- 1School of Energy and Mining Engineering, China University of Mining and Technology, Beijing, China
- 2Beijing Key Laboratory for Precise Mining of Intergrown Energy and Resources, China University of Mining and Technology, Beijing, China
- 3Research Institute of Macro-Safety Science, University of Science and Technology Beijing, Beijing, China
- 4School of Civiland Resource Engineering, University of Science and Technology Beijing, Beijing, China
- 5Key Laboratory of Deep Earth Science and Engineering (Sichuan University), Ministry of Education, Chengdu, China
Gas adsorption and desorption capacities and ad-/desorption hysteresis in coal are important for carbon capture and storage (CCS) and coalbed methane (CBM) development. To investigate the impact of fractal features on gas adsorption and desorption capacities and ad-/desorption hysteresis in coals, five coal samples were collected and carried out methane (CH4) and CO2 isothermal ad-/desorption experiments. Small angle X-ray scattering (SAXS) was applied to characterize the fractal features of the coal pore structure. The results show that five coal samples show surface fractal features, represented by surface fractal dimension (Ds). The adsorption and desorption capacities of CO2 are stronger than those of CH4. In the adsorption stage, Ds and Langmuir adsorption volume (VL-ad) show a positive relationship for CH4 and CO2, due to the van der Waals force and available adsorption sites. In the desorption stage, Ds and Langmuir desorption volume (VL-de) show a positive relationship for CH4 and CO2, because most adsorbed gas molecules can desorb and diffuse out of the pores when gas pressure decreases. No obvious correlation was found between Ds and Langmuir adsorption pressure (PL-ad) as well as between Ds and Langmuir desorption pressure (PL-de) for CO2 and CH4. An improved hysteresis index (IHI) was adopted to characterize the degree of gas ad-/desorption hysteresis. The IHI values of CO2 vary from 12.2 to 35.2%, and those of CH4 vary from 8.9 to 50.3%. The curves of Ds vs. IHI for CO2 and CH4 are like an irreversible “V” shape, which yields to be further studied. This work further extends SAXS application in exploring the impact of coal pore structure on gas adsorption related phenomena, which is beneficial for CCS technology and CBM development.
Introduction
In the context of carbon emission peaks and carbon neutral in China, carbon capture and storage (CCS) has become a research hotspot (Budinis et al., 2018; Fan et al., 2018). International Energy Agency (IEA) pointed out that if the warming rate by the end of this century is about to achieve 1.75°C, the CCS technology needs to contribute 32% of CO2 emission reduction (IEA, 2017). Coalbed methane (CBM) is a typically geological gas mainly consisting of methane (CH4), which contains huge energy and thus serves as an emerging energy resource (Wang et al., 2014; Zhang et al., 2016). In 2020, CBM production is 58.2 × 108 cubic meters, which is of great significance to ensure energy security and reduce foreign dependence on natural gas (Xu et al., 2021). Gas in coal has three occurrence states: dissolution state, adsorption state, and free state, respectively, and the majority of gases are in the adsorption state (Cui et al., 2004). Ad-/desorption isotherms can quantitatively reflect the gas adsorption and desorption capacities in coal; meanwhile, it has been observed that it exists a positive difference between the ad-/desorption isotherms for CH4 and CO2, which is termed as adsorption–desorption hysteresis (Wang G. et al., 2016), sorption hysteresis (Ekundayo and Rezaee, 2019; He et al., 2020), desorption hysteresis (Ma et al., 2012), and degree of irreversibility (Zhang and Liu, 2017) and so on in different literature. In this work, the authors adopted the term—ad-/desorption hysteresis—to describe this difference for the sake of unification. For CCS technology, coal reservoirs have been proven to be a viable CSS geological body, with a CO2 storage ability of 12 Gt (Liu et al., 2005). As is well known, CO2 adsorption and desorption features are closely related to the amount and stability of CO2 storage (Sun et al., 2018). Similarly, in CBM industry, CH4 adsorption and desorption properties directly affect CBM production, the larger the amount of CH4 that can be desorbed is, the higher the recovery rate is, whereas the ad-/desorption hysteresis can hinder the recovery rate (Ma et al., 2012).
The pore structure is one of the key factors affecting gas adsorption, desorption, and ad-/desorption hysteresis in coal (Tan et al., 2018; Yin et al., 2019; Zhou et al., 2020) because the pore structure is where the gas is adsorbed and desorbed. Fractal dimension is a parameter that can comprehensively reflect the features of coal pore structure (Liu and Nie, 2016). In recent years, some scholars just tentatively began to adopt fractal theory to explore the intrinsic mechanism of gas adsorption-related phenomena. Li et al. (2015) stated that based on the fractal dimension tested by N2 adsorption, the CH4 adsorption capacity enhances with surface fractal dimension increasing but weakens with pore fractal dimension increasing. However, similarly based on the N2 adsorption method, Sun et al. (2015) drew a conclusion that is not fully consistent with Li et al. (2015), reporting that the CH4 adsorption capacity increases with the increase of surface fractal dimension, but there is no obvious relationship between CH4 adsorption capacity and pore fractal dimension. Niu et al. (2019) applied three different measurement methods, including N2 adsorption, SAXS, and scanning electron microscopy to research the effect of fractal dimension on CH4 adsorption behavior, and found that Langmuir adsorption pressure shows a negative relationship with fractal dimension. He et al. (2020) applied CO2 and N2 adsorption methods to calculate the fractal dimension and analyzed the relevancy between the fractal dimension and methane sorption hysteresis in coal, and found that there is a potential positive correlation between the methane sorption hysteresis and the coal heterogeneity. In summary, even though if any, research on the combination of fractal and gas adsorption related phenomena remains to be scarce and unsystematic; meanwhile, the characterization of fractal dimension in previous studies mainly relies on traditional gas adsorption methods.
As a non-destructive testing technology, synchrotron radiation Small Angle X-Ray Scattering (SAXS) has been extensively applied to measure the pore structure of multiporous materials (Mares et al., 2009; Syed et al., 2018); it can concurrently measure both open and closed pores in its testing scope (Pan et al., 2016; Zhao et al., 2019). As a relatively novel and innovative method for pore structure measurement, SAXS application has gradually extended to the field of coal fractal feature characterization. Zhao and Peng, (2017) used SAXS to test the fractal of six coal samples with different ranks, and found that coal samples show surface fractal at the region of low q value and pore fractal at the region of high q value. Song et al. (2014) found that the surface fractal dimension of coal tested by SAXS increases with the increase in deformation extent. Nie et al. (2021) found that when gas adsorption occurs in coal, the transformation of the coal pore structure can be characterized by fractal dimension tested by SAXS. Xie et al. (2019) conducted SAXS fractal experiments on bituminous coal and stated that the bituminous coal shows surface fractal features throughout the carbonization process. Wang et al. (2021) conducted SAXS fractal experiments on anthracite coal and demonstrated that with the development of carbonization, the fractal feature of the coal pore structure varies between pore fractal and surface fractal. However, research on the correlation between the fractal dimension on the basis of SAXS fractal theory and gas adsorption related phenomena in coal is relatively scarce. Accordingly, it is greatly necessary to enhance and expand the application of SAXS fractal theory to the investigation of gas adsorption and desorption and ad-/desorption hysteresis in coal.
In this work, five coal samples were performed on SAXS experiments and adsorption and desorption isothermal experiments. Their surface fractal dimensions were calculated based on the SAXS fractal theory, their adsorption and desorption capacities were quantified by Langmuir constants, the degrees of their ad-/desorption hysteresis were quantified by a hysteresis index, and the impact of coal fractal features on gas adsorption and desorption capacities and ad-/desorption hysteresis was investigated.
Experimental Setup
Sampling and Sample Preparation
In this work, five experimental samples were collected from five different mines. Sampling sites and properties of coal samples were listed in Table 1. The mean maximum vitrinite reflectance (Ro,max) is from 0.68 to 4.33%, covering low-rank, middle-rank, and high-rank coals. The moisture content (M) ranges from 0.58 to 1.60%, the volatile content (V) ranges from 9.51 to 32.83%, the fixed carbon content (Fc) ranges from 51.87 to 76.79%, and the ash content (A) ranges from 8.71 to 20.84%. The mineral content of sample #2 (17.5%) is the highest and that of sample #5 (1.5%) is the lowest, the vitrinite group content of sample #5 (91.0%) is the highest and that of sample #1 (36.4%) is the lowest, and the exinite contents of samples #3, #4 and #5 are 0.
For SAXS experiments, the first step is to process samples into round slices with a thickness of 0.5 mm and a diameter of 9.5 mm, as shown in Figure 1, and then a sample chamber, which was specifically covered by Kapton film, was used to contain the processed samples for the following experiment.
For gas isothermal ad-/desorption measurements, samples were pulverized into 60–80 meshes (180–250 μm), and about 10 g of pulverized samples was selected to be degassed at 30°C for 24 h and heated 1 h to fully remove the moisture inside samples.
Synchrotron SAXS Experiments
Beijing Synchrotron Radiation Facilities (BSRF) were applied to conduct SAXS experiments in this work. The authors have described the parameters of BSRF in detail in our previous research (Zhao et al., 2014).
For SAXS experiments, the chamber loaded with samples was placed on the sample table, 1,650 mm far away from the detector, and then the SAXS device was started to record the photodiode readings and collect SAXS images; this process lasted for about 5 s. And then the FIT2D software was applied to convert the SAXS images into one-dimensional scattering data (Hammersley et al., 1996). The authors have given the details of SAXS image processing in the FIT2D software in our previous research (Zhao et al., 2019).
Gas Isothermal Ad-/Desorption Experiments
An H-Sorb sorption device (Sun et al., 2020) was used for CO2 and CH4 adsorption and desorption experiments. The processed samples were first loaded into an adsorption tank and evacuated, and the remaining volume of the adsorption tank was measured. Then, a certain volume of gas was charged into the adsorption tank or discharged from the adsorption tank until the pressure in each section reached an equilibrium state. At this moment, part of gas was adsorbed, and part of gas was still in the remaining volume in the free state. The volume of gas charged was known, and the free volume after deducting the remaining volume was the adsorption volume in samples. Repeat the above procedures to obtain the adsorption volume in each pressure section. The adsorption isotherms were obtained when the gas was charged into the adsorption tank from low pressure to high pressure, and the desorption isotherms were obtained when the gas was discharged out of the adsorption tank from high pressure to low pressure.
Results and Discussion
Fractal Characterization by SAXS
Wijnen et al. (1991) have ever found that the power-law formula can well express the SAXS intensity of a fractal object,
where α varies from 0 to 4, serving as the fractal parameter.
The fractal features of the coal pore structure are defined as surface fractal and pore fractal based on the SAXS fractal theory, which are represented by Ds and Dp, respectively. If α is between 3 and 4, the pore structure is of surface fractal feature, and the value of Ds is Ds = 6‐α. If α is between 0 and 3, the pore structure is of pore fractal feature, and the value of Dp is Dp = α (Reich et al., 1992).
Figure 2 shows the fractal curves of five coal samples. The fractal dimensions were obtained by making a tangent to the lnI(q)—lnq curves within linear ranges. The results show that the α values range between 3 and 4, indicating that all samples show surface fractal features. The Ds values vary from 2.34 to 2.93 with sample #4 being of the highest Ds value and sample #1 being of the lowest Ds value, as listed in Table 2.
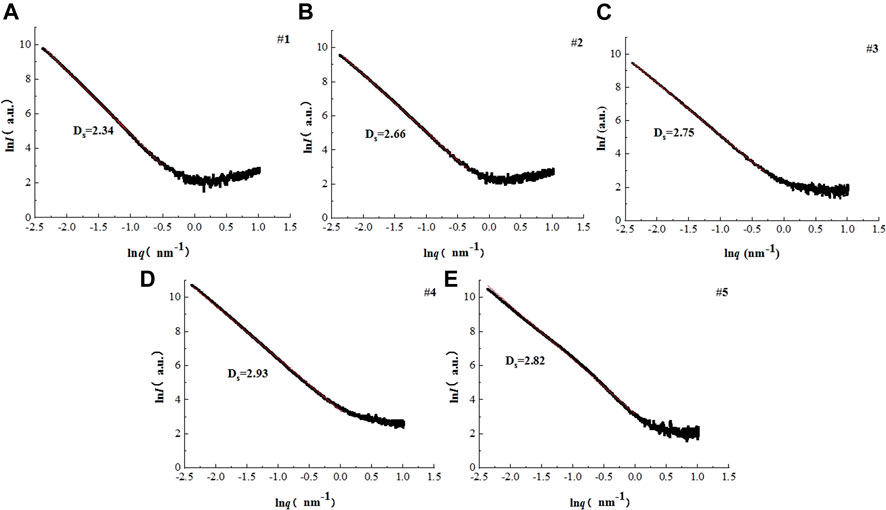
FIGURE 2. Fractal curves. (A) Sample #1, (B) Sample #2, (C) Sample #3, (D) Sample #4, and (E) Sample #5.
Determination of Langmuir Constants
In this work, the adsorption isotherms are determined by the classic Langmuir formula (Langmuir, 1918) and the desorption isotherms are determined by the modified Langmuir formula (Ma et al., 2011) since it exists residual adsorption volume under a lack pressure between adsorption and desorption isotherms, which is to say that the gas volume is not zero when the pressure decreases to zero in the desorption stage. Eqs 2, 3 represent these two models, respectively,
where P stands for the equilibrium gas pressure in the adsorption or desorption stage, MPa; V is the adsorbed gas volume when the gas pressure reaches P, ml/g; VL-ad and VL-de are the Langmuir adsorption and desorption volumes, which is equal to the maximum monolayer adsorption and desorption values of gas, ml/g; PL-ad and PL-de are the Langmuir adsorption and desorption pressures, the pressure when the adsorbed gas volume is half of VL-ad and VL-de, MPa; and M is the residual adsorption volume under a lack pressure, ml/g.
Figure 3 shows the adsorption and desorption isotherms of CO2 and CH4. Tables 3, 4 show the Langmuir constants of CO2 and CH4, respectively.
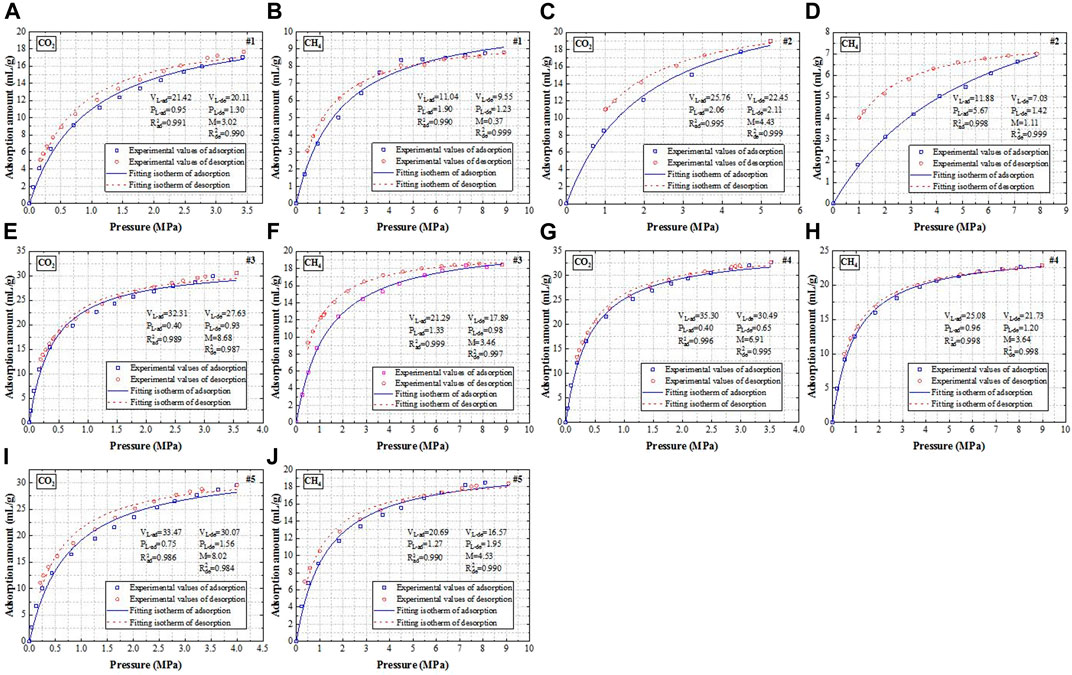
FIGURE 3. Adsorption and desorption isotherms of CO2 and CH4. (A-B) Sample #1, (C-D) Sample #2, (E-F) Sample #3, (G-H) Sample #4, and (I-J) Sample #5.
As is widely known, the Langmuir volume and Langmuir pressure have a significant impact on gas adsorption and desorption performance. The higher VL-ad is, the stronger the gas adsorption capacity is, the higher PL-ad is, the harder the gas is adsorbed to the coal pore surface; similarly, the higher VL-de is, the stronger the gas desorption capacity is, the higher PL-de is, the harder the gas is desorbed to the coal pore surface (Niu et al., 2019). It can be seen from Tables 3, 4 that for the same sample, the VL-ad and VL-de values of CO2 are greater than those of CH4, demonstrating that the adsorption and desorption capacities of CO2 are stronger than those of CH4, which is in line with previous studies (Sander et al., 2016; Zhang et al., 2018). This is attributed to the fact that CO2 owns a smaller kinetic diameter than CH4 does (CO2: 0.33 nm versus CH4: 0.38 nm); therefore, CO2 is much easier to diffuse into pores with smaller size (Hou et al., 2020), and the affinity of coal matrix to CO2 is stronger than that of coal matrix to CH4. Similarly, for the same sample, the PL-ad value of CH4 is greater than that of CO2, indicating that CH4 is harder to be adsorbed on the coal pore surface than CO2; nevertheless, there is no obvious rule concerning the PL-de values.
Impact of Ds on Adsorption and Desorption Capacities
Figure 4 shows Ds vs. Langmuir constants curves. It can be seen from Figure 4A, in the CO2 adsorption stage, VL-ad increases with Ds increasing, demonstrating that the larger Ds is, the stronger the CO2 adsorption capacity is. Figure 4E shows that in the CH4 adsorption stage, Ds and VL-ad show a positive relationship. VL-ad increases with Ds increasing, demonstrating that the larger Ds is, the stronger the CH4 adsorption capacity is, which is consistent with studies by Niu et al. (2019) using SAXS and Li et al. (2015) using N2 adsorption.
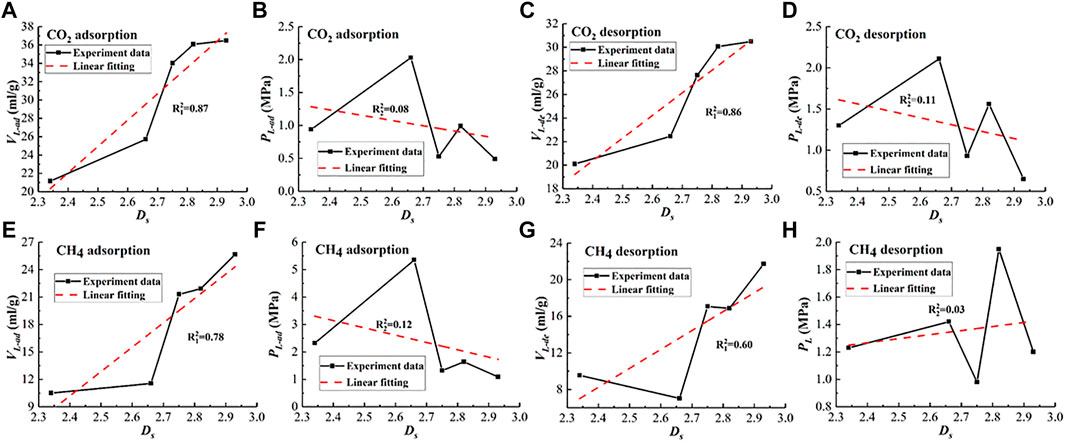
FIGURE 4. Relationship between Ds and Langmuir constants. (A) Ds vs. VL-ad for CO2, (B) Ds vs. PL-ad for CO2, (C) Ds vs. VL-de for CO2, (D) Ds vs. PL-de for CO2, (E) Ds vs. VL-ad for CH4, (F) Ds vs. PL-ad for CH4, (G) Ds vs. VL-de for CH4, and (H) Ds vs. PL-de for CH4.
The gas adsorption in coal includes monolayer adsorption and multilayer adsorption (Yang and Liu, 2019). In the monolayer adsorption stage, there are two main factors affecting the gas adsorption volume, one is the van der Waals force existing between the pore surface and the gas molecules and the other is the number of adsorption sites available on the pore surface. Ds can represent the strength of the van der Waals force (Chen et al., 2017). The van der Waals force is an indicator of the mutual attraction strength between molecules.
In the adsorption process, gas molecules can diffuse into the pores with a size larger than or equal to the average free path of gas molecules under the effect of gas pressure. The coal matrix can be regarded as organic solids composed of carbon atoms, the gas molecules entered the pore structure can be attracted by the carbon atoms on the pore surface, this is where the van der Waals force works, thereby the gas molecules can earn a tendency to move toward the pore surface. This tendency grants the carbon atoms on the pore surface extra energy—the surface free energy (He et al., 1996). The carbon atoms on the pore surface are always trying to absorb other substances around them to reduce their surface free energy. Hence, the stronger the van der Waals force is, the stronger the gas adsorption performance is. Meanwhile, Ds can also represent the roughness and irregularity of the pore surface (Yao et al., 2008; Niu et al., 2019). The higher Ds value indicates the rougher pore surface, at which more sites are available for gas adsorption (Wang Y. et al., 2016), further enhancing the gas adsorption capacities. Hence, the fact that Ds and VL-ad show a positive relationship can be explained from the perspectives of the pore surface roughness and the van der Waals force.
However, with the increase of relative pressure, the monolayer adsorption transits to the multilayer adsorption. During the multilayer adsorption stage, more adsorption layers are established, and the adsorbent interface becomes smooth. At this time, the effect of the capillary condensation, which can be represented by Dp, accounts for a leading position (Qi et al., 2002). Unfortunately, SAXS failed to test the pore fractal in this study, leading to counting for the gas amount of multilayer adsorption mainly affected by the pore fractal when we discuss the impact of Ds on the gas adsorption capacities. However, according to Wang (2015), the Langmuir model is based on the hypothesis of monolayer adsorption and its good application in actual projects illustrates that the monolayer adsorption takes up a leading proportion. Besides, since the gas pressure during the adsorption and desorption at normal temperature is much higher than the gas pressure range applicable to the multilayer adsorption, the multilayer adsorption is generally considered to be rather weak. Therefore, although the gas amount of multilayer adsorption is counted into the total gas adsorption amount, it doesn’t actually affect the discussion of the impact of Ds on the gas adsorption capacities.
As shown in Figures 4C, G, VL-de for CO2 and VL-de for CH4 exhibit an increasing trend with the increase of Ds. It can be explained by the fact that VL-ad shows a positive relationship with VL-de, as demonstrated by Figure 5. And as discussed above, larger Ds values represent the rougher pore surface providing more available adsorption sites and stronger van der Waal force reinforcing the mutual attraction strength between the gas molecules and pore surface, which enhances the total adsorption volume in the adsorption stage. On the contrary, in the desorption stage, the van der Waals force plays a role in restricting gas molecules to escape from the pore surface; besides, rougher pore surface produces stronger friction resistance hindering the desorbed gas molecules to diffuse out of the pore structure. However, the restricted effects caused by these two factors are limited, most adsorbed gas molecules still can desorb and diffuse out of the pores when the gas pressure decreases. Therefore, the fact that VL-ad shows a positive relationship with VL-de is reasonable; meanwhile, Figures 4A, E show Ds shows a positive relationship with VL-ad, so it is no wonder that VL-de increases with Ds increasing.
However, no correlation was found between Ds and PL-ad as well as between Ds and PL-de according to the fluctuation presented in Figures 4B, D, F, H.
Determination of Ad-/Desorption Hysteresis and Impact of Ds on Ad-/Desorption Hysteresis
Scholars in different fields, such as soil, rock, and high-molecular polymer, have ever proposed various hysteresis indexes for evaluating ad-/desorption hysteresis phenomenon based on the Freundlich index, the equilibrium concentration of solid phase, slope (Ran et al., 2003; Wu and Sun, 2010; Ding and Rice, 2011); however, they are not quite applicable to gas adsorption and desorption in coal (Wang G. et al., 2016). Wang et al. (2014) put forward an improved hysteresis index (IHI) based on the area formed by ad-/desorption isotherms, which has been proved to be effective in quantitatively characterizing the degree of gas ad-/desorption hysteresis in coal (Wang G. et al., 2016; Sun et al., 2020). Hence, IHI was adopted in this work, as shown by Eqs 4–9,
where Aad and Ade stand for the zones below the adsorption and desorption isotherms; Pf stands for the final equilibrium pressure, MPa; Ahy represents the measured hysteresis area; and Ahf and Asf represent the hysteresis area and the adsorption area in the fully irreversible case, respectively.
Figure 6 shows the IHI calculation of CO2 and CH4, and the results are listed in Table 5.
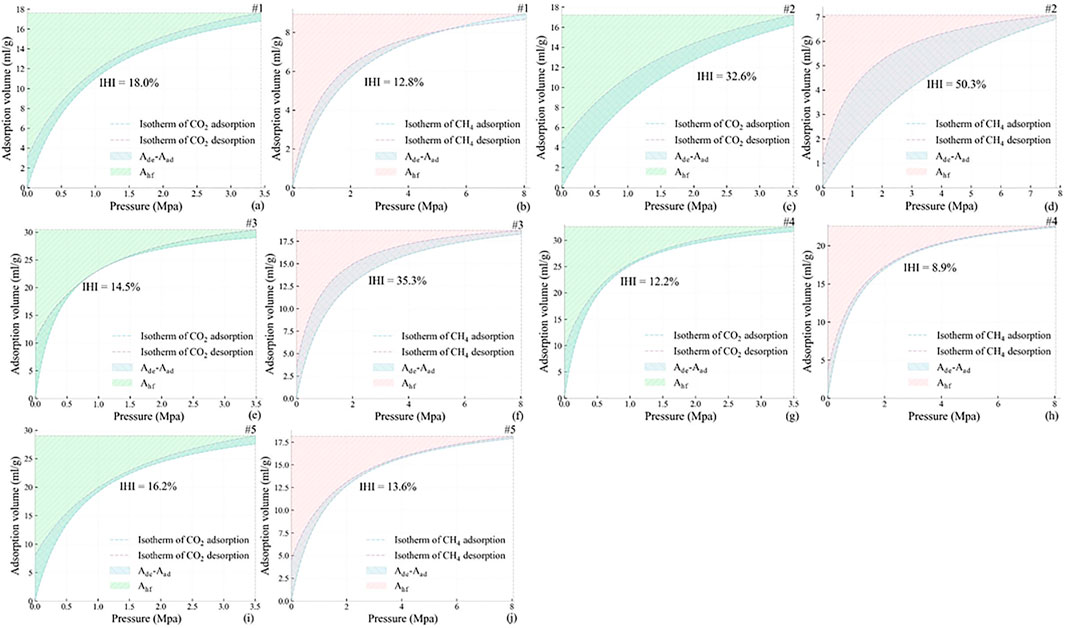
FIGURE 6. IHI calculation. (A), (C), (E), (G), and (I) represent CO2 IHI calculation; (B), (D), (F), (H), and (J) represent CH4 IHI calculation.
Jessen et al. (2008) have demonstrated that desorption isotherms depend on the initial pressure at the beginning of depressurization; therefore, when the final equilibrium pressure set in Eqs 5–7 varies, ad-/desorption hysteresis varies as well. To avoid the possible error caused by the difference of the final equilibrium pressure of every sample, the final equilibrium pressures of CO2 and CH4 were uniformly set to 3.5 and 8 MPa for IHI calculation in this work. It should be noted that desorption points at 0 MPa were not measured in this work; to enable the adsorption and desorption isotherms to form a closed interval with the y axis and the vertical axis passed through (Pf, 0), the desorption isotherms were extended to the (0, M) point according to Eq. 3. Since the proposed IHI is calculated based on the area instead of a single point, a slight change at the end of desorption isotherms cannot significantly affect the calculation results of the IHI value; thus, the process of desorption isotherm extension is theoretically acceptable.
Figure 7 shows the relationship between Ds and IHI. It can be seen that for CO2, IHI fluctuates with Ds changing. It first increases from 18.0 to 32.6% when Ds increases from 2.34 to 2.66, and then decreases to 14.5%, followed with a slight bounce to 16.1%, and finally drops to 12.2%. For CH4, IHI rises to the maximum value of 50.3% when Ds increases from 2.34 to 2.66, and keeps falling when Ds varies between 2.66 and 2.93. In general, the curves of Ds vs. IHI for CO2 and CH4 are like an irreversible “V” shape.
As mentioned in Determination of Langmuir Constants, coal physical properties that can be directly reflected by Ds are the van der Waals force and the pore surface irregularity. Zhang et al. (2005) have reported that the adsorbed gas molecules on the pore surface of coal require a certain amount of energy to offset the van der Waals force so that they can escape from the pore surface; therefore, the desorption process will lag behind adsorption to varying degrees. According to the viewpoint by Zhang et al. (2005), the stronger the van der Walls force is, the more obvious the ad-/desorption hysteresis is. Besides, Seri-Levy and Avnir (1993) have studied the effect of the heterogeneity of the pore surface on CH4 ad-/desorption hysteresis. He demonstrated that the pore surface heterogeneity is sufficient to induce an ad-/desorption hysteresis loop and the degree of ad-/desorption hysteresis increases with the pore surface heterogeneity increasing. According to the statements by Zhang et al. (2005) and Seri-Levy and Avnir (1993), Ds and IHI are also more likely to present a positive relationship, but in this work, they present an irreversible “V-like” shape, primarily caused by that the ad-/desorption hysteresis in the sample with the Ds value 2.66 is rather dramatical, but the intrinsic mechanism of the irreversible “V-like” shape is hard to be explained by the existing theories and yields to be further investigated.
Conclusion
Fractal features of five coals were characterized by SAXS, the classic Langmuir model and the improved Langmuir model were adopted to characterize the gas adsorption and desorption capacities, and IHI was adopted to characterize the degree of gas ad-/desorption hysteresis. The evidence leads to the following conclusions:
1) Different samples show different surface fractal features with Ds values varying from 2.34 to 2.93.
2) The VL-ad and VL-de values of CO2 are greater than those of CH4, demonstrating that the adsorption and desorption capacities of CO2 are stronger than those of CH4. The PL-ad values of CH4 are greater than those of CO2, demonstrating that CH4 is harder to be adsorbed on the coal pore surface than CO2.
3) In the adsorption stage, Ds and VL-ad show a positive relationship for CH4 and CO2, which is attributed to the van der Waals force and available adsorption sites. In the desorption stage, Ds and VL-de show a positive relationship for CH4 and CO2 because most adsorbed gas molecules can desorb and diffuse out of the pores when the gas pressure decreases. No correlation was found between Ds and PL-ad as well as between Ds and PL-de on a basis of SAXS fractal theory.
4) The curves of Ds vs. IHI for CO2 and CH4 are like an irreversible “V” shape, primarily caused by the fact that the ad-/desorption hysteresis in the sample with the Ds value 2.66 is rather dramatical, but the intrinsic mechanism yields to be further studied.
Data Availability Statement
The original contributions presented in the study are included in the article, further inquiries can be directed to the corresponding author.
Author Contributions
YZ: Provide the experiment condition, design the experiment, theoretical analysis, revise the manuscript. CH: Conduct the experiment, draft the manuscript. YS: Theoretical analysis, revise the manuscript. YG: Data collection and figure drawing. HQ: Data collection and figure drawing. ZT: Conduct the experiment.
Funding
The research is financially sponsored by the National Natural Science Foundation of China (Grant Nos. U1910206, 51861145403, 51874312 and 52004293); the Open Project Program of Key Laboratory of Deep Earth Science and Engineering (Sichuan University), Ministry of Education (No. DESE 202004); the Fundamental Research Funds for the Central Universities (No. FRF-TP-20-034A1); the China Postdoctoral Science Foundation (No. 2018M641526); and the Yue Qi Distinguished Scholar Project of the China University of Mining and Technology (Beijing).
Conflict of Interest
The authors declare that the research was conducted in the absence of any commercial or financial relationships that could be construed as a potential conflict of interest.
Publisher’s Note
All claims expressed in this article are solely those of the authors and do not necessarily represent those of their affiliated organizations, or those of the publisher, the editors and the reviewers. Any product that may be evaluated in this article, or claim that may be made by its manufacturer, is not guaranteed or endorsed by the publisher.
Acknowledgments
The authors thank Zhihong Li, Guang Mo, and Wanxia Huang of the Beijing Synchrotron Radiation Laboratory (BSRF) for providing SAXS experimental facilities and for their suggestions in conducting experiments and data processing.
References
Budinis, S., Krevor, S., Dowell, N. M., Brandon, N., and Hawkes, A. (2018). An Assessment of CCS Costs, Barriers and Potential. Energ. Strategy Rev. 22, 61–81. doi:10.1016/j.esr.2018.08.003
Chen, L., Jiang, Z., Wen, N., Gao, F., Wang, P., Ji, W., et al. (2017). Fractal Characteristics of Nanopores and Their Effect on Methane Adsorption Capacity in Shales. Sci. Technol. Eng. 53, 1689–1699. doi:10.1017/CBO9781107415324.004
Cui, X., Bustin, R. M., and Dipple, G. (2004). Selective Transport of CO2, CH4, and N2 in Coals: Insights from Modeling of Experimental Gas Adsorption Data. Fuel 83, 293–303. doi:10.1016/j.fuel.2003.09.001
Ding, G., and Rice, J. A. (2011). Effect of Lipids on Sorption/desorption Hysteresis in Natural Organic Matter. Chemosphere 84, 519–526. doi:10.1016/j.chemosphere.2011.03.009
Ekundayo, J. M., and Rezaee, R. (2019). Volumetric Measurements of Methane-Coal Adsorption and Desorption Isotherms-Effects of Equations of State and Implication for Initial Gas Reserves. Energies 12, 1–13. doi:10.3390/en12102022
Fan, J.-L., Xu, M., Li, F., Yang, L., and Zhang, X. (2018). Carbon Capture and Storage (CCS) Retrofit Potential of Coal-Fired Power Plants in China: The Technology Lock-In and Cost Optimization Perspective. Appl. Energ. 229, 326–334. doi:10.1016/j.apenergy.2018.07.117
Hammersley, A. P., Svensson, S. O., Hanfland, M., Fitch, A. N., and Hausermann, D. (1996). Two-dimensional Detector Software: From Real Detector to Idealised Image or Two-Theta Scan. High Press. Res. 14, 235–248. doi:10.1080/08957959608201408
He, X., Cheng, Y., Hu, B., Wang, Z., Wang, C., Yi, M., et al. (2020). Effects of Coal Pore Structure on Methane‐coal Sorption Hysteresis: An Experimental Investigation Based on Fractal Analysis and Hysteresis Evaluation. Fuel 269, 117438. doi:10.1016/j.fuel.2020.117438
He, X., Wang, E., and Lin, H. (1996). Coal Deformation and Fracture Mechanism under Pore Gas Action. J. China Univ. Min Technol. 25, 6–11. CNKI:SUN:ZGKD.0.1996-01-001.
Hou, X., Liu, S., Zhu, Y., and Yang, Y. (2020). Experimental and Theoretical Investigation on Sorption Kinetics and Hysteresis of Nitrogen, Methane, and Carbon Dioxide in Coals. Fuel 268, 117349. doi:10.1016/j.fuel.2020.117349
Jessen, K., Tang, G.-Q., and Kovscek, A. R. (2008). Laboratory and Simulation Investigation of Enhanced Coalbed Methane Recovery by Gas Injection. Transp Porous Med. 73, 141–159. doi:10.1007/s11242-007-9165-9
Langmuir, I. (1918). The Adsorption of Gases on Plane Surfaces of Glass, Mica and Platinum. J. Am. Chem. Soc. 40, 1361–1403. doi:10.1021/ja02242a004
Li, Z., Hao, Z., Pang, Y., and Gao, Y. (2015). Fractal Dimensions of Coal and Their Influence on Methane Adsorption. J. China Coal Soc. 40, 863–869. doi:10.13225/j.cnki.jccs.2014.3022
Liu, X., and Nie, B. (2016). Fractal Characteristics of Coal Samples Utilizing Image Analysis and Gas Adsorption. Fuel 182, 314–322. doi:10.1016/j.fuel.2016.05.110
Liu, Y., Li, X., and Bai, B. (2005). Preliminary Estimation of CO2 Storage Capacity of Coalbeds in China. Chin. J. Rock Mech. Eng. 16, 2947–2952. doi:10.3321/j.issn:1000-6915.2005.16.02310.1360/jos161833
Ma, D., Ma, W., and Lin, Y. B. (2012). Desorption Hysteresis Characteristics of CBM. Meitan Xuebao/journal China Coal Soc. 37, 1885–1889. doi:10.13225/j.cnki.jccs.2012.11.011
Ma, D., Zhang, S., and Lin, Y. (2011). Isothermal Adsorption and Desorption experiment of Coal and Experimental Results Accuracy Fitting. J. China Coal Soc. 36, 477–480. doi:10.13225/j.cnki.jccs.2011.03.006
Mares, T. E., Radliński, A. P., Moore, T. A., Cookson, D., Thiyagarajan, P., Ilavsky, J., et al. (2009). Assessing the Potential for CO2 Adsorption in a Subbituminous Coal, Huntly Coalfield, New Zealand, Using Small Angle Scattering Techniques. Int. J. Coal Geology. 77, 54–68. doi:10.1016/j.coal.2008.07.007
Nie, B., Wang, K., Fan, Y., Zhao, J., Zhang, L., Ju, Y., et al. (2021). Small Angle X-ray Scattering Test of Hami Coal Sample Nanostructure Parameters with Gas Adsorbed under Different Pressures. J. Nanosci. Nanotechnol. 21, 538–546. doi:10.1166/jnn.2021.18725
Niu, Q., Pan, J., Jin, Y., Wang, H., Li, M., Ji, Z., et al. (2019). Fractal Study of Adsorption-Pores in Pulverized Coals with Various Metamorphism Degrees Using N2 Adsorption, X-ray Scattering and Image Analysis Methods. J. Pet. Sci. Eng. 176, 584–593. doi:10.1016/j.petrol.2019.01.107
Pan, J., Niu, Q., Wang, K., Shi, X., and Li, M. (2016). The Closed Pores of Tectonically Deformed Coal Studied by Small-Angle X-ray Scattering and Liquid Nitrogen Adsorption. Microporous Mesoporous Mater. 224, 245–252. doi:10.1016/j.micromeso.2015.11.057
Qi, H., Ma, J., and Wong, P.-z. (2002). Adsorption Isotherms of Fractal Surfaces. Colloids Surf. A: Physicochemical Eng. Aspects 206, 401–407. doi:10.1016/S0927-7757(02)00063-8
Ran, Y., Xiao, B., Fu, J., and Sheng, G. (2003). Sorption and Desorption Hysteresis of Organic Contaminants by Kerogen in a sandy Aquifer Material. Chemosphere 50, 1365–1376. doi:10.1016/S0045-6535(02)00762-2
Reich, M. H., Snook, I. K., and Wagenfeld, H. K. (1992). A Fractal Interpretation of the Effect of Drying on the Pore Structure of Victorian Brown Coal. Fuel 71, 669–672. doi:10.1016/0016-2361(92)90170-S
Sander, R., Pan, Z., Connell, L. D., Camilleri, M., and Heryanto, D. (20162016). Experimental Investigation of Gas Diffusion in Coal - Comparison between Crushed and Intact Core Samples. PE Asia Pac. Oil Gas Conf. Exhibition. doi:10.2118/182466-MS
Seri-Levy, A., and Avnir, D. (1993). Effects of Heterogeneous Surface Geometry on Adsorption. Langmuir 9, 3067–3076. doi:10.1021/la00035a054
Song, X., Tang, Y., Li, W., Zeng, F., and Xiang, J. (2014). Pore Structure in Tectonically Deformed Coals by Small Angle X-ray Scattering. J. China Coal Soc. 39, 719–724. doi:10.13225/j.cnki.jccs.2013.1932
Sun, W., Feng, Y., Jiang, C., and Chu, W. (2015). Fractal Characterization and Methane Adsorption Features of Coal Particles Taken from Shallow and Deep Coalmine Layers. Fuel 155, 7–13. doi:10.1016/j.fuel.2015.03.083
Sun, Y., Zhao, Y., and Yuan, L. (2018). CO2-ECBM in Coal Nanostructure: Modelling and Simulation. J. Nat. Gas Sci. Eng. 54, 202–215. doi:10.1016/j.jngse.2018.04.007
Sun, Y., Zhao, Y., and Yuan, L. (2020). Impact of Coal Composition and Pore Structure on Gas Adsorption: a Study Based on a Synchrotron Radiation Facility. Greenhouse Gas Sci. Technol. 10, 116–129. doi:10.1002/ghg.1935
Syed, R., Sen, D., Mani Krishna, K. V., and Ghosh, S. K. (2018). Fabrication of Highly Ordered Nanoporous Alumina Membranes: Probing Microstructures by SAXS, FESEM and AFM. Microporous Mesoporous Mater. 264, 13–21. doi:10.1016/j.micromeso.2017.12.034
Tan, Y., Pan, Z., Liu, J., Kang, J., Zhou, F., Connell, L. D., et al. (2018). Experimental Study of Impact of Anisotropy and Heterogeneity on Gas Flow in Coal. Part I: Diffusion and Adsorption. Fuel 232, 444–453. doi:10.1016/j.fuel.2018.05.173
Wang, G. (2015). Adsorption and Desorption Hysteresis of Coal Seam Gas and its Influence on Gas Permeability. Beijing: China University of Mining and Technology. [dissertation]. [China (LP)].
Wang, G., Ren, T., Qi, Q., Wang, K., and Zhang, L. (2016a). Mechanism of Adsorption-Desorption Hysteresis and its Influence on Deep CBM Recovery. J. China Coal Soc. 41, 49–56. doi:10.13225/j.cnki.jccs.2015.9022
Wang, K., Wang, G., Ren, T., and Cheng, Y. (2014). Methane and CO 2 Sorption Hysteresis on Coal: A Critical Review. Int. J. Coal Geology. 132, 60–80. doi:10.1016/j.coal.2014.08.004
Wang, Y., Li, Z., Kong, J., Chang, L., Li, D., and Lv, B. (2021). In-situ SAXS Study on Fractal of Jincheng Anthracite during High-Temperature Carbonisation. Phil. Mag. Lett. 101, 320–329. doi:10.1080/09500839.2021.1936259
Wang, Y., Zhu, Y., Liu, S., and Zhang, R. (2016b). Pore Characterization and its Impact on Methane Adsorption Capacity for Organic-Rich marine Shales. Fuel 181, 227–237. doi:10.1016/j.fuel.2016.04.082
Wijnen, P. W. J. G., Beelen, T. P. M., Rummens, K. P. J., Saeijs, H. C. P. L., and van Santen, R. A. (1991). Silica Gel from Water Glass: a SAXS Study of the Formation and Ageing of Fractal Aggregates. J. Appl. Cryst. 24, 759–764. doi:10.1107/S0021889891000924
Wu, W., and Sun, H. (2010). Sorption-desorption Hysteresis of Phenanthrene - Effect of Nanopores, Solute Concentration, and Salinity. Chemosphere 81, 961–967. doi:10.1016/j.chemosphere.2010.07.051
Xie, F., Li, D., Li, Z., Li, Z., Mo, G., and Lv, B. (2019). Small-angle X-ray Scattering Study on the Fractal Structure of Solid Products of Bituminous Coal at Different Carbonization Temperatures. Phil. Mag. Lett. 99, 95–101. doi:10.1080/09500839.2019.1628366
Xu, F., Wang, B., Zhao, X., Yun, J., Zhang, S., Wang, H., et al. (2021). Thoughts and Suggestions on Promoting High Quality Development of China’s CBM Business under the Goal of “Double Carbon. China Pet. Explor. 26 (3), 9–18. doi:10.3969/j.issn.1672-7703.2021.03.002
Yang, Y., and Liu, S. (2019). Estimation and Modeling of Pressure-dependent Gas Diffusion Coefficient for Coal: A Fractal Theory-Based Approach. Fuel 253, 588–606. doi:10.1016/j.fuel.2019.05.009
Yao, Y., Liu, D., Tang, D., Tang, S., and Huang, W. (2008). Fractal Characterization of Adsorption-Pores of Coals from North China: An Investigation on CH4 Adsorption Capacity of Coals. Int. J. Coal Geology. 73, 27–42. doi:10.1016/j.coal.2007.07.003
Yin, T., Liu, D., Cai, Y., and Zhou, Y. (2019). Methane Adsorption Constrained by Pore Structure in High-Rank Coals Using FESEM, CO2 Adsorption, and NMRC Techniques. Energy Sci. Eng. 7, 255–271. doi:10.1002/ese3.275
Zhang, L., Ye, Z., Li, M., Zhang, C., Bai, Q., and Wang, C. (2018). The Binary Gas Sorption in the Bituminous Coal of the Huaibei Coalfield in China. Adsorption Sci. Tech. 36, 1612–1628. doi:10.1177/0263617418798125
Zhang, R., and Liu, S. (2017). Experimental and Theoretical Characterization of Methane and CO2 Sorption Hysteresis in Coals Based on Langmuir Desorption. Int. J. Coal Geology. 171, 49–60. doi:10.1016/j.coal.2016.12.007
Zhang, S., Ye, J., Tang, S., Ma, D., and Huo, Y. (2005). Theoretical Analysis of Coal-Methane Adsorption/desorption Mechanism and its Reversibility experiment. Nat. Gas Industry 25, 1–29. doi:10.1016/j.molcatb.2005.02.001
Zhang, X. G., Ranjith, P. G., Perera, M. S. A., Ranathunga, A. S., and Haque, A. (2016). Gas Transportation and Enhanced Coalbed Methane Recovery Processes in Deep Coal Seams: A Review. Energy Fuels 30, 8832–8849. doi:10.1021/acs.energyfuels.6b01720
Zhao, Y., Liu, S., Elsworth, D., Jiang, Y., and Zhu, J. (2014). Pore Structure Characterization of Coal by Synchrotron Small-Angle X-ray Scattering and Transmission Electron Microscopy. Energy Fuels 28, 3704–3711. doi:10.1021/ef500487d
Zhao, Y., and Peng, L. (2017). Investigation on the Size and Fractal Dimension of Nano-Pore in Coals by Synchrotron Small Angle X-ray Scattering. Chin. Sci. Bull. 62, 2416–2427. doi:10.1360/N972016-00970
Zhao, Y., Peng, L., Liu, S., Cao, B., Sun, Y., and Hou, B. (2019). Pore Structure Characterization of Shales Using Synchrotron SAXS and NMR Cryoporometry. Mar. Pet. Geology. 102, 116–125. doi:10.1016/j.marpetgeo.2018.12.041
Keywords: SAXS, fractal, adsorption, desorption, hysteresis, coal
Citation: Zhao Y, Han C, Sun Y, Gao Y, Qiao H and Tai Z (2022) Impact of Fractal Features on Gas Adsorption and Desorption Capacities and Ad-/Desorption Hysteresis in Coals Based on Synchrotron Radiation SAXS. Front. Earth Sci. 10:824348. doi: 10.3389/feart.2022.824348
Received: 29 November 2021; Accepted: 21 March 2022;
Published: 10 May 2022.
Edited by:
Yiwen Ju, University of Chinese Academy of Sciences, ChinaReviewed by:
Jianhong Kang, China University of Mining and Technology, ChinaMengdi Sun, Northeast Petroleum University, China
Copyright © 2022 Zhao, Han, Sun, Gao, Qiao and Tai. This is an open-access article distributed under the terms of the Creative Commons Attribution License (CC BY). The use, distribution or reproduction in other forums is permitted, provided the original author(s) and the copyright owner(s) are credited and that the original publication in this journal is cited, in accordance with accepted academic practice. No use, distribution or reproduction is permitted which does not comply with these terms.
*Correspondence: Yixin Zhao, emhhb3l4QGN1bXRiLmVkdS5jbg==