging data in the Ulleung Basin, East Sea. Mar. Petroleum Geol. 28 (10), 1979–1985. doi:10.1016/j.marpetgeo.2011.01.011
Kim, J.-H., Hachikubo, A., Kida, M., Minami, H., Lee, D.-H., Jin, Y. K., et al. (2020). Upwarding gas source and postgenetic processes in the shallow sediments from the ARAON Mounds, Chukchi Sea. J. Nat. Gas Sci. Eng. 76, 103223. doi:10.1016/j.jngse.2020.103223
Kim, J.-H., Park, M.-H., Tsunogai, U., Cheong, T.-J., Ryu, B.-J., Lee, Y.-J., et al. (2007). Geochemical characterization of the organic matter, pore water constituents and shallow methane gas in the eastern part of the Ulleung Basin, East Sea (Japan Sea). Isl. Arc 16 (1), 93–104. doi:10.1111/j.1440-1738.2007.00560.x
Kvenvolden, K. A. (1998). A primer on the geological occurrence of gas hydrate. Geol. Soc. Lond. Spec. Publ. 137 (1), 9–30. doi:10.1144/gsl.sp.1998.137.01.02
Kvenvolden, K. A., Ginsburg, G. D., and Soloviev, V. A. (1993). Worldwide distribution of subaquatic gas hydrates. Geo-Marine Lett. 13 (1), 32–40. doi:10.1007/bf01204390
Kvenvolden, K. A., and Kaster, M. (1990). Gas hydrates of the Peruvian outer continental margin. Proc. Ocean Drill. Program Sci. Results 112, 517–526. doi:10.2973/odp.proc.sr.112.147.1990
Kvenvolden, K. A., and McMenamin, M. A. (1980). Hydrates of natural gas; a review of their geologic occurrence. U. S. Geol. Surv. Rep. doi:10.3133/cir825
Le, A. N., Huuse, M., Redfern, J., Gawthorpe, R. L., and Irving, D. (2015). Seismic characterization of a Bottom Simulating Reflection (BSR) and plumbing system of the Cameroon margin, offshore West Africa. Mar. Petroleum Geol. 68, 629–647. doi:10.1016/j.marpetgeo.2014.12.006
Li, L., Lei, X., Zhang, X., and Zhang, G. (2012). Heat flow derived from BSR and its implications for gas hydrate stability zone in Shenhu Area of northern South China Sea. Mar. Geophys Res. 33 (1), 77–87. doi:10.1007/s11001-012-9147-3
Li, L., Liu, H., Zhang, X., Lei, X., and Sha, Z. (2015). BSRs, estimated heat flow, hydrate-related gas volume and their implications for methane seepage and gas hydrate in the Dongsha region, northern South China Sea. Mar. Petroleum Geol. 67, 785–794. doi:10.1016/j.marpetgeo.2015.07.008
Liu, C.-S., Schnurle, P., Wang, Y., Chung, S.-H., Chen, S.-C., and Hsiuan, T.-H. (2006). Distribution and characters of gas hydrate offshore of southwestern Taiwan. Terr. Atmos. Ocean. Sci. 17 (4), 615. doi:10.3319/tao.2006.17.4.615(gh)
Lu, Z., Tomaru, H., and Fehn, U. (2008). Iodine ages of pore waters at Hydrate Ridge (ODP Leg 204), Cascadia Margin: Implications for sources of methane in gas hydrates. Earth Planet. Sci. Lett. 267 (3), 654–665. doi:10.1016/j.epsl.2007.12.015
Lüdmann, T., and Wong, H. K. (1999). Neotectonic regime on the passive continental margin of the northern South China Sea. Tectonophysics 311 (1), 113–138.
Lüdmann, T., Wong, H. K., and Wang, P. (2001). Plio–Quaternary sedimentation processes and neotectonics of the northern continental margin of the South China Sea. Mar. Geol. 172 (3), 331–358. doi:10.1016/S0025-3227(00)00129-8
Luo, M., Chen, L., Tong, H., Yan, W., and Chen, D. (2014). Gas Hydrate Occurrence Inferred from Dissolved Cl− Concentrations and δ18O Values of Pore Water and Dissolved Sulfate in the Shallow Sediments of the Pockmark Field in Southwestern Xisha Uplift, Northern South China Sea. Energies 7 (6), 3886–3899. doi:10.3390/en7063886
Makogon, Y., Holditch, S., and Makogon, T. (2007). Natural gas-hydrates—A potential energy source for the 21st Century. J. Petroleum Sci. Eng. 56 (1), 14–31. doi:10.1016/j.petrol.2005.10.009
Martin, J. B., Gieskes, J. M., Torres, M., and Kastner, M. (1993). Bromine and iodine in Peru margin sediments and pore fluids: implications for fluid origins. Geochimica Cosmochimica Acta 57 (18), 4377–4389. doi:10.1016/0016-7037(93)90489-j
Milkov, A. V., Claypool, G. E., Lee, Y.-J., Xu, W., Dickens, G. R., Borowski, W. S., et al. (2003). In Situ methane concentrations at Hydrate Ridge, offshore Oregon: New constraints on the global gas hydrate inventory from an active margin. Geology 31 (10), 833–836. doi:10.1130/g19689.1
Miller, J. J., Lee, M. W., and von Huene, R. (1991). An Analysis of a Seismic Reflection from the Base of a Gas Hydrate Zone, Offshore Peru (1). Aapg Bull. 75 (5), 910–924. doi:10.1306/0c9b288f-1710-11d7-8645000102c1865d
Mitchell, J. F. B. (1989). The “greenhouse” effect and climate change. Rev. Geophys. 27 (1), 115–139. doi:10.1029/rg027i001p00115
Muramatsu, Y., Doi, T., Tomaru, H., Fehn, U., Takeuchi, R., and Matsumoto, R. (2007). Halogen concentrations in pore waters and sediments of the Nankai Trough, Japan: implications for the origin of gas hydrates. Appl. Geochem. 22 (3), 534–556. doi:10.1016/j.apgeochem.2006.12.015
Newberry, C. J., Webster, G., Cragg, B. A., Parkes, R. J., Weightman, A. J., and Fry, J. C. (2004). Diversity of prokaryotes and methanogenesis in deep subsurface sediments from the Nankai Trough, Ocean Drilling Program Leg 190. Environ. Microbiol. 6 (3), 274–287. doi:10.1111/j.1462-2920.2004.00568.x
Niewöhner, C., Hensen, C., Kasten, S., Zabel, M., and Schulz, H. (1998). Deep sulfate reduction completely mediated by anaerobic methane oxidation in sediments of the upwelling area off Namibia. Geochimica Cosmochimica Acta 62 (3), 455–464.
Park, K.-P., Bahk, J.-J., Kwon, Y., Kim, G.-Y., Riedel, M., Holland, M., et al. (2008). Korean national Program expedition confirms rich gas hydrate deposit in the Ulleung Basin, East Sea. Fire Ice Methane Hydrate Newsl. 8 (2), 6–9.
Paull, C. K., and Matsumoto, R. (2000). 1. Leg 164 overview. Proc. ocean Drill. program. Sci. Results 164, 3–10. doi:10.2973/odp.proc.sr.164.204.2000
Paull, C., Matsumoto, R., Wallace, P., Black, N., Borowski, W., Collett, T., et al. (1996). “Proceedings of the Ocean Drilling Program,”. Initial Reports 164, 241–275.
Pogodaeva, T. V., Poort, J., Aloisi, G., Bataillard, L., Makarov, M. M., Khabuev, A. V., et al. (2020). Fluid migrations at the Krasny Yar methane seep of Lake Baikal according to geochemical data. J. Gt. Lakes. Res. 46 (1), 123–131. doi:10.1016/j.jglr.2019.08.003
Raiswell, R. (1988). Chemical model for the origin of minor limestone-shale cycles by anaerobic methane oxidation. Geol 16 (7), 641–644. doi:10.1130/0091-7613(1988)016<0641:cmftoo>2.3.co;2
Reay, D. S., Smith, P., Christensen, T. R., James, R. H., and Clark, H. (2018). Methane and Global Environmental Change. Annu. Rev. Environ. Resour. 43 (1), 165–192. doi:10.1146/annurev-environ-102017-030154
Rodriguez, N., Paull, C., and Borowski, W. (2000). 30. Zonation of authigenic carbonates within gas hydrate-bearing sedimentary sections on the Blake Ridge: offshore southeastern north America. Proc. Ocean Drill. Program, Sci. Results 164, 30. doi:10.2973/odp.proc.sr.164.227.2000
Schulz, H. D. (2006). “Quantification of early diagenesis: Dissolved constituents in pore water and signals in the solid phase,” in Marine Geochemistry. Editors H. D. Schulz, and M. Zabel (Berlin,Germany: Springer), 73–124.
Song, H.-B., Wu, S.-G., and Jiang, W.-W. (2007). The Characteristics of BSRs and Their Derived Heat Flow on the Profile 973 in the Northeastern South China Sea. Chin. J. Geophys. 50 (5), 1321–1331. doi:10.1002/cjg2.1151
Teichert, B. M., Gussone, N., and Torres, M. E. (2009). Controls on calcium isotope fractionation in sedimentary porewaters. Earth Planet. Sci. Lett. 279 (3), 373–382. doi:10.1016/j.epsl.2009.01.011
Tong, H., Feng, D., Cheng, H., Yang, S., Wang, H., Min, A. G., et al. (2013). Authigenic carbonates from seeps on the northern continental slope of the South China Sea: new insights into fluid sources and geochronology. Mar. Petroleum Geol. 43, 260–271. doi:10.1016/j.marpetgeo.2013.01.011
Torres, M., Wallmann, K., Tréhu, A., Bohrmann, G., Borowski, W., and Tomaru, H. (2004). Gas hydrate growth, methane transport, and chloride enrichment at the southern summit of Hydrate Ridge, Cascadia margin off Oregon. Earth Planet. Sci. Lett. 226 (1), 225–241. doi:10.1016/j.epsl.2004.07.029
Wallmann, K., Aloisi, G., Haeckel, M., Obzhirov, A., Pavlova, G., and Tishchenko, P. (2006). Kinetics of organic matter degradation, microbial methane generation, and gas hydrate formation in anoxic marine sediments. Geochimica Cosmochimica Acta 70 (15), 3905–3927. doi:10.1016/j.gca.2006.06.003
Wallmann, K. J., Dallimore, S., Biastoch, A., Westbrook, G., Shakova, N., Severinghaus, J., et al. (2014). Assessment of the Sensitivity and Response of Methane Gas Hydrate to Global Climate Change. Nairobi, Kenya: UNEP.
Wang, L., Sun, X., Shen, S., Wu, P., Liu, T., Liu, W., et al. (2021). Undrained triaxial tests on water-saturated methane hydrate-bearing clayey-silty sediments of the South China Sea. Can. Geotech. J. 58 (3), 351–366. doi:10.1139/cgj-2019-0711
Wang, P., Li, Q., Tian, J., Jian, Z., Liu, C., Li, L., et al. (2013). Long-Term Cycles in the Carbon Reservoir of the Quaternary Ocean: A Perspective From the South China Sea. Nat. Sci. Rev. 1 (1), 119–143. doi:10.1093/nsr/nwt028
Wang, X., Wu, S., Lee, M., Guo, Y., Yang, S., and Liang, J. (2011). Gas hydrate saturation from acoustic impedance and resistivity logs in the Shenhu area, South China Sea. Mar. Petroleum Geol. 28 (9), 1625–1633. doi:10.1016/j.marpetgeo.2011.07.002
Wu, L., Yang, S., Liang, J., Su, X., Fu, S., Sha, Z., et al. (2013). Variations of pore water sulfate gradients in sediments as indicator for underlying gas hydrate in Shenhu Area, the South China Sea. Sci. China Earth Sci. 56 (4), 530–540. doi:10.1007/s11430-012-4545-6
Wu, N., Zhang, H., Yang, S., Zhang, G., Liang, J., Lu, J. a., et al. (2011). Gas hydrate system of Shenhu area, northern South China Sea: geochemical results. J. Geol. Res. 2011, 370298. doi:10.1155/2011/370298
Wu, S., Zhang, G., Guo, C., Huang, Y., and Zhong, S. (2004). Geological constraint on the distribution of gas hydrate in the Dongsha continental slope of South China Sea. Acta Pet. Sin. 25, 7–12. doi:10.7623/syxb200404002
Yang, S., Ming, Z., Liang, J., Lu, J., Zijiang, Z., Holland, M., et al. (2015). Preliminary results of China’s third gas hydrate drilling expedition: A critical step from discovery to development in the South China Sea. Fire Ice 15 (2), 1–5.
Yang, S. X., Liang, J. Q., and Lei, Y. (2017). GMGS4 gas hydrate drilling expedition in the South China Sea. Fire Ice 17 (1), 7–11.
Yang, T., Jiang, S., Ge, L., Yang, J., Wu, N., Zhang, G., et al. (2010). Geochemical characteristics of pore water in shallow sediments from Shenhu area of South China Sea and their significance for gas hydrate occurrence. Chin. Sci. Bull. 55 (8), 752–760. doi:10.1007/s11434-009-0312-2
Yao, B. (2001). The gas hydrate in the South China Sea. J. Trop. Oceanogr. 20 (2), 20–28. doi:10.3969/j.issn.1009-5470.2001.02.004
Ye, H., Yang, T., Zhu, G., Jiang, S., and Wu, L. (2016). Pore water geochemistry in shallow sediments from the northeastern continental slope of the South China sea. Mar. Petroleum Geol. 75, 68–82. doi:10.1016/j.marpetgeo.2016.03.010
Ye, J., Wei, J., Liang, J., Lu, J., Lu, H., and Zhang, W. (2019). Complex gas hydrate system in a gas chimney, South China Sea. Mar. Petroleum Geol. 104, 29–39. doi:10.1016/j.marpetgeo.2019.03.023
Zabel, M., and Schulz, H. D. (2001). Importance of submarine landslides for non-steady state conditions in pore water systems—lower Zaire (Congo) deep-sea fan. Mar. Geol. 176 (1), 87–99. doi:10.1016/s0025-3227(01)00164-5
Zhang, G.-x., Huang, Y.-y., Zhu, Y.-h., and Wu, B.-h. (2002). Prospect of gas hydrate resources in the South China Sea. Mar. Geol. Quat. Geol. 22 (1), 75–82. doi:10.3724/SP.J.1140.2013.02097
Zhang, G., Chen, F., Yang, S., Su, X., Sha, Z., Wang, H., et al. (2012). Accumulation and exploration of gas hydrate in deep-sea sediments of northern South China Sea. Chin. J. Ocean. Limnol. 30, 876–888. doi:10.1007/s00343-012-1313-6
Zhang, G., Liang, J., Lu, J. a., Yang, S., Zhang, M., Holland, M., et al. (2015). Geological features, controlling factors and potential prospects of the gas hydrate occurrence in the east part of the Pearl River Mouth Basin, South China Sea. Mar. Petroleum Geol. 67, 356–367. doi:10.1016/j.marpetgeo.2015.05.021
Keywords: Pore water, gas hydrate, geochemistry, South China Sea, sulfate, calcium, magnesium, iodide
Citation: Ai X, Zha R, Lai Y, Yang T and Su P (2022) Pore-Water Geochemical Gradients of Sulfate, Calcium, Magnesium, and Iodide Correlated With Underlying Gas Hydrate Potential: A Case Study of the Shenhu Area, South China Sea. Front. Earth Sci. 10:882207. doi: 10.3389/feart.2022.882207
Received: 23 February 2022; Accepted: 16 June 2022;
Published: 14 July 2022.
Edited by:
Zhifeng Wan, Sun Yat-sen University, ChinaReviewed by:
Junxi Feng, Guangzhou Marine Geological Survey, ChinaChao Cao, Third Institute of Oceanography, Ministry of Natural Resources, China
Copyright © 2022 Ai, Zha, Lai, Yang and Su. This is an open-access article distributed under the terms of the Creative Commons Attribution License (CC BY). The use, distribution or reproduction in other forums is permitted, provided the original author(s) and the copyright owner(s) are credited and that the original publication in this journal is cited, in accordance with accepted academic practice. No use, distribution or reproduction is permitted which does not comply with these terms.
*Correspondence: Tao Yang, eWFuZ3Rhb0BuanUuZWR1LmNu