- 1College of Geography and Environmental Sciences, Northwest Normal University, Lanzhou, China
- 2College of Urban and Environmental Sciences, Peking University, Beijing, China
- 3State Key Laboratory of Cryospheric Science, Northwest Institute of Eco-Environment and Resources, Chinese Academy of Sciences, Lanzhou, China
- 4Chinese Academy of Meteorological Sciences, Beijing, China
Water stable isotopes are crucial for paleoclimate reconstruction and water cycle tracing in Antarctica. Accurate measurement of atmospheric water vapor isotopic composition of hydrogen and oxygen is required urgently for understanding the processes controlling the atmosphere–snow interaction and associated isotope fractionation. This study presents in situ real-time measurements of water vapor isotopes along the transect from Zhongshan Station to Dome Argus (hereafter Dome A) in East Antarctica for the first time. The results reveal that the surface vapor stable isotopes of δ18O and δ D showed a gradual decreasing trend in the interior plateau region with the distance away from the coast, with significant δ18O-temperature correlation gradient of 1.61‰°/C and δ18O-altitude gradient of –2.13‰/100 m. Meanwhile, d-excess gradually arises with elevation rise. Moreover, the spatial variation of vapor isotopic composition displays three different characters implying different atmosphere circulation backgrounds controlling the inland water cycle; it can be divided as the coastal steep area below 2,000 m, a vast inland area with an elevation varied between 2,000 and 3,000 m, and high central plateau. Thirdly, observed high inland Antarctica water vapor d-excess quantitatively confirms stratosphere air intrusion and vapor derived from low latitudes by Brewer–Dobson circulation. Finally, the diurnal cycle signals of interior area water vapor isotopes δ18O, δ D, and air temperature highlighted the substantial domination of the supersaturation sublimation/condensation effect in inland, and this suggests that fractionation occurs during sublimation and vapor–snow exchanges should no longer be considered insignificant for the isotopic composition of near-surface snow in Antarctica.
Introduction
Since the identification of the relationship between the temperature and the isotopic composition of condensed precipitation in the 1960s (Dansgaard et al., 1969), stable isotopic compositions of ice core (δ18O and δ D) have been used as temperature and moisture proxies to reconstruct past climate and the atmospheric water cycle (Jouzel et al., 1997; Augustin et al., 2004; Jouzel and Masson-Delmotte, 2010; Fudge et al., 2013; Münch and Laepple, 2018; Jing et al., 2019). However, the hydrogen and oxygen isotopes of snowfall are usually affected by multiple factors including fractionations in water phase transitions (e.g., evaporation and condensation) during its atmospheric transport and precipitation (Ritter et al., 2016; Pang et al., 2019; Hughes et al., 2021), so the isotope–temperature relationship varies with time and space (Jouzel et al., 1997; Masson-Delmotte et al., 2008; Casado et al., 2018), especially in the polar regions, after snow deposition, there is substantial isotope exchange between air (water vapor) and snow through sublimation and condensation because of the supersaturation condition, which can significantly change the isotopic composition of snow (Krinner et al., 1997; LeGrande and Schmidt, 2006; Masson-Delmotte et al., 2011; Werner et al., 2011; Pang et al., 2019). The so-called postdepositional processing and its effects on snow isotopic compositions need to be carefully assessed for properly interpreting ice core water isotopic records in terms of the reconstruction of past climate (Ritter et al., 2016; Hughes et al., 2021). The water vapor isotopic composition together with snow isotopes can provide information on the air–snow–ice interaction processes, including isotopic fractionation, related evaporation temperature, and condensation condition (Frezzotti et al., 2002; Ekaykin et al., 2004; Masson-Delmotte et al., 2008). In addition, equilibrium fractionation coefficients of water isotopes have been determined either by spectroscopic calculations or by laboratory experiments mainly at about 20°C (Merlivat and Nief, 1967; Majoube, 1970; Cappa et al., 2003; Steen-Larsen et al., 2013), and it needs further field work measurement and experiment for low-temperature application.
In inland Antarctica, accurate measurements of atmosphere water vapor isotopes are difficult due to major logistical challenges and a very low water vapor content resulting from low temperature. Recently, the development of infrared spectroscopy enables direct measurements of isotopic composition of atmospheric water vapor in the field. With careful calibration methodologies, these devices have already been successfully used for studies of water vapor isotopes in some Arctic and Antarctic sites (Steen-Larsen et al., 2013; Bonne et al., 2014; Casado et al., 2016). In Antarctica, although much data of snow and ice core water isotopes are available in the literature (Masson-Delmotte et al., 2008; Ding et al., 2010; Wang et al., 2010; Xiao et al., 2013; Pang et al., 2015), atmospheric water vapor isotopes in most regions of the ice sheet and their variations remain unknown, except of Dome C and Kohnen Station, where limited observations were recently conducted (Casado et al., 2016). Along the transect from coastal Zhongshan Station to Dome A (the summit of the East Antarctic), previous studies have used surface snow and snowpit samples to characterize the isotopic composition (δ18O, δ D, and 17O-excess) of precipitations, and the results indicate apparent latitudinal and altitudinal variations of water isotopes (Ding et al., 2011; Xiao et al., 2013; Pang et al., 2015), but the potential mechanism(s) controlling the observed spatial variations of snow isotopic composition is still a key issue (Pang et al., 2019). Data of atmospheric water vapor isotopic composition covering the same region would provide additional information that is useful for understanding the air–snow exchange of moistures and the associated isotope processes. In this study, we conducted field real-time measurements of water vapor isotopes during the 31st Chinese National Antarctica Research Expedition (CHINARE 31) from December 2014 to January 2015, along the transect from the Zhongshan Station to Dome A. Data were used to characterize the spatial variations in atmospheric moisture isotopes and the relationship between isotopic data and climatic/meteorological parameters, to explore the possible moisture source and the transport route.
Field Measurements and Data Calibration
Instrumentation and Field Measurements
We conducted field measurements of water vapor isotopes along the transect from Zhongshan Station to Dome A (80°22′51″S, 77°27′23″E, 4,093 m above the sea level, the summit of the Antarctic ice sheet) in East Antarctica. This transect covers about 1,250 km with elevation rising more than 4,000 m from the coast to Dome A. In these regions, the snow accumulation rates vary between ∼70 and ∼9 cm a−1(Ding et al., 2011). The field measurements were conducted in austral summer over the period of December 2014 to January 2015 when the expedition team traveled from the coast to Dome A. The atmospheric water vapor isotope composition measurements were performed every day along the route when the expedition team camped (Figure 1A).
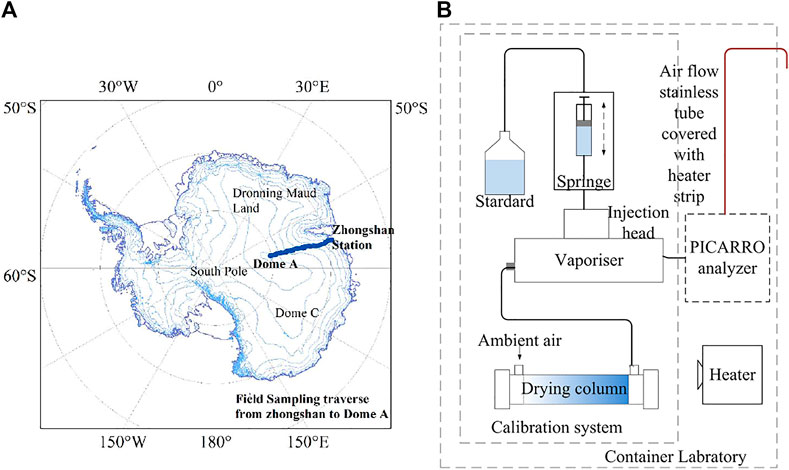
FIGURE 1. (A) Sites (blue dots) of in situ measurements along the transect from the Zhongshan station to Dome A; (B) Schematics of the field instrumentation used for in situ measurements of water vapor isotopic composition.
Measurements of water vapor isotopes were performed using a Wavelength Scanned Cavity Ring-Down Spectrometry analyzer (PICARRO Inc., 1102-i). The analyzer was installed in a laboratory cabin modified by a container, which was installed on sledge. When camped, the carry-on container was removed from the sledge and placed upwind 50 m away from the camp site in order to prevent pollution from the power generator and any artificial effects during the measurements, and then the electric heat booster would warm up the temperature in the container laboratory cabin to about 20°C, and the tube will get heated well above the dew point temperature of atmospheric air in order to prevent condensation in the line. Figure 1B displays the experimental setup used in the water vapor isotope monitoring and mainly illustrates the calibration system for the special extreme cold and dry conditions.
Simultaneous observations of meteorological conditions (e.g., temperature, relative humility (RH), and wind speed) and surface snow sampling were also conducted. Temperature and RH were measured by a Campbell Scientific HMP155A Vaisala Temperature and RH Probe, respectively. Wind speed and direction were monitored using a 05106 Wind Monitor with the 05603C Wind Sensor Interface.
Calibration Protocol
Calibration of the spectrometer is crucial for such dry conditions. Measurement deviations arise mainly from instrumental drift, systematic errors, and water vapor concentration. To correct such deviations, especially under polar conditions regarding the very low water vapor content, protocols have been developed and adapted with good performance (Steen-Larsen et al., 2013; Bonne et al., 2014). Briefly, calibration protocols include instrumental humidity-induced bias correction and VSMOW-SLAP calibration and drift correction. In this study, as the measurement is carried out on the remote ice sheet, the calibration will not be exactly the same as in the laboratory. The specific calibration processes are as follows: before the field work, isotopic values of liquid standards (NEEM (δ18O = −33.56‰ and δ D = −257.6‰), SLAP (δ18O = −55.50‰ and δ D = −427.5‰), and ROSS (δ18O = −18.75‰ and δ D = −144.6‰)) were measured in order to evaluate the sensitivity of the spectrometer, and the liquid standards calibration were performed as follows:
Then at the beginning of every measurement in each site, we conduct a standard measurement four times repeatedly, and followed by different level vapor concentrations (ppm), this work was carried out every day. Based on the repeated measure of standards, we carried instrumental error and drift calibration, then the calibrated vapor concentration bias based on different level vapor standards (showed in Figure 2). We observed that the measurement precision and stability decreased with reduced humidity, and especially at humidity below 1,000 ppm, the uncertainties of the measurements dropped to 8.2 and 1.3‰ for δ D and δ18O, respectively, as the primary goal is to disentangle the separate influences to the water cycle and large spatial scale characteristic, postdeposition, and circulation background, so we mainly focus on relative variations of the vapor isotopes.
Results and Discussion
Spatial Variation Characteristics
The observed atmospheric vapor hydrogen and oxygen isotopes changed regularly along the distance from the coast (Zhongshang Station) along with their changes of altitudes. As shown in Figure 3, along the ice sheet transect, atmospheric vapor δ18O varied from −40‰ to lower than −80‰ and δ D varied approximately from −280 to −390‰. The relationship between water vapor isotopes (δ18O, δ D, and d-excess) and temperature are plotted in Figure 4. Despite the large variability, we observed significant decreases in δ18O and δ D with distance increasing from the coast. And on the contrary, deuterium excess [d-excess, defined as d = δ D –8δ18O (Dansgaard, 1964)] increased with distance away from the coast and showed a significantly high value near inland Dome A where the elevation is above 3,000 m. What needs to be pointed out is that the vapor isotope variation is different among the near coast region and the inland high latitude region, especially in inner land near Dome A, d-excess became higher than other place. This phenomenon displays the temperature effect that dominant water stable isotope fractionation and intensified by the altitude effect. Because when more inner from the coast, the temperature became colder, and higher the elevation made heavy water isotopes to deplete more.
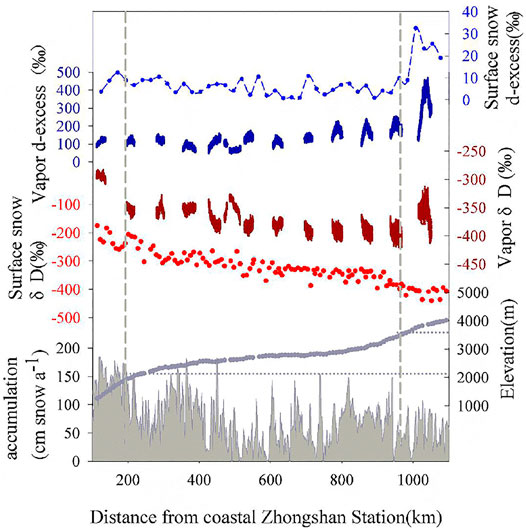
FIGURE 3. Synthesis multi-index comparison of isotopes and accumulation along transect from Zhongshan to Dome A, all these coincidently shows three climatic regimes, which controls the circulation and isotopic fractionation of the water cycle.
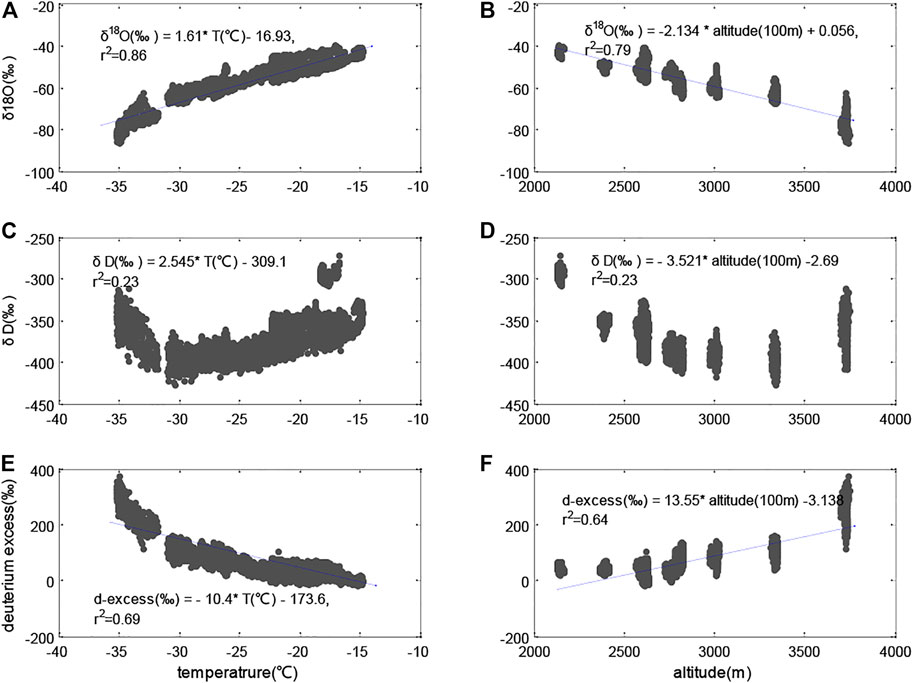
FIGURE 4. Atmospheric water vapor isotopic composition measured along the transect from Zhongshan Station to Dome A and the relationships between δ18O (A,B), δ D (C,D), and d-excess (E,F) with temperature and altitude, respectively.
The δ18O of atmospheric water vapor shows a temperature gradient of 1.61‰ °C–1, and an altitude gradient of –2.13‰ per 100 m. Similarly, δ D increased with temperature with a gradient of 2.54‰ °C–1 and decreased with altitude with a gradient of −3.52‰ per 100 m, and d-excess decreased with temperature with a gradient of −10.4‰ °C–1 and increased with altitude with a gradient of 13.55‰ per100 m. As shown in Figure 4, the data of interior region near Dome A show apparent different trends with altitudes compared to data from other regions. This may indicate different water vapor sources or dominant fractionation processes in inland plateau because of the extreme cool and dry conditions in comparison with the coast and transition regions from the coast to the plateau. In addition, we compared the relationships of isotopes with temperature and altitude for both surface snow and atmospheric water vapor and found that the isotopes of snow show similar temperature and altitude gradients as water vapor isotopes but the latter with larger variation.
We also observed diurnal cycles of water vapor isotopes along with that of air temperature and humidity (Figure 5), and the magnitude of the diurnal cycle increased as the distance increased from the coast.
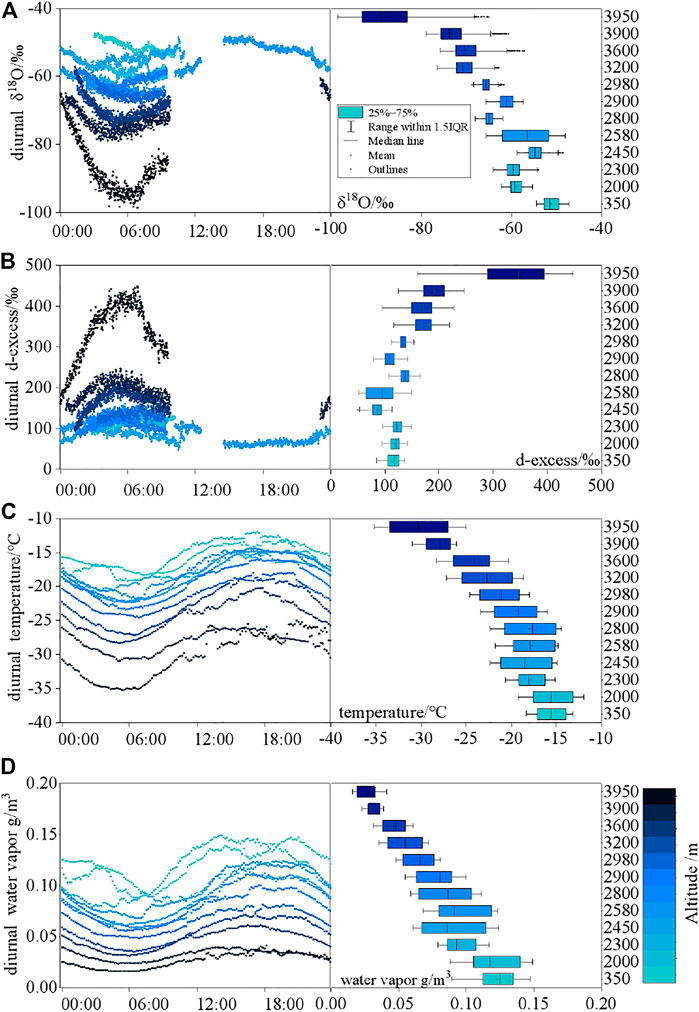
FIGURE 5. Diurnal cycles during the monitoring period. (A) δ18O, (B) d-excess, (C) 2 m air temperature, and (D) water vapor content. The color successive change represents gradual distance variation from near coastal to interior inland Antarctica. All the signals are dominated by the presence of diurnal cycles with the isotope variation amplitude increased to interior.
Implication to Moisture Transport
Based on the data of Antarctic snow and ice isotopes and isotope enabled general circulation models, potential moisture sources for the Antarctic ice sheet, especially the inland high elevation areas near Dome A have been explored (Noone and Simmonds, 2002; Sodemann and Stohl, 2009; Wang et al., 2013; Pang et al., 2015). But up to date, there is still no agreement on the origin of inland Antarctic precipitation (Ding et al., 2015). One of the many factors limits the understanding of inland moisture sources is the insufficient of water vapor isotope data.
For regions below 2,000 m, which are within 200 km from the coast, it has already reached a consensus that the steep coastal orography effectively blocks air masses from mid- and high latitudes with cold potential temperatures and prevents the air masses from penetrating to higher elevations to reach inland Antarctica (Masson-Delmotte et al., 2008), which leads to frequent precipitations in the coastal regions with high snow accumulation of about 100–200 kg m-1a-1 (Ding et al., 2015). Therefore, in this region, the moisture source and the isotopes of water vapor and precipitation are dominated by marine moisture near the coast (Masson-Delmotte et al., 2008; Becagli et al., 2017). For the vast inland areas with elevations between 2,000 and 3,000 m, atmospheric water vapor isotopes were depleted compared to the coast region. This pattern is consistent with the spatial variations in accumulation rates. Accumulation rates decrease from the coast to inland, and lower accumulation rates indicate drier air masses than the coastal region. At the same time, more moisture with depleted isotope compositions prevailing with katabatic wind come from the plateau, where the moistures are more affected by the upper stratosphere. It is noteworthy that in these areas, with distance further from the coast, the vapor isotope composition gradually changed, indicating the intensified influence of fractionations of inner plateau (Figure 3).
Here, we focus on the atmospheric vapor δ D and δ18O of the central Antarctic Dome vicinity area. Apparently, the mechanism controlling isotope fractionation in Dome vicinity is distinct, characterized by much higher d-excess. It is known that central Antarctic plateau prevailed with katabatic wind and air descending, or anticyclonic type of weather. The latter is formed by the formation of the circumpolar vortex in the free atmosphere, which is characterized by low pressure in the center and with clockwise rotation. A previous study has already suggested the possible influence of stratospheric water vapor intrusion from stratosphere to troposphere exchange (STE) (Brewer, 1949; Dobson, 1956; Butchart, 2014).These influences of δ18O and d-excess caused by very strong Rayleigh distillation effects were showed in this study. Previous studies in Vostok (Winkler et al., 2013) revealed that the inland Vostok located within the Antarctica vortex about a quarter of precipitation originates from tropospheric snowfall, whereas 75% is due to hoar frost deposition and diamond dust fall, which may originate from the stratosphere. Despite stratospheric air contains a very low water content (4–6.5 ppm) and the annual supply of stratosphere vapor into troposphere is very small and have very little influence on tropospheric water vapor and isotopic composition. However, for the central Antarctic Dome A region, it may be more important because the extremely low water vapor content and the very low accumulation rate, even more most of the descending flux from the stratosphere occurs at this high latitudes, Dome A is located within the Antarctic descending vortex, which makes it more sensitive to stratospheric air masses input and have strong influence on atmosphere vapor isotope composition as our measurement indicated.
Inland Snow–Air Sublimation/Condensation and the Isotopic Processes
The interior Antarctic region is located within the Antarctic vortex and characterized by very dry and cold backgrounds. The dry and cold conditions also cause substantial isotope exchange between air and snow through supersaturation sublimation and condensation. During our monitoring period, the signals of water vapor isotopes δ18O, δ D, 2 m air temperature, vapor water content (humidity), and d-excess showed apparent diurnal cycles, and the magnitudes of the isotope diurnal variations increased as the distance increased from the coast and close to Dome A. The field observations were conducted when camped every day and were mainly in local “nighttime,” even though the Sun never actually passed below the horizon; however, we have also obtained a whole day data when camped in Taishan Station from 21 December to 23 December. Figure 4 displays the daily isotope and temperature variations from the coastal region to the interior plateau; it is notable that the vapor isotopes varied synchronously with temperature and humidity diurnally, with more depleted isotopes in colder temperatures. Moreover, the inland Dome region is marked by larger magnitude of the diurnal cycle under the colder and drier conditions. These observations suggest substantial temperature-dependent fractionations in Antarctica snow surface and kinetic isotope fractionation occurred in the interior Dome area and that dominates local isotope variations(Ritter et al., 2016; Touzeau et al., 2016; Pang et al., 2019; Hughes et al., 2021), as what has been observed in the Greenland and Antarctic Dronning Maud Land (Steen-Larsen et al., 2013; Steen-Larsen et al., 2014; Ritter et al., 2016).
Since the saturated water vapor pressure over ice is less than that over water, the atmosphere over the Antarctic plateau is saturated (or even supersaturated) by moisture in relation to ice, which favors the formation and growth of ice crystals. Observations showed that sublimation (as high as 10–20% of the total precipitation) mainly happens when air temperature is below −15°C. Thus, the seemly temperature-dependent isotope diurnal cycles of our observation could be deemed to frequent snow–air isotope exchange under an approximate equilibrium fractionation status in sublimation/condensation cycles, and this could also explain that the snow surface was successively acting as a sink during the night and as a source during the day. Because the inland high plateau accumulation is very low, it is not difficult to understand the very high d-excess of isotope composition in both atmosphere and surface was principally influenced by local effects of sublimation and condensation, the partially stratosphere descended air mass with very depleted isotope make the surface snow analogous, and d-excess varied stronger due to very low condensation temperature (<−30°C).
Conclusion
In this study, we implemented atmosphere water vapor isotope measurement near the ice sheet surface of East Antarctica, the observation data revealed spatial variation characteristic and influences to the water cycle. Identical measurements in the field showed spatial variation characteristic of atmosphere water vapor isotope composition from Zhongshan Station to Dome A, with a significant interior gradual decrease of δ18O varies from −40‰ to lower than −80, and δ D varies approximately from −410 to −280‰. The relation between vapor isotope and temperature/altitude revealed distinct temperature gradient and altitude gradient. In addition, the distinct spatial variation of vapor isotopic composition indicated three subgroups of vapor δ18O vs. δ D relationship, denoting three different regimes controlling the inland water cycle and corresponding fractionation processes, therefore dividing as the coastal steep area below 2,000 m, the vast inland area with elevation between 2,000 and 3,000 m, and high central plateau.
Additionally, the observed high inland Antarctica water vapor may indicate the substantial influence of stratosphere air and vapor intrusion derived by Brewer–Dobson circulation from low latitudes, which may no longer be considered insignificant for origination of vapor and isotopic composition in central Antarctica plateau. Another conclusion is the signals of water vapor isotopes δ18O, δ D, and air temperature diurnal cycles in Antarctica plateau with amplitude increased for more interior sites, which implied the substantial domination role of supersaturation sublimation/condensation effect in inland; this temperature-related isotope diurnal cycles could attribute to frequent snow–air isotope exchange under the extreme cold equilibrium fractionation condition sublimation/condensation cycles.
In conclusion, our study opens new perspectives on the influence of supersaturation sublimation/condensation effects and postdeposition effects on water stable isotope signal recorded in deep ice cores, and also identifies particular moisture sources and water cycle regimes of inland snow and ice. However, more accurate atmosphere vapor isotope observation and modeling studies are needed for further understanding of past ice core records.
Data Availability Statement
The original contributions presented in the study are included in the article; further inquiries can be directed to the corresponding author.
Author Contributions
JL led the conception and design of the manuscript, collected and analyzed data, and wrote the manuscript. All authors contributed to the development of ideas and manuscript revision, and approved the submitted version.
Funding
This study is supported by the National Science Foundation of China (41801054, 41771064, and 42071086) and the Basic Research Fund of the Chinese Academy of Meteorological Sciences (Grant Nos. 2021Y021 and 2021Z006).
Conflict of Interest
The authors declare that the research was conducted in the absence of any commercial or financial relationships that could be construed as a potential conflict of interest.
Publisher’s Note
All claims expressed in this article are solely those of the authors and do not necessarily represent those of their affiliated organizations, or those of the publisher, the editors, and the reviewers. Any product that may be evaluated in this article, or claim that may be made by its manufacturer, is not guaranteed or endorsed by the publisher.
Acknowledgments
The authors are grateful for logistical and financial support provided by the Chinese National Antarctic Research Expedition. We would like to thank professor Lei Geng from University of University and Technology of China (Hefei) for manuscript revision, and pengling Lin and Jia Du for data procession.
References
Augustin, L., Barbante, C., Barnes, P. R. F., Marc Barnola, J., Bigler, M., Castellano, E., et al. (2004). Eight Glacial Cycles from an Antarctic Ice Core. Nature 429 (6992), 623–628. doi:10.1038/nature02599
Becagli, S., Proposito, M., Benassai, S., Flora, O., Genoni, L., Gragnani, R., et al. (2017). Chemical and Isotopic Snow Variability in East Antarctica along the 2001/02 ITASE Traverse. Ann. Glaciol. 39, 473–482. doi:10.3189/172756404781814636
Bonne, J.-L., Masson-Delmotte, V., Cattani, O., Delmotte, M., Risi, C., Sodemann, H., et al. (2014). The Isotopic Composition of Water Vapour and Precipitation in Ivittuut, Southern Greenland. Atmos. Chem. Phys. 14 (9), 4419–4439. doi:10.5194/acp-14-4419-2014
Brewer, A. W. (1949). Evidence for a World Circulation provided by the Measurements of Helium and Water Vapour Distribution in the Stratosphere. Q.J R. Met. Soc. 75 (326), 351–363. doi:10.1002/qj.49707532603
Butchart, N. (2014). The Brewer‐Dobson Circulation. Rev. Geophys. 52 (2), 157–184. doi:10.1002/2013RG000448
Cappa, C. D., Hendricks, M. B., DePaolo, D. J., and Cohen, R. C. (2003). Isotopic Fractionation of Water during Evaporation. J. Geophys. Res. Atmospheres 108 (D16), 4526. doi:10.1029/2003jd003597
Casado, M., Landais, A., Masson-Delmotte, V., Genthon, C., Kerstel, E., Kassi, S., et al. (2016). Continuous Measurements of Isotopic Composition of Water Vapour on the East Antarctic Plateau. Atmos. Chem. Phys. 16 (13), 8521–8538. doi:10.5194/acp-16-8521-2016
Casado, M., Landais, A., Picard, G., Münch, T., Laepple, T., Stenni, B., et al. (2018). Archival Processes of the Water Stable Isotope Signal in East Antarctic Ice Cores. The Cryosphere 12 (5), 1745–1766. doi:10.5194/tc-12-1745-2018
Dansgaard, W., Johnsen, S. J., Møller, J., and Langway, C. C. (1969). One Thousand Centuries of Climatic Record from Camp Century on the Greenland Ice Sheet. Science 166(3903), 377–381. doi:10.1126/science.166.3903.377
Dansgaard, W. (1964). Stable Isotopes in Precipitation. Tellus 16 (4), 436–468. doi:10.3402/tellusa.v16i4.8993
Ding, M., Xiao, C., Jin, B., Ren, J., Qin, D., and Sun, W. (2010). Distribution of δ 18O in Surface Snow along a Transect from Zhongshan Station to Dome A, East Antarctica. Chin. Sci. Bull. 55 (24), 2709–2714. doi:10.1007/s11434-010-3179-3
Ding, M., Xiao, C., Li, C., Qin, D., Jin, B., Shi, G., et al. (2015). Surface Mass Balance and its Climate Significance from the Coast to Dome A, East Antarctica. Sci. China Earth Sci. 58 (10), 1787–1797. doi:10.1007/s11430-015-5083-9
Ding, M., Xiao, C., Li, Y., Ren, J., Hou, S., Jin, B., et al. (2011). Spatial Variability of Surface Mass Balance along a Traverse Route from Zhongshan Station to Dome A, Antarctica. J. Glaciology 57 (204), 658–666. doi:10.3189/002214311797409820
Dobson, G. M. B. (1956). Origin and Distribution of the Polyatomic Molecules in the Atmosphere. Proc. R. Soc. Lond. Ser. A, Math. Phys. Sci. 236 (1205), 187–193. doi:10.1098/rspa.1956.0127
Ekaykin, A. A., Lipenkov, V. Y., Kuzmina, I. N., Petit, J. R., Masson-Delmotte, V., and Johnsen, S. J. (2004). The Changes in Isotope Composition and Accumulation of Snow at Vostok Station, East Antarctica, over the Past 200 Years. Ann. Glaciol. 39 (1), 569–575. doi:10.3189/172756404781814348
Frezzotti, M., Gandolfi, S., Marca, F. L., and Urbini, S. (2002). Snow Dunes and Glazed Surfaces in Antarctica: New Field and Remote-Sensing Data. Ann. Glaciol. 34 (1), 81–88. doi:10.3189/172756402781817851
Fudge, T. J., Steig, E. J., Markle, B. R., Schoenemann, S. W., Ding, Q., Taylor, K. C., et al. (2013). Onset of Deglacial Warming in West Antarctica Driven by Local Orbital Forcing. Nature 500 (7463), 440–444. doi:10.1038/nature12376
Gao, J., Yao, T., Masson-Delmotte, V., Steen-Larsen, H. C., and Wang, W. (2019). Collapsing Glaciers Threaten Asia's Water Supplies. Nature 565, 19–21. doi:10.1038/d41586-018-07838-4
Hughes, A. G., Wahl, S., Jones, T. R., Zuhr, A., Hörhold, M., White, J. W. C., et al. (2021). The Role of Sublimation as a Driver of Climate Signals in the Water Isotope Content of Surface Snow: Laboratory and Field Experimental Results. The Cryosphere 15 (10), 4949–4974. doi:10.5194/tc-15-4949-2021
Jouzel, J., Alley, R. B., Cuffey, K. M., Dansgaard, W., Grootes, P., Hoffmann, G., et al. (1997). Validity of the Temperature Reconstruction from Water Isotopes in Ice Cores. J. Geophys. Res. 102 (C12), 26471–26487. doi:10.1029/97JC01283
Jouzel, J., and Masson‐Delmotte, V. (2010). Paleoclimates: what Do We Learn from Deep Ice Cores? Wires Clim. Change 1 (5), 654–669. doi:10.1002/wcc.72
Krinner, G., Genthon, C., and Jouzel, J. (1997). GCM Analysis of Local Influences on Ice Coreδsignals. Geophys. Res. Lett. 24 (22), 2825–2828. doi:10.1029/97GL52891
LeGrande, A. N., and Schmidt, G. A. (2006). Global Gridded Data Set of the Oxygen Isotopic Composition in Seawater. Geophys. Res. Lett. 33 (12), L12604. doi:10.1029/2006gl026011
Majoube, M. (1970). Fractionation Factor of 18O between Water Vapour and Ice. Nature 226 (5252), 1242. doi:10.1038/2261242a0
Masson-Delmotte, V., Buiron, D., Ekaykin, A., Frezzotti, M., Gallée, H., Jouzel, J., et al. (2011). A Comparison of the Present and Last Interglacial Periods in Six Antarctic Ice Cores. Clim. Past 7 (2), 397–423. doi:10.5194/cp-7-397-2011
Masson-Delmotte, V., Hou, S., Ekaykin, A., Jouzel, J., Aristarain, A., Bernardo, R. T., et al. (2008). A Review of Antarctic Surface Snow Isotopic Composition: Observations, Atmospheric Circulation, and Isotopic Modeling. J. Clim. 21 (13), 3359–3387. doi:10.1175/2007JCLI2139.1
Merlivat, L., and Nief, G. (1967). Fractionnement isotopique lors des changements d'état solide-vapeur et liquide-vapeur de l'eau Á des températures inférieures Á 0°C. Tellus 19 (1), 122–127. doi:10.3402/tellusa.v19i1.9756
Münch, T., and Laepple, T. (2018). What Climate Signal Is Contained in Decadal- to Centennial-Scale Isotope Variations from Antarctic Ice Cores? Clim. Past 14 (12), 2053–2070. doi:10.5194/cp-14-2053-2018
Noone, D., and Simmonds, I. (2002). Annular Variations in Moisture Transport Mechanisms and the Abundance of δ 18 O in Antarctic Snow. J.‐Geophys.‐Res. 107 (D24), ACL 3-1–ACL 3-11. doi:10.1029/2002JD002262
Pang, H., Hou, S., Landais, A., Masson‐Delmotte, V., Jouzel, J., Steen‐Larsen, H. C., et al. (2019). Influence of Summer Sublimation on δD, δ 18 O, and δ 17 O in Precipitation, East Antarctica, and Implications for Climate Reconstruction from Ice Cores. J. Geophys. Res. Atmos. 124 (13), 7339–7358. doi:10.1029/2018JD030218
Pang, H., Hou, S., Landais, A., Masson-Delmotte, V., Prie, F., Steen-Larsen, H. C., et al. (2015). Spatial Distribution of 17O-Excess in Surface Snow along a Traverse from Zhongshan Station to Dome A, East Antarctica. Earth Planet. Sci. Lett. 414, 126–133. doi:10.1016/j.epsl.2015.01.014
Ritter, F., Steen-Larsen, H. C., Werner, M., Masson-Delmotte, V., Orsi, A., Behrens, M., et al. (2016). Isotopic Exchange on the Diurnal Scale between Near-Surface Snow and Lower Atmospheric Water Vapor at Kohnen Station, East Antarctica. The cryosphere 10 (4), 1647–1663. doi:10.5194/tc-10-1647-2016
Sodemann, H., and Stohl, A. (2009). Asymmetries in the Moisture Origin of Antarctic Precipitation. Geophys. Res. Lett. 36 (22), L22803. doi:10.1029/2009gl040242
Steen-Larsen, H. C., Johnsen, S. J., Masson-Delmotte, V., Stenni, B., Risi, C., Sodemann, H., et al. (2013). Continuous Monitoring of Summer Surface Water Vapor Isotopic Composition above the Greenland Ice Sheet. Atmos. Chem. Phys. 13 (9), 4815–4828. doi:10.5194/acp-13-4815-2013
Steen-Larsen, H. C., Masson-Delmotte, V., Hirabayashi, M., Winkler, R., Satow, K., Prié, F., et al. (2014). What Controls the Isotopic Composition of Greenland Surface Snow? Clim. Past 10 (1), 377–392. doi:10.5194/cp-10-377-2014
Touzeau, A., Landais, A., Stenni, B., Uemura, R., Fukui, K., Fujita, S., et al. (2016). Acquisition of Isotopic Composition for Surface Snow in East Antarctica and the Links to Climatic Parameters. The Cryosphere 10 (2), 837–852. doi:10.5194/tc-10-837-2016
Wang, Y., Hou, S., Masson-Delmotte, V., and Jouzel, J. (2010). A Generalized Additive Model for the Spatial Distribution of Stable Isotopic Composition in Antarctic Surface Snow. Chem. Geology. 271 (3–4), 133–141. doi:10.1016/j.chemgeo.2010.01.004
Wang, Y., Sodemann, H., Hou, S., Masson-Delmotte, V., Jouzel, J., and Pang, H. (2013). Snow Accumulation and its Moisture Origin over Dome Argus, Antarctica. Clim. Dyn. 40 (3), 731–742. doi:10.1007/s00382-012-1398-9
Werner, M., Langebroek, P. M., Carlsen, T., Herold, M., and Lohmann, G. (2011). Stable Water Isotopes in the ECHAM5 General Circulation Model: Toward High-Resolution Isotope Modeling on a Global Scale. J. Geophys. Res. Atmospheres 116 (D15), D15109. doi:10.1029/2011jd015681
Winkler, R., Landais, A., Risi, C., Baroni, M., Ekaykin, A., Jouzel, J., et al. (2013). Interannual Variation of Water Isotopologues at Vostok Indicates a Contribution from Stratospheric Water Vapor. Proc. Natl. Acad. Sci. 110 (44), 17674–17679. doi:10.1073/pnas.1215209110
Keywords: atmospheric water vapor isotope, Antarctic, Dome Argus, Brewer–Dobson circulation, water cycle
Citation: Liu J, Du Z, Zhang D and Wang S (2022) Diagnoses of Antarctic Inland Water Cycle Regime: Perspectives From Atmospheric Water Vapor Isotope Observations Along the Transect From Zhongshan Station to Dome A. Front. Earth Sci. 10:823515. doi: 10.3389/feart.2022.823515
Received: 27 November 2021; Accepted: 20 January 2022;
Published: 24 February 2022.
Edited by:
Xichen Li, Chinese Academy of Sciences (CAS), ChinaReviewed by:
Amzad Hussain Laskar, Physical Research Laboratory, IndiaCopyright © 2022 Liu, Du, Zhang and Wang. This is an open-access article distributed under the terms of the Creative Commons Attribution License (CC BY). The use, distribution or reproduction in other forums is permitted, provided the original author(s) and the copyright owner(s) are credited and that the original publication in this journal is cited, in accordance with accepted academic practice. No use, distribution or reproduction is permitted which does not comply with these terms.
*Correspondence: Jingfeng Liu, bGl1amluZ2ZlbmdAcGt1LmVkdS5jbg==