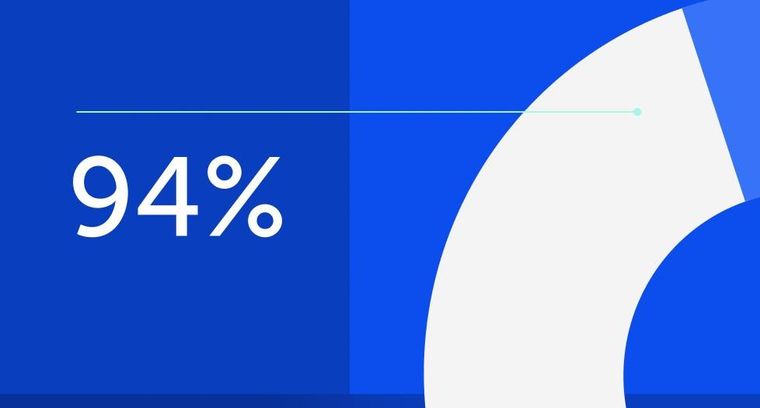
94% of researchers rate our articles as excellent or good
Learn more about the work of our research integrity team to safeguard the quality of each article we publish.
Find out more
ORIGINAL RESEARCH article
Front. Earth Sci., 18 March 2022
Sec. Hydrosphere
Volume 10 - 2022 | https://doi.org/10.3389/feart.2022.839151
This article is part of the Research TopicLake Changes, Drivers and Consequences in High Mountain AsiaView all 7 articles
Lake water temperature and the related thermal structure influence not only the provision of ecosystem services in lacustrine environments but also the interactions with regional climate. However, continuous lake temperature monitoring across the Tibetan Plateau is sparse, limiting our understanding of lake thermal and mixing dynamics and hindering the verification of modeling results in this region. Based on in-situ water temperature and meteorological observations, this study revealed a special summer destratification phenomenon of a deep alpine lake, Langa Co. The results indicate that Langa Co is a discontinuous cold polymictic lake, which becomes completely mixed and reaches a homogeneous water temperature frequently during spring and autumn. Further, the intermittent periods of stratification in summer only last a few days, which is rare for such a deep lake. As an example of a discontinuous cold polymictic lake that contrasts with the typical dimictic pattern of alpine lakes, studies of Langa Co help to gain insights into lake thermal processes and thermoregulation mechanisms and establish a reference for lake model evaluation and parameterization on the Tibetan Plateau.
Thermal stratification exerts an important control on the vertical fluxes of dissolved and particulate material in lakes (Robertson and Imberger, 1994; Foley et al., 2012), with strong potential impacts on local and even regional climate through its influence on water and heat exchanges between the lake and atmosphere (Lim et al., 2012; Wang et al., 2015; Wang et al., 2019a). Stratification is facilitated by the thermal expansion properties of water, which creates a stable vertical density gradient. Density gradients are often observed within a region with sharp changes in water temperature (metalimnion) that delineates an upper well-mixed region (epilimnion) from a relatively quiescent deep zone (hypolimnion) (Macintyre and Melack, 2010). Seasonal stratification and mixing patterns serve as a basis for the classification of lake regimes and are essential in projections of lake response to climate change. Recent global studies show that climatic trends have resulted in an overall increase in thermal stability with longer periods of summer stratification and steeper thermoclines and mixing type changes (Kraemer et al., 2015; Woolway and Merchant, 2019). A change in mixing regime can trigger changes in physicochemical parameters (e.g., anoxia) that can feedback to the vertical density distribution or heat budget (Mesman et al., 2021).
The Tibetan Plateau (TP) is one of the most sensitive areas to global climate change (Kuang and Jiao, 2016; Duan et al., 2019) and is experiencing warming at approximately three times the rate of the global mean (Field and Barros, 2014). More than 1,400 lakes with a water area greater than 1 km2 are scattered across the TP (Zhang et al., 2019). Lakes on the TP serve as an effective indicator and sentinel of climate changes because they are less strongly affected by human activities. Since the 1990s, lake areas and water storage have remarkably increased (Song et al., 2013; Zhang et al., 2017; Zhang et al., 2021). In addition, the lake ice duration has increased in the north but decreased in the south during the past 2 decades (Cai et al., 2019). Some lakes on the southern TP have even transitioned from being seasonally ice-covered to being ice-free all year. However, the temperature changes and lake regime shifts in response to climate change have rarely been addressed (Zhang et al., 2020). Currently, lake temperature observations in the TP are still scarce because of the higher risk in instrument detecting and more difficulty in data acquisition (Duan et al., 2021). The long-term measurements (with at least one full season of water temperature monitoring) are available only at Nam Co, Paiku Co, Bangong Co, and Dagze Co (for its mixomolimnion), but these lakes are all dimictic, meaning that they mix from top to bottom twice yearly and stratify throughout the summer (Wang et al., 2019c; Wang et al., 2020b; Lei et al., 2021b; Lazhu et al., 2021; Wang et al., 2021). It is therefore necessary to enrich the observed lake types and achieve a more detailed understanding of the lake thermal structure.
In this study, we present the water temperature variability based on continuous in-situ monitoring during the ice-free period at the 40 m-deep lake on the TP, Langa Co, with a focus on the pattern of stratification and mixing and circulation type of this lake. The peculiar phenomenon of summer destratification emerges from observational data, and its reasons are discussed. Moreover, the performance of a one-dimensional lake model in simulating the phenomenon was assessed. The main findings of this work complete our understanding of the lake thermal processes and establish a reference for lake model improvement on the TP.
Langa Co (30.58°–30.85°N, 81.10°–81.33°E, 4,575 m a.s.l.; Figure 1) is a spoon-shaped lake, with an area of approximately 258.9 km2 (Liu et al., 2021). River inputs to the lake are the greatest during the summer season, and the largest supply is glacial melt water from the north. A bathymetric survey showed that the lake reaches a depth of 49.03 m (Wang et al., 2013). The southern part, which has a mostly flat lake bed, is ∼40 m deep. The northern part of the lake is an alluvial fan with a depth of ∼10 m. The narrow central portion is ∼25 m deep and links the two parts. Climatically, the region belongs to semi-arid climate for interactions between westerly and monsoon climate. The annual mean air temperature and precipitation are approximately 2°C and ∼150 mm, respectively (Su et al., 2021). The precipitation mainly occurs in summer. The mean annual wind speed is ∼5 m s−1. The dominant wind direction is southwest throughout the year. The ice period is from December to next May, and the evaporation amount during the ice-free season is around 959.6 mm (Wang et al., 2020a).
The lake water temperature observation station was located in the southern deep part of the lake (30.63°N, 81.19°E; Figure 1). The vertical temperature profile was measured with the HOBO Water Temperature Pro v2 Data Logger U22-001 (Onset, United States) at depths of 0.5, 1, 1.5, 4.5, 9.5, 14.5, 19.5, 24.5, 29.5, 34.5, and 39.5 m. These levels were chosen to monitor the vertical variability of temperature within the water column and to evaluate model performances when simulating the lake thermal structure. As the lake can be completely covered by ice in winter, ice movement can cause instruments to drift significantly from their original location. Therefore, observations were only collected from June 6 to October 28, 2019. The water level was measured using HOBO Water level loggers U20-001 (Onset, United States). The fluctuations of water level were recorded as changes in pressure; hence, the air pressure data from the weather station were also used to estimate the water level. The Zongdui River discharge (30.96°N, 81.32°E) in the northern part of Langa Co was measured 11 times using handheld current meters during the ice-free period in 2019.
An automatic weather station (30.63°N, 81.18°E) was established on an island in the southern part of the lake from June 6, 2019 to help understand the meteorological conditions in the lake area and drive the lake model. The air temperature, humidity, precipitation, pressure, wind speed, and wind direction were measured at a 2 m height; downward short-wave and long-wave radiation fluxes were monitored at 1.5 m.
The Lake Analyzer is a MATLAB package used to calculate indices of stratification and mixing of lakes and reservoirs (Read et al., 2011). The program requires diverse inputs, including bathymetry, water temperature, wind, water level, and salinity. Here, the bathymetry and salinity were adopted from Wang et al. (2013) and Liu et al. (2021), while other parameters were based on the observations. The mixed layer depth (MLD), lake number (Ln), Wedderburn number (W), and Schmidt stability (St) were selected for subsequent analysis. Small values of Ln and W represent a high likelihood of upwelling events (Imberger and Patterson, 1989; Macintyre and Melack, 2010). Moreover, St represents the energy required at the moment when the stratified state is disintergrated. For details of the parameters and computation methods, please refer to the user manual (https://github.com/GLEON/Lake-Analyzer).
Lake model is the most common and effective tool to study the vertical temperature structure in lakes of various depths (Figure 2C). To evaluate the performance of the lake model in simulating the summer destratification phenomenon, a commonly used and proven lake model is used in this study. The WRF-Lake is based on CLM4-LISSS with some differences in lake surface properties and heat diffusivity (Gu et al., 2015). It is based on the original concept of the Hostetler model (Hostetler and Bartlein, 1990) and modified by Subin et al. (2012a). The model has been validated more on the TP and has proven to show the best at simulating the amplitude and pattern of temperature variability at all depths in Flake, WRF-Lake, and CoLM-Lake (Wen et al., 2016; Xu et al., 2016; Huang et al., 2019; Wu et al., 2020).
The WRF-Lake model is a mass and energy balance scheme with 20–25 model layers and solves the 1D heat diffusion equation. The lake includes 0–5 snow layers, 10 lake liquid water and ice layers, and 10 sediment layers (Subin et al., 2012b). The lake body water content is assumed to be constant, and sediment layers are fully saturated. It first calculates the energy and momentum exchange between the lake surface and the overlying atmosphere and then obtains the residual energy fluxes as the top boundary condition by surface energy balance. Second, it solves the thermal diffusion equation by dividing the lake vertical profile into several discrete layers corresponding to snow, lake body (containing liquid water and ice), and underlying substrates (sediment, soil, and bedrock). The convective mixing module is executed after the heat diffusion equation solution. The mixing processes in the lake body include molecular diffusion, wind-driven eddy diffusion, and convective mixing (Hostetler and Bartlein, 1990).
We focus on the intermittent stratification processes during summer in a discontinuous polymictic lake; therefore, the lake ice and snow processes are not considered. Meteorological observations with a temporal resolution of 30 min were used as input data to force WRF-Lake. Wind speeds at a 2 m height were adjusted to a 10 m height based on the wind profile law (Schertzer et al., 2003). The lake depth was set to 40 m, and a 25 layer vertical discretization was adopted for the lake column; this configuration has been demonstrated as optimal in simulating the variations of metalimnion temperature (Xiao et al., 2016). Experiments were run from June 6 to October 31, 2019, an the ice-free period of Langa Co, and the first 10 days were used for spin-up. The initial vertical temperature was set based on the observation profile. To improve the WRF-Lake model’s capability in reproducing the lake thermodynamics, we modified the model parameters as follows (Table 1).
First, we carried out a control experiment (CTR) with the default model configurations. Second, we modified the surface properties, diffusivity factor, light extinction coefficient, and the temperature of maximum water density by referring to the scheme from Wu et al. (2020) in SEN1. This scheme replaced the constant lake surface roughness lengths and improved the parameters based on the characteristics of alpine lakes, that is, a smaller temperature at the maximum water density (Tdmax) influenced by water salinity and lower surface air pressure, and higher water clarity under the less anthropogenic contaminations, and successfully reproduced the lake thermodynamics of Nam Co on the TP. Meanwhile, SEN1 was designed to evaluate the offset applicability of the scheme and explore the possibility of simulating the lakes on TP by the uniform parameterization scheme.
Because of the specificity of lakes, the transferability of the parameterization scheme is usually unsatisfactory. Based on results of the previous studies (Mallard et al., 2015; Xu et al., 2016; Wang et al., 2019b; Huang et al., 2019), we further conducted a series of sensitivity experiments by tuning the key parameters and amplified the enhanced diffusion term to calibrate and improve the model performance. The key parameters tuned with more than 7,000 combinations in the current study included the wind-driven eddy diffusivity multiplicative factor (fe; 0–200), the enhanced diffusion multiplicative factor (fed; 0–200), the light extinction coefficient multiplicative factor (feta; 0–2), and Tdmax (1–4°C). The wind-driven eddy diffusivity declines exponentially with depth, so simply magnifying it may trigger an overestimation in lake surface layers and an underestimation in deep layers (Wang et al., 2019b). The added fed could compensate for unresolved turbulence at a large depth (Subin et al., 2012b). Then, we determined the scale factor and parameters in SEN2 by minimizing the root-mean-square error (RMSE) between the simulations and observations. Finally, the scheme in SEN2 compared with SEN1 enlarged the fe and fed to strengthen the mixing, especially in deeper layers where wind-driven eddies cannot penetrate. The larger extinction coefficient was also closer to the lower clarity condition in Langa Co compared with Nam Co (Liu et al., 2021).
Performance was quantified against observed temperatures using the following four statistics: bias, RMSE, temporal correlation (TC), and Taylor score (TS) (Taylor, 2001)
Here,
The water temperature showed distinct seasonality at different depths (Figure 2A). June to mid-July was the mixing period when the water temperature during this period was uniform and gradually increased. The surface water temperature increased from 4.8 to 7.4°C. A weak temperature difference between the surface water and bottom occasionally developed but remained less than 1°C. From mid-July, stratification gradually developed and was promoted by gradually increasing radiative heating. With the rapid increase of lake surface temperature and relatively slow warming of deeper water, a clear thermal structure was developed by early August. On August 17, the vertical water body suddenly mixed and resulted in destratification. The surface lake water temperature decreased from 10.2 to 8.8°C, and the average temperature of the water body dropped to 8.5°C. The entire water temperature was generally homogeneous with the temperature difference between the surface and bottom being 0.7°C. Subsequently, the surface temperature increased under strong solar radiation conditions, but the bottom water warmed slower. The stratification reappeared as the difference in the heating rate at different layers and thus stabilized the thermal structure. The average temperature difference between the surface and bottom was 1.4°C through August, and the maximum difference was 3.2°C. In September, the solar radiation weakened, and the lake surface temperature fluctuated diurnally. However, the bottom temperature still increased slowly. This prompted the water body to overturn and minimized the temperature gradient. The temperatures of the upper and bottom then converged. In October, the surface water temperature decreased from 9.6 to 7.0°C as the water body constantly lost heat to the atmosphere with a lower air temperature. The average temperature difference between the surface and bottom was 0.3°C. The orange shading also showed the discontinuous stratification period, which is defined as the period when the temperature difference between the lake surface and bottom exceeds 1°C (Foley et al., 2012; Wang et al., 2019c).
FIGURE 2. Water temperature and stratification index variations. (A) Isotherms at Langa Co; numbers are the lake water temperatures (°C); (B) thermocline depth; (C) Wedderburn number and Lake number; (D) Schmidt stability. The orange shading represents the lake stratification period when the temperature difference between the lake surface and bottom exceeds 1°C.
Several stability indices were calculated by Lake Analyzer. This also enabled the attribution of the respective contributions of various factors such as wind (W and Ln) and convective cooling (St).
During the overturning in spring, the MLD (characterized by a homogeneous temperature in the upper water column) reached the full lake depth, indicating that the whole vertical profile was uniformly mixed (Figure 2B); in addition, W and Ln were small (about 10−3, Figure 2C). Both these indices were used to explain the potential for diapycnal mixing events. Index W showed greater variability when compared to Ln. This is because Ln was dependent on the depth to the midpoint of the metalimnion, which tended to reduce variability through depth averaging with the more stable base of the metalimnion; in contrast, W relied on the highly variable mixed layer depth (Read et al., 2011). During the stratification period in summer, MLD < 1 m and a temperature gradient developed below the mixed layer. W and Ln were larger, reflecting greater lake stability. However, W and Ln decreased in late August, and overturning restarted. From October onward, MLD reached the full lake depth. Rapid mixing led to a homogeneous temperature profile. Overall, Langa Co’s weak stratification and strong wind-driven mixing led to W and Ln values always below 1, representing a high likelihood of substantial diapycnal fluxes which may be contrary to the traditional assumptions of one-dimensional lake thermal characteristics.
The St index is shown in Figure 2D, where seasonal patterns indicate oscillations driven by alternating periods of mixing and stratification. St indicates the energy required at the moment the lake stratification is disrupted (Schmidt, 1928; Idso, 1973). During the mixing period in spring and autumn, St was closer to 0, showing that less energy was required to initiate lake overturning, leading to a higher likelihood of mixing. St gradually increased from mid-July. The mixing events that increased MLD in late July and early August as well as the cooling events that decreased the lake surface temperature in mid-August were shown clearly as a sudden decrease in St. The St index is strongly influenced by lake size and morphometry, resulting in a large reported range [from 0 to 5784 J m−2 (Kling, 1988)]. Compared with other lakes on the TP (Wang et al., 2014; Wang et al., 2019c), the maximum and mean value of St in Langa Co were smaller, indicating that less energy was needed for overturning to occur. Meanwhile, the changes of St and lake surface temperature were more consistent. At the beginning of June, St was close to 0. Increasing the surface water temperature made the water body unstable during the mixing period. The minimum value (1.5 J m−2) was on June 7, when the temperature was 4.7°C, and the maximum value of St was on August 10, when the lake surface temperature was also the maximum (11.4°C). After the thermal stratification was established, as the temperature increased, the rapidly increased St corresponded to the great enhancement in stratification stability.
Considering that a single observation location may not be representative, we collected temperature profile measurements at additional sites in 2020 and 2021, respectively (2020, 30.63°N, 81.19°E, water depth 45 m; 2021, 30.63°N, 81.29°E, water depth 40 m). Although there were some differences in the timing and magnitude of temperature changes, the observation in 2020 and 2021 also illustrated a similar pattern and summer destratification phenomenon at Langa Co (Supplementary Figure S1). The longer stratification break in summer occurred from August 16 to 27 in 2019, from August 13 to 28 in 2020, and earlier in 2021 which was from August 10 to 16. These measurements indicated that the stratification interruption was not an accidental occurrence. In addition, visible satellite imagery indicated that the ice period of Langa Co is from November to May (Supplementary Figure S2). After lake ice had melted, the water body had a homogeneous thermal status based on the water temperature observation. With the absorption of heat, the water temperature increased until thermal stratification developed. However, the observations showed that this stratification did not sustain during the summer and instead only lasted for a few days. During autumn, the epilimnion increasingly deepened until the water temperature became generally homogeneous. Finally, the temperature decreased and the lake froze over again. Thus, based on the definition and classification of lake mixing types, Langa Co can be classified as a discontinuous cold polymictic lake (Lewis, 1983; Kirillin and Shatwell, 2016; Woolway and Merchant, 2019), which is defined as a lake that is ice-covered for part of the year, ice-free above 4°C, and stratified during the warm season for periods of several days to weeks, but with irregular interruption by mixing. This regime often occurs in shallow lakes (Kalff, 2002), that is, Clear Lake (mean depth = 8.1 m; maximum depth = 18.4 m) (Rueda and Schladow, 2003), Lake Peipsi (mean depth = 7.1 m; maximum depth = 15.3 m) (Minella et al., 2013), and Lake Vortsjarv (mean depth = 2.8 m; maximum depth = 6.1 m) (Woolway et al., 2017). Rarely, Langa Co with a mean depth of around 22 m and a maximum depth of 49 m cannot sustain stratification in summer.
Currently, temperature observations of lakes on the TP have shown that nearly all completely mixed lakes are of the dimictic type, including Bangong Co (Wang et al., 2021), Nam Co (Wang et al., 2019c), Paiku Co (Lei et al., 2021a), Gongzhu Co (Lazhu et al., unpublished data), Ngoring Lake, and Gyaring Lake (Kirillin et al., 2017). Langa Co is the first reported of a different mixing type lake on the TP. Among these, the mean depths of Gongzhu Co, Ngoring Lake, and Gyaring Lake are shallower than that of Langa Co, with values of 6.6 m (Messager et al., 2016), 17 m, and 8.9 m, respectively. All three are clearly dimictic and stratified continuously in summer. In particular, Gongzhu Co and Langa Co have close climatic conditions with a distance of only 80 km. A similar summer stratified interruption phenomenon has not been observed in other lakes in TP at present.
Weather conditions set up stratification and currents in lakes (Boehrer and Schultze, 2008). The contemporaneous observation at the island showed that the formation and destruction of stratification corresponded to the meteorological changes (Figure 3). The onset of stratification usually coincided with a decrease in wind speed and an increase in solar radiation, while interruptions often coincided with an abrupt increase in wind speed. For example, the wind speed on July 19 was around 8.9 m/s and then gradually dropped to 1.3 m/s on July 23 when the temperature difference between the lake surface and bottom was more than 1°C. Meanwhile, the air temperature and solar radiation also increased so that the upper water gained heat and the density gradient of the entire water body increased. Subsequently, the wind speed continued to increase, reaching 6.5 m/s on July 24. The wind disturbance intensified the vertical turbulent mixing, causing water mixing. The decrease in the air temperature and radiation also lowered the stability of the water column within the lake. As for the deep mixing and cooling event on August 15, although the changes in radiation and wind speed were relatively less, the lake surface temperature was above the air temperature when the boundary layer was unstable, resulting in heat transfer from the water to the atmosphere (Wen et al., 2016). The surface layer cooled faster than the deep layer, which sparked the mixing of water up and down and destroyed the thermal structure. The average wind speed in August at Langa Co was 5.5 m/s, which was higher than those of Nam Co (around 2.9 m/s, Lazhu et al., 2016) and Bangong Co (around 3 m/s, Wang et al., 2021), although the solar radiation was slightly higher than that of Nam Co. Thus, the wind disturbance may cause weak stratification at Langa Co.
FIGURE 3. Comparison between meteorological parameters with the thermal processes. (A) Daily mean temperature of air (solid line) and that of the water surface (dotted line); (B) average wind speed; (C) daily mean solar radiation. The shading represents the lake stratification period when the temperature difference between the lake surface and bottom exceeds 1°C.
To assess the performance of the thermal structure simulation of the alpine lake of this type and the possibility of determining the mixing regime by the lake model, a series of experiments with WRF-Lake were carried out at the profile observation site from June 6 to October 31 in 2019 (Figure 4). Using the default model configurations (CTR), the water temperature was underestimated and significantly faster cooling was simulated in autumn as a result of the unrealistic parameters for the alpine lake. The higher light extinction coefficient led to less heat allocation at bottom water. Moreover, the default surface roughness length was larger than the real value; thus, unrealistically strong wind-driven mixing triggered excessive loss of latent heat. By adjusting the Tdmax from 4°C for the freshwater lake to the observed 3.5°C in Nam Co on TP, replacing the surface roughness scheme and decreasing the light extinction coefficient, SEN1 increased the warming amplitude of upper water. Meanwhile, the smaller light extinction in SEN1 allowed more heat to be transferred downward, slowing the rate of heat loss. On the whole, SEN1 could reproduce the changing temperature distribution and warming–brief cooling–warming–cooling pattern, but the simulated lake mixing was also too weak. Both CTL and SEN1 used a single multiplier to expand the wind-driven eddy diffusivity, which likely overestimated the diffusivity in surface layers and underestimated it in deeper layers (Wang et al., 2019b). By amplifying the enhanced diffusion term in SEN2, the mixing of the bottom layers was more complete in spring. Meanwhile, the modified parameter scheme (SEN2) based on the minimum RMSE increased the extinction coefficient and decreased the Tdmax, which was closer to the reality of the lower water clarity at Langa Co (Liu et al., 2021). Overall, SEN2 was better to simulate the warming and cooling processes in spring and autumn. The mixing depth was closer to the observed one, and the heat can be transmitted to the bottom. The result reproduced the destratification phenomenon in summer in late August, although the magnitude of cooling was much weaker than the observed one.
FIGURE 4. Water temperature (unit: °C) distribution from observation (A) and simulations with CTR (B), SEN1 (C), and SEN2 (D).
Figure 5 shows the model’s accuracy in reproducing the amplitude and pattern of water temperature variability during the simulation period and the individual months. Overall, the bias and RMSE of SEN2 were 0.3 and 0.4°C, respectively, significantly lower than those of CTR and SEN1. In CTR, the greatest error was from October onward because of rapid cooling in autumn. The bias and RMSE in CTR were 0.6 and 0.9°C, respectively. The error in SEN1 was the greatest in August due to overestimated heat storage. The bias and RMSE in SEN1 were 0.4 and 0.5°C, respectively. The TC of all experiments showed consistency in the simulated temporal variability, except in August and September. This suggested that WRF-Lake cannot accurately represent the discontinuous summer stratification in Langa Co. It may be necessary to consider additional physical processes during this period in simulation for Langa Co. Meanwhile, the little improvement in accuracy by parameter adjustment also implied that the failed replication of the cooling process in summer may not be the reason for the parameterization scheme but the model itself.
FIGURE 5. Bias, RMSE, TC, and TS between the simulated and observed daily mean temperatures during the simulation period and the individual months.
The simulation errors are probably due to the lack of physical processes for the lateral exchange and runoff in WRF-Lake. The uneven bathymetry of the lake basin could intensify horizontal advection, making the water temperature more susceptible to local disturbance (Monismith and Morison, 1990). As for Langa Co, the slope of the topography can lead to more complex lake mixing processes through its effects on the advective heat transport across the thermal front and on the wind stress (Rao and Schwab, 2007; Beletsky et al., 2012; Xue et al., 2015). Meanwhile, from the observation of the main river in the Langa Co basin, the runoff reached its maximum (16.2 m3/s) during the summer stratification interruption (Supplementary Figure S3). The intrusion of river inflow may exacerbate lateral exchange (Ambrosetti et al., 2010). The turbid cold glacial melt water mass invaded from the shallow northern part of Langa Co and moved downward along the lake slopes, decreasing the lake water temperature and increasing light attenuation (Peeters et al., 2003). Several similar mixing events during the summer were observed in four glacier-fed lakes (all of less than 16 m depth) in the western Austrian Alps (Peter and Sommaruga, 2017). The cooling and mixing effects of glacier melt water discharge may cause an atypical lake mixing pattern for temperate alpine lakes (Catalan et al., 2002). However, the evaluation of how the thermal structure of the Langa Co water column is influenced by glacier melt requires further observations of runoff and multi-point continuous lake temperature monitoring. In addition, as both the availability of observations and computing power increase, the evaluation and development of three-dimensional lake models should be considered in the future. Furthermore, the TP holds a glacier ice volume of ∼7.0 × 103 km2 (Farinotti et al., 2019), which feeds numerous lakes. Under the background of glacier retreat and snowmelt (Cheng and Wu, 2007; Bolch et al., 2019), it is essential to consider the effect of glacier runoff in lake simulations. This will also help in qualitative examinations of lake responses to the local cryosphere, which are absent in lake studies on the TP (Zhang et al., 2020).
Based on the in-situ water temperature and meteorological observations, we investigated the rare summer destratification phenomenon at a deep alpine lake, Langa Co. A comparison of observations with the definition of lake mixing types suggests that Langa Co should be classified as a discontinuous cold polymictic lake instead of the typical dimictic pattern of an alpine lake. Stratification occurred intermittently in summer, but these periods lasted only several days. The lake was completely mixed during spring and autumn. This is the first continuous time series of lake temperature observations for a lake with this thermal regime on the TP, and this is in contrast to the typical dimictic pattern of alpine lakes. The calculated MLD indicated the discontinuous stratification period, while the small values of W, Ln, and St indicated the high likelihood of mixing and weak stratification. This study is important for understanding temperature variations of polymictic lakes and for revealing the diverse thermal regimes of the TP lakes. Furthermore, compared with meteorological observation, the formation and destruction of the thermal stratification may mainly result from the changes in radiation and strong wind. The observation-driven lake model could reproduce the disruption of stratification in summer.
The raw data supporting the conclusion of this article will be made available by the authors, without undue reservation.
RS: conceptualization, methodology, investigation, data curation, visualization, and writing—original draft. ZX: methodology, investigation, and writing—review and editing. WM: project administration, funding acquisition, and writing—review and editing. YM: supervision and writing—review and editing. BW: investigation and writing—review and editing. WH: investigation and data curation. ZS: investigation and writing—review and editing.
This research has been funded by the National Natural Science Foundation of China (41830650, 91837208, and 42075085), the Second Tibetan Plateau Scientific Expedition and Research (STEP) program (Grant no. 2019QZKK0103), the Strategic Priority Research Program of Chinese Academy of Sciences (XDA20060101), the National Program on Key Basic Research Project (2018YFC1505701), and the China Scholarship Council (award to RS for 1 year’s study abroad at the University of Twente).
The authors declare that the research was conducted in the absence of any commercial or financial relationships that could be construed as a potential conflict of interest.
All claims expressed in this article are solely those of the authors and do not necessarily represent those of their affiliated organizations or those of the publisher, the editors, and the reviewers. Any product that may be evaluated in this article or claim that may be made by its manufacturer is not guaranteed or endorsed by the publisher.
We thank Prof. Anning Huang and Dr. Yang Wu for providing us with the code of WRF-Lake. We also appreciate all the members who took part in the fieldwork. We gratefully thank the two reviewers whose comments contributed to the improvement of the original text.
The Supplementary Material for this article can be found online at: https://www.frontiersin.org/articles/10.3389/feart.2022.839151/full#supplementary-material
Ambrosetti, W., Barbanti, L., and Carrara, E. A. (2010). Mechanisms of Hypolimnion Erosion in a Deep lake (Lago Maggiore, N. Italy). J. Limnol. 69, 3. doi:10.4081/jlimnol.2010.3
Beletsky, D., Hawley, N., Rao, Y. R., Vanderploeg, H. A., Beletsky, R., Schwab, D. J., et al. (2012). Summer thermal Structure and Anticyclonic Circulation of Lake Erie. Geophys. Res. Lett. 39. doi:10.1029/2012GL051002
Boehrer, B., and Schultze, M. (2008). Stratification of Lakes. Rev. Geophys. 46, 1–27. doi:10.1029/2006RG000210
Bolch, T., Shea, J. M., Liu, S., Azam, F. M., Gao, Y., Gruber, S., et al. (2019). Status and Change of the Cryosphere in the Extended Hindu Kush Himalaya Region, in The Hindu Kush Himalaya Assessment, ed. S. A. Wester P., Mishra A., Mukherji A. (Springer, Cham), 209–255. doi:10.1007/978-3-319-92288-1_7
Cai, Y., Ke, C. Q., Li, X., Zhang, G., Duan, Z., and Lee, H. (2019). Variations of Lake Ice Phenology on the Tibetan Plateau from 2001 to 2017 Based on MODIS Data. J. Geophys. Res. Atmos. 124, 825–843. doi:10.1029/2018JD028993
Catalan, J., Ventura, M., Brancelj, A., Granados, I., Thies, H., Nickus, U., et al. (2002). Seasonal Ecosystem Variability in Remote mountain lakes: Implications for Detecting Climatic Signals in Sediment Records. J. Paleolimnol. 28, 25–46. doi:10.1023/a:1020315817235
Cheng, G., and Wu, T. (2007). Responses of Permafrost to Climate Change and Their Environmental Significance, Qinghai-Tibet Plateau. J. Geophys. Res. Earth Surf. 112, 3. doi:10.1029/2006jf000631
Duan, W., Hanasaki, N., Shiogama, H., Chen, Y., Zou, S., Nover, D., et al. (2019). Evaluation and Future Projection of Chinese Precipitation Extremes Using Large Ensemble High-Resolution Climate Simulations. J. Clim. 32, 2169–2183. doi:10.1175/jcli-d-18-0465.1
Duan, W., Maskey, S., Chaffe, P. L. B., Luo, P., He, B., Wu, Y., et al. (2021). Recent Advancement in Remote Sensing Technology for Hydrology Analysis and Water Resources Management. Remote Sensing 13, 1097. doi:10.3390/rs13061097
Farinotti, D., Huss, M., Fürst, J. J., Landmann, J., Machguth, H., Maussion, F., et al. (2019). A Consensus Estimate for the Ice Thickness Distribution of All Glaciers on Earth. Nat. Geosci. 12, 168–173. doi:10.1038/s41561-019-0300-3
Field, C. B., and Barros, V. R. (2014). Climate Change 2014–Impacts, Adaptation and Vulnerability: Regional Aspects. Cambridge: Cambridge University Press, 1101.
Foley, B., Jones, I. D., Maberly, S. C., and Rippey, B. (2012). Long-term Changes in Oxygen Depletion in a Small Temperate lake: Effects of Climate Change and Eutrophication. Freshw. Biol. 57, 278–289. doi:10.1111/j.1365-2427.2011.02662.x
Gu, H., Jin, J., Wu, Y., Ek, M. B., and Subin, Z. M. (2015). Calibration and Validation of lake Surface Temperature Simulations with the Coupled WRF-lake Model. Climatic Change 129, 471–483. doi:10.1007/s10584-013-0978-y
Hostetler, S. W., and Bartlein, P. J. (1990). Simulation of lake Evaporation with Application to Modeling lake Level Variations of Harney-Malheur Lake, Oregon. Water Resour. Res. 26, 2603–2612. doi:10.1029/WR026i010p02603
Huang, A., LazhuWang, J., Wang, J., Dai, Y., Yang, K., Wei, N., et al. (2019). Evaluating and Improving the Performance of Three 1‐D Lake Models in a Large Deep Lake of the Central Tibetan Plateau. J. Geophys. Res. Atmos. 124, 3143–3167. doi:10.1029/2018JD029610
Idso, S. B. (1973). On the Concept of lake Stability1. Limnol. Oceanogr. 18, 681–683. doi:10.4319/lo.1973.18.4.0681
Kalff, J. (2002). Limnology: Inland Water Ecosystems. CA: National Scientific Programmes Unit: CSIR.
Kirillin, G., and Shatwell, T. (2016). Generalized Scaling of Seasonal thermal Stratification in Lakes. Earth-Science Rev. 161, 179–190. doi:10.1016/j.earscirev.2016.08.008
Kirillin, G., Wen, L., and Shatwell, T. (2017). Seasonal thermal Regime and Climatic Trends in Lakes of the Tibetan highlands. Hydrol. Earth Syst. Sci. 21, 1895–1909. doi:10.5194/hess-21-1895-2017
Kling, G. W. (1988). Comparative Transparency, Depth of Mixing, and Stability of Stratification in Lakes of Cameroon, West Africa1. Limnol. Oceanogr. 33, 27–40. doi:10.4319/lo.1988.33.1.0027
Kraemer, B. M., Anneville, O., Chandra, S., Dix, M., Kuusisto, E., Livingstone, D. M., et al. (2015). Morphometry and Average Temperature Affect lake Stratification Responses to Climate Change. Geophys. Res. Lett. 42, 4981–4988. doi:10.1002/2015GL064097
Kuang, X., and Jiao, J. J. (2016). Review on Climate Change on the Tibetan Plateau during the Last Half century. J. Geophys. Res. Atmos. 121, 3979–4007. doi:10.1002/2015JD024728
Lazhu, Y., Yang, K., Hou, J., Wang, J., Lei, Y., Zhu, L., et al. (2021). A New Finding on the Prevalence of Rapid Water Warming during lake Ice Melting on the Tibetan Plateau. Sci. Bull. 66, 2358–2361. doi:10.1016/j.scib.2021.07.022
Lazhu, Y., Yang, K., Wang, J., Lei, Y., Chen, Y., Zhu, L., et al. (2016). Quantifying Evaporation and its Decadal Change for Lake Nam Co, central Tibetan Plateau. J. Geophys. Res. Atmos. 121, 7578–7591. doi:10.1002/2015JD024523
Lei, Y., Yao, T., Tian, L., Sheng, Y., LiaoLiao, J., Zhao, H., et al. (2021a). Response of Downstream Lakes to Aru Glacier Collapses on the Western Tibetan Plateau. The Cryosphere 15, 199–214. doi:10.5194/tc-15-199-2021
Lei, Y., Yao, T., Yang, K., Ma, Y., and Bird, B. W. (2021b). Contrasting Hydrological and thermal Intensities Determine Seasonal lake-level Variations - a Case Study at Paiku Co on the Southern Tibetan Plateau. Hydrol. Earth Syst. Sci. 25, 3163–3177. doi:10.5194/hess-25-3163-2021
Lewis Jr., W. M. (1983). A Revised Classification of Lakes Based on Mixing. Can. J. Fish. Aquat. Sci. 40, 1779–1787. doi:10.1139/f83-207
Lim, S., Jang, C. J., Oh, I. S., and Park, J. (2012). Climatology of the Mixed Layer Depth in the East/Japan Sea. J. Mar. Syst. 96-97, 1–14. doi:10.1016/j.jmarsys.2012.01.003
Liu, C., Zhu, L., Wang, J., Ju, J., Ma, Q., Qiao, B., et al. (2021). In-situ Water Quality Investigation of the Lakes on the Tibetan Plateau. Sci. Bull. 66, 1727–1730. doi:10.1016/j.scib.2021.04.024
Macintyre, S., and Melack, J. M. (2010). Mixing Dynamics in Lakes across Climatic Zones, in Lake ecosystem ecology: A global perspective, ed. G. E. Likens (Encyclopedia of Inland Waters), 86–89.
Mallard, M. S., Nolte, C. G., Spero, T. L., Bullock, O. R., Alapaty, K., Herwehe, J. A., et al. (2015). Technical Challenges and Solutions in Representing Lakes when Using WRF in Downscaling Applications. Geosci. Model. Dev.Model Dev. 8, 1085–1096. doi:10.5194/gmd-8-1085-2015
Mesman, J. P., Stelzer, J. A. A., Dakos, V., Goyette, S., Jones, I. D., Kasparian, J., et al. (2021). The Role of Internal Feedbacks in Shifting Deep lake Mixing Regimes under a Warming Climate. Freshw. Biol. 66, 1021–1035. doi:10.1111/fwb.13704
Messager, M. L., Lehner, B., Grill, G., Nedeva, I., and Schmitt, O. (2016). Estimating the Volume and Age of Water Stored in Global Lakes Using a Geo-Statistical Approach. Nat. Commun. 7. doi:10.1038/ncomms13603
Minella, M., De Laurentiis, E., Buhvestova, O., Haldna, M., Kangur, K., Maurino, V., et al. (2013). Modelling lake-water Photochemistry: Three-Decade Assessment of the Steady-State Concentration of Photoreactive Transients (OH, and 3CDOM∗) in the Surface Water of Polymictic Lake Peipsi (Estonia/Russia). Chemosphere 90, 2589–2596. doi:10.1016/j.chemosphere.2012.10.103
Monismith, S. G., Imberger, J., and Morison, M. L. (1990). Convective Motions in the Sidearm of a Small Reservoir. Limnol. Oceanogr. 35, 1676–1702. doi:10.4319/lo.1990.35.8.1676
Peeters, F., Finger, D., Hofer, M., Brennwald, M., Livingstone, D. M., and Kipfer, R. (2003). Deep-water Renewal in Lake Issyk-Kul Driven by Differential Cooling. Limnol. Oceanogr. 48, 1419–1431. doi:10.4319/lo.2003.48.4.1419
Peter, H., and Sommaruga, R. (2017). Alpine Glacier-Fed Turbid Lakes Are Discontinuous Cold Polymictic rather Than Dimictic. Inland Waters 7, 45–54. doi:10.1080/20442041.2017.1294346
Rao, Y. R., and Schwab, D. J. (2007). Transport and Mixing between the Coastal and Offshore Waters in the Great Lakes: A Review. J. Great Lakes Res. 33, 202–218. doi:10.3394/0380-1330(2007)33[202:tambtc]2.0.co;2
Read, J. S., Hamilton, D. P., Jones, I. D., Muraoka, K., Winslow, L. A., Kroiss, R., et al. (2011). Derivation of lake Mixing and Stratification Indices from High-Resolution lake Buoy Data. Environ. Model. Softw. 26, 1325–1336. doi:10.1016/j.envsoft.2011.05.006
Robertson, D. M., and Imberger, J. (1994). Lake Number, a Quantitative Indicator of Mixing Used to Estimate Changes in Dissolved Oxygen. Int. Revue Ges. Hydrobiol. Hydrogr. 79, 159–176. doi:10.1002/iroh.19940790202
Rongmingzhu, S., Ma, W., Weiqiang, M., Yaoming, M., Zhipeng, X., Binbin, W., et al. (2021). Investigation of thermal Stratification and Mixed Layer Depth in La'ang Co in the Tibetan Plateau. J. Lake Sci. 33, 550–560. doi:10.18307/2021.0220
Rueda, F. J., and Schladow, S. G. (2003). Dynamics of Large Polymictic Lake. II: Numerical Simulations. J. Hydraul. Eng. 129, 92–101. doi:10.1061/(asce)0733-9429(2003)129:2(92)
Schertzer, W. M., Rouse, W. R., Blanken, P. D., and Walker, A. E. (2003). Over-Lake Meteorology and Estimated Bulk Heat Exchange of Great Slave Lake in 1998 and 1999. J. Hydrometeor 4, 649–659. doi:10.1175/1525-7541(2003)004<0649:omaebh>2.0.co;2
Schmidt, W. (1928). Über Die Temperatur- Und Stabili-Tätsverhältnisse Von Seen. Geografiska Annaler 10, 145–177. doi:10.1080/20014422.1928.11880475
Song, C., Huang, B., and Ke, L. (2013). Modeling and Analysis of lake Water Storage Changes on the Tibetan Plateau Using Multi-mission Satellite Data. Remote Sensing Environ. 135, 25–35. doi:10.1016/j.rse.2013.03.013
Subin, Z. M., Murphy, L. N., Li, F., Bonfils, C., and Riley, W. J. (2012a). Boreal Lakes Moderate Seasonal and Diurnal Temperature Variation and Perturb Atmospheric Circulation: Analyses in the Community Earth System Model 1 (CESM1). Tellus A: Dynamic Meteorology and Oceanography 64, 15639. doi:10.3402/tellusa.v64i0.15639
Subin, Z. M., Riley, W. J., and Mironov, D. (2012b). An Improved lake Model for Climate Simulations: Model Structure, Evaluation, and Sensitivity Analyses in CESM1. J. Adv. Model. Earth Syst. 4, M02001. doi:10.1029/2011MS000072
Taylor, K. E. (2001). Summarizing Multiple Aspects of Model Performance in a Single Diagram. J. Geophys. Res. 106, 7183–7192. doi:10.1029/2000JD900719
Wang, B., Ma, Y., Chen, X., Ma, W., Su, Z., and Menenti, M. (2015). Observation and Simulation of lake-air Heat and Water Transfer Processes in a High-Altitude Shallow lake on the Tibetan Plateau. J. Geophys. Res. Atmos. 120, 327–344. doi:10.1002/2015JD023863.Received
Wang, B., Ma, Y., Su, Z., Wang, Y., and Ma, W. (2020a). Quantifying the Evaporation Amounts of 75 High-Elevation Large Dimictic Lakes on the Tibetan Plateau. Sci. Adv. 6. doi:10.1126/sciadv.aay8558
Wang, B., Ma, Y., Wang, Y., Su, Z., and Ma, W. (2019a). Significant Differences Exist in lake-atmosphere Interactions and the Evaporation Rates of High-Elevation Small and Large Lakes. J. Hydrol. 573, 220–234. doi:10.1016/j.jhydrol.2019.03.066
Wang, F., Ni, G., Riley, W. J., Tang, J., Zhu, D., and Sun, T. (2019b). Evaluation of the WRF lake Module (v1.0) and its Improvements at a Deep Reservoir. Geosci. Model. Dev. 12, 2119–2138. doi:10.5194/gmd-12-2119-2019
Wang, J., Huang, L., Ju, J., Daut, G., Ma, Q., Zhu, L., et al. (2020b). Seasonal Stratification of a Deep, High-Altitude, Dimictic lake: Nam Co, Tibetan Plateau. J. Hydrol. 584, 124668. doi:10.1016/j.jhydrol.2020.124668
Wang, J., Huang, L., Ju, J., Daut, G., Wang, Y., Ma, Q., et al. (2019c). Spatial and Temporal Variations in Water Temperature in a High-Altitude Deep Dimictic mountain lake (Nam Co), central Tibetan Plateau. J. Great Lakes Res. 45, 212–223. doi:10.1016/j.jglr.2018.12.005
Wang, J., Peng, P., Ma, Q., and Zhu, L. (2013). Investigation of Water Depth, Water Quality and Modern Sedimentation Rate in Mapam Yumco and La’ang Co, Tibet. J. Lake Sci. 25, 609–616.
Wang, M., Hou, J., Lazhu, L., Li, X., Liang, J., Xie, S., et al. (2021). Changes in the lake thermal and Mixing Dynamics on the Tibetan Plateau. Hydrological Sci. J. 66, 838–850. doi:10.1080/02626667.2021.1887487
Wang, M., Hou, J., and Lei, Y. (2014). Classification of Tibetan Lakes Based on Variations in Seasonal lake Water Temperature. Chin. Sci. Bull. 59, 4847–4855. doi:10.1007/s11434-014-0588-8
Wen, L., Lyu, S., Kirillin, G., Li, Z., and Zhao, L. (2016). Air-lake Boundary Layer and Performance of a Simple lake Parameterization Scheme over the Tibetan highlands. Tellus A: Dynamic Meteorology and Oceanography 68, 31091. doi:10.3402/tellusa.v68.31091
Woolway, R. I., Meinson, P., Nõges, P., Jones, I. D., and Laas, A. (2017). Atmospheric Stilling Leads to Prolonged thermal Stratification in a Large Shallow Polymictic lake. Climatic Change 141, 759–773. doi:10.1007/s10584-017-1909-0
Woolway, R. I., and Merchant, C. J. (2019). Worldwide Alteration of lake Mixing Regimes in Response to Climate Change. Nat. Geosci. 12, 271–276. doi:10.1038/s41561-019-0322-x
Wu, Y., Huang, A., LazhuYang, X., Yang, X., Qiu, B., Wen, L., et al. (2020). Improvements of the Coupled WRF-Lake Model over Lake Nam Co, Central Tibetan Plateau. Clim. Dyn. 55, 2703–2724. doi:10.1007/s00382-020-05402-3
Xiao, C., Lofgren, B. M., Wang, J., and Chu, P. Y. (2016). Improving the lake Scheme within a Coupled WRF‐lake Model in the L Aurentian G Reat L Akes. J. Adv. Model. Earth Syst. 8, 1969–1985. doi:10.1002/2016MS000717
Xu, L., Liu, H., Du, Q., and Wang, L. (2016). Evaluation of the WRF-lake Model over a highland Freshwater lake in Southwest China. J. Geophys. Res. Atmos. 121 (13), 989–1014. doi:10.1002/2016JD025396
Xue, P., Schwab, D. J., and Hu, S. (2015). An Investigation of the thermal Response to Meteorological Forcing in a Hydrodynamic Model of Lake Superior. J. Geophys. Res. Ocean. 120, 775–791. doi:10.1002/2015jc010740
Zhang, G., Bolch, T., Chen, W., and Crétaux, J.-F. (2021). Comprehensive Estimation of lake Volume Changes on the Tibetan Plateau during 1976-2019 and basin-wide Glacier Contribution. Sci. Total Environ. 772, 145463. doi:10.1016/j.scitotenv.2021.145463
Zhang, G., Yao, T., Chen, W., Zheng, G., Shum, C. K., Yang, K., et al. (2019). Regional Differences of lake Evolution across China during 1960s-2015 and its Natural and Anthropogenic Causes. Remote Sensing Environ. 221, 386–404. doi:10.1016/j.rse.2018.11.038
Zhang, G., Yao, T., Xie, H., Yang, K., Zhu, L., Shum, C. K., et al. (2020). Response of Tibetan Plateau Lakes to Climate Change: Trends, Patterns, and Mechanisms. Earth-Science Rev. 208, 103269. doi:10.1016/j.earscirev.2020.103269
Keywords: thermal stratification, polymictic lake, Tibetan Plateau, Langa Co, WRF-Lake model
Citation: Su R, Xie Z, Ma W, Ma Y, Wang B, Hu W and Su Z (2022) Summer Lake Destratification Phenomenon: A Peculiar Deep Lake on the Tibetan Plateau. Front. Earth Sci. 10:839151. doi: 10.3389/feart.2022.839151
Received: 19 December 2021; Accepted: 21 February 2022;
Published: 18 March 2022.
Edited by:
Qiao Liu, Institute of Mountain Hazards and Environment (CAS), ChinaReviewed by:
Weili Duan, Xinjiang Institute of Ecology and Geography (CAS), ChinaCopyright © 2022 Su, Xie, Ma, Ma, Wang, Hu and Su. This is an open-access article distributed under the terms of the Creative Commons Attribution License (CC BY). The use, distribution or reproduction in other forums is permitted, provided the original author(s) and the copyright owner(s) are credited and that the original publication in this journal is cited, in accordance with accepted academic practice. No use, distribution or reproduction is permitted which does not comply with these terms.
*Correspondence: Weiqiang Ma, d3FtYUBpdHBjYXMuYWMuY24=
Disclaimer: All claims expressed in this article are solely those of the authors and do not necessarily represent those of their affiliated organizations, or those of the publisher, the editors and the reviewers. Any product that may be evaluated in this article or claim that may be made by its manufacturer is not guaranteed or endorsed by the publisher.
Research integrity at Frontiers
Learn more about the work of our research integrity team to safeguard the quality of each article we publish.