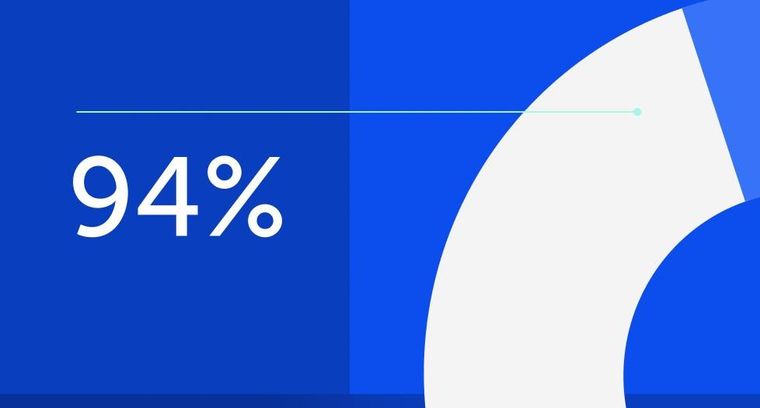
94% of researchers rate our articles as excellent or good
Learn more about the work of our research integrity team to safeguard the quality of each article we publish.
Find out more
ORIGINAL RESEARCH article
Front. Earth Sci., 21 February 2022
Sec. Geochemistry
Volume 10 - 2022 | https://doi.org/10.3389/feart.2022.809418
This article is part of the Research TopicIsotopic Geochemistry of Natural GasView all 14 articles
A series of investigations have been conducted concerning the study of traditional stable isotopes and rare gas stable isotopes in natural gas. However, little is known regarding non-traditional stable isotopes of mercury in natural gas, especially in the development and utilization of shale gas in recent years. In fact, the presence of mercury in natural gas (including shale gas) provides a basis for research on mercury isotopes. Mercury was extracted from shale gas at the Wufeng-Longmaxi Formation in the YS108 block of the Zhaotong National shale gas demonstration area in the Sichuan Basin by using an acid potassium permanganate solution, followed by the analysis of mercury content and stable isotope composition. The mercury content in the marine shale gas at the Wufeng-Longmaxi Formation ranged from 171 to 2,906 ng/m3, with an average of 1,551.08 ± 787.08 ng/m3 (n = 37, 1 SD). The Δ199Hg values of mercury stable isotopes range from 02‰ to 0.39‰, with an average of 22‰ ± 0.08‰ (n = 37, 1 SD); the δ202Hg values range from −1.68‰ to −0.04‰, with an average of −0.87‰ ± 0.31‰ (n = 37, 1 SD), which are significantly different from the Δ199Hg and δ202Hg information of coalbed gas, but similar to the Δ199Hg and δ202Hg information of terrestrial oil-type gas and the Δ199Hg in the main hydrocarbon-forming organic matter of lower organisms such as algae (t-test, p > 0.05). This indicates that terrestrial target strata with abundant algae or strata with positive Δ199Hg are the target strata for the exploration of terrestrial oil and gas.
As proposed by Ozerova (1983), the concentration of mercury (elemental mercury, Hg0) reflects the origin of natural gas. Dai (1992) showed that Hg0 concentrations are greater than 700 ng/m3 in coalbed gas and less than 500 ng/m3 in oil-type gas. However, Hg0 concentrations vary significantly within a single gas field (Liu, 2013). As a result, the Hg0 concentration alone is an imprecise estimate of the natural gas source.
Mercury is commonly found in oil and gas (including shale gas) and mainly exists in the form of elemental mercury, which provides a basis for the study of mercury isotopes. There are seven stable isotopes of mercury: 196Hg (0.15%), 198Hg (9.97%), 199Hg (16.87%), 200Hg (23.10%), 201Hg (13.18%),202Hg (29.86%), and 204Hg (6.87%). The relative mass difference between the isotopes was up to 4%. Mercury is the only identified heavy metal element that can undergo mass-dependent fractionation (MDF) and mass-independent fractionation (MIF). A series of important natural processes involved in the geochemical cycle of mercury, such as volatilization and evaporation (Lefticariu et al., 2011), oxidation/reduction (Foucher et al., 2009), microbial methylation/demethylation (Feng et al., 2010; Yin et al., 2013), photoreduction reactions (Bergquist and Blum, 2007; Kwon et al., 2020), and natural hydrothermal systems [(Smith et al., 2008; Stetson et al., 2009), can lead to significant mercury isotope mass-dependent fractionation. Photoreaction is the main natural process leading to the mass-independent fractionation of mercury stable isotopes (Bergquist and Blum, 2007; Kwon et al., 2020), where mercury isotopes are essential in tracing mercury sources and geochemical processes. Many studies have been conducted on mercury isotopes in organic matter correlated to oil and gas, such as algae and phytoplankton (Perrot et al., 2012; Tsui et al., 2012), invertebrates (Blum et al., 2014), lake sediments, marine sediments (Feng et al., 2010; Ma et al., 2013), terrestrial plants (Sun et al., 2017; Wang et al., 2017), lichens (Carignan et al., 2009), peat (Enrico et al., 2017; Enrico et al., 2016; Shi et al., 2011), coal (Biswas et al., 2008; Lefticariu et al., 2011; Sun et al., 2016), and soil (Foucher et al., 2009; Yin et al., 2013). The Hg isotopic ratios show promise as a method for distinguishing different sources of natural gas. Stable isotopes of mercury in oil-type gas and coalbed gas have been studied (Washburn et al., 2018; Tang et al., 2019); however, stable isotopes of mercury in shale gas have not yet been reported.
Shale gas, as a clean, efficient, and low-carbon unconventional natural gas, has multiple effects such as economy, society, and environment. The development and utilization of shale gas help achieve the goals of “carbon peak” and “carbon neutrality,” which has become an important part of the energy structure of the world. The research object of this study is the existing shale gas in the Zhaotong Block of the Sichuan Basin. The mercury in shale gas was collected on-site, whereby we analyzed the mercury content and stable isotope composition of mercury in the samples. Further, we compared the mercury stable isotope compositions of oil-type gas and coalbed gas in previous studies. As such, we summarized, herein, the general characteristics of the mercury isotope composition of shale gas, and discussed the main material sources and exploration indicative significance of shale gas.
The sampling site is located in the YS108 Block, Zhaotong National Shale Gas Demonstration Area, Sichuan Basin (Figure 1). The block is one of the first national shale gas demonstration zones in China and is an important part of commercial shale gas in China (Chen et al., 2019). The YS108 block is located in Gongxian County and Junlian County, Yibin City, Sichuan Province, adjacent to the Changning shale gas block, which is structurally located at the southern edge of the low-steep fold belt in the southern Sichuan Tai Depression and adjacent to the northern depression of Yunnan and Guizhou (Figure 1A). The main gas-producing zone is the upper Wufeng Formation of the Ordovician and lower Longmaxi Formation of Silurian, characterized by a buried depth of 2,000–2,500 m, and it is the main zone for shale gas horizontal well development in the block (Figure 1B). As a whole, it belongs to a set of black siliceous shale and organic-rich black carbonaceous shale deposited in marine deepwater shelf subfacies, with large sedimentary thickness (30–40 m), high organic matter abundance, thermal evolution degree (TOC ≥2.0%, Ro: 2.0–3.5%), adequate gas content (3.0–4.6 m3/t), adequate reservoir performance (Φ: 1.56–5.27%), and high compressibility (brittleness index: 44%–78%). The target formation has the characteristics of overpressure (pressure coefficient 1–1.9), and is a favorable shale gas development formation (Dai et al., 2014; Wang et al., 2016).
FIGURE 1. Structural map of Zhaotong Demonstration Zone (A) and YS108 Block (B) [Figure A is modified according to Xu et al. (2019), and Figure B is modified according to Huang et al. (2019)]. In Figure B, the red wells are the sampling sites, and the horizontal wells of the related wells are drawn. The yellow and gray wells are other wells in the block.
The mercury in the shale gas of seven gas accumulation wells from three gas accumulation platforms in Block YS108 of Zhaotong National Shale Gas Demonstration Zone in Sichuan Basin was collected on-site, denoted as H1-3, H1-6, H3-1, H3-2, H11-1, H11-2, and H11-3 gas accumulation wells. A total of 37 samples of mercury capture fluid from shale gas were collected.
Mercury in shale gas is captured by potassium permanganate (KMnO4) and sulfuric acid (H2SO4) mixture: Hg0 in shale gas was oxidized to ionic form mercury and captured and retained in acidic potassium permanganate solution (Figure 2) by taking advantage of the strong oxidation and super absorption of mercury in acidic potassium permanganate solution, such that the acidic potassium permanganate solution was recovered as a sample for mercury content and mercury isotope analysis. In previous studies (Tang et al., 2019), we used three series of acid potassium permanganate cylinders to capture mercury in oil-type gas and coalbed gas, but laboratory simulations and mercury isotope tests found that Hg0 is mainly enriched in the first impinger (the percentage of mercury content is 76%–97%), and the isotopic composition of total mercury is consistent with that of the solution of the first impinger. Therefore, we simplified the mercury capture device for shale gas, and the main body of the mercury capture device was composed of three impingers (Environmental Supply Company, United States, 500 ml for each sampling bottle) in series. The first impinger was an empty bottle for separating water vapor in shale gas, ensuring the concentration of acidic potassium permanganate mixture in the second impinger, mercury capture efficiency, and mercury capture time. The second impinger contained 100 ml of 4% (w/v) KMnO4 and 1% (v/v) H2SO4. Hg0 in shale gas was oxidized to ionic mercury and captured and retained in an acid potassium permanganate solution. The acid potassium permanganate solution was recovered as a sample for mercury content and mercury isotope analysis in shale gas. The third impinger contained approximately 100 mg of discolored silica gel, which was used to absorb the water vapor attached to the remaining gas to prevent its impact on the subsequent gas flowmeter. The three impingers were fixed in a cold-water tank.
FIGURE 2. Mercury capture device for shale gas [Description: 1. Sampling port; 2. Empty bottles; 3. Impact bottle with 4% (W/V) KMnO4+1% (V/V) H2SO4 solution; 4. Impact bottle with color-changing silicone; 5. Gas flowmeter].
To ensure the authenticity of mercury capture in shale gas, the used chemical reagents, drugs, impingers, and their connecting tubes need to be processed in advance, which requires a low blank value of mercury. Potassium permanganate was purchased from the United States (mercury content is <0.002 ng/ml), sulfuric acid is eminently pure (mercury content is <0.004 ng/ml), and the impinger and its connecting parts are borosilicate glass bottles purchased from the United States and have been subjected to high-temperature treatment in a muffle furnace. The purchased PTFE pipe was used to connect the pipe from the shale gas wellhead to the impinger. Before sampling, the entire sampling device was directly flushed with the original natural gas of the shale gas wellhead for approximately 3–5 min, and the acid potassium permanganate mercury-capturing mixture prepared on-site was quickly filled and connected to the whole device. Each sample captures 1 m3 of mercury in shale gas, which ensures the representativeness of the capture shale gas and enriches enough mercury to meet the requirements of mercury isotope analysis. During the sampling process, the color of the mercury capture liquid should always be purple. Once it becomes colorless, the sampling should be immediately stopped. The acidic potassium permanganate mixture was recovered as the sample, and the volume of the mixture and gas flowmeter data were recorded. After each sampling, the mercury capture solution was recovered, and the volume was recorded with a measuring cylinder and transferred to a borosilicate glass bottle. Approximately 0.5 ml of potassium dichromate solution (30%, w/v) was immediately added, sealed, numbered, and transported back to the laboratory for testing.
The mercury content of the captured mercury from acid potassium permanganate was determined by using the RA-915 M mercury meter and its liquid attachment. After the standard curve was plotted depicting the mercury standard solution, approximately 10 ml of acidic KMnO4 solution sample was added to the centrifuge tube, and then approximately 0.5 ml of NH2OH-HCl solution with a mass volume fraction of 15% was slowly added with a pipette. Shaking resulted in the captured mercury solution to be colorless and transparent, where 5 ml of the sample solution was added with a pipette to a 20% (w/v) SnCl2 solution foaming bottle open on the left side of the liquid attachment. At this time, Hg2+ in the sample was reduced to Hg0 and was pumped by the instrument to the right foaming bottle containing 30% NaOH solution (the purpose was to remove the volatile acidic gas in the sample and prevent the host from being corroded). Hg0 was sent to the host of the mercury analyzer to determine the mercury content. The sampling process was powered by a constant-speed gas pump in the liquid attachment, and the average value of each sample was taken as the final result after two measurements (relative deviation less than 10%).
The mercury isotopic composition in the mercury capture solution was determined using multi-collector inductively coupled plasma mass spectrometry (MC-ICP-MS, Neptune Plus) at the Institute of Geochemistry, Chinese Academy of Sciences according to previous methods (Tang et al., 2017; Tang et al., 2019). The mercury concentration of the sample of mercury capture liquid to be diluted before the determination of mercury isotope was 0.5 ppb. A certain amount of mercury captured solution samples (calculated according to mercury content) with known mercury content were taken into a 15-ml centrifuge tube. At most, 0.5 NH2OH-HCl (30%, w/v) was dropped into the solution to make it colorless and transparent, and the solution was then diluted to 15 ml with secondary distilled water. The mercury concentrations of the process standard solution NIST SRM3133 and the external standard NIST SRM3177 at the time of injection were configured to be 0.5 ppb. The mass fractionation of mercury isotopes is usually expressed by the thousandth fractionation (‰) of δXXXHg, where δ refers to the deviation between the sample mercury isotope ratio and the standard mercury isotope ratio (Blum and Bergquist, 2007). For better data comparison, NIST SRM3133 Hg is usually used as the standard (Blum, 2012). The calculation formula is as follows:
where XXX refers to 199, 200, 201, 202, and 204, respectively. The mass-independent fractionation of mercury isotopes is represented by ΔxxxHg (‰), which refers to the deviation between the measured and theoretical values. It is specifically calculated according to the following formula (Blum and Bergquist, 2007):
The mercury content of shale gas in the YS108 block of the Zhaotong demonstration area is shown in Figure 3 and Supplementary Table S1 (Supporting Information). The mercury content of 37 shale gas samples ranged from 171 to 2,906 ng/m3, with an average of 1,551.08 ± 787.08 ng/m3 (n = 37, 1 SD). The mercury content in shale gas detected by Han et al. (2021) in the southern Sichuan Basin (mean: 1,420 ng/m3) is consistent with that detected by Han et al. (2021), which is significantly lower than the average mercury content in the coal-formed gas of the Shanshan oilfield (Li, 2020) and the oil-type gas of the Liaohe oilfield, and higher than that in the coalbed gas of the Hebi Coal Mine (Tang et al., 2019). The overall mercury content demonstrates coal-formed gas > oil-type gas > shale gas > coalbed gas, but the mercury content varies by one to several orders of magnitude.
Natural gas can be divided into organic and inorganic gases according to genetic type, among which organic gas includes oil-type gas mainly from marine or lacustrine sapropelic and sapropelic to humic-type organic matter and oil-type gas that forms crude oil, and coal-formed gas mainly from terrestrial humic-type organic matter (Ni et al., 2019; Li et al., 2012a). According to the lithology of the reservoir, natural gas can be divided into coal-formed gas (including coalbed gas generated by coal seams and coal-formed gas, produced by coal seams but migrated to other coal seams or other reservoirs), shale gas, and tight sandstone gas (Liu, 2018). It is generally believed that mercury in natural gas is mainly derived from organic matter in gas source rocks or crude oil. During the thermal evolution of organic matter into hydrocarbons, mercury accumulates with natural gas in the form of volatile components. The mercury accumulation capacity of terrestrial organic matter (such as coal and dispersed humus) is relatively strong (Liu et al., 2020; Li et al., 2012b). The mercury content is relatively high; therefore, the mercury content in coal-formed gas is generally higher than that in oil-type gas, and the organic matter content in shale gas is relatively lower than that in coal-formed gas and oil-type gas; therefore, the mercury content is also relatively low (Figure 3). The mercury content in coalbed gas is the lowest in mine no. 6 of the Hebi coal mine, which is related to the low mercury content in the coal seam. The mercury content of shale gas in the YS108 block of the Zhaotong demonstration area is also lower than the average mercury content (6,840 ng/m3) of over-mature marine gas (also oil-type gas) in the Sichuan Basin (Han et al., 2021).
In addition, the mercury content may be related to the state of natural gas occurrence. After the formation of alkane gas, it is stored in micron- or nanometer-sized reservoir pores in the form of free, adsorption, and dissolution (Gong et al., 2014). Mercury migrates with free natural gas in the form of steam during hydrocarbon generation. Coalbed gas and shale gas are self-generation and self-accumulation modes, respectively. About 70%–95% of coalbed gas exists in the adsorption state (Liu, 2018), the adsorption state of shale gas accounts for approximately 20%–85% of the total gas (Curtis, 2002; Montgomery et al., 2005), and the adsorption of shale gas at the Wufeng-Longmaxi Formation in Sichuan Basin accounts for approximately 40% (Li et al., 2018). Coal-formed gas and oil-type gas are mainly in the free state, CBM is mainly in the adsorption state, and shale gas has free and adsorbed gas. Therefore, the mercury content of coal-formed gas and oil-type gas is generally higher than that of shale gas and CBM, and the mercury content of CBM is the lowest. The mercury content in shale gas varies greatly between wells (Supplementary Table S1) mainly because of the maldistribution of organic matter in the source rock (Tian et al., 2014; Wu et al., 2016; Deng and Sun, 2019).
The mercury isotopic composition of shale gas in the Zhaotong YS108 block is shown in Figure 4. The Δ199Hg values (relative to NIST 3133) are positive (0.02‰–0.39‰), with an average of 22‰ ± 0.08‰ (n = 37,1 SD), which is consistent with the Hg-MIF values (Δ199Hg: +0.05‰–+0.15‰, with an average of 0.12‰ ± 0.03‰) of the Wufeng-Longmaxi black shale in the Sichuan Basin reported by Shen et al. (2019), indicating that there is no evident mass-independent fractionation of mercury isotopes during gas formation from gas source rock to shale gas owning to the lack of exposure to light in the underground gas formation process leading to the MIF of the mercury stable isotope. Although some scholars have reported that the stable isotopes of mercury produce very small Hg-MIF under dark conditions (Zheng and Hintelmann, 2010) (Blum et al., 2014; Tang et al., 2019), photochemical reduction is believed to be the most important process causing Hg-MIF. Therefore, it is considered that the mass-independent fractionation of the stable isotope of mercury does not occur in the underground dark environment of hydrocarbon generation, and the information characteristics of the mass-independent fractionation of the stable isotope of mercury in the organic matter of the gas source rocks are retained, which also indicates the main organic matter types of the gas source rocks. The δ202Hg values in shale gas range from −1.68‰ to −0.04‰, with an average of −0.87‰ ± 0.31‰ (n = 37, 1 SD), and the δ202Hg values in shale gas of the same formation range from −2.5‰ to −1.0‰ with an average value of −1.36‰ ± 0.34‰, n = 22, 2 SD) (Shen et al., 2019), but relatively positive, indicating that shale gas is more enriched in heavy isotopes of mercury than its source rock. Laboratory studies have demonstrated that many redox reactions involving Hg cause MDF including abiotic and biotic processes (Kritee et al., 2018; Wiederhold et al., 2010; Jiskra et al., 2012; Zheng et al., 2019); therefore, this study does not describe all geochemical processes that contribute to the mass fractionation of mercury isotopes.
FIGURE 4. Characteristics of shale gas Δ199Hg and δ202Hg (A) and Δ199Hg and δ 201Hg (B): The ellipse in Figure A shows the mean values of Δ199Hg and δ202Hg (inner ellipse) and 95% confidence level (outer ellipse).
It is generally believed that the nuclear volume effect (NVE) (Schauble, 2007) and magnetic isotope effect (MIE) (Buchachenko et al., 2007) are the main mechanisms for the generation of mercury stable isotope mass-independent fractionation (MIF), and the two can be effectively distinguished by the ratio of Δ199Hg/Δ201Hg. The ratio of MIFΔ199Hg/Δ201Hg caused by NVE was greater than 1.6, such as the mercury-thiol complex (Δ199Hg/Δ201Hg = 1.54) and mercury reduction process caused by abiotic action under dark conditions (Δ199Hg/Δ201Hg = 1.5–1.6) (Wiederhold et al., 2010; Zheng and Hintelmann, 2010) and elemental mercury volatilization (Δ199Hg/Δ201Hg = 2.0) (Estrade et al., 2009). The ratio of Δ199Hg/Δ201Hg of MIF caused by MIE is 1–1.36, such that the photoreduction reaction of HgIIaq Δ199Hg/Δ201Hg = 1.0 (Bergquist and Blum, 2007; Zheng and Hintelmann, 2009) and the photodegradation of methylmercury Δ199Hg/Δ201Hg = 1.36 (Bergquist and Blum, 2007). The mercury in the studied shale gas sample Δ199Hg/Δ201Hg = 1.001 ± 0.18 (Figure 4B) indicates that the main mechanism for the occurrence of MIF in the mercury isotope in shale gas is MIE caused by HgIIaq photoreduction reaction, which also indicates that the mercury in shale gas undergoes the photoreduction reaction of Hg2+ in water before deposition.
There are relatively few studies on mercury isotopes in natural gas (Tang et al., 2019; Washburn et al., 2018), and the development and utilization of mercury isotopes in shale gas in recent years have not been reported. Figure 5 summarizes the results of shale gas and Tang et al. (2019) on mercury isotopes in oil-type gas and coalbed gas.
FIGURE 5. Comparison of mercury isotopic composition in different types of natural gas: the data of oil-type gas and coalbed gas are quoted from the literature (Tang et al., 2019).
The Δ199Hg of shale gas in the Zhaotong YS108 block is positive and similar to that of oil-type gas in the Liaohe oilfield and Zhongyuan oilfield, and δ202Hg is also consistent (Figure 5), indicating that they have similar sources of mercury and types of organic matter. The Δ199Hg range of shale gas in the Zhaotong YS108 block is 0.02‰–0.39‰, with an average of 0.22‰ ± 0.08‰ (n = 37, 1 SD). The gas source rock is deep-sea sedimentary shale, and its hydrocarbon-forming organic matter is mainly rich in algae and low phytoplankton, and algae is the only confirmed lower plant with positive Δ199Hg at present (Figure 6, Perrot et al., 2012; Tsui et al., 2012). This is similar to the positive value in Δ199Hg of Ordovician black shale (Gong et al., 2017), Ordovician argillaceous limestone (Shen et al., 2019; Gong et al., 2017), and modern marine sediments (Gehrke et al., 2009; Ogrinc et al., 2019), which is also consistent with the positive value for Δ199Hg of marine shale gas and continental oil-type gas (Tang et al., 2019), but it is significantly different from the negative Δ199Hg value of coalbed gas and modern higher plants (Figure 6). Therefore, the isotopic composition of mercury in shale gas most likely retains that of hydrocarbon-forming algae and lower plankton. In fact, approximately 90% of the mercury in the ocean originates from atmospheric mercury deposition (Mason et al., 2012). Most of the mercury exists in the form of dissolved Hg2+ ions or other mercury oxides that are easily soluble in seawater. Algal organic matter is rich in −SH, which is thermodynamically favorable for the rapid uptake and accumulation of inorganic Hg2+ in seawater (Fitzgerald and Lamborg, 2014; Le Faucheur et al., 2014), allowing algae to reach a higher mercury concentration level. After algal death, the deposition of algal debris transfers mercury from surface waters to deeper waters and sediments, and the process is analogous to a “biological pump” of mercury (Hirner et al., 1990; Li et al., 2012b; Verburg et al., 2014). The average Hg content in algal organic matter in Arctic lake sediments before 1800 was as high as 159–776 μg/kg (n = 24), which is 2–39 times higher than that in the sediments, demonstrating the “biological pump” mechanism of algal cleaning of mercury in seawater (Outridge et al., 2019). Shale gas reservoirs in the Wufeng-Longmaxi Formation in the study area are mainly formed in a shelf retention environment with limited water circulation and are dominated by black carbonaceous shale and siliceous shale with rich organic matter content (Wu et al., 2016). Organic components such as planktonic algae, acritarch, bacteria, and animal detritus such as graptolite, chitin, and sponge needles have been observed in shales (Tengger et al., 2017; Nie et al., 2019). Ma et al. (2020) conducted hydrocarbon-generating thermal simulation experiments on shales and graptolites with different maturities in the upper Ordovician and lower Silurian and found that hydrocarbon-rich and fat-rich organic matter such as algae and acritarch are the main organic matter for shale gas generation. With an increase in burial depth and formation temperature, mercury in the complex and adsorbed state of algae organic matter gradually desorbed under thermal action and migrated to oil and gas reservoirs or oil reservoirs in the form of steam (Peng et al., 2019). Therefore, the Δ199Hg information in shale gas and the Δ199Hg of the main hydrocarbon-forming organic matter—algae (0.17‰–0.64‰, with an average of 0.39 ± 0.21‰, n = 4,1 SD) (Perrot et al., 2012; Tsui et al., 2012)—showed no significant difference (t-test, p > 0.05) and all values are positive, which also indicates that Δ199Hg in marine crude oil is similar to that of algae.
FIGURE 6. Mercury isotopic composition information of shale gas and its hydrocarbon generating medium Δ199Hg comparison, in which I-1 is Ordovician black shale [quoted from literature (Gong et al., 2017)], I-2 is Ordovician argillaceous limestone [quoted from literature (Shen et al., 2019; Gong et al., 2017)], I-3 is modern marine sediment [quoted from literature (Gehrke et al., 2009; Ogrinc et al., 2019)], I-4 is Arctic seawater [quoted from literature (Štrok et al., 2015)], I-5 is planktonic algae [quoted from literature (Perrot et al., 2012; Tsui et al., 2012)], II-1 is shale gas (this study), II-2 is continental oil-type gas [quoted from literature (Tang et al., 2019)] II-3 refers to coalbed gas [quoted from literature (Tang et al., 2019)], III-1 refers to leaves [quoted from literature (Demers et al., 2013; Zheng et al., 2016)], III-2 refers to litter on the Qinghai Tibet Plateau [quoted from literature (Wang et al., 2017)], and III-3 refers to freshwater sediments [quoted from literature (Gantner et al., 2009; Donovan et al., 2013; Sherman and Blum, 2013)].
The oil-type gas in the Liaohe and Zhongyuan oilfields is formed by the evolution of source rocks in continental lakes or delta sedimentary environments. The source rocks, herein, are continental dark mudstone and shale (E2-3s) of the Eocene Shahejie Formation. The sources of mercury in lakes and oceans are significantly different: atmospheric mercury deposition is the main entry pathway of mercury in pelagic sediments, while lake or delta sedimentary environments receive various forms of mercury from various sources, such as mercury dissolved in catchment runoff, tributaries, and adsorbed and fixed in particulate matter and sediments (Fitzgerald and Lamborg, 2014). There exist both higher plant and animal sources of mercury, as well as planktonic sources of mercury such as algae in lake and river delta sedimentary environments (Outridge et al., 2007).
The Δ199Hg values of freshwater sediments have both positive and negative values (Figure 6 III-3; Donovan et al., 2013; Gantner et al., 2009; Sherman and Blum, 2013), but in the continental oil-type gas, we studied Δ199Hg as positive (Figure 6 II-2, Tang et al., 2019). As mentioned earlier, algae are the only lower plants with positive Δ199Hg that have been confirmed so far, and the continental oil-type gas and mercury present in them are mainly derived from source rocks. Therefore, it is believed that the main hydrocarbon-forming organic matter of continental oil-type gas cannot come from higher plants but are mainly derived from plankton such as algae that self-accumulate in the sedimentary environment of the source rock. This also makes the Δ199Hg between shale gas in the Zhaotong YS108 block and oil-type gas in the Liaohe and Zhongyuan oilfields (Δ199Hg:0.06‰–0.30‰, mean 0.17‰ ± 0.06‰, n = 24, 1 SD) positive, showing no significant difference. The above results also indicate that the main organic matter of continental crude oil is abundant algae and other plankton in lake or delta environments, and Δ199Hg is positive. Therefore, continental oil and gas exploration can find algae-rich target strata or delineate the target strata with a positive Δ199Hg value. For example, most of the planktonic and algal fossils have been found in the source rocks of the continental oilfields in China, such as the Shengli, Maling, and Wangchang oilfields (Liu, 2018).
The Δ199Hg of shale gas in the Zhaotong YS108 block, oil-type gas from the Liaohe oilfield, and Zhongyuan oilfield is significantly different from that of coalbed gas in mine no. 6 of the Hebi Coal Mine (Figure 5). The coal seam in mine no. 6 of the Hebi coal mine is the continental coal seam of the Permian fluvial and lacustrine facies. The main coal-forming organic matter was terrestrial higher plants, and the Δ199Hg was negative (Figure 6). Therefore, the mercury Δ199Hg (−0.19‰ to −0.01‰, −0.11‰ ± 0.06‰, n = 7, 1 SD) in coalbed gas was also negative. Δ199Hg is significantly different from that of shale gas and oil-type gas.
The mercury content of marine shale gas in the YS108 block of Zhaotong demonstration area ranges from 171 to 2,906 ng/m3, with an average of 1,551.08 ± 787.08 ng/m3 (n = 37,1 SD). The Δ199Hg values of mercury isotopes range from 0.02‰ to 0.39‰, with an average of 0.22‰ ± 0.08‰ (n = 37, 1 SD), and the δ202Hg values range from −1.68‰ to −0.04‰, with an average of −0.87‰ ± 0.31‰ (n = 37, 1 SD), which are significantly different from the Δ199Hg and δ202Hg values of coalbed gas, but not significantly different from the Δ199Hg and δ202Hg values of terrestrial oil-type gas. We can use it as an indicator to search for algae-rich continental strata or delineate the strata with positive Δ199Hg as exploration targets.
The original contributions presented in the study are included in the article/Supplementary Material, Further inquiries can be directed to the corresponding authors.
ST: conceived the idea for this study and provided manuscript guidance; GZ: assisted in sample collection and manuscript revision; YD and ST: data analysis, manuscript writing and revision; XF, HZ and PL: helped supervise the projects. All authors listed have made a contribution to the work and approved it for publication.
This research was supported by the National Natural Science Foundation of China (41573006 and 41372360) and the CNPC (China National Petroleum Corporation) Scientific Research and Technology Development Project (Grant No. 2021DJ05).
Author GZ was employed by the company PetroChina.
The remaining authors declare that the research was conducted in the absence of any commercial or financial relationships that could be construed as a potential conflict of interest.
All claims expressed in this article are solely those of the authors and do not necessarily represent those of their affiliated organizations, or those of the publisher, the editors, and the reviewers. Any product that may be evaluated in this article, or claim that may be made by its manufacturer, is not guaranteed or endorsed by the publisher.
We thank Gaoen Wu of State Key Laboratory of Environmental Geochemistry, Chinese Academy of Sciences, for assistance in measuring Hg isotopes. We thank the editor and reviewers for their thoughtful comments, which significantly improved the quality of this paper.
The Supplementary Material for this article can be found online at: https://www.frontiersin.org/articles/10.3389/feart.2022.809418/full#supplementary-material
Bergquist, B. A., and Blum, J. D. (2007). Mass-Dependent and -Independent Fractionation of Hg Isotopes by Photoreduction in Aquatic Systems. Science 318 (5849), 417–420. doi:10.1126/science.1148050
Biswas, A., Blum, J. D., Bergquist, B. A., Keeler, G. J., and Xie, Z. (2008). Natural Mercury Isotope Variation in Coal Deposits and Organic Soils. Environ. Sci. Technol. 42 (22), 8303–8309. doi:10.1021/es801444b
Blum, J. D. (2012). “Applications of Stable Mercury Isotopes to Biogeochemistry,” in Handbook of Environmental Isotope Geochemistry: Vol I. Editor M. Baskaran (Berlin, Heidelberg: Springer Berlin Heidelberg), 229–245. doi:10.1007/978-3-642-10637-8_12
Blum, J. D., and Bergquist, B. A. (2007). Reporting of Variations in the Natural Isotopic Composition of Mercury. Anal. Bioanal. Chem. 388 (2), 353–359. doi:10.1007/s00216-007-1236-9
Blum, J. D., Sherman, L. S., and Johnson, M. W. (2014). Mercury Isotopes in Earth and Environmental Sciences. Annu. Rev. Earth Planet. Sci. 42 (1), 249–269. doi:10.1146/annurev-earth-050212-124107
Buchachenko, A. L., Ivanov, V. L., Roznyatovskii, V. A., Artamkina, G. A., Vorob’ev, A. K., and Ustynyuk, Y. A. (2007). Magnetic Isotope Effect for Mercury Nuclei in Photolysis of Bis(p-Trifluoromethylbenzyl)mercury. Dokl. Phys. Chem. 413, 39–41. doi:10.1134/S0012501607030013
Carignan, J., Estrade, N., Sonke, J. E., and Donard, O. F. X. (2009). Odd Isotope Deficits in Atmospheric Hg Measured in Lichens. Environ. Sci. Technol. 43 (15), 5660–5664. doi:10.1021/es900578v
Chen, Z., Cui, J., Ren, Z., Jiang, S., Liang, X., Wang, G., et al. (2019). Geochemistry, Paleoenvironment and Mechanism of Organic‐Matter Enrichment in the Lower Silurian Longmaxi Formation Shale in the Sichuan Basin, China. Acta Geol. Sin-engl. 93 (3), 505–519. doi:10.1111/1755-6724.13868
Curtis, J. B. (2002). Fractured Shale-Gas Systems. AAPG Bull. 86 (11), 1921–1938. doi:10.1306/61EEDDBE-173E-11D7-8645000102C1865D
Dai, J. X., Zou, C. N., Liao, S. M., Dong, D. Z., Ni, Y. Y., Huang, J. L., et al. (2014). Geochemistry of the Extremely High thermal Maturity Longmaxi Shale Gas, Southern Sichuan Basin. Org. Geochem. 74, 3–12. doi:10.1016/j.orggeochem.2014.01.018
Demers, J. D., Blum, J. D., and Zak, D. R. (2013). Mercury Isotopes in a Forested Ecosystem: Implications for Air-Surface Exchange Dynamics and the Global Mercury Cycle. Glob. Biogeochem. Cy. 27 (1), 222–238. doi:10.1002/gbc.20021
Deng, Y., and Sun, T. (2019). Discussion on the Symbiotic Relationship between High Quality Source Rocks and Large Oilfields. China Offshore Oil Gas 31 (5), 1–8. doi:10.11935/j.issn.1673-1506.2019.05.001
Donovan, P. M., Blum, J. D., Yee, D., Gehrke, G. E., and Singer, M. B. (2013). An Isotopic Record of Mercury in San Francisco Bay Sediment. Chem. Geol. 349-350, 87–98. doi:10.1016/j.chemgeo.2013.04.017
Enrico, M., Le Roux, G., Heimbürger, L., Van Beek, P., Souhaut, M., Chmeleff, J., et al. (2017). Holocene Atmospheric Mercury Levels Reconstructed from Peat Bog Mercury Stable Isotopes. Environ. Sci. Technol. 51 (11), 5899–5906. doi:10.1021/acs.est.6b05804
Enrico, M., Roux, G. L., Marusczak, N., Heimbürger, L., Claustres, A., Fu, X., et al. (2016). Atmospheric Mercury Transfer to Peat Bogs Dominated by Gaseous Elemental Mercury Dry Deposition. Environ. Sci. Technol. 50 (5), 2405–2412. doi:10.1021/acs.est.5b06058
Estrade, N., Carignan, J., Sonke, J. E., and Donard, O. F. X. (2009). Mercury Isotope Fractionation during Liquid–Vapor Evaporation Experiments. Geochim. Cosmochim. Acta 73 (10), 2693–2711. doi:10.1016/j.gca.2009.01.024
Feng, X. B., Foucher, D., Hintelmann, H., Yan, H. Y., He, T. R., and Qiu, G. L. (2010). Tracing Mercury Contamination Sources in Sediments Using Mercury Isotope Compositions. Environ. Sci. Technol. 44 (9), 3363–3368. doi:10.1021/es9039488
Fitzgerald, W. F., and Lamborg, C. H. (2014). “11.4 - Geochemistry of Mercury in the Environment,” in Treatise on Geochemistry. Editors H. D. Holland, and K. K. Turekian. Second Edition (Oxford: Elsevier), 91–129. doi:10.1016/b978-0-08-095975-7.00904-9
Foucher, D., Ogrinc, , and Hintelmann, H. (2009). Tracing Mercury Contamination from the Idrija Mining Region (Slovenia) to the Gulf of Trieste Using Hg Isotope Ratio Measurements. Environ. Sci. Technol. 43 (1), 33–39. doi:10.1021/es801772b
Gantner, N., Hintelmann, H., Zheng, W., and Muir, D. C. (2009). Variations in Stable Isotope Fractionation of Hg in Food Webs of Arctic Lakes. Environ. Sci. Technol. 43 (24), 9148–9154. doi:10.1021/es901771r
Gehrke, G. E., Blum, J. D., and Meyers, P. A. (2009). The Geochemical Behavior and Isotopic Composition of Hg in a Mid-pleistocene Western Mediterranean Sapropel. Geochim. Cosmochim. Acta 73 (6), 1651–1665. doi:10.1016/j.gca.2008.12.012
Gong, Q., Wang, X. D., Zhao, L. S., Grasby, S. E., Chen, Z. Q., Zhang, L., et al. (2017). Mercury Spikes Suggest Volcanic Driver of the Ordovician-Silurian Mass Extinction. Sci. Rep. 7 (1). doi:10.1038/s41598-017-05524-5
Gong, X. F., He, J. X., Wu, C. K., Yang, J., Li, K., Li, D., et al. (2014). Basic Geological and Geochemical Characteristics of Unconventional Natural Gas Resources in China. Mar. Geol. Quat. Geol. 34 (05), 95–105. doi:10.3724/SP.J.1140.2014.05095
Han, Z. X., Gou, Y. X., Li, J., Gou, S. G., Tian, W. N., and Huang, H. (2021). Content Distribution Characteristics and Genetic Analysis of Mercury in the Natural Gas from Sichuan Basin. Nat. Gas Geosci. 32 (03), 356–362. doi:10.11764/j.issn.1672-1926.2020.11.014
Hirner, A. V., Kritsotakis, K., and Tobschall, H. J. (1990). Metal-organic Associations in Sediments—I. Comparison of Unpolluted Recent and Ancient Sediments and Sediments Affected by Anthropogenic Pollution. Appl. Geochem. 5 (4), 491–505. doi:10.1016/0883-2927(90)90023-X
Huang, X. Q., Wang, J. J., Du, Y., Li, L., and Zhang, Z. (2019). Discussion on Development Mode of Smaller Well Spacing and Tridimensional Development in the YS108 Block, Zhaotong National Shale Gas Demonstration Area. Nat. Gas Geosci. 30 (04), 557–565. doi:10.11764/ji.ssn1672-1926.2018.12.018
Jiskra, M., Wiederhold, J. G., Bourdon, B., and Kretzschmar, R. (2012). Solution Speciation Controls Mercury Isotope Fractionation of Hg(II) Sorption to Goethite. Environ. Sci. Technol. 46 (12), 6654–6662. doi:10.1021/es3008112
Kritee, K., Motta, L. C., Blum, J. D., Tsui, M. T., and Reinfelder, J. R. (2018). Photomicrobial Visible Light-Induced Magnetic Mass Independent Fractionation of Mercury in a Marine Microalga. ACS Earth Space Chem. 2 (5), 432–440. doi:10.1021/acsearthspacechem.7b00056
Kwon, S. Y., Blum, J. D., Yin, R. S., Tsui, M. T., Yang, Y. H., and Choi, J. W. (2020). Mercury Stable Isotopes for Monitoring the Effectiveness of the Minamata Convention on Mercury. Earth-sci. Rev. 203, 103111. doi:10.1016/j.earscirev.2020.103111
Le Faucheur, S., Campbell, P. G. C., Fortin, C., and Slaveykova, V. I. (2014). Interactions between Mercury and Phytoplankton: Speciation, Bioavailability, and Internal Handling. Environ. Toxicol. Chem. 33 (6), 1211–1224. doi:10.1002/etc.2424
Lefticariu, L., Blum, J. D., and Gleason, J. D. (2011). Mercury Isotopic Evidence for Multiple Mercury Sources in Coal from the Illinois Basin. Environ. Sci. Technol. 45 (4), 1724–1729. doi:10.1021/es102875n
Li, J., Han, Z. X., Yan, Q. T., Wang, S. Y., Ge, S. G., and Wang, C. Y. (2012a). Genesis of Mercury in Natural Gas of Chinese Gas Fields. Nat. Gas Geosci. 23 (3), 413–419. doi:10.11764/j.issn.1672-1926.2012.03.413
Li, J., Xie, S. L., Feng, J., Li, Y. H., and Chen, L. (2012b). Heavy Metal Uptake Capacities by the Common Freshwater green Alga Cladophora Fracta. J. Appl. Phycol. 24 (4), 979–983. doi:10.1007/s10811-011-9721-0
Li, Q. W., Pang, X. Q., Tang, L., Chen, G., Shao, X. H., and Jia, N. (2018). Occurrence Features and Gas Content Analysis of marine and continental Shales: A Comparative Study of Longmaxi Formation and Yanchang Formation. J. Nat. Gas Sci. Eng. 56, 504–522. doi:10.1016/j.jngse.2018.06.019
Li, Z. P. (2020).Preliminary Study on Mercury Content and Mercury Isotopes in Coal-Derived Gas and Terrestrial Oil-type Gas in Xinjiang. [dissertation/master's thesis]. jiaozuo: Henan Polytechnic University.
Liu, Q. Y. (2013). Mercury Concentration in Natural Gas and its Distribution in the Tarim Basin. Sci. China Earth Sci. 56 (8), 1371–1379. doi:10.1007/s11430-013-4609-2
Liu, Q. Y., Peng, W. L., Li, J., and Wu, X. Q. (2020). Source and Distribution of Mercury in Natural Gas of Major Petroliferous Basins in China. Sci. China Earth Sci. 63 (5), 643–648. doi:10.1007/s11430-019-9596-6
Ma, J., Hintelmann, H., Kirk, J. L., and Muir, D. C. G. (2013). Mercury Concentrations and Mercury Isotope Composition in lake Sediment Cores from the Vicinity of a Metal Smelting Facility in Flin Flon, Manitoba. Chem. Geol. 336, 96–102. doi:10.1016/j.chemgeo.2012.10.037
Ma, Z. L., Shen, B. J., Pan, A. Y., TenggerNing, C. X., and Zheng, L. J. (2020). Shale Gas Genesis and Carbon Isotope Inversion Mechanism of Wufeng Longmaxi Formation in Sichuan Basin -- Understanding from thermal Simulation experiment. Pet. Geol. Exp. 42 (03), 428–433. doi:10.11781/sysydz202003428
Mason, R. P., Choi, A. L., Fitzgerald, W. F., Hammerschmidt, C. R., Lamborg, C. H., Soerensen, A. L., et al. (2012). Mercury Biogeochemical Cycling in the Ocean and Policy Implications. Environ. Res. 119, 101–117. doi:10.1016/j.envres.2012.03.013
Montgomery, S. L., Jarvie, D. M., Bowker, K. A., and Pollastro, R. M. (2005). Mississippian Barnett Shale, Fort Worth basin, north-central Texas: Gas-Shale Play with Multi–Trillion Cubic Foot Potential. AAPG Bull 89 (02), 155–175. doi:10.1306/09170404042
Ni, Y. Y., Liao, F. R., Gong, D. Y., Jiao, L. X., Gao, J. L., and Yao, L. M. (2019). Stable Carbon and Hydrogen Isotopic Characteristics of Natural Gas from Taibei Sag, Turpan-Hami Basin, NW China. Pet. Explor. Dev. 46 (3), 531–542. doi:10.1016/S1876-3804(19)60033-9
Nie, H. K., Jin, Z. J., Sun, C. X., He, Z. L., Liu, G. X., and Liu, Q. Y. (2019). Organic Matter Types of the Wufeng and Longmaxi Formations in the Sichuan Basin, South China: Implications for the Formation of Organic Matter Pores. Energy Fuels 33 (9), 8076–8100. doi:10.1021/acs.energyfuels.9b01453
Ogrinc, N., Hintelmann, H., Kotnik, J., Horvat, M., and Pirrone, N. (2019). Sources of Mercury in Deep-Sea Sediments of the 481 Mediterranean Sea as Revealed by Mercury Stable Isotopes. Sci. Rep. 9 (1), 11626. doi:10.1038/s41598-019-48061-z
Outridge, P. M., Sanei, H., Stern, G. A., Hamilton, P. B., and Goodarzi, F. (2007). Evidence for Control of Mercury Accumulation Rates in Canadian High Arctic Lake Sediments by Variations of Aquatic Primary Productivity. Environ. Sci. Technol. 41 (15), 5259–5265. doi:10.1021/es070408x
Outridge, P. M., Stern, G. A., Hamilton, P. B., and Sanei, H. (2019). Algal Scavenging of Mercury in Preindustrial Arctic Lakes. Limnol. Oceanogr. 64 (4), 1558–1571. doi:10.1002/lno.11135
Ozerova, N. (1983). New Mercury Ore belt in Western Europe. Int. Geol. Rev. 25 (9), 1095–1100. doi:10.1080/00206818309466806
Peng, W. L., Liu, Q. Y., Feng, Z. Q., Fang, C. C., Gong, D. Y., Li, P., et al. (2019). First Discovery and Significance of Liquid Mercury in a Thermal Simulation Experiment on Humic Kerogen. Energy Fuels 33 (3), 1817–1824. doi:10.1021/acs.energyfuels.8b03294
Perrot, V., Pastukhov, M. V., Epov, V. N., Husted, S., Donard, O. F., and Amouroux, D. (2012). Higher Mass-independent Isotope Fractionation of Methylmercury in the Pelagic Food Web of Lake Baikal (Russia). Environ. Sci. Technol. 46 (11), 5902–5911. doi:10.1021/es204572g
Schauble, E. A. (2007). Role of Nuclear Volume in Driving Equilibrium Stable Isotope Fractionation of Mercury, Thallium, and Other Very Heavy Elements. Geochim. Cosmochim. Acta 71 (9), 2170–2189. doi:10.1016/j.gca.2007.02.004
Shen, J., Algeo, T. J., Chen, J. B., Planavsky, N. J., Feng, Q. L., Yu, J. X., et al. (2019). Mercury in marine Ordovician/Silurian Boundary Sections of South China Is Sulfide-Hosted and Non-volcanic in Origin. Earth Planet. Sci. Lett. 511, 130–140. doi:10.1016/j.epsl.2019.01.028
Sherman, L. S., and Blum, J. D. (2013). Mercury Stable Isotopes in Sediments and Largemouth Bass from Florida Lakes, USA. Sci. Total Environ. 448, 163–175. doi:10.1016/j.scitotenv.2012.09.038
Shi, W. F., Feng, X. B., Zhang, G., Ming, L. L., Yin, R. S., Zhao, Z. Q., et al. (2011). High-precision Measurement of Mercury Isotope Ratios of Atmospheric Deposition over the Past 150 Years Recorded in a Peat Core Taken from Hongyuan, Sichuan Province, China. Sci. Bull. 56 (9), 877–882. doi:10.1007/s11434-011-4396-0
Smith, C. N., Kesler, S. E., Blum, J. D., and Rytuba, J. J. (2008). Isotope Geochemistry of Mercury in Source Rocks, mineral Deposits and spring Deposits of the California Coast Ranges, USA, Earth Planet. Sci. Lett. 269 (3), 399–407. doi:10.1016/j.epsl.2008.02.029
Stetson, S. J., Gray, J. E., Wanty, R. B., and Macalady, D. L. (2009). Isotopic Variability of Mercury in Ore, Mine-Waste Calcine, and Leachates of Mine-Waste Calcine from Areas Mined for Mercury. Environ. Sci. Technol. 43 (19), 7331–7336. doi:10.1021/es9006993
Štrok, M., Baya, P. A., and Hintelmann, H. (2015). The Mercury Isotope Composition of Arctic Coastal Seawater. C. R. Geosci. 347 (7-8), 368–376. doi:10.1016/j.crte.2015.04.001
Sun, L., Lu, B., Yuan, D., Hao, W., and Zheng, Y. (2017). Variations in the Isotopic Composition of Stable Mercury Isotopes in Typical Mangrove Plants of the Jiulong Estuary, SE China. Environ. Sci. Pollut. Re. 24 (2), 1459–1468. doi:10.1007/s11356-016-7933-1
Sun, R. Y., Sonke, J. E., and Liu, G. L. (2016). Biogeochemical Controls on Mercury Stable Isotope Compositions of World Coal Deposits: A Review. Earth-sci. Rev. 152, 1–13. doi:10.1016/j.earscirev.2015.11.005
Tang, S. L., Feng, C. H., Feng, X. B., Zhu, J. M., Sun, R. Y., Fan, H. P., et al. (2017). Stable Isotope Composition of Mercury Forms in Flue Gases from a Typical Coal-Fired Power Plant, Inner Mongolia, Northern China. J. Hazard. Mater. 328, 90–97. doi:10.1016/j.jhazmat.2017.01.014
Tang, S. L., Zhou, Y. P., Yao, X. J., Feng, X. B., Li, Z. P., and Wu, G. N. (2019). The Mercury Isotope Signatures of Coalbed Gas and Oil-type Gas: Implications for the Origins of the Gases. Appl. Geochem. 109, 104415. doi:10.1016/j.apgeochem.2019.104415
Tengger, B., Shen, B. J., Yu, L. J., Yang, Y. F., Zhang, W. T., Tao, C., et al. (2017). Mechanisms of Shale Gas Generation and Accumulation in the Ordovician Mechanisms of Shale Gas Generation and Accumulation in the Ordovician Wufeng-Longmaxi Formation, Sichuan Basin, SW China. Petrol. Explor. Dev. 44 (01), 69–78. doi:10.1016/S1876-3804(17)30009-5
Tian, J. C., Liu, W. W., Wang, F., Chen, R., and Lin, X. B. (2014). Heterogeneity of the Paleozoic Tight sandstone Reservoirs in Gaoqiao Area of Ordos Basin. Oil Gas Geol. 35 (02), 183–189. doi:10.11743/ogg20140202
Tsui, M. T., Blum, J. D., Kwon, S. Y., Finlay, J. C., Balogh, S. J., and Nollet, Y. H. (2012). Sources and Transfers of Methylmercury in Adjacent River and forest Food Webs. Environ. Sci. Technol. 46 (20), 10957–10964. doi:10.1021/es3019836
Verburg, P., Hickey, C. W., and Phillips, N. (2014). Mercury Biomagnification in Three Geothermally-Influenced Lakes Differing in Chemistry and Algal Biomass. Sci. Total Environ. 493 (sep.15), 342–354. doi:10.1016/j.scitotenv.2014.05.097
Wang, X., Luo, J., Yin, R. S., Yuan, W., Lin, C. J., Sommar, J., et al. (2017). Using Mercury Isotopes to Understand Mercury Accumulation in the Montane Forest Floor of the Eastern Tibetan Plateau. Environ. Sci. Technol. 51 (2), 801–809. doi:10.1021/acs.est.6b03806
Wang, Z., Li, X. Q., Zhang, J. Z., Xu, H. W., Wang, G., Zhou, B., et al. (2016). Longmaxi Formation Shale Gas Geochemical Features Comparison between Different Blocks in Sichuan Basin. Coal Geol. China. 28 (02), 22–27. doi:10.1016/j.jngse.2015.11.045
Washburn, S. J., Blum, J. D., Johnson, M. W., Tomes, J. M., and Carnell, P. J. (2018). Isotopic Characterization of Mercury in Natural Gas via Analysis of Mercury Removal Unit Catalysts. ACS Earth Space Chem. 2 (5), 462–470. doi:10.1021/acsearthspacechem.7b00118
Wiederhold, J. G., Cramer, C. J., Daniel, K., Infante, I., Bourdon, B., and Kretzschmar, R. (2010). Equilibrium Mercury Isotope Fractionation between Dissolved Hg(II) Species and Thiol-Bound Hg. Environ. Sci. Technol. 44 (11), 4191–4197. doi:10.1021/es100205t
Wu, K. Y., Zhang, T. S., Yang, Y., Liang, X., Zhou, S. Y., and Zhang, C. (2016). Geological Characteristics of Wufeng-Longmaxi Shale-Gas Reservoir in the Huangjinba Gas Field, Zhaotong National Shale Gas Demonstration Area. Geol. China. 43 (01), 275–287. doi:10.12029/gc20160120
Xu, Z. Y., Liang, X., Lu, H. L., Zhang, J. H., Shu, H. L., Xu, Y. J., et al. (2019). Structural Deformation Characteristics and Shale Gas Preservation Conditions in the Zhaotong National Shale Gas Demonstration Area along the Southern Margin of the Sichuan Basin.china. Nat. Gas Ind. 39 (10), 22–31. doi:10.1016/j.ngib.2019.10.004
Yin, R. S., Feng, X. B., Wang, J, X., Bao, Z. D., Yu, B., and Chen, J. B. (2013). Mercury Isotope Variations between Bioavailable Mercury Fractions and Total Mercury in Mercury Contaminated Soil in Wanshan Mercury Mine, SW China. Chem. Geol. 336, 80–86. doi:10.1016/j.chemgeo.2012.04.017
Zheng, W., Demers, J. D., Lu, X., Bergquist, B. A., Anbar, A. D., Blum, J. D., et al. (2019). Mercury Stable Isotope Fractionation during Abiotic Dark Oxidation in the Presence of Thiols and Natural Organic Matter. Environ. Sci. Technol. 53 (4), 1853–1862. doi:10.1021/acs.est.8b05047
Zheng, W., and Hintelmann, H. (2009). Mercury Isotope Fractionation during Photoreduction in Natural Water Is Controlled by its Hg/DOC Ratio. Geochim. Cosmochim. Acta 73 (22), 6704–6715. doi:10.1016/j.gca.2009.08.016
Zheng, W., and Hintelmann, H. (2010). Nuclear Field Shift Effect in Isotope Fractionation of Mercury during Abiotic Reduction in the Absence of Light. J. Phys. Chem. A. 114 (12), 4238–4245. doi:10.1021/jp910353y
Keywords: mercury stable isotope, mass-independent fractionation, mercury content, shale gas, exploration target layer
Citation: Tang S, Ding Y, Zhu G, Feng X, Zhang H and Li P (2022) Mercury Isotopes in Shale Gas From Wufeng-Longmaxi Shale Formation of Sichuan Basin, Southern China: A Preliminary Investigation. Front. Earth Sci. 10:809418. doi: 10.3389/feart.2022.809418
Received: 05 November 2021; Accepted: 17 January 2022;
Published: 21 February 2022.
Edited by:
Qinhong Hu, University of Texas at Arlington, United StatesReviewed by:
Xiao Xianming, China University of Geosciences, ChinaCopyright © 2022 Tang, Ding, Zhu, Feng, Zhang and Li. This is an open-access article distributed under the terms of the Creative Commons Attribution License (CC BY). The use, distribution or reproduction in other forums is permitted, provided the original author(s) and the copyright owner(s) are credited and that the original publication in this journal is cited, in accordance with accepted academic practice. No use, distribution or reproduction is permitted which does not comply with these terms.
*Correspondence: Shunlin Tang, dGFuZ3NodW5saW5AaHB1LmVkdS5jbg==; Guangyou Zhu, emh1Z3Vhbmd5b3VAcGV0cm9jaGluYS5jb20uY24=
Disclaimer: All claims expressed in this article are solely those of the authors and do not necessarily represent those of their affiliated organizations, or those of the publisher, the editors and the reviewers. Any product that may be evaluated in this article or claim that may be made by its manufacturer is not guaranteed or endorsed by the publisher.
Research integrity at Frontiers
Learn more about the work of our research integrity team to safeguard the quality of each article we publish.