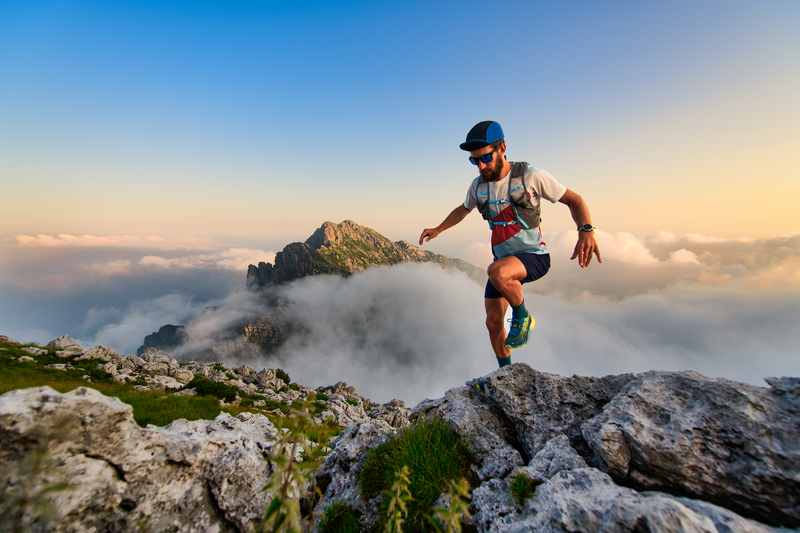
95% of researchers rate our articles as excellent or good
Learn more about the work of our research integrity team to safeguard the quality of each article we publish.
Find out more
MINI REVIEW article
Front. Earth Sci. , 02 February 2022
Sec. Geomagnetism and Paleomagnetism
Volume 10 - 2022 | https://doi.org/10.3389/feart.2022.834024
The Cretaceous Normal Superchron (CNS) was first defined in the 1960s to explain the Cretaceous Quiet Zone in marine magnetic anomaly profiles, which includes no or fewer geomagnetic reversals. This ∼37 million years period is considered the most unique and extreme geomagnetic feature for the last 160 Myr. Superchrons may be caused by the geodynamo operating at peak efficiency with a unique heat flux at the core-mantle boundary (CMB). Previous studies suggest that the CNS is a sign of the connection between Earth’s interior and surface. During the CNS, the geomagnetic intensity may have fluctuated significantly, and the average may have changed with time, and the paleosecular variations had unique features. The warm climate around the CNS may have been caused by volcanic activity associated with active mantle convection. Such mantle convection increases heat flux at the CMB during the CNS, but geodynamo simulations predict small heat flux, which are inconsistent. This discrepancy may be resolved by the growth and collapse of a superplume or by an increase and decrease in the subduction flux.
The Cretaceous Normal Superchron (CNS) is an irregular stable polarity period in which no or few geomagnetic reversals occurred, lasting from 120 to 83 million years ago (Ma) (e.g., Ogg, 2020). Marine magnetic anomaly (MMA) includes the Cretaceous Quiet Zone (KQZ), which is considered evidence for the absence of geomagnetic reversals, defined as superchron. Until now, the absence of magnetic stripes in the KQZ made it challenging to use them for plate reconstructions. In 2012, two time-markers (named Q1 and Q2) were discovered by deep-tow magnetic anomaly observations in the Atlantic Ocean and the Southwest Indian Ridge (Granot et al., 2012). These possible time-markers are expected to be helpful for plate reconstructions during the CNS. Other superchrons during the Phanerozoic have also been actively discussed, of which the CNS is the most well-studied. The CNS is generally considered a consequence of the thermal effects of mantle activity on the outer core (e.g., McFadden and Merrill, 1984). Superchrons may result from the geodynamo operating at peak efficiency with a unique heat flux at the core-mantle boundary (CMB), as suggested by geodynamo numerical simulations and paleointensities estimated from single silicate crystals (Tarduno et al., 2006).
From the 1960s, terrestrial paleomagnetic data and MMA began to reveal periods of no or fewer reversals. By summarizing published paleomagnetic measurements of igneous and sedimentary rocks at more than 35 sites in North America and elsewhere, Helsley and Steiner (1968) found that there was a period when normal magnetic polarity was dominant for at least 25 million years (Myr) in the Cretaceous. Between ∼150 Ma and ∼110 Ma, Larson and Chase (1972) revealed that there are reversal periods sandwiched between periods of normal polarity (KQZ and the Jurassic Quiet Zone, JQZ). In fact, during the JQZ, geomagnetic reversals were frequent and the geomagnetic intensity was weak, as shown by MMA (e.g., Tominaga et al., 2021) and the absolute paleomagnetic intensity (paleointensity) of volcanic rocks (e.g., Tauxe et al., 2013). Larson and Pitman (1972) pointed out that the CNS corresponded in time to the KQZ of previous studies (e.g., Helsley and Steiner, 1968), designating C34n as the KQZ.
Here, I introduce a short reversal event that may exist during the CNS. Ryan et al. (1978) summarized three events or clusters of brief reversed polarity during the CNS that have been reported from drill cores, particularly in deep-sea sediments: (1) Late Aptian Chron M″-1r″, referred to as the ISEA event (Tarduno, 1990); (2) the Chron M″-2r″ event group during the mid-Albian; and, (3) the Chron M″-3r″ event group in the late Albian. The details of these short events are enigmatic because they have not been resolved in surveys of coeval MMA (Ogg, 2020). The paleomagnetic directions of possible ISEA event have also been found from Chinese lavas (Rao and Rao, 1996; Zhu et al., 2004a; Shi et al., 2004). The name of the ISEA event comes from the name of a section of the Umbria Apennines in northern Italy (VandenBerg et al., 1978). Furthermore, a recent study reports multiple geomagnetic reversals during the CNS (Zhang et al., 2021). Using paleomagnetic direction and U-Pb ages, they conclude that samples from two sections of Laos, and other magnetostratigraphy from previous studies indicate that there are at least five global and two single reversals during the CNS. However, no reversals such as the ISEA event have been found from deep-tow observations of MMA around the Atlantic Ocean (Granot et al., 2012). The altitude from the seafloor of deep-tow observations and the slow rate of seafloor spreading around the Atlantic Ocean may prevent the detection of such events shorter than 0.1 Myr (Granot et al., 2012).
Even if we consider potential short geomagnetic reversal events, the geomagnetic reversal frequency during the CNS is likely to be significantly lower than in other periods (Figure 1A). For example, if 6 to 14 reversals occurred during the CNS (37.3 Myr, Ogg, 2020), a simple calculation gives a reversal frequency range of 0.16–0.37/Myr for the period. In Constable (2000), the reversal frequency appears to be mainly above 1/Myr for all ages except the CNS. Considering them, perhaps we should call the KQZ period the “Cretaceous Reversal Minimum” rather than the CNS.
FIGURE 1. (A) Geomagnetic polarity time scale for 0–154.9 Ma based on Ogg (2020) and geomagnetic reversal frequency curve calculated using fixed kernel density estimation (Constable, 2000). Possible short geomagnetic reversal events during the CNS are shown as dashed white lines based on the summary of Zhang et al. (2021). Note that the duration of the events are not reflected in this figure. Constable (2000) calculated the reversal frequency from Harland et al. (1990) and Cande and Kent (1995). Pink zone expresses a range of the geomagnetic reversal frequency during the CNS (Chron C34n) calculated from short geomagnetic reversal events during the Superchron suggested by Zhang et al. (2021). Dotted gray lines express a time interval of the CNS. The CNS, Cretaceous Normal Superchron. (B) Virtual (axial) dipole moments during the CNS (Cottrell and Tarduno, 2000; Pick and Tauxe, 1993; Juarez et al., 1998; Thomas et al., 2000; Riisager et al., 2001; Tarduno et al., 2001; Tanaka and Kono, 2002; Tarduno et al., 2002; Zhu et al., 2002; Riisager et al., 2003; Tauxe and Staudigel, 2004; Zhu et al., 2004a; Zhu et al., 2004b; Zhao et al., 2004; Shi et al., 2005; Tauxe, 2006; Granot et al., 2007; Shcherbakova et al., 2007; Shcherbakova et al., 2008; Zhu et al., 2008; Shcherbakova et al., 2009; Tsunakawa et al., 2009; Qin et al., 2011; Shcherbakova et al., 2011; Shcherbakova et al., 2012; Di Chiara et al., 2021). The long-term median value and the medians of 5 Myr bins for the last 200 Myr by Tauxe et al. (2013) and Tauxe and Yamazaki (2015) are displayed by the solid blue line and the blue stars, respectively. The present field intensity is indicated by the solid gray line.
It has been investigated whether the paleointensity during the CNS is strong or weak because a strong geomagnetic intensity may have suppressed geomagnetic reversals (Cox, 1968). However, while the geomagnetic reversal frequency during the CNS is relatively well understood from the geomagnetic polarity time scale (e.g., Constable, 2000), the paleointensity during the CNS remains ambiguous.
I summarize previous paleointensities during the CNS of submarine basaltic glass (SBG) (Pick and Tauxe, 1993; Selkin and Tauxe, 2000; Riisager et al., 2003; Tauxe and Staudigel, 2004; Tauxe, 2006; Di Chiara et al., 2021), subaerial volcanic whole rocks (Thomas et al., 2000; Riisager et al., 2001; Tanaka and Kono, 2002; Zhu et al., 2002; Zhu et al., 2004a, 2004b; Zhao et al., 2004; Shi et al., 2005; Shcherbakova et al., 2007; Shcherbakova et al., 2008; Zhu et al., 2008; Qin et al., 2011; Shcherbakova et al., 2011; Shcherbakova et al., 2012), baked contact (Shcherbakova et al., 2008; Shcherbakova et al., 2009), and gabbro (Granot et al., 2007). Virtual (axial) dipole moments (V(A)DMs) in the CNS have been reported from 1.1 to 19.9 × 1022 Am2 (Figure 1B). The average of these paleointensities is 5.6 ± 3.2 × 1022 Am2 (140 sites), which is stronger than ∼4 × 1022 Am2 recently reported as reliable time-averaged V(A)DMs for other periods (Yamamoto and Tsunakawa, 2005; Yamazaki and Yamamoto, 2014; Ahn et al., 2016; Yoshimura et al., 2020), and the median V(A)DM of 4.2 × 1022 Am2 for the past 160 million years (Tauxe et al., 2013). However, this average value is weaker than the paleointensity of granite (∼9 × 1022 Am2) (Tsunakawa et al., 2009; Kato et al., 2018), which average out paleosecular variations of paleointensity due to its slow cooling rate (e.g., Bono et al., 2019). The reported time-averaged dipole moments vary from study to study. Considering these results, perhaps the average geomagnetic intensity during the CNS varied with time. Besides, Granot et al. (2007) found quasi-cyclic paleointensity variations around 5.4 ± 2.0 × 1022 Am2. This paleointensity variability is consistent with the temporal changes in the amplitude of MMA during the CNS (Granot et al., 2012).
On the other hand, absolute paleointensities estimated from single plagioclase crystals from the CNS show a different pattern. Two coincident strong paleomagnetic intensities have been estimated from two flood basalts that erupted in the CNS, 12.5 ± 1.4 × 1022 Am2 (Tarduno et al., 2001) and 12.7 ± 0.7 × 1022 Am2 (Tarduno et al., 2002). They concluded that the average geomagnetic field strength of the CNS was stronger than the present strength, and its variability was small based on the two studies. This feature is consistent with the geodynamo simulation (Driscoll and Olson, 2011). If the strong average of the CNS is true, it could be caused by the large heat flux heterogeneity of the CMB with equatorial symmetry (Takahashi et al., 2008).
I propose that there is a possibility that the number of paleomagnetic units used for time-averaging is insufficient. Tauxe and Staudigel (2004) presented the minimum number as 25 sampling sites required to average the paleosecular variations (PSVs) of paleointensity. Based on this required number of paleointensities, the studies using single plagioclase crystals lack the number of paleointensities required for the average PSVs. A sufficient time span is also required to average out PSVs. Future studies should estimate a lot of paleointensities using single plagioclase crystals with a sufficient time span, and test whether time-averaged paleointensity during the CNS is strong or weak.
The primary characteristic of the CNS is the dearth of geomagnetic reversals. It is essential to investigate the PSVs during this period because it may have been a different style of PSVs compared to other periods when reversals are relatively common. The analysis of PSV is usually analyzed by collecting virtual geomagnetic poles from a large number of lava flows and using dispersion curves of the virtual geomagnetic poles, in which the angular dispersion of the poles is plotted against the paleolatitude of the samples. McFadden et al. (1991) reported that there are apparently large differences in the dispersion curves of virtual geomagnetic poles at different times of the mean geomagnetic reversal frequency over the past 195 million years. They found that the VGP scatter tends to be lower at low paleolatitudes during periods of low reversal frequency than during periods of high reversal frequency and that the scatter significantly increases with high paleolatitude in the former compared to the latter. In other words, during periods of low reversal frequency, the slope of the dispersion curve of the virtual geomagnetic pole is larger than during periods of high reversal frequency. However, it has been reported that the slope of the dispersion curve is significantly smaller than McFadden’s result based on more reliable research results (Tarduno et al., 2002; Biggin et al., 2008; Doubrovine et al., 2019).
The mid-Cretaceous had a warm climate, with carbon dioxide concentrations of ∼1,000 ppm, more than twice the present-day levels (Foster et al., 2017). The sea level of 160–85 Ma was the highest (>150 m) during the Phanerozoic (van der Meer et al., 2017). There was a temperate rainforest in Antarctica during the Turonian-Santonian period (92–83 Ma) (Klages et al., 2020). Why did the warm climate of the mid-Cretaceous occur? Lee et al. (2013) proposed the hypothesis that the Cretaceous to Paleogene was a period when the continental arc was dominant and that the mid-Cretaceous greenhouse Earth was caused by the release of carbon dioxide into the atmosphere due to the interaction of ancient carbonates and magma in the continents. Brune et al. (2017) proposed that the length of the Cretaceous continental rift may have been a driving force for carbon dioxide release and warming, based on reconstructions of the length of the continental rift over the past 200 Myr and numerical carbon cycle models. Both hypotheses may have contributed to the warming of the mid-Cretaceous. On the other hand, Lee et al. (2013) explained that Large Igneous Provinces (LIPs) would have to erupt every 1 Myr for LIPs to cause the Cretaceous global warming, which is based on the response time of CO2 withdrawal from the exogenic system (<1 Myr) and the typical duration of LIPs (<2 Myr). However, it has recently been found that there were some LIPs with long eruption durations that lasted for tens of millions of years during the CNS (Dockman et al., 2018; Jiang et al., 2021). In addition, Johansson et al. (2018) suggested that there is a link between the timing of LIPs eruptions around 90–50 Ma and the increase in atmospheric CO2. Therefore, it is possible that the activities of LIPs had an influence on the warm climate during the mid-Cretaceous.
LIPs are massive volcanic complex formed by the eruption or intrusion of giant, mainly mafic magmas, which was not formed by the spreading or subduction of the ocean floor (Coffin and Eldholm, 1994). On the continent, it is often called a continental flood basalt, and on the ocean floor, it is called an oceanic plateau. At the time of the CNS, the eruption of LIPs was frequent (Figures 2A,B). This has been termed the “pulse” during the mid-Cretaceous by Larson (1995), and discussions of its relevance to the effects on the geomagnetic field through the CMB, as indicated by the occurrence of the CNS, have been continued (Courtillot and Olson, 2007; Biggin et al., 2012; Olson and Amit, 2015). Based on geochronological data, the LIPs known to have erupted during the CNS period include the Ontong-Java Plateau (Mahoney et al., 1993; Timm et al., 2011), the Kerguelen Plateau (Duncan, 2002; Jiang et al., 2021), Rajmahal, Bengal, Sylhet Traps (Coffin et al., 2002; Kent et al., 2002; Ray et al., 2005), the High Arctic LIP (Dockman et al., 2018), the Caribbean LIP (Serrano et al., 2011), Madagascan flood basalts (Storey et al., 1995; Cucciniello et al., 2021), the Agulhas Plateau, the Northeast Georgia and Maud Rise (Parsiegla et al., 2008), and the Hess Rise (Pringle and Dalrymple, 1993). It is interesting to note that volcanism in China (Zhu et al., 2008), production of granite (Yokoyama et al., 2016), and kimberlites (Griffin et al., 2014) were active during the CNS. Besides, seamount volcanism was active in the Western Pacific during the Cretaceous of 115–60 Ma (Koppers et al., 2003). What they show is the fact that mantle convection was unusually active during the CNS. East et al. (2020) proposed that high subduction fluxes before the CNS caused the mantle return flow, which increased the activity of LIPs.
FIGURE 2. (A,B) Distribution of Large Igneous Provinces at 120 Ma (A) and 84 Ma (B) with paleogeography based on Johansson et al. (2018), which erupted during the CNS. LIPs, Large Igneous Provinces. (C,D) Two hypotheses for the cause of the CNS. (C) Equatorial cross-sections of the core (red and orange) and lower mantle (green) illustrating superplume growth and collapse in the D″-region (light green) above the core-mantle boundary based on Olson and Amit (2015). Arrows indicate the lower mantle circulation patterns. Superplume growth thins the D″-region below lower mantle downwelling, which increases the heat flux average at the core-mantle boundary. The geodynamo expresses a higher geomagnetic reversal frequency at that time. On the contrary, superplume collapses reduces the heat flux average at the core-mantle boundary and reduce reversal frequency, which is Superchron. (D) Cross-sections of the core (red and orange) and lower mantle (green) illustrating changing of the subduction flux (the volume of slabs) based on Hounslow et al. (2018), which reaches the D″ layer (light green) above the core-mantle boundary. Higher subduction flux increases the volume of slabs reaching the D″ layer, increases the heat flux average at the core-mantle boundary, and increases geomagnetic reversal frequency. On the contrary, lower subduction flux reduces the volume of slabs reaching the D″ layer, reduces the heat flux average at the core-mantle boundary, and reduces reversal frequency, which is Superchron.
The cause of the CNS is an open question in earth science. The influence of mantle convection has long been considered as a cause of the CNS (McFadden and Merrill, 1984; Larson, 1991; Larson and Olson, 1991; Larson, 1995; Glatzmaier et al., 1999; Courtillot and Olson, 2007; Olson et al., 2010; Amit and Olson, 2015; Olson and Amit, 2015). During the CNS, there was a lot of volcanic activity and seafloor production. These activities imply vigorous mantle convection, which could have affected the outer core. The numerical geodynamo simulation predicts a minimum heat flux at the CMB during the CNS (Olson et al., 2010), while the mantle convection simulation predicts a maximum heat flux (Zhang and Zhong, 2011). This is a contradiction.
Changes in the CMB heat flux due to growth and collapse of the superplume were proposed as the cause of the CNS, which can resolve the above contradiction (Olson and Amit, 2015) (Figure 2C). When superplumes (they referred it as Large low-shear-velocity provinces, LLSVP) grow, the D″ layer is pulled down by superplumes and becomes thinner. Conversely, when superplumes collapse, the original shortage returns to the D″ layer and thickens it. The thicker the D″ layer, the harder it is for slabs to affect the outer core thermally, which reduces geomagnetic reversals. On the other hand, as it becomes thinner, the outer core becomes more strongly influenced by the slabs, and geomagnetic reversals increase. The change in the thickness of the D″ layer is predicted by viscose fluids experiments (Olson and Kincaid, 1991) and numerical thermochemical convection simulation (Li et al., 2018). When a superplume collapses, a hot plume is thought to be generated from its edge (Steinberger and Torsvik, 2012). Olson and Amit (2015) argued that it was a reasonable scenario because the time-lag (30–60 Myr) that the hot plume reaches the lithosphere and erupts LIPs is consistent with the time lag between the geomagnetic reversal frequency and the frequency of LIPs. Such correlation was also pointed out by Biggin et al. (2012). However, can the shape of the superplume (LLSVP) and D″ layer variable with time? Whether the shape of LLSVP is vertically variable or remains stable is still inconclusive (Garnero et al., 2016).
Another candidate for the cause of the CNS is the change in subduction rates (Figure 2D). Hounslow et al. (2018) found a 120 Myr time lag between the subduction flux and the geomagnetic reversal frequency. This time lag is intermediate between the seismologically expected long time lag for the subducted slab to reach the CMB from the surface (∼150–300 Ma) and the short time lag predicted by numerical mantle convection models (∼30–60 Ma), which may be real value. More recently, Williams et al. (2021) found that the two peaks of oceanic heat flow were roughly consistent with the onset of two superchrons (CNS and the Permian-Carboniferous Reversed Superchron), respectively. These results are consistent with Hounslow et al. (2018). In summary, the subduction flux may control the reversal frequency. This means that the occurrence of the CNS may be due to low subduction flux.
Why do subduction fluxes vary so widely and periodically on a scale of tens of millions of years? Based on the relationship between the subduction zone lengths and the convergence rates (Ruff and Kanamori, 1980), the long subduction zone would have accelerated the plate spreading rate since 180 Ma. Or, as an exotic idea, a true polar wander (TPW) might change the regime of mantle convection and affect its convection speed. In addition, when the spreading rate of the mid-ocean ridge becomes high like around the CNS, the density and temperature of the subducting plate are lower and hotter than in other periods. In the future, we will also have to consider the effect of this hot and low-density plate subduction on mantle convection. I think it is more likely that a relatively hot slab would reach the CMB but not cool it, which decreases the heat flux average at the CMB, which may also cause the CNS. The slab effect on the CMB must be tested by the slab-sinking model.
The small CMB heat flux would cause the CNS. The mantle convection was active during the CNS and may have affected the geomagnetic field and the Earth’s environment. Future studies need to elucidate the effects of active mantle convection on the CMB.
YY designed the research and prepared the manuscript.
This work was supported by R3QR Program (Qdai-jump Research Program) 01265, “Wakaba Challenge” of Kyushu University.
The author declares that the research was conducted in the absence of any commercial or financial relationships that could be construed as a potential conflict of interest.
All claims expressed in this article are solely those of the authors and do not necessarily represent those of their affiliated organizations, or those of the publisher, the editors and the reviewers. Any product that may be evaluated in this article, or claim that may be made by its manufacturer, is not guaranteed or endorsed by the publisher.
I appreciate Masakazu Fujii, Roi Granot, Chie Kato, Joseph Kirschvink, Hironao Matsumoto, Kyoko Okino, Masahiko Sato, and Toshitsugu Yamazaki for discussion. I thank Catherine Constable and Lisa Tauxe for providing data. I also thank Adam Sproson for English language editing. The manuscript was greatly improved by constructive comments from Peter Driscoll and the Associate Editor Yohan Guyodo.
Ahn, H.-S., Kidane, T., Yamamoto, Y., and Otofuji, Y.-i. (2016). Low Geomagnetic Field Intensity in the Matuyama Chron: Palaeomagnetic Study of a Lava Sequence from Afar Depression, East Africa. Geophys. J. Int. 204 (1), 127–146. doi:10.1093/gji/ggv303
Amit, H., and Olson, P. (2015). Lower Mantle Superplume Growth Excites Geomagnetic Reversals. Earth Planet. Sci. Lett. 414, 68–76. doi:10.1016/j.epsl.2015.01.013
Ballmer, M. D., Schmerr, N. C., Nakagawa, T., and Ritsema, J. (2015). Compositional Mantle Layering Revealed by Slab Stagnation at ∼1000-km Depth. Sci. Adv. 1 (11), e1500815. doi:10.1126/sciadv.1500815
Biggin, A. J., Steinberger, B., Aubert, J., Suttie, N., Holme, R., Torsvik, T. H., et al. (2012). Possible Links between Long-Term Geomagnetic Variations and Whole-Mantle Convection Processes. Nat. Geosci 5 (8), 526–533. doi:10.1038/ngeo1521
Biggin, A. J., and Thomas, D. N. (2003). Analysis of Long-Term Variations in the Geomagnetic Poloidal Field Intensity and Evaluation of Their Relationship with Global Geodynamics. Geophys. J. Int. 152 (2), 392–415. doi:10.1046/j.1365-246x.2003.01849.x
Biggin, A. J., van Hinsbergen, D. J., Langereis, C. G., Straathof, G. B., and Deenen, M. H. (2008). Geomagnetic Secular Variation in the Cretaceous Normal Superchron and in the Jurassic. Phys. Earth Planet. Interiors 169 (1-4), 3–19. doi:10.1016/j.pepi.2008.07.004
Bono, R. K., Tarduno, J. A., Nimmo, F., and Cottrell, R. D. (2019). Young Inner Core Inferred from Ediacaran Ultra-low Geomagnetic Field Intensity. Nat. Geosci 12 (2), 143–147. doi:10.1038/s41561-018-0288-0
Brune, S., Williams, S. E., and Müller, R. D. (2017). Potential Links between continental Rifting, CO2 Degassing and Climate Change through Time. Nat. Geosci 10 (12), 941–946. doi:10.1038/s41561-017-0003-6
Cande, S. C., and Kent, D. V. (1995). Revised Calibration of the Geomagnetic Polarity Timescale for the Late Cretaceous and Cenozoic. J. Geophys. Res. 100 (B4), 6093–6095. doi:10.1029/94jb03098
Coffin, M. F., and Eldholm, O. (1994). Large Igneous Provinces: Crustal Structure, Dimensions, and External Consequences. Rev. Geophys. 32 (1), 1–36. doi:10.1029/93rg02508
Coffin, M. F., Pringle, M. S., Duncan, R. A., Gladczenko, T. P., Storey, M., Müller, R. D., et al. (2002). Kerguelen Hotspot Magma Output since 130 Ma. J. Petrol. 43 (7), 1121–1137. doi:10.1093/petrology/43.7.1121
Constable, C. (2000). On Rates of Occurrence of Geomagnetic Reversals. Phys. Earth Planet. Interiors 118 (3-4), 181–193. doi:10.1016/s0031-9201(99)00139-9
Cottrell, R. D., and Tarduno, J. A. (2000). In Search of High‐Fidelity Geomagnetic Paleointensities: A Comparison of Single Plagioclase Crystal and Whole Rock Thellier‐Thellier Analyses. Journal of Geophysical Research: Solid Earth 105, 23579–23594. doi:10.1029/2000JB900219
Courtillot, V., and Olson, P. (2007). Mantle Plumes Link Magnetic Superchrons to Phanerozoic Mass Depletion Events. Earth Planet. Sci. Lett. 260 (3-4), 495–504. doi:10.1016/j.epsl.2007.06.003
Cox, A. (1968). Lengths of Geomagnetic Polarity Intervals. J. Geophys. Res. 73 (10), 3247–3260. doi:10.1029/jb073i010p03247
Cucciniello, C., Morra, V., Melluso, L., and Jourdan, F. (2021). Constraints on Duration, Age and Migration of the Feeder Systems of the Madagascan Flood basalt Province from High-Precision 40Ar/39Ar Chronology. London: Geological SocietySpecial Publications, 518.
Di Chiara, A., Tauxe, L., Staudigel, H., Florindo, F., Protti, M., Yu, Y., et al. (2021). Earth's Magnetic Field Strength and the Cretaceous Normal Superchron: New Data from Costa Rica. Geochem. Geophys. Geosystems 22, e2020GC009605. doi:10.1029/2020gc009605
Dockman, D. M., Pearson, D. G., Heaman, L. M., Gibson, S. A., and Sarkar, C. (2018). Timing and Origin of Magmatism in the Sverdrup Basin, Northern Canada-Implications for Lithospheric Evolution in the High Arctic Large Igneous Province (HALIP). Tectonophysics 742-743, 50–65. doi:10.1016/j.tecto.2018.05.010
Doubrovine, P. V., Veikkolainen, T., Pesonen, L. J., Piispa, E., Ots, S., Smirnov, A. V., et al. (2019). Latitude Dependence of Geomagnetic Paleosecular Variation and its Relation to the Frequency of Magnetic Reversals: Observations from the Cretaceous and Jurassic. Geochem. Geophys. Geosyst. 20 (3), 1240–1279. doi:10.1029/2018gc007863
Driscoll, P., and Olson, P. (2011). Superchron Cycles Driven by Variable Core Heat Flow. Geophys. Res. Lett. 38 (9), L09304. doi:10.1029/2011gl046808
Duncan, R. A. (2002). A Time Frame for Construction of the Kerguelen Plateau and Broken Ridge. J. Pet. 43 (7), 1109–1119. doi:10.1093/petrology/43.7.1109
East, M., Müller, R. D., Williams, S., Zahirovic, S., and Heine, C. (2020). Subduction History Reveals Cretaceous Slab Superflux as a Possible Cause for the Mid-cretaceous Plume Pulse and Superswell Events. Gondwana Res. 79, 125–139. doi:10.1016/j.gr.2019.09.001
Foster, G. L., Royer, D. L., and Lunt, D. J. (2017). Future Climate Forcing Potentially without Precedent in the Last 420 Million Years. Nat. Commun. 8 (1), 14845–14848. doi:10.1038/ncomms14845
Garnero, E. J., McNamara, A. K., and Shim, S.-H. (2016). Continent-sized Anomalous Zones with Low Seismic Velocity at the Base of Earth's Mantle. Nat. Geosci 9 (7), 481–489. doi:10.1038/ngeo2733
Glatzmaier, G. A., Coe, R. S., Hongre, L., and Roberts, P. H. (1999). The Role of the Earth's Mantle in Controlling the Frequency of Geomagnetic Reversals. Nature 401 (6756), 885–890. doi:10.1038/44776
Granot, R., Dyment, J., and Gallet, Y. (2012). Geomagnetic Field Variability during the Cretaceous Normal Superchron. Nat. Geosci 5 (3), 220–223. doi:10.1038/ngeo1404
Granot, R., Tauxe, L., Gee, J. S., and Ron, H. (2007). A View into the Cretaceous Geomagnetic Field from Analysis of Gabbros and Submarine Glasses. Earth Planet. Sci. Lett. 256 (1-2), 1–11. doi:10.1016/j.epsl.2006.12.028
Griffin, W. L., Batumike, J. M., Greau, Y., Pearson, N. J., Shee, S. R., and O’Reilly, S. Y. (2014). Emplacement Ages and Sources of Kimberlites and Related Rocks in Southern Africa: U–Pb Ages and Sr–Nd Isotopes of Groundmass Perovskite. Contrib. Mineralogy Pet. 168 (1), 1–13. doi:10.1007/s00410-014-1032-4
Harland, W. B., Armstrong, R. L., Cox, A. V., Craig, L. E., Smith, A. G., and Smith, D. G. (1990). A Geologic Time Scale 1989. Cambridge University Press.
Heller, R., Merrill, R. T., and McFadden, P. L. (2002). The Variation of Intensity of Earth’s Magnetic Field with Time. Phys. Earth Planet. Interiors 131 (3-4), 237–249. doi:10.1016/s0031-9201(02)00038-9
Helsley, C. E., and Steiner, M. B. (1968). Evidence for Long Intervals of normal Polarity during the Cretaceous Period. Earth Planet. Sci. Lett. 5, 325–332. doi:10.1016/s0012-821x(68)80060-3
Hounslow, M. W., Domeier, M., and Biggin, A. J. (2018). Subduction Flux Modulates the Geomagnetic Polarity Reversal Rate. Tectonophysics 742-743, 34–49. doi:10.1016/j.tecto.2018.05.018
Jiang, Q., Jourdan, F., Olierook, H. K. H., Merle, R. E., and Whittaker, J. M. (2021). Longest Continuously Erupting Large Igneous Province Driven by Plume-ridge Interaction. Geology 49 (2), 206–210. doi:10.1130/g47850.1
Johansson, L., Zahirovic, S., and Müller, R. D. (2018). The Interplay between the Eruption and Weathering of Large Igneous Provinces and the Deep‐Time Carbon Cycle. Geophys. Res. Lett. 45 (11), 5380–5389. doi:10.1029/2017gl076691
Juárez, M. T., Tauxe, L., Gee, J. S., and Pick, T. (1998). The Intensity of the Earth's Magnetic Field over the Past 160 Million Years. Nature 394 (6696), 878–881. doi:10.1038/29746
Kato, C., Sato, M., Yamamoto, Y., Tsunakawa, H., and Kirschvink, J. L. (2018). Paleomagnetic Studies on Single Crystals Separated from the Middle Cretaceous Iritono Granite. Earth, Planets and Space 70 (1), 1–19. doi:10.1186/s40623-018-0945-y
Kent, R. W., Pringle, M. S., Müller, R. D., Saunders, A. D., and Ghose, N. C. (2002). 40Ar/39Ar Geochronology of the Rajmahal Basalts, India, and Their Relationship to the Kerguelen Plateau. J. Pet. 43 (7), 1141–1153. doi:10.1093/petrology/43.7.1141
Klages, J. P., Salzmann, U., Salzmann, U., Bickert, T., Hillenbrand, C.-D., Gohl, K., et al. (2020). Temperate Rainforests Near the South Pole during Peak Cretaceous Warmth. Nature 580 (7801), 81–86. doi:10.1038/s41586-020-2148-5
Koppers, A. A., Staudigel, H., Pringle, M. S., and Wijbrans, J. R. (2003). Short‐lived and Discontinuous Intraplate Volcanism in the South Pacific: Hot Spots or Extensional Volcanism. Geochem. Geophys. Geosystems 4 (10), 1089. doi:10.1029/2003gc000533
Larson, R. L., and Chase, C. G. (1972). Late Mesozoic Evolution of the Western Pacific Ocean. Geol. Soc. America Bull. 83 (12), 3627–3644. doi:10.1130/0016-7606(1972)83[3627:lmeotw]2.0.co;2
Larson, R. L. (1991). Geological Consequences of Superplumes. Geol 19 (10), 963–966. doi:10.1130/0091-7613(1991)019<0963:gcos>2.3.co;2
Larson, R. L., and Olson, P. (1991). Mantle Plumes Control Magnetic Reversal Frequency. Earth Planet. Sci. Lett. 107 (3-4), 437–447. doi:10.1016/0012-821x(91)90091-u
Larson, R. L., and Pitman, W. C. (1972). World-wide Correlation of Mesozoic Magnetic Anomalies, and its Implications. Geol. Soc. America Bull. 83 (12), 3645–3662. doi:10.1130/0016-7606(1972)83[3645:wcomma]2.0.co;2
Larson, R. L. (1995). The Mid-cretaceous Superplume Episode. Sci. Am. 272 (2), 82–86. doi:10.1038/scientificamerican0295-82
Lee, C.-T. A., Shen, B., Slotnick, B. S., Liao, K., Dickens, G. R., Yokoyama, Y., et al. (2013). Continental Arc-Island Arc Fluctuations, Growth of Crustal Carbonates, and Long-Term Climate Change. Geosphere 9 (1), 21–36. doi:10.1130/ges00822.1
Li, M., Zhong, S., and Olson, P. (2018). Linking Lowermost Mantle Structure, Core-Mantle Boundary Heat Flux and Mantle Plume Formation. Phys. Earth Planet. Interiors 277, 10–29. doi:10.1016/j.pepi.2018.01.010
Mahoney, J. J., Storey, M., Duncan, R. A., Spencer, K. J., and Pringle, M. (1993). “Geochemistry and Geochronology of Leg 130 Basement Lavas: Nature and Origin of the Ontong Java Plateau,” in Proceedings of the Ocean Drilling Program, Scientific Results, Texas A&M University, College Station 130, 3–22.
McFadden, P. L., and Merrill, R. T. (1984). Lower Mantle Convection and Geomagnetism. J. Geophys. Res. 89 (B5), 3354–3362. doi:10.1029/jb089ib05p03354
McFadden, P. L., Merrill, R. T., McElhinny, M. W., and Lee, S. (1991). Reversals of the Earth's Magnetic Field and Temporal Variations of the Dynamo Families. J. Geophys. Res. 96 (B3), 3923–3933. doi:10.1029/90jb02275
Ogg, J. G. (2020). “Geomagnetic Polarity Time Scale,” in Geologic Time Scale 2020 (Elsevier), 159–192. doi:10.1016/b978-0-12-824360-2.00005-x
Olson, P., and Amit, H. (2015). Mantle Superplumes Induce Geomagnetic Superchrons. Front. Earth Sci. 3, 38. doi:10.3389/feart.2015.00038
Olson, P., and Kincaid, C. (1991). Experiments on the Interaction of thermal Convection and Compositional Layering at the Base of the Mantle. J. Geophys. Res. 96 (B3), 4347–4354. doi:10.1029/90jb02530
Olson, P. L., Coe, R. S., Driscoll, P. E., Glatzmaier, G. A., and Roberts, P. H. (2010). Geodynamo Reversal Frequency and Heterogeneous Core–Mantle Boundary Heat Flow. Phys. Earth Planet. Interiors 180 (1-2), 66–79. doi:10.1016/j.pepi.2010.02.010
Parsiegla, N., Gohl, K., and Uenzelmann-Neben, G. (2008). The Agulhas Plateau: Structure and Evolution of a Large Igneous Province. Geophys. J. Int. 174 (1), 336–350. doi:10.1111/j.1365-246x.2008.03808.x
Pick, T., and Tauxe, L. (1993). Geomagnetic Palaeointensities during the Cretaceous normal Superchron Measured Using Submarine Basaltic Glass. Nature 366 (6452), 238–242. doi:10.1038/366238a0
Pringle, M. S., and Dalrymple, G. B. (1993). Geochronological Constraints on a Possible Hot Spot Origin for Hess Rise and the Wentworth Seamount Chain, 77. Washington DC American Geophysical Union Geophysical Monograph Series, 263–277. doi:10.1029/gm077p0263
Qin, H., He, H., Liu, Q., and Cai, S. (2011). Palaeointensity Just at the Onset of the Cretaceous Normal Superchron. Phys. Earth Planet. Interiors 187 (3-4), 199–211. doi:10.1016/j.pepi.2011.05.009
Rao, G. V. S. P., and Rao, J. M. (1996). Palaeomagnetism of the Rajmahal Traps of India: Implication to the Reversal in the Cretaceous Normal Superchron. J. Geomagn. Geoelec 48 (7), 993–1000. doi:10.5636/jgg.48.993
Ray, J. S., Pattanayak, S. K., and Pande, K. (2005). Rapid Emplacement of the Kerguelen Plume–Related Sylhet Traps, Eastern India: Evidence from 40Ar‐39Ar Geochronology. Geophys. Res. Lett. 32 (10), L10303. doi:10.1029/2005gl022586
Riisager, J., Perrin, M., Riisager, P., and Vandamme, D. (2001). Palæomagnetic Results and Palæointensity of Late Cretaceous Madagascan basalt. J. Afr. Earth Sci. 32 (3), 503–518. doi:10.1016/s0899-5362(01)90111-3
Riisager, P., Riisager, J., Zhao, X., and Coe, R. S. (2003). Cretaceous Geomagnetic Paleointensities: Thellier Experiments on Pillow Lavas and Submarine Basaltic Glass from the Ontong Java Plateau. Geochem. Geophys. Geosystems 4 (12), 8803. doi:10.1029/2003gc000611
Ruff, L., and Kanamori, H. (1980). Seismicity and the Subduction Process. Phys. Earth Planet. interiors 23 (3), 240–252. doi:10.1016/0031-9201(80)90117-x
Ryan, W. B., Bolli, H. M., Foss, G. N., Natland, J. H., Hottman, W. E., and Foresman, J. B. (1978). Objectives, Principal Results, Operations and Explanatory Notes of Leg 40, South Atlantic, 40. Washington, DC: Initial reports of the deep sea drilling project, 5–28. doi:10.2973/dsdp.proc.40.101.1978
Selkin, P. A., and Tauxe, L. (2000). Long-term Variations in Palaeointensity. Philos. Trans. R. Soc. Lond. Ser. A: Math. Phys. Eng. Sci. 358 (1768), 1065–1088. doi:10.1098/rsta.2000.0574
Serrano, L., Ferrari, L., Martínez, M. L., Petrone, C. M., and Jaramillo, C. (2011). An Integrative Geologic, Geochronologic and Geochemical Study of Gorgona Island, Colombia: Implications for the Formation of the Caribbean Large Igneous Province. Earth Planet. Sci. Lett. 309 (3-4), 324–336. doi:10.1016/j.epsl.2011.07.011
Shcherbakova, V. V., Asanidze, B. Z., Shcherbakov, V. P., and Zhidkov, G. V. (2007). Geomagnetic Field Paleointensity in the Cretaceous from Upper Cretaceous Rocks of Georgia. Izv., Phys. Solid Earth 43 (11), 951–959. doi:10.1134/s1069351307110055
Shcherbakova, V. V., Bakhmutov, V. G., Shcherbakov, V. P., Zhidkov, G. V., and Shpyra, V. V. (2012). Palaeointensity and Palaeomagnetic Study of Cretaceous and Palaeocene Rocks from Western Antarctica. Geophys. J. Int. 189 (1), 204–228. doi:10.1111/j.1365-246x.2012.05357.x
Shcherbakova, V. V., Kovalenko, D. V., Shcherbakov, V. P., and Zhidkov, G. V. (2011). Paleointensity of the Geomagnetic Field in the Cretaceous (From Cretaceous Rocks of Mongolia). Izv., Phys. Solid Earth 47 (9), 775–791. doi:10.1134/s1069351311070081
Shcherbakova, V. V., Perrin, M., Shcherbakov, V. P., Pavlov, V. E., Ayvaz’yan, A., and Zhidkov, G. V. (2009). Rock Magnetic and Paleointensity Results from Mesozoic Baked Contacts of Armenia. Earth Planet. Sp 61 (1), 23–39. doi:10.1186/bf03352882
Shcherbakova, V. V., Zhidkov, G. V., and Shcherbakov, V. P. (2008). Revised Determination of the Paleointensity in the Cretaceous from the Collection of A.S. Bol'shakov and G.M. Solodovnikov. Izv., Phys. Solid Earth 44 (10), 816–821. doi:10.1134/s1069351308100091
Shi, R., He, H., Zhu, R., and Pan, Y. (2004). ISEA Reversed Event in the Cretaceous Normal Superchron (CNS):40Ar/39Ar Dating and Paleomagnetic Results. Chin. Sci. Bull. 49 (9), 926–930. doi:10.1007/bf03184013
Shi, R., Hill, M. J., Zhu, R., He, H., and Shaw, J. (2005). 40Ar/39Ar Dating and Preliminary Paleointensity Determination on a Single Lava Flow from Chifeng, Inner Mongolia. Phys. Earth Planet. interiors 152 (1-2), 78–89. doi:10.1016/j.pepi.2005.06.005
Steinberger, B., and Torsvik, T. H. (2012). A Geodynamic Model of Plumes from the Margins of Large Low Shear Velocity Provinces. Geochem. Geophys. Geosystems 13 (1). doi:10.1029/2011gc003808
Storey, M., Mahoney, J. J., Saunders, A. D., Duncan, R. A., Kelley, S. P., and Coffin, M. F. (1995). Timing of Hot Spot-Related Volcanism and the Breakup of Madagascar and India. Science 267 (5199), 852–855. doi:10.1126/science.267.5199.852
Takahashi, F., Tsunakawa, H., Matsushima, M., Mochizuki, N., and Honkura, Y. (2008). Effects of Thermally Heterogeneous Structure in the Lowermost Mantle on the Geomagnetic Field Strength. Earth Planet. Sci. Lett. 272 (3-4), 738–746. doi:10.1016/j.epsl.2008.06.017
Tanaka, H., and Kono, M. (2002). Paleointensities from a Cretaceous basalt Platform in Inner Mongolia, Northeastern China. Phys. Earth Planet. Interiors 133 (1-4), 147–157. doi:10.1016/s0031-9201(02)00091-2
Tarduno, J. A. (1990). Brief Reversed Polarity Interval during the Cretaceous normal Polarity Superchron. Geol 18 (8), 683–686. doi:10.1130/0091-7613(1990)018<0683:brpidt>2.3.co;2
Tarduno, J. A., Cottrell, R. D., and Smirnov, A. V. (2001). High Geomagnetic Intensity during the Mid-cretaceous from Thellier Analyses of Single Plagioclase Crystals. Science 291 (5509), 1779–1783. doi:10.1126/science.1057519
Tarduno, J. A., Cottrell, R. D., and Smirnov, A. V. (2002). The Cretaceous Superchron Geodynamo: Observations Near the tangent cylinder. Proc. Natl. Acad. Sci. 99 (22), 14020–14025. doi:10.1073/pnas.222373499
Tarduno, J. A., Cottrell, R. D., and Smirnov, A. V. (2006). The Paleomagnetism of Single Silicate Crystals: Recording Geomagnetic Field Strength during Mixed Polarity Intervals, Superchrons, and Inner Core Growth. Rev. Geophys. 44 (1), RG1002. doi:10.1029/2005rg000189
Tauxe, L., Gee, J. S., Steiner, M. B., and Staudigel, H. (2013). Paleointensity Results from the Jurassic: New Constraints from Submarine Basaltic Glasses of ODP Site 801C. Geochem. Geophys. Geosyst. 14 (10), 4718–4733. doi:10.1002/ggge.20282
Tauxe, L. (2006). Long-term Trends in Paleointensity: the Contribution of DSDP/ODP Submarine Basaltic Galss Collections. Phys. Earth Planet. Interiors 156 (3-4), 223–241. doi:10.1016/j.pepi.2005.03.022
Tauxe, L., and Staudigel, H. (2004). Strength of the Geomagnetic Field in the Cretaceous Normal Superchron: New Data from Submarine Basaltic Glass of the Troodos Ophiolite. Geochem. Geophys. Geosystems 5 (2). doi:10.1029/2003gc000635
Tauxe, L., and Yamazaki, T. (2015). “Paleointensities,” in Treatise on Geophysics. 2nd ed. (Elsevier), Vol. 5, 461–509. doi:10.1016/b978-0-444-53802-4.00107-x
Thomas, D. N., Biggin, A. J., and Schmidt, P. W. (2000). A Palaeomagnetic Study of Jurassic Intrusives from Southern New South Wales: Further Evidence for a Pre-cenozoic Dipole Low. Geophys. J. Int. 140 (3), 621–635. doi:10.1046/j.1365-246x.2000.00049.x
Timm, C., Hoernle, K., Werner, R., Hauff, F., van den Bogaard, P., Michael, P., et al. (2011). Age and Geochemistry of the Oceanic Manihiki Plateau, SW Pacific: New Evidence for a Plume Origin. Earth Planet. Sci. Lett. 304 (1-2), 135–146. doi:10.1016/j.epsl.2011.01.025
Tominaga, M., Tivey, M. A., and Sager, W. W. (2021). A New Middle to Late Jurassic Geomagnetic Polarity Time Scale (GPTS) From a Multiscale Marine Magnetic Anomaly Survey of the Pacific Jurassic Quiet Zone. Journal of Geophysical Research: Solid Earth 126 (3), e2020JB021136. doi:10.1029/2020JB021136
Tsunakawa, H., Wakabayashi, K. I., Mochizuki, N., Yamamoto, Y., Ishizaka, K., Hirata, T., et al. (2009). Paleointensity Study of the Middle Cretaceous Iritono Granite in Northeast Japan: Implication for High Field Intensity of the Cretaceous Normal Superchron. Phys. Earth Planet. Interiors 176 (3-4), 235–242. doi:10.1016/j.pepi.2009.07.001
Van der Meer, D. G., van den Berg van Saparoea, A. P. H., Van Hinsbergen, D. J. J., Van de Weg, R. M. B., Godderis, Y., Le Hir, G., et al. (2017). Reconstructing First-Order Changes in Sea Level during the Phanerozoic and Neoproterozoic Using Strontium Isotopes. Gondwana Res. 44, 22–34. doi:10.1016/j.gr.2016.11.002
VandenBerg, J., Klootwijk, C. T., and Wonders, A. A. H. (1978). Late Mesozoic and Cenozoic Movements of the Italian Peninsula: Further Paleomagnetic Data from the Umbrian Sequence. Geol. Soc. America Bull. 89 (1), 133–150. doi:10.1130/0016-7606(1978)89<133:lmacmo>2.0.co;2
Williams, S., Wright, N. M., Cannon, J., Flament, N., and Müller, R. D. (2021). Reconstructing Seafloor Age Distributions in Lost Ocean Basins. Geosci. Front. 12 (2), 769–780. doi:10.1016/j.gsf.2020.06.004
Yamamoto, Y., and Tsunakawa, H. (2005). Geomagnetic Field Intensity during the Last 5 Myr: LTD-DHT Shaw Palaeointensities from Volcanic Rocks of the Society Islands, French Polynesia. Geophys. J. Int. 162 (1), 79–114. doi:10.1111/j.1365-246x.2005.02651.x
Yamazaki, T., and Yamamoto, Y. (2014). Paleointensity of the Geomagnetic Field in the Late Cretaceous and Earliest Paleogene Obtained from Drill Cores of the Louisville Seamount Trail. Geochem. Geophys. Geosyst. 15 (6), 2454–2466. doi:10.1002/2014gc005298
Yokoyama, K., Shigeoka, M., Otomo, Y., Tokuno, K., and Tsutsumi, Y. (2016). Uraninite and Thorite Ages of Around 400 Granitoids in the Japanese Islands. Mem. Natl. Mus. Nat. Sci. 51, 1–24.
Yoshimura, Y., Yamazaki, T., Yamamoto, Y., Ahn, H. S., Kidane, T., and Otofuji, Y. (2020). Geomagnetic Paleointensity Around 30 Ma Estimated from Afro‐Arabian Large Igneous Province. Geochem. Geophys. Geosystems 21 (12), e2020GC009341. doi:10.1029/2020gc009341
Zhang, D., Yan, M., Song, C., Zhang, W., Fang, X., and Li, B. (2021). Frequent Polarity Reversals in the Cretaceous Normal Superchron. Geophys. Res. Lett. 48 (5), e2020GL091501. doi:10.1029/2020gl091501
Zhang, N., and Zhong, S. (2011). Heat Fluxes at the Earth's Surface and Core–Mantle Boundary since Pangea Formation and Their Implications for the Geomagnetic Superchrons. Earth Planet. Sci. Lett. 306 (3-4), 205–216. doi:10.1016/j.epsl.2011.04.001
Zhao, X., Riisager, P., Riisager, J., Draeger, U., Coe, R. S., and Zheng, Z. (2004). New Palaeointensity Results from Cretaceous basalt of Inner Mongolia, China. Phys. Earth Planet. Interiors 141 (2), 131–140. doi:10.1016/j.pepi.2003.12.003
Zhu, R., Hoffman, K. A., Nomade, S., Renne, P. R., Shi, R., Pan, Y., et al. (2004a). Geomagnetic Paleointensity and Direct Age Determination of the ISEA (M0r?) Chron. Earth Planet. Sci. Lett. 217 (3-4), 285–295. doi:10.1016/s0012-821x(03)00613-7
Zhu, R., Lo, C. H., Shi, R., Shi, G., Pan, Y., and Shao, J. (2004b). Palaeointensities Determined from the Middle Cretaceous basalt in Liaoning Province, Northeastern China. Phys. Earth Planet. Interiors 142 (1-2), 49–59. doi:10.1016/j.pepi.2003.12.013
Zhu, R., Pan, Y., He, H., Qin, H., and Ren, S. (2008). Palaeomagnetism and 40Ar/39Ar Age from a Cretaceous Volcanic Sequence, Inner Mongolia, China: Implications for the Field Variation during the Cretaceous Normal Superchron. Phys. Earth Planet. Interiors 169 (1-4), 59–75. doi:10.1016/j.pepi.2008.07.025
Keywords: cretaceous normal superchron, paleointensity, paleosecular variation, paleoenviroment, large igneous province (LIP)
Citation: Yoshimura Y (2022) The Cretaceous Normal Superchron: A Mini-Review of Its Discovery, Short Reversal Events, Paleointensity, Paleosecular Variations, Paleoenvironment, Volcanism, and Mechanism. Front. Earth Sci. 10:834024. doi: 10.3389/feart.2022.834024
Received: 12 December 2021; Accepted: 13 January 2022;
Published: 02 February 2022.
Edited by:
Yohan Guyodo, UMR7154 Institut de Physique du Globe de Paris (IPGP), FranceReviewed by:
Peter E. Driscoll, Carnegie Institution for Science, United StatesCopyright © 2022 Yoshimura. This is an open-access article distributed under the terms of the Creative Commons Attribution License (CC BY). The use, distribution or reproduction in other forums is permitted, provided the original author(s) and the copyright owner(s) are credited and that the original publication in this journal is cited, in accordance with accepted academic practice. No use, distribution or reproduction is permitted which does not comply with these terms.
*Correspondence: Yutaka Yoshimura, eW9zaGltdXJhLnl1dGFrYS4zNndAa3lvdG8tdS5qcA==
Disclaimer: All claims expressed in this article are solely those of the authors and do not necessarily represent those of their affiliated organizations, or those of the publisher, the editors and the reviewers. Any product that may be evaluated in this article or claim that may be made by its manufacturer is not guaranteed or endorsed by the publisher.
Research integrity at Frontiers
Learn more about the work of our research integrity team to safeguard the quality of each article we publish.