- State Key Laboratory of Continental Dynamics, Department of Geology, Northwest University, Xi’an, China
The mechanical properties of the slip zone soil play an important role in the evolution of the loess landslides. To further understand these characteristics, a series of ring shear tests was conducted on the slip zone soils obtained from Tianshuigou landslide, to investigate the influence of moisture content, dry density, shear rate and shear method on the mechanical characteristics of slip zone soils. The experimental results showed that: an increase in the moisture content of the slip zone loess causes a significant reduction in the residual strength. Specially, both the residual cohesion and residual internal friction angle show a deceasing tendency with moisture content. The change in the residual cohesion is more sensitive to the variation in moisture content. Additionally, a trend that strength increased with the increasing of dry density was observed, and the influence degree of dry density on the increased strength is more pronounced at low moisture contents. Thirdly, shear strength shows a negative relationship with shear rate when the shear rate ranges from 0.01 mm/min and 1 mm/min. When the shear rate increased up to 10 mm/min, a stepped shear band is developed and the strength increased. In addition, the strain-softening phenomenon was observed in the single-stage shear tests, which was not noticed in the multi-stage shear tests and pre-shear tests. The residual strength obtained in pre-shear test and multi-stage shear test is slightly greater than that in the single-stage shear test. The experimental results herein can provide an important basis for analyzing the evolution mechanism and prevention of loess landslides.
Introduction
Loess landslides are widely distributed in northwestern of China. Most loess landslides can trigger catastrophic results (Derbyshire et al., 1994; Wang et al., 2020), even causing serious casualties and road damage (Xu et al., 2012; Pei et al., 2017; Leng et al., 2018). It has been found that the evolution mechanism of a landslide is closely related to the stress state and strength of slip zone soil (Zou et al., 2020). Therefore, understanding the mechanical behavior of slip zone soils is of great significance to study the formation mechanism of landslides and the landslide disaster mitigation (Wen and Aydin, 2003; Chen et al., 2014; Sassa et al., 2014; Zhang et al., 2018).
It is widely known that shear strength parameters can be determined by conducting direct shear test, triaxial compression test and ring shear test (Okada et al., 1998; Nam et al., 2011). Direct shear test and triaxial compression test are efficient ways determining the strength parameters, while the parameters measured cannot completely agree with that determined at site because the shear displacement is small, which cannot simulate the sliding movements of landslides which are characterized by a long-run out distance. However, ring shear test has been identified as a good method for determining strength parameter due to the fact that the specimen is subjected to a continuous shear deformation without changing the shear area in the ring shear test (Tan et al., 1998; Sadrekarimi and Olson 2009), which is capable of simulating landslides with a great shear deformation (Bishop et al., 1971; Lupini et al., 1981; Tika and Hutchinson 1999; Meehan et al., 2007; Sugimoto et al., 2009). Especially, residual strength of soil is often measured by ring shear apparatus due to its advantages mentioned above (Skempton. 1985; Bishop et al., 1971).
Many scholars have paid great attention to the mechanical behavior of slip zone soils in recent years (Vithana et al., 2012; Zou et al., 2020; Xu et al., 2021). For example, by using the Bromhead-type ring shear apparatus, Dai et al. (1998) concluded that there is an obvious nonlinear relationship between residual strength and effective normal stress. Furthermore, Tika and Hutchinson (1999) conducted ring shear tests on the slip zone soil obtained from Vaiont landslide and pointed out that the soil sample shows a negative rate effect as the shear rate increases. Tiwari and Marui, (2004) carried out ring shear tests with different shear methods, including single-stage shear test, multi-stage ring shear test with increasing load, multi-stage ring shear test with decreasing load. The results revealed that three test methods all showed similar effective residual internal friction angles, while the effective residual shear intercept is different. Lian et al. (2019) conducted ring shear tests on the soil collected from a loess landslide and found that the increase of moisture content causes the color of the scratches on the shear surface to change from the original color yellow to brown, and the thickness of the shear band decreases as well. Zhang et al. (2015) proposed the reactivation mechanism of landslides after carrying out ring shear tests on the soil collected from a giant landslide in southwestern of China. Based on the high-speed ring shear test, Hu et al. (2015) explored the cause of the giant Yigong landslide and reported that the pore water pressure could not be dissipated in time during the high-speed shear process in which the shear surface was liquefied. In general, there are many factors that affect the shear strength of slip zone soil, including normal stress (Dai et al., 1998; Kimura et al., 2015), moisture content (Derbyshire et al., 1994; Wang et al., 2007; Wang et al., 2021), dry density (Liu et al., 2020; Xu et al., 2020), shear rate (Lemos et al., 1985; Duong et al., 2018; Lian et al., 2020), and shear method (Tiwari and Marui, 2004).
The effect of shear rate on residual strength is complicated (Sugimoto et al., 2009). Previous studies have revealed that the residual strength of soil is various at different shear rates. Lemos et al. (1985) found that the residual strength of the soil with high clay content increased with the increase of the shear rate, while the residual strength of the soil with low clay content was the opposite. Bhat et al. (2013) concluded that the residual strength of the mineral slip surface may vary with the shear rate. The residual strength of kaolin decreases as the shear rate increases from 0.073 mm/min to 0.162 mm/min. When the shear rate is increased from 0.233 mm/min to 0.586 mm/min, the change in residual strength is negligible. However, except for a few studies (Wang et al., 2015; Lian et al., 2020), researchers have not widely investigated the residual strength of slip zone loess at different shear rate. In addition, few studies have been conducted on the residual strength of slip zone loess under different shear methods. Therefore, it is necessary to perform ring shear tests on loess at various shear rates. With this background, to have a deeper understanding in the influence of different factors on the shear strength of slip zone soil of loess landslide, slip zone soils obtained from the Tianshuigou loess landslide were used to conduct ring shear tests. A series of ring shear tests were carried out on slip zone soils to explore the effect of factors (moisture content, dry density, shear rate and shear method) on the shear characteristics of loess. In addition, the development and change law of the strength parameter index were analyzed and compared. This study would provide a reference for a deep insight into the mechanical properties of slip zone soils, the occurrence mechanism of landslides and the prevention and mitigation of landslides.
Background of the Study Site
The Tianshuigou loess landslide is located at Tianshuigou village (37°31′12.31″N, 110°15′20.27″E), Suide county, Shaanxi province, China (Figure 1), which occurred in 2010 and 2015, destroying 20 cave dwellings without causing casualties. The landslide, on the north side of a national highway, directly threatened 54 households with 168 people (including 48 households with 137 permanent residents), 118 cave dwellings and 60 rooms in the south of the slope.
The landslide site is characterized by a concave topography. The arc-shaped landslide had a volume of approximately 1.24 × 105 m3 (71 m long, 350 m wide and 5 m thick), and a travel distance of about 7 m. The elevation at the foot of the landslide is about 835 m asl (above the sea level).
Field investigation revealed that the landslide is located at the loess hilly and gully area (Figure 2A). Landslide scarps, with a height of 3–4 m, are visible on the back edge of the landslide (Figure 2B). The back wall of the landslide is in the shape of a circle chair. Gullies and sinkholes with varying sizes were observed on the slope (Figure 2C). Clearly, the vertical joints are well-developed (Figure 2D) and the slope toe on the front edge of the landslide is steep (Figure 2E), threatening the safety of the local residents. According to the field investigation, the study region exposes quaternary stratum, the main profile (Figure 2F) shows a typical loess layer with different sedimentary history. According to the lithological characteristics, the strata from new to old can be divided into the Holocene slope deposit (Q4del), the Upper Pleistocene wind-deposited loess (Q3eol) and the Middle Pleistocene wind-deposited loess (Q2eol), respectively. Among them, the overall sedimentary thickness of the Middle Pleistocene wind-deposited loess layer is greater compared to that of the Holocene slope deposit loess layer and the Upper Pleistocene wind-deposited loess layer. It is found that the sliding mass belongs to the Holocene slope deposit. Additionally, the slip zone is clearly exposed on the crown scarp and also found in boreholes, with the buried depth of approximately 2–6 m. The slip zone soils consist mainly of light reddish yellow silt soils with calcareous nodules. The structure of the slip zone soil is clumpy, penetrated by plant roots and humus.
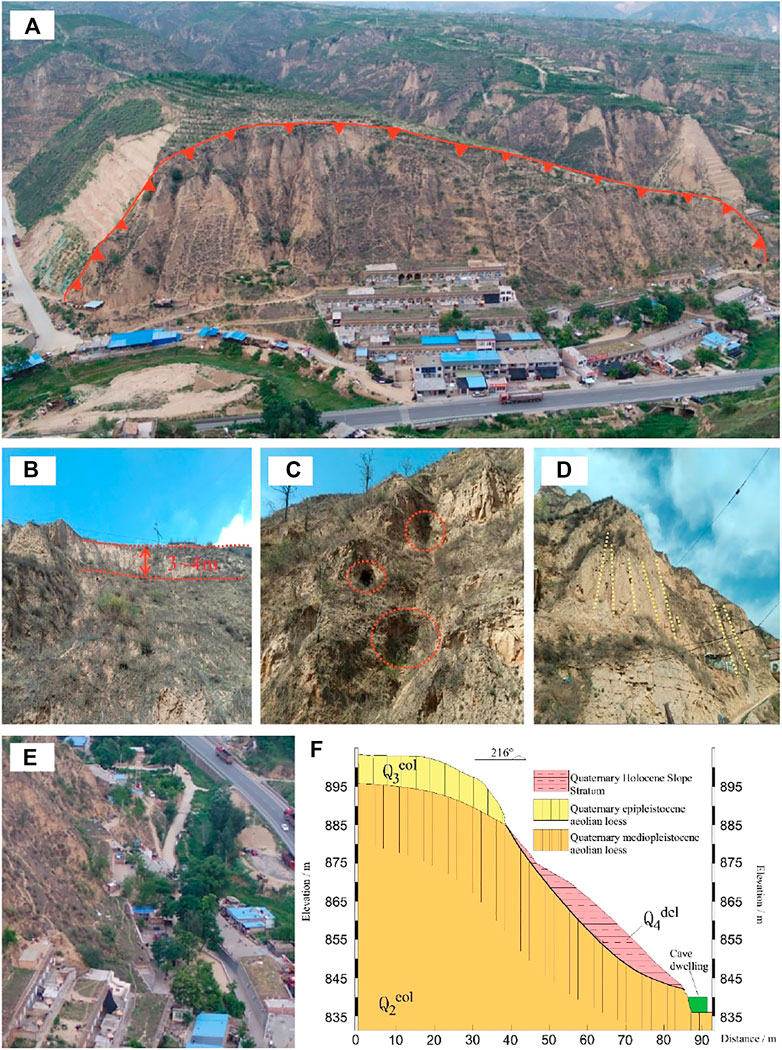
FIGURE 2. Filed investigation on Tianshuigou landslide. (A) Landslide boundary; (B) Scarps on the back edge of the landslide; (C) Slope gullies and sinkholes; (D) Well-developed vertical joints; (E) Steep toe at the front of the landslide; (F) Main profile of landslide showing stratums.
Materials and Methods
Sample Material and Preparation
The slip zone samples that have been identified as Middle Pleistocene silt, were collected from the artificial exploration wells (Figure 3). The sampling depth is about 6 m below ground surface. The samples were carefully cut to column shape with diameter of 15 cm and height of about 30 cm, and then wrapped carefully with membrane to reduce sample disturbance during transport to the laboratory.
The loess samples retrieved from the field were firstly air-dried at a temperature of 105 °C for more than 24 h (Yuan et al., 2019; Hong et al., 2021). According to the quality of the samples before and after drying, the natural moisture content of the sliding zone soil was calculated to be 12%. The soil samples were then passed through a 2 mm sieve. After that, the physical parameters such as dry density, plastic limit and liquid limit were determined using sieved soil samples according to the Chinese National Standards (CNS) GB/T50123-2019. The physical index of slip zone soils is shown in Table 1. Additionally, the particle size distribution of soils was determined by using a laser particle size analyzer with a dispersant and was plotted in Figure 4. Laser particle size analysis demonstrated that the slip zone loess is mainly consist of silt particles (between 0.005 and 0.075 mm), with the percentage of about 70.59%, while the sand content (> 0.075 um) accounts for 19.80%. The soil sample of the slip zone is classified as sandy silt according to the Chinese National Standards (CNS) GB 5007-2011. This type of loess has strong water-sensitive properties, and its mechanical strength will be drastically reduced when it encounters water, which often leads to the occurrence of landslides (Leng et al., 2018).
Prior to testing, deionized water was mixed with the sieved soil samples until the target moisture contents was obtained. Next, the soil samples were placed in cling film bags and then were kept in a sealed container for more than 24 h to hydrate (Zhang and Wang, 2018).
Testing Apparatus
The SRS-150 ring shear apparatus manufactured by the American GCTS company was used and shown in Figure 5. The apparatus mainly consists of three modules: main ring shear cell, including axial force and torque actuators; PCP-15U panel for implementation of axis-translation technique; and the data acquisition and transmission system, with data software for real-time processing of the stress and the shear displacements. For the shearing cell, it is mainly composed of a lower shear box and an upper shear box. The apparatus is capable of shearing the soil specimen with a large displacement without change the shear area, thus the residual strength of the soil can be determined with a good accuracy (Lian et al., 2019).
The torsional shear test principle is shown in Figure 6. Prior to shearing, the axial load is applied by the pneumatic actuator via the upper shear platen, as shown in Figure 6. During the shearing process, the upper platen is rotated, while the bottom platen is kept still. The specimen is rotated by applying a torque T via the upper shear disk, after which the specimen would undergo circular shearing upon the application of the torque. During this process, it is assumed that the shear stress is uniformly distributed along the shear plane. The relationship can be expressed as (Trandafir and Sassa, 2004):
Where T is the torque; F is the axial load; R1 is the inner diameter of the ring shear disc; R2 is the outer diameter of the ring shear disc; t is the shear time;
Experimental Scheme
In this study, four group tests were performed on specimens to investigate the effect of moisture content, dry density, shear rate and shear method on the shear characteristics of slip zone soil (Table 2). In this study, 47 sets of ring shear tests were carried out. In addition, the variation law in the strength parameter index with the change in the factor mentioned above was compared and analyzed.
The natural moisture content of the slip zone soil at site is about 12%. According to the physical parameters of the loess (Table 1), the saturated water content is calculated to be about 22.4%. Considering the fact that an increase in the moisture content is prevailed due to the rainfall infiltration, the moisture content of the specimens in this study is set as 12%, 14%, 16%, 18%, and 20% respectively. The natural dry density of slip zone soil is 1.35 g/cm3. The dry density of the deepest slip soil obtained during field sampling (Figure 3) is approximately 1.45 g/cm3. Therefore, the dry density of 1.35 g/cm3, 1.45 g/cm3 and 1.55 g/cm3 was chosen according to the natural dry density in-situ. The shear rate of the loess ring shear test conducted by scholars can reach hundreds of millimeters per minute (Hong et al., 2021; Ma et al., 2021). Referring to previous research results, Duong et al. (2018) chose a shear rate of 0.02 mm/min∼20 mm/min in the process of studying the effect of shear rate on the residual strength of kaolin-bentonite mixtures. To investigate the shear rate level on the mechanical behavior of loess soil, according to instrument conditions the specimens were sheared at rates of 0.01 mm/min, 0.1 mm/min, 1 mm/min, 10 mm/min, 100 mm/min at the normal stress of 100 kPa, 200 and 300 kPa.
One main advantage of the ring shear apparatus is that the residual strength can be obtained through various shear methods (Tiwari and Marui, 2004; Duong and Hai, 2021). In this work, a total of seven samples were sheared by using three different shear methods, including single-stage shear test, multi-stage shear test and pre-shear test. 1) A series of three single-stage shear tests have been firstly carried out on specimens under different normal stresses, samples 1, 2 and 3 has been sheared at rate of 10 mm/min. The single-stage shear tests mainly consist of consolidation stage and shearing stage. In the consolidation stage, specimens were consolidated for about 12 h in the shear box (Lian et al., 2020). In the shearing stage, the specimens were sheared until the shear stress is basically stable. The basically steady state means that the shear stress remains at a roughly stable minimum shear resistance value within a certain shear displacement range (Lian et al., 2021). 2) Multi-stage shear tests have been performed to investigate the influence of the pre-shear history on the value of the residual strength. In multi-stage shear tests, the tests were performed by following a multi-stage scheme in which residual strength assessments were made at one or more normal stress value: 50 kPa, 100kPa, 200kPa or 300 kPa. To be more specific, a low normal stress (i.e., 50 kPa) was firstly applied to allow the consolidation of the specimen and then the specimen was sheared until the stable state was achieved. After that, the specimen was subjected to shear until the residual state at this stage was reached under a higher normal stress level (i.e., 100kPa). In this work, the specimen was sheared at four stresses level to obtain the residual strength value of specimens under at four stages. 3) In pre-shear tests, the specimen was firstly sheared at a faster rate until a shear surface was formed. After that, the specimen was subjected to shear at a lower shear rate until the residual state was achieved.
Results
Moisture Content Effect on Residual Strength
The typical stress-shear displacement curves of loess specimens with different moisture contents are shown in Figure 7. It can be seen that a sharp increase in shear strength was observed before the peak strength after which shear resistance decrease gradually, and the residual state was achieved at a large shear displacement. The test results revealed that the residual strength of the slip zone soil decreases with the increase of the moisture content. Taking the sample at normal stress of 300 kPa as an example, the residual strength of the specimen fell from 237.8 kPa at the moisture content of 12%–198.9 kPa at the moisture content of 20%, decreasing by roughly 16.4%.
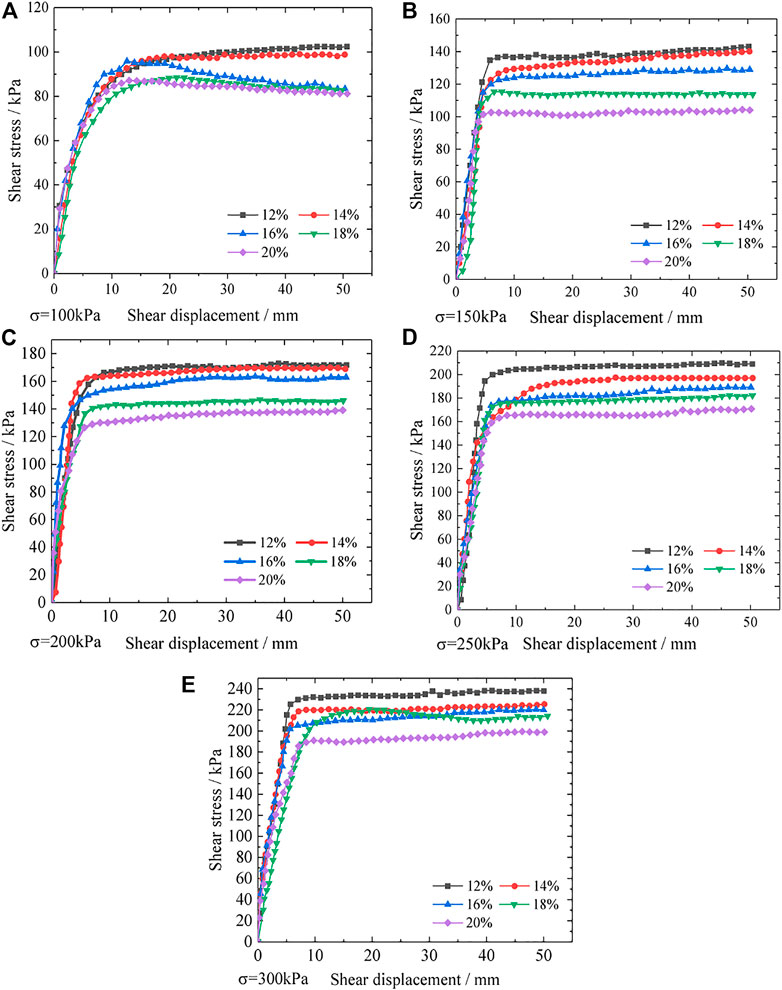
FIGURE 7. The relationship curve of shear stress-shear displacement of sample with moisture content. (A) Normal stress is 100 kPa (B) Normal stress is 150 kPa; (C) Normal stress is 200 kPa; (D) Normal stress is 250 kPa; (E) Normal stress is 300 kPa.
The residual strength parameters including the residual cohesion and residual internal friction angle were determined by using the Mohr-Coulomb shear strength criterion (Labuz and Zang, 2012) according to the changes in residual strength of specimens with different moisture contents (Figure 8) and the shear parameters are listed in Table 3. Clearly, the residual cohesion decreases greatly as the moisture content increases from 12% to 20%. Specially, the residual cohesion of the specimen decreases from 40.88 kPa with moisture content of 12%–17.94 kPa at the moisture content of 20%, decreasing by about 56.12%. Additionally, a decreasing tendency of the residual internal friction angle with moisture content was observed (Table 3). The residual internal friction angle decreases by 7.1% as the moisture content increases from 12% to 20%.
Dry Density Effect on Residual Strength
The shear stress-shear displacement curves of specimens with different dry densities are shown in Figure 9. The results showed that a significant increase in the shear strength was observed when the dry density increases. For example, when the moisture content is constant (12%), the residual strength increases from 171.9 kPa at the dry density of 1.35 g/cm3 to 200.0 kPa at the dry density of 1.55 g/cm3. Furthermore, the effect of dry density on the shear displacement at which the residual state of loess soil was achieved is obvious.
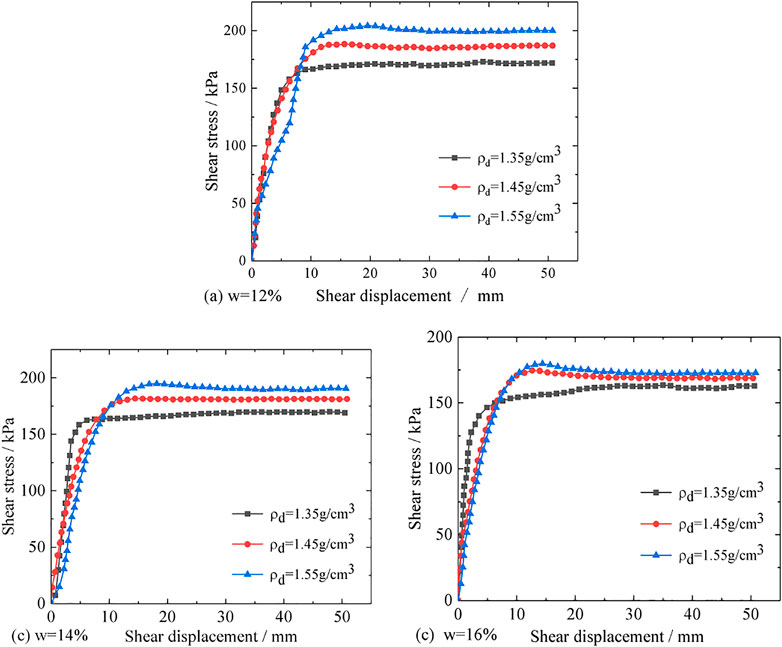
FIGURE 9. Shear stress-shear displacement curve of specimens under different dry densities. (A) Moisture content is 12%; (B) Moisture content is 14%; (C) Moisture content is 16%.
Take the sample with 12% moisture content as an example (Figure 9A), the corresponding shear displacement at residual state is about 22 mm with the dry density of 1.35 g/cm3, however, the residual strength of the specimens with the dry density of 1.45 g/cm3 and 1.55 g/cm3 were achieved at the shear displacement of approximately 25 mm and 31 mm, respectively.
Shear Rate Effect on Residual Strength
Figure 10 presents shear stress against shear displacement at different shear rates. To facilitate a clear view of the variation in the residual strength with shear rate and normal stress, Figure 11A shows the residual strength envelope surface in the three-dimensional space of normal stress-residual strength-shear rate. It can be seen that the three-dimensional strength envelope plotted in Figure 11A is not continuous and flat, indicating that the turning point of shear rate can be obtained at which the trend of the change in residual strength with shear rate changes. To quantitatively analyze the change trend of residual strength with shear rate and normal stress, Figure 11B plots the residual strength against shear rate at the normal stress of 100 kPa, 200kPa, and 300kPa. Obviously, the residual strength decreases as shear rate ranges from 0.01 mm/min to 1 mm/min and from 10 mm/min to 100/min at all normal stress levels, while that increases as shear rate ranges between 1 mm/min and 10 mm/min. The experimental results herein show the decreasing tendency of residual strength with increasing shear rate from 0.01 mm/min to 1 mm/min (speed weakening characteristics (Kimura et al., 2014), i.e., residual strength decreases with increasing shear rate), which is agree well with the conclusion reported by Tika and Hutchinson, (1999) that a negative rate effect on the residual strength was observed when shear rate is within this range.
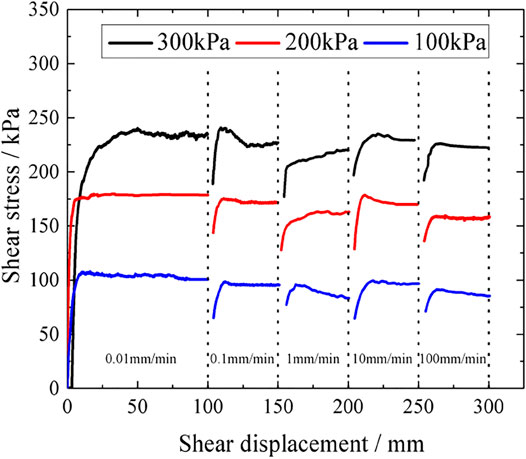
FIGURE 10. The relationship curve of shear stress-shear displacement of specimen under different shear rates.
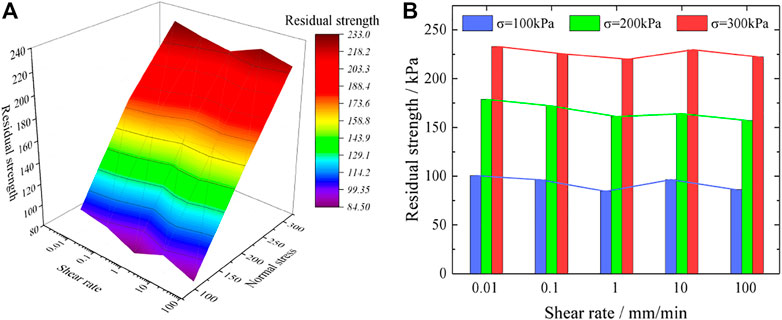
FIGURE 11. The relationship between residual strength and shear rate. (A) Plot of residual strength versus shear rate and normal stress; (B) Plot of residual strength and shear rate.
Shear Methods Effect on Residual Strength
Figure 12 shows shear stress-displacement curves under three different shear methods, respectively. It can be seen from Figure 12A that strain-softening phenomenon was observed when the specimen is sheared in the single-stage shear tests.
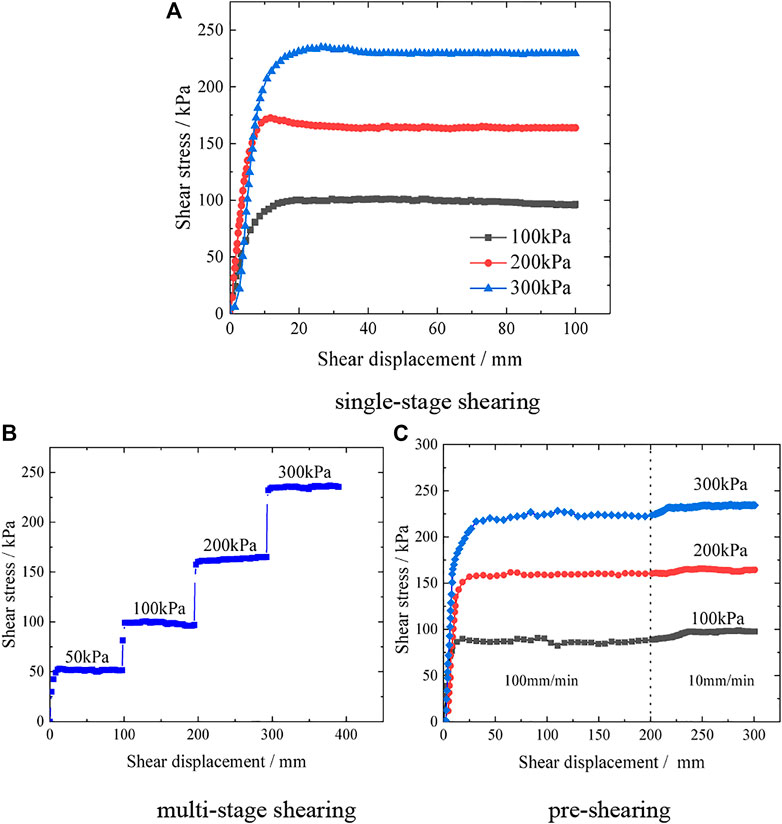
FIGURE 12. Shear stress-shear displacement relation curve under different shear method. (A) single-stage shearing (B) multi-stage shearing (C) pre-shearing.
For example, taking the test results at normal stress of 200 kPa as an example, the peak strength is 172.5 kPa and the residual strength is 163.9 kPa, with the peak-residual strength drop of 8.6 kPa. However, no obvious strain-softening phenomenon was observed in the multi-stage shear tests and pre-shear tests (Figure 12bc). Table 4 shows the residual strength of the slip zone soil in different shear tests. It is clear that the residual strength obtained in multi-stage shear tests and pre-shear tests are slightly greater than that obtained in the single-stage shear tests.
Discussion
State of Soil Effect on the Loess Residual Shear Strength Parameters
Moisture Content
The ring shear test results revealed that the shear strength of loess decreases significantly with the increase of moisture content. Other studies have reported the similar conclusion (Derbyshire et al., 1994; Tian et al., 2021). These research results indicated that when water penetrates into the loess, the residual strength of the slip zone soil would be decreased, which contribute to the slope movements and the slope unstability.
Additionally, it was found that the residual cohesion of loess in the ring shear test decreases with moisture content (Figure 13). This may be explained as follows: the increase of moisture content can thicken the bound water film attached to the surface of the soil particles (Tian et al., 2021). Furthermore, moisture will dissolve the cement and salt between the particles, causing a significant drop in cohesion (Zhang et al., 2013). With the increase of moisture content, the free water content between soil particles increases. The lubricity between particles will be enhanced, and the occlusal friction and sliding friction between particles will be significantly reduced, which causes the residual internal friction angle to be reduced. It is worth noting that with the increase of moisture content, the reduction in the residual cohesion is much greater than the reduction in the residual internal friction angle, implying that residual cohesion is more sensitive to changes of moisture content. In addition, the increased moisture content is an important factor resulting in the softening of loess. In the test, it was also found that when the moisture content of the sample is great, the soil was rotated out of the gap between the shear boxes during the shearing process (Figure 14). Loess is characterized by a strong water sensitivity (Dijkstra et al., 1995; Lian et al., 2020). For a slope formed in a certain geological environment, the soil in the slope has been in a balanced state of the coupling between a certain stress level and groundwater for a long time. Once water enters the slope, it would destroy the original balance system. Therefore, most unstable slopes and landslides are closely related to the action of water (Ma et al., 2019). The two slides of the Tianshuigou landslide in 2010 and 2015 occurred after summer rainfall. According to the test results and the actual situation, it can be concluded that the reduction of loess strength caused by water is the main reason for the Tianshuigou landslide.
Dry Density
The value of the dry density reflects the degree of solidity and compression of the soil, and it is manifested in the microscopic aspect as the size of the voids between soil particles and the degree of compactness of the particles (Liu et al., 2020). The results of this test revealed that the shear strength of the sample increases with the dry density at all moisture contents (Figure 15). The phenomenon may be explained as follows: when the dry density of the sample increases, the pores between particles decrease, which causes the particle contact to be close, the pore ratio decreases, and the cohesion between particles increases. The close contact of the particles will increase the occlusion, and the friction between the particles will also increase (Li, 2018; Xu et al., 2020). For Tianshuigou landslide, the natural dry density of the sliding zone soil is 1.35 g/cm3. The method of improving the residual strength of soil by increasing the dry density under the natural moisture content is very impressive, which provides a way of thinking for prevention of the landslide.
For specimens with lower moisture contents, the effect of dry density on increased shear strength is more obvious (Figure 15). For example, when the dry density increases from 1.35 g/cm3 to 1.55 g/cm3 at the normal pressure of 200 kPa, the increase in the residual strength was decreased from about 29.1 kPa at the moisture content of 12% to 9.9 kPa at the moisture content of 16%. The fitting formula in the figure can describe the relationship between dry density and residual strength. For samples with lower moisture content, the larger the coefficient before dry density in the formula, it means that each unit increase in dry density contributes more to residual strength. The reason is that water in the soil sample mainly exists in the form of strongly bound water at a low moisture content, which causes the soil in relatively stable and immobile state, the shear strength can be improved to a greater extent (Lian et al., 2019). While more free water will appear on the specimen at the higher moisture content, which weaken the connection between soil particles. Thus, the influence of dry density on the increased shear strength is little.
When the shear stress of the sample is reduced from the peak strength to the residual strength, the soil particles on the shear surface are constantly adjusted and positioned along the shear direction under the action of torsion shear force to gradually form a new arrangement. Consequently, the original structure of the soil is destroyed (Sebastian and Luise, 2006). For the samples with a low dry density, the pore space of the soil particles is relatively large during the shearing process under the action of axial pressure, and the adjustment of the particle arrangement is relatively easy. Compared with samples with a lower dry density, the particles at the shear surface of the sample with a higher dry density are dense and the movable space is small, which causes the adjustment of the particles to become more difficult. Therefore, the specimen with a high dry density undergoes a greater shear displacement to reach the residual state (Figure 9).
Influence of Shear Behavior on Shear Strength of Loess
Shear Rate Effect on Loess Residual Shear Strength
In general, soils exhibit different shear resistance with the change of shear rate, which may show negative rate effect, positive rate effect and neutral effect (Hungr and Morgenstern, 1984; Skempton, 1985; Tika and Hutchinson, 1999; Kimura et al., 2015). The results obtained in this study show that the shear rate exhibits a negative rate effect from 0.01 mm/min to 1 mm/min, which is consistent with the conclusion reported by the Tika and Hutchinson, 1999. Additionally, it is widely acknowledged that the shear band structure changes in the ring shear test (Agung et al., 2004; Sadrekarimi and Olson, 2010). In this study, the slip surface of loess is relatively smooth when the shear rate is 1 mm/min, whereas a stepped slip zone which is relatively rough is formed in the slip surface when the rate increased to 10 mm/min. This may be attributed to the difficulty of the pore water pressure dissipation in time under a high shearing, resulting in a lower residual strength. In the laboratory test, it was found that the effect of shear rate on the residual strength of sliding zone soil shows a negative rate effect. when the shear rate is between 0.01 mm/min and 1 mm/min. When the Tianshuigou landslide is in instability or limit equilibrium, if a critical combination of landslide displacement and velocity is induced, the shear strength of the soil will decrease due to the negative rate effect, which will induce landslide reactivate.
When the shear rate increases up to 1 mm/min, the minimum residual strength was achieved. However, as shear rate increases from 1 mm/min to 10 mm/min, the residual strength increases. The change trend of residual strength with shear rate may be explained as follows: the increase in shear rate causes disturbances of the shear surface in a certain range, and the shear surface transitions from a single, smooth shape (Figure 16A) to a stepped shear zone with a certain thickness (Figure 16B), the residual strength is increased. As the shear rate continues to increase to 100 mm/min, the residual strength decreases, which may be attributed to the fact that the built-up pore water pressure cannot be dissipated in time under high-speed shearing and the effective residual strength decreased consequently. Additionally, the test shows that the residual strength is less affected by the shear rate at the higher normal stress level. For example, when the normal pressure is 100 kPa, the difference between the maximum and minimum residual strength of the specimen within the range of the test shear rate accounts for about 16.08%. However, when normal stress increases to 200 and 300 kPa, the difference between the maximum and minimum residual strength is roughly 12.08% and 5.58%, respectively.
Shear Method Effect on the Loess Residual Shear Strength Parameters
The residual strength obtained by different shearing methods is different (Tiwari and Marui, 2004). The test results show that the residual strength obtained by pre-shearing and multi-stage shearing are slightly larger than those obtained by single-stage shearing. Similar laws were also found in the slip zone soil of the Three Gorges Huangtupo landslide (Wang et al., 2012). The residual strength obtained by the single-stage shearing can be understood as the residual strength of the slip zone soil during the one-time sliding of the landslide. The residual strength obtained by pre-shearing is the residual strength of the slip zone soil when the sliding surface has been formed and slides again after a period of time. The residual strength obtained by multi-stage shearing can be understood as the residual strength of slip zone soil in the process of step-by-step penetration and sliding over time. In the early stage of the pre-shear test, the high-speed ring shear disturbed the soil particles near the shear surface greatly, which increased the thickness of the shear band, and therefore the shear strength was improved to a certain extent. In the multi-stage shear test, the slip zone soil was consolidated and sheared step by step for many times, which increased the compactness of the soil and the effective stress. In addition, it takes a relatively long time to complete a set of shear tests, and the moisture content of the sample may be reduced. Therefore, the residual strength of the soil sample under the multi-stage shear method is relatively increased. Although the single-stage shear test is more time-consuming than the other two shear methods, it is less affected by other factors and the error is relatively small.
The strain-softening phenomenon was observed when the specimen was sheared at single-stage shear method. The directional arrangement of soil particles during the shearing process is the cause of strain softening. In the multi-stage shearing and pre-shear tests, the previous shearing makes the soil particles of the shear zone basically form a directional arrangement, thus no obvious strain softening phenomenon was observed in the pre-shear tests and multi-stage shear tests.
Limitation
It is well known that the loess soil in different areas of the Loess Plateau is characterized by various geotechnical properties (Zhang and Wang, 2018). A series of ring shear tests was conducted on loess soil obtained from a loess landslide that recently occurred on the Loess Plateau in northern Shaanxi. Therefore, our experiment has certain regional limitations. In addition, the stability of slopes is significantly affected by pore water pressure generation and dissipation (Carey et al., 2017; Askarinejad et al., 2018). However, due to the fact that the equipment used in current study is not capable of recording real-time pore water pressure generated, the further investigation in the mechanical behavior of slip zone soils with a advanced apparatus which is capable of obtaining the accurate parameters such as pore water pressure is needed. Due to the large workload of the designed test, the parallel test of the ring shear test is not set up. In this experiment, it was concluded that the cohesion of the soil in the Tianshuigou landslide zone was higher at low water content, which may be related to the fine-grained composition of the soil. In view of this situation, we intend to use the X-ray diffraction method to study it in the follow-up research.
Conclusion
A series of drainage ring shear tests were conducted on distributed loess samples collected from a loess landslide occurred on the Loess Plateau in northern Shaanxi, China. The influence of moisture content, dry density, shear rate and shear method on the residual strength was explored. The following conclusions are obtained:
(1) The increase in moisture content causes a significant reduction in the residual strength of slip zone loess. In terms of strength parameters, the residual cohesion and residual internal friction angle decrease with the increase of moisture content. It is worth noting that the reduction in residual cohesion is much greater than that in residual internal friction angle, indicating that residual cohesion is more sensitive to changes in moisture content.
(2) Under the same conditions, the residual strength of slip zone soil increases with dry density, and the increase in the residual strength with dry density is more obvious at a lower moisture content.
(3) The residual strength shows a negative rate effect with the shear rate ranging from 0.01 mm/min to 1 mm/min. When the shear rate increased to 10 mm/min, a stepped shear band was observed and the residual strength increased. When the shear rate ranges from 10 mm/min to 100 mm/min, the decreasing tendency in residual strength was found.
(4) The residual strength obtained in the pre-shear tests and the multi-stage shear tests is slightly greater than that obtained in the single-stage shear tests. In addition, the strain-softening phenomenon was observed in single-stage shear tests, while the strain softening phenomenon was not obvious in the multi-stage shear tests and pre-shear tests.
Data Availability Statement
The original contributions presented in the study are included in the article/Supplementary Material, further inquiries can be directed to the corresponding author.
Author Contributions
KL and XW designed the experiments, analyzed data and wrote the whole paper; BL guided the writing of the paper and revised the paper; ZZ and CX designed the experiments and helped to select the experimental area. All authors have read and agreed to the published version of the manuscript.
Funding
The National Natural Science Foundation of China (No. 41902268), Nature Science Basic Research Plan in Shaanxi Province of China (No. 2022JQ-253) and China Postdoctoral Science Foundation (No. 2021M702648) are gratefully acknowledged.
Conflict of Interest
The authors declare that the research was conducted in the absence of any commercial or financial relationships that could be construed as a potential conflict of interest.
Publisher’s Note
All claims expressed in this article are solely those of the authors and do not necessarily represent those of their affiliated organizations, or those of the publisher, the editors, and the reviewers. Any product that may be evaluated in this article, or claim that may be made by its manufacturer, is not guaranteed or endorsed by the publisher.
References
Agung, M. W., Sassa, K., Fukuoka, H., and Wang, G. (2004). Evolution of Shear-Zone Structure in Undrained Ring-Shear Tests. Landslides 1 (2), 101–112. doi:10.1007/s10346-004-0001-9
Askarinejad, A., Akca, D., and Springman, S. M. (2018). Precursors of Instability in a Natural Slope Due to Rainfall: a Full-Scale experiment. Landslides 15 (9), 1745–1759. doi:10.1007/s10346-018-0994-0
Bhat, D. R., Bhandary, N. P., and Yatabe, R. (2013). Effect of Shearing Rate on Residual Strength of Kaolin Clay. Electron. J. Geotechnical Eng. 18, 387–1396.
Bishop, A. W., Green, G. E., Garga, V. K., Andresen, A., and Brown, J. D. (1971). A New Ring Shear Apparatus and its Application to the Measurement of Residual Strength. Géotechnique 21 (4), 273–328. doi:10.1680/geot.1971.21.4.273
Carey, J. M., McSaveney, M. J., and Petley, D. N. (2017). Dynamic Liquefaction of Shear Zones in Intact Loess during Simulated Earthquake Loading. Landslides 14 (3), 789–804. doi:10.1007/s10346-016-0746-y
Chen, J., Dai, F., Xu, L., Chen, S., Wang, P., Long, W., et al. (2014). Properties and Microstructure of a Natural Slip Zone in Loose Deposits of Red Beds, Southwestern China. Eng. Geology. 183, 53–64. doi:10.1016/j.enggeo.2014.10.004
Dai, F. C., Wang, S. J., and Li, C. F. (1998). The Drained Residual Strength of Volcanics-Derived Soil Sampled on Lantau Island, Hong Kong. J. Eng. Geology. 6 (3), 223–229. (in Chinese).
Derbyshire, E., Dijkstra, T. A., Smalley, I. J., and Li, Y. (1994). Failure Mechanisms in Loess and the Effects of Moisture Content Changes on Remoulded Strength. Quat. Int. 24, 5–15. doi:10.1016/1040-6182(94)90032-9
Dijkstra, T. A., Smalley, I. J., and Rogers, C. D. F. (1995). Particle Packing in Loess Deposits and the Problem of Structure Collapse and Hydroconsolidation. Eng. Geology. 40 (1-2), 49–64. doi:10.1016/0013-7952(95)00022-4
Duong, N. T., and Hai, N. V. (2021). Residual Strength of Weakly Cemented Kaolin Clay in Multi-Stage Ring Shear Test. Arabian J. Sci. Eng. 2021, 1–15. doi:10.1007/s13369-021-06132-2
Duong, N. T., Suzuki, M., and Van Hai, N. (2018). Rate and Acceleration Effects on Residual Strength of Kaolin and Kaolin-Bentonite Mixtures in Ring Shearing. Soils and foundations 58 (5), 1153–1172. doi:10.1016/j.sandf.2018.05.011
Hong, Y., Ling, X. Z., and He, K. Q. (2021). Effects of Sliding Liquefaction on Homogeneous Loess Landslides in Western China. Scientific Rep. 11 (1), 1–14. doi:10.1038/s41598-021-91411-z
Hu, M.-j., Pan, H.-l., Zhu, C.-q., and Wang, F.-w. (2015). High-speed Ring Shear Tests to Study the Motion and Acceleration Processes of the Yingong Landslide. J. Mt. Sci. 12 (6), 1534–1541. doi:10.1007/s11629-014-3059-4
Hungr, O., and Morgenstern, N. R. (1984). High Velocity Ring Shear Tests on Sand. Géotechnique 34 (3), 415–421.
Kimura, S., Nakamura, S., and Vithana, S. B. (2015). Influence of Effective normal Stress in the Measurement of Fully Softened Strength in Different Origin Landslide Soils. Soil Tillage Res. 145, 47–54. doi:10.1016/j.still.2014.07.018
Kimura, S., Nakamura, S., Vithana, S. B., and Sakai, K. (2014). Shearing Rate Effect on Residual Strength of Landslide Soils in the Slow Rate Range. Landslides 11 (6), 969–979. doi:10.1007/s10346-013-0457-6
Labuz, J. F., and Zang, A. (2012). Mohr-Coulomb Failure Criterion. Rock Mech. Rock Eng. 45 (6), 975–979. doi:10.1007/s00603-012-0281-7
Lemos, L., Skempton, A. W., and Vaughan, P. R. (1985) Earthquake Loading of Shear Surfaces in Slopes. Proceedings of 11th International Conference on Soil Mechanics and Foundation Engineering 4, 1955–1958.
Leng, Y., Peng, J., Wang, Q., Meng, Z., and Huang, W. (2018). A Fluidized Landslide Occurred in the Loess Plateau: A Study on Loess Landslide in South Jingyang Tableland. Eng. Geology. 236, 129–136. doi:10.1016/j.enggeo.2017.05.006
Li, Y. (2018). A Review of Shear and Tensile Strengths of the Malan Loess in China. Eng. Geology. 236, 4–10. doi:10.1016/j.enggeo.2017.02.023
Lian, B., Peng, J., Wang, X., and Huang, Q. (2019). Moisture Content Effect on the Ring Shear Characteristics of Slip Zone Loess at High Shearing Rates. Bull. Eng. Geol. Environ. 79 (2), 999–1008. doi:10.1007/s10064-019-01597-w
Lian, B. Q., Wang, X. G., Liu, K., Hu, S., and Feng, X. (2021). A Mechanical Insight into the Triggering Mechanism of Frequently Occurred Landslides along the Contact between Loess and Red clay. Scientific Rep. 11 (1), 1–15. doi:10.1038/s41598-021-96384-7
Lian, B., Wang, X., Peng, J., and Huang, Q. (2020). Shear Rate Effect on the Residual Strength Characteristics of Saturated Loess in Naturally Drained Ring Shear Tests. Nat. Hazards Earth Syst. Sci. 20 (10), 2843–2856. doi:10.5194/nhess-20-2843-2020
Liu, J., Li, X. A., Xue, Q., and Guo, Z. (2020). Experimental Study on Air Permeability and Microscopic Mechanism of Intact and Remolded Malan Loess, Loess Plateau, China. Bull. Eng. Geol. Environ. 79 (8), 3909–3919. doi:10.1007/s10064-020-01810-1
Lupini, J. F., Skinner, A. E., and Vaughan, P. R. (1981). The Drained Residual Strength of Cohesive Soils. Géotechnique 31 (2), 181–213. doi:10.1680/geot.1981.31.2.181
Ma, J., Zhao, X., Li, S., and Duan, Z. (2021). Effects of High Shearing Rates on the Shear Behavior of Saturated Loess Using Ring Shear Tests. Geofluids 2021, 1–12. doi:10.1155/2021/6527788
Ma, P., Peng, J., Wang, Q., Zhuang, J., and Zhang, F. (2019). The Mechanisms of a Loess Landslide Triggered by Diversion-Based Irrigation: a Case Study of the South Jingyang Platform, China. Bull. Eng. Geol. Environ. 78 (7), 4945–4963. doi:10.1007/s10064-019-01467-5
Meehan, C. L., Brandon, T. L., and Duncan, J. M. (2007). Measuring Drained Residual Strengths in the Bromhead Ring Shear. Geotechnical Test. J. 30 (6), 466–473.
Nam, S., Gutierrez, M., Diplas, P., and Petrie, J. (2011). Determination of the Shear Strength of Unsaturated Soils Using the Multistage Direct Shear Test. Eng. Geology. 122, 272–280. doi:10.1016/j.enggeo.2011.06.003
Okada, Y., Sassa, K., and Fukuoka, H. (1998). Comparison of Shear Behaviour of sandy Soils by Ring-Shear Test with Conventional Shear Tests. Environ. For. Sci. 54, 623–632. doi:10.1007/978-94-011-5324-9_66
Pei, X., Zhang, X., Guo, B., Wang, G., and Zhang, F. (2017). Experimental Case Study of Seismically Induced Loess Liquefaction and Landslide. Eng. Geology. 223, 23–30. doi:10.1016/j.enggeo.2017.03.016
Sadrekarimi, A., and Olson, S. M. (2009). A New Ring Shear Device to Measure the Large Displacement Shearing Behavior of Sands. Geotechnical Test. J. 32 (3), 197–208.
Sadrekarimi, A., and Olson, S. M. (2010). Shear Band Formation Observed in Ring Shear Tests on Sandy Soils. J. Geotech. Geoenviron. Eng. 136 (2), 366–375. doi:10.1061/(asce)gt.1943-5606.0000220
Sassa, K., Dang, K., He, B., Takara, K., Inoue, K., and Nagai, O. (2014). A New High-Stress Undrained Ring-Shear Apparatus and its Application to the 1792 Unzen-Mayuyama Megaslide in Japan. Landslides 11, 827–842. doi:10.1007/s10346-014-0501-1
Sebastian, Lobo-Guerrero., and Luise, V. (2006). Modeling Granular Crushing in Ring Shear Tests: Experimental and Numerical Analyses. Soils and Foundations 46 (2), 147–157.
Skempton, A. W. (1985). Residual Strength of Clays in Landslides, Folded Strata and the Laboratory. Géotechnique 35 (1), 3–18. doi:10.1680/geot.1985.35.1.3
Sugimoto, M., Nakamura, K., Sakai, N., and Toyota, H. (2009). Ring Shear Tests to Evaluate Strength Parameters in Various Remoulded Soils. Géotechnique 59 (8), 649–659.
Tan, S. A., Chew, S. H., and Wong, W. K. (1998). Sand-geotextile Interface Shear Strength by Torsional Ring Shear Tests. Geotextiles and Geomembranes 16 (3), 161–174. doi:10.1016/s0266-1144(98)00007-7
Tian, W.-t., Dong, J.-h., Sun, J.-j., and Yang, B. (2021). Experimental Study on Main Physical Parameters Controlling Shear Strength of Unsaturated Loess. Adv. Civil Eng. 2021, 1–11. doi:10.1155/2021/6652210
Tika, T. E., and Hutchinson, J. N. (1999). Ring Shear Tests on Soil from the Vaiont Landslide Slip Surface. Géotechnique 49 (1), 59–74. doi:10.1680/geot.1999.49.1.59
Tiwari, B., and Marui, H. (2004). Objective Oriented Multistage Ring Shear Test for Shear Strength of Landslide Soil. J. Geotech. Geoenviron. Eng. 130 (2), 217–222. doi:10.1061/(asce)1090-0241(2004)130:2(217)
Trandafir, A. C., and Sassa, K. (2004). Undrained Cyclic Shear Response Evaluation of Sand Based on Undrained Monotonic Ring Shear Tests. Soil Dyn. Earthquake Eng. 24 (11), 781–787. doi:10.1016/j.soildyn.2004.06.017
Vithana, S. B., Nakamura, S., Gibo, S., Yoshinaga, A., and Kimura, S. (2012). Correlation of Large Displacement Drained Shear Strength of Landslide Soils Measured by Direct Shear and Ring Shear Devices. Landslides 9, 305–314. doi:10.1007/s10346-011-0301-9
Wang, G., Sassa, K., Fukuoka, H., and Tada, T. (2007). Experimental Study on the Shearing Behavior of Saturated Silty Soils Based on Ring-Shear Tests. J. Geotech. Geoenviron. Eng. 133 (3), 319–333. doi:10.1061/(asce)1090-0241(2007)133:3(319)
Wang, S., Wu, W., Xiang, W., and Liu, Q. (2015). Shear Behaviors of Saturated Loess in Naturally Drained Ring-Shear Tests. Springer Ser. Geomechanics Geoengineering, 19–27. doi:10.1007/978-3-319-11053-0_3
Wang, S., Xiang, W., Cui, D. S., Yang, J., and Huang, X. (2012). Study of Residual Strength of Slide Zone Soil under Different Ring-Shear Tests. Rock Soil Mech. 33 (10), 2967–2972.
Wang, X. G., Lian, B. Q., Liu, K., and Luo, L. (2021). Trigger Mechanism of Loess-Mudstone Landslides Inferred from Ring Shear Tests and Numerical Simulation. J. Mountain Sci. 18 (09), 2412–2426. doi:10.1007/s11629-021-6791-6
Wang, X., Wang, J., Zhan, H., Li, P., Qiu, H., and Hu, S. (2020). Moisture Content Effect on the Creep Behavior of Loess for the Catastrophic Baqiao Landslide. CATENA 187, 104371. doi:10.1016/j.catena.2019.104371
Wen, B. P., and Aydin, A. (2003). Microstructural Study of a Natural Slip Zone: Quantification and Deformation History. Eng. Geology. 68, 289–317. doi:10.1016/s0013-7952(02)00234-x
Xu, J. B., Wei, W., Bao, H., Zhang, K. K., Lan, H. X., Yan, C. G., et al. (2020). Study on Shear Strength Characteristics of Loess Dam Materials under Saturated Conditions. Environ. Earth Sci. 79 (13), 1–14. doi:10.1007/s12665-020-09089-x
Xu, L., Dai, F. C., Gong, Q. M., Tham, L. G., and Min, H. (2012). Irrigation-induced Loess Flow Failure in Heifangtai Platform, north-west China. Environ. Earth Sci. 66 (6), 1707–1713. doi:10.1007/s12665-011-0950-y
Xu, Q., Wang, W., Li, L., and Cao, Y. (2021). Failure Mechanism of Gently Inclined Shallow Landslides along the Soil-Bedrock Interface on Ring Shear Tests. Bull. Eng. Geol. Environ. 80 (5), 3733–3746. doi:10.1007/s10064-021-02171-z
Yuan, W., Fan, W., Jiang, C., and Peng, X. (2019). Experimental Study on the Shear Behavior of Loess and Paleosol Based on Ring Shear Tests. Eng. Geology. 250, 11–20. doi:10.1016/j.enggeo.2019.01.007
Zhang, F., and Wang, G. (2018). Effect of Irrigation-Induced Densification on the post-failure Behavior of Loess Flowslides Occurring on the Heifangtai Area, Gansu, China. Eng. Geology. 236, 111–118. doi:10.1016/j.enggeo.2017.07.010
Zhang, F., Wang, G., Kamai, T., Chen, W., Zhang, D., and Yang, J. (2013). Undrained Shear Behavior of Loess Saturated with Different Concentrations of Sodium Chloride Solution. Eng. Geology. 155 (6), 69–79. doi:10.1016/j.enggeo.2012.12.018
Zhang, Y., Guo, C., Lan, H., Zhou, N., and Yao, X. (2015). Reactivation Mechanism of Ancient Giant Landslides in the Tectonically Active Zone: a Case Study in Southwest China. Environ. Earth Sci. 74 (2), 1719–1729. doi:10.1007/s12665-015-4180-6
Zhang, Z., Wang, T., Wu, S., Tang, H., and Liang, C. (2018). Dynamics Characteristic of Red clay in a Deep-Seated Landslide, Northwest China: An experiment Study. Eng. Geology. 239, 254–268. doi:10.1016/j.enggeo.2018.04.005
Keywords: loess, landslide, slip zone soil, ring shear, mechanical behavior
Citation: Liu K, Wang X, Lian B, Zhu Z and Xue C (2022) Study on Mechanical Behavior of Slip Zone Soils Under Different Factors—A Case Study. Front. Earth Sci. 10:847772. doi: 10.3389/feart.2022.847772
Received: 03 January 2022; Accepted: 04 March 2022;
Published: 21 March 2022.
Edited by:
Fanyu Zhang, Lanzhou University, ChinaReviewed by:
Yulong Cui, Anhui University of Science and Technology, ChinaSujatha Evangelin Ramani, SASTRA University, India
Copyright © 2022 Liu, Wang, Lian, Zhu and Xue. This is an open-access article distributed under the terms of the Creative Commons Attribution License (CC BY). The use, distribution or reproduction in other forums is permitted, provided the original author(s) and the copyright owner(s) are credited and that the original publication in this journal is cited, in accordance with accepted academic practice. No use, distribution or reproduction is permitted which does not comply with these terms.
*Correspondence: Xingang Wang, eGd3YW5nQG53dS5lZHUuY24=