- School of Water Conservancy Engineering, Zhengzhou University, Zhengzhou, China
The adsorption state is one of the main states for shale gas occurrence, and the gas adsorption behavior in shale directly affects shale gas content under reservoir conditions. This paper provides a comprehensive literature review on shale gas adsorption behavior and its affecting factors that have been developed in recent years. Influence factors of gas adsorption behavior are examined, including total organic carbon content (TOC), organic matter type, organic matter maturity, minerals and clay minerals, moisture content, pore characteristics and other characteristics of the shale itself. The characteristics of gas adsorption behavior under high temperature and pressure conditions showed that adsorption behaviors were difficult to describe by the Langmuir equation. This review indicates that shale contains higher organic matter content and organic matter maturity and has a higher adsorption capacity. The adsorption capacity with type III kerogen is higher than that for type II or type I. Clay minerals can provide free space for gas adsorption and promote adsorption. Normally, as the moisture content increased, adsorption capacity decreased. Micro pores provided a larger specific surface area for gas adsorption. As the temperature increased, the adsorption capacity decreased. As the pressure increased, shale adsorption characteristics showed two different behaviors as follows: one obeyed the Langmuir equation, and the other presented an inverted, U-shaped, single-peak distribution. However, there are some controversies surrounding adsorption, especially regarding the aspects of clay minerals, water content, pore characteristics, etc. The key is that the mechanism of adsorption in shale is unclear. There will be many new challenges in the field of shale gas adsorption research. Such challenges include studying the organic matter chemical structure, understanding the interaction between organic matter and clay minerals and how they affect adsorption, clarifying gas adsorption behavior changes, predicting favorable areas of adsorbed gas with the coupling of reservoir temperature and pressure, and building a better theory and model of shale gas adsorption.
1 Introduction
With the increasing worldwide demand of natural gas, shale gas, as a new and unconventional natural gas resource, has attracted more and more attention. In the United States. Energy Information Administration’s International Energy Outlook 2016 and Annual Energy Outlook 2016, global shale gas is expected to account for 30% of the world’s total natural gas output by the end of 2040. At present, commercial development of shale gas has been achieved in the United States, Canada, Argentina and China. The United States successfully drilled the first shale gas well in the Appalachian Basin in 1821 and shale gas production has continued to develop for the last 200 ears. In the 21st century, shale gas production has increased rapidly in the United States. That production reached 3,800×108 m3 in 2015, accounting for more than half of total natural gas production. The rapid development of shale gas production has changed the US natural gas supply pattern, leading to significant drops in natural gas and liquefied natural gas imports (Zhang, 2012). Since 2005, China has evaluated geological conditions and conducted development tests for shale gas. Now, China has made significant breakthroughs in geological theory, exploration and development techniques (Dong et al., 2012), especially in the study of marine and continental organic-rich shales in the southern Paleozoic/northern Mesozoic areas of the Sichuan Basin, the Triassic area in the Ordos Basin, as well as several other regions and ages (Zou et al., 2010; Lin et al., 2012; Ren et al., 2014; Yu et al., 2014), and has made great progress in geological characteristics with rich organic, formation, occurrence and reservoir conditions, prospective zone optimization for shale gas, etc. In the past 5 years, more than 700 shale gas wells have been drilled in China. Shale gas production surpassed 50×108 m3 in 2016. Shale gas is primarily a natural gas preserved in dark mud and high carbonaceous shale (Yang et al., 2014a). Zhang et al. (2008) showed that adsorbed gas and free gas are mainly states. The adsorption effect is one important mechanism of shale gas accumulation. Mavor, 2003 showed that adsorbed gas accounts for 61% of original geological reserves of shale gas in the Barnett Formation. Li et al. (2007) showed that adsorbed shale gas occupies at least 40% of the total gas content. Nie and Zhang, 2010 and Rani et al. (2015) reported that 40%–60% of gas is adsorbed. According to Barnett shale gas characteristics, shale gas is stored in matrix pores and accounts for more than 50% of the total gas content (Montgomery et al., 2005; Bowker, 2007; Kinley et al., 2008). It has been shown that shale gas occurs primarily in the adsorption state, free state or dissolved state; of these, adsorption state is the most important. Adsorbed gas accounts for 20%–85% of the total shale gas content, which mainly accounts for 40%–60% (Mavor, 2003; Li et al., 2007; Zou et al., 2011; Chareonsuppanimit et al., 2012). Therefore, adsorption capacity of shale is one of the key factors that affect shale gas content. In recent years, with the large-scale development of shale gas, shale gas exploration depths are increasing. Exploration depths have increased from 180–2000 m to 2,300–4,500 m, and have reached up to 6,000 m in individual basins in America (Nie and Zhang, 2010). In China, the Weiyuan-Changning gas field was the first to realize commercialized production, Cambrian-Ordovician shale has a depth of 1,500–4,500 m (Zou et al., 2016). The adsorption behavior in shale directly affects adsorption gas content with increasing burial depth, which becomes the key to obtaining more accurate information on the amount of shale gas resources, and has an important significance for shale gas reserve evaluation, reserve prediction and productivity predictions.
Therefore, the estimation of initial adsorbed gas in place is one of the primary concerns in shale gas reservoir studies, and it is also important for reservoir-engineering analysis, such as gas production forecasting (Wu et al., 2012; Li et al., 2016).
In this review, based on previous research results, influencing factors of adsorption capacity in shale are summarized, effects of different factors on adsorption capacity are analyzed in detail, a main adsorption theory and adsorption model is noted, and challenges and development prospects associated with the field of shale gas adsorption are put forth.
2 Change of gas adsorption behaviors on shale
In the current environment of rapid changing climate conditions and increasing human activities, meteorological and hydrological conditions may change. Bernaola Galvan proposed a heuristic segmentation algorithm (BG algorithm) in 2001 (Bernaola-Galvan et al., 2001), which can test the stationary status of the sequence, detect the mutation point, and divide the non-stationary sequence into several stationary sequences. The main ideas are as follows:
Adsorption is a phenomenon by which gas molecules remain attached or retained on the surface of a solid substance when a gas interacts with a solid. The gas molecules remain attached on the surface of a solid by molecular attraction. The attraction of gas adsorption in shale reserves is mainly Van der Waals force. Therefore, the adsorption process is reversible and is called physisorption. Adsorption depends on temperature, pressure, specific surface area and activation energy of solid. In a given system of gas-solid, the activation energy of a solid is certain, and the adsorption volume is a function related to pressure when temperature is fixed. The relationship is called adsorption isothermal, which is often used to describe a change in adsorption behaviors. The isothermal of gas adsorption in shale can be divided into two types. Type I is Langmuir pattern. With the increase of pressure, the adsorption volume first increases, eventually reaches a maximum value, which is represented by the Langmuir volume constant, and then stabilizes. The relationship between adsorption volume and pressure conforms to the Langmuir equation (Figure 1) (Lu et al., 1995; Ross and Bustin, 2007; Chalmers and Bustin, 2008; Liu and Wang, 2012). Gas adsorption needs more binding energies in low pressure. With increases of pressure, necessary binding energy decreases, and the amount of adsorption increases (Raut et al., 2007). This is the most common type of adsorption isothermal that can be explained by monolayer adsorption theory.
Type II is an inverted “U” pattern. As the pressure increases, the adsorption volume first increases but then decreases. The relationship between adsorption volume and pressure does not completely follow the Langmuir equation. This adsorption phenomenon has been found by many scholars (Chalmers and Bustin, 2008; Ross and Bustin, 2008; Chareonsuppanimit et al., 2012). We studied adsorption capacity of Permian, Triassic and Jurassic shale in the Jiyuan Depression and the Carboniferous shale in Ordos Basin and showed that with a pressure increase, the adsorption isothermal showed two kinds of changes. One change is that the relationship between the adsorption volume and pressure obeys the Langmuir equation (type I); the other is that a “single peak” adsorption phenomenon is observed (type II), and the maximum adsorption value occurs between 5 MPa and 12 MPa (Figure 2). The same conclusion has been found in the study of CH4 and CO2 adsorption on coal, and the maximum adsorption value appears with an increase in pressure (Type II) (Nandi and Walker, 1975; Marecka and Mianowski, 1998; Tang et al., 2003; Yu et al., 2004; Day et al., 2008; Zhang et al., 2009; Gensterblum et al., 2013). Chilev et al. (2012) indicated that the maximum gas adsorption in porous media might appear at the maximum adsorption value in the range of 10 MPa and 30 MPa above the critical temperature. Many other researchers also found a similar phenomenon (Menon, 1965; Malbrunot et al., 1992; Zhou et al., 1994; Aranovich et al., 2000; Dreisbach et al., 2002; Do and Do, 2005; Siemons and Busch, 2007). Malbrunot et al. (Malbrunot et al., 1992) and Menon, 1965 noted that the adsorption capacity reached a maximum value at 10 MPa–30 MPa with a minimum value between 550 MPa and 650 MPa as the pressure increased.
In view of this phenomenon, it is generally believed that the adsorption amount follows the Gibbs definition of adsorption.
Gibbs’ definition states that the adsorbate molecules in the adsorbed phase on the surface of the adsorbent cannot all be classified as “adsorption,” in which the gas molecule distributed in adsorbed space by way of the bulk gas density is independent of the force between the gas and solid. The adsorbed gas amount is the excess amount at the interface between the two bulk phases. The absolute adsorption is a function of the bulk gas phase density and adsorbed phase density. The Gibbs adsorption is expressed as:
where
If the density function of the adsorbed phase
where
The critical temperature of CH4 is 190.6 K, the critical pressure is 4.54 MPa CH4 is supercritical at room temperature and cannot be liquefied at high pressure. Therefore, the gas molecules cannot condense on the surface of solid. The force between the gas-solid is greater than the force between gas-gas. The gas adsorption is monolayer. When the gas coverage on the solid surface is low, the adsorbed gas volume increases with an increase of pressure, the gas distribution is monolayer, and the adsorption isotherm shows Type I. The distribution and amount of adsorption space determine the amount and density of the adsorbed phase, both of which are capped. The gas cannot condense, so
However, this view could not reasonably explain why a difference exists in the pressure at maximum adsorption values of different adsorbents. Some researchers explained this phenomenon based on other influencing factors. Gensterblum et al. (2013) considered that differences in the maximum values of different adsorption capacities in coal are related to coal characteristics such as temperature, moisture and adsorbed gas properties. Day et al. (2008) and Siemons and Busch, 2007 (Day et al., 2008) concluded that supercritical absorption capacities in coal are related to coal rank, porosity, moisture content and other factors; with the effect of porosity on adsorption capacity being significant. Chilev et al. (2012) believed that the appearance of an adsorption maximum value is related to the following: solid-phase deformation under high pressure, change of direction of molecular motion, filling of pores and others, but it is unknownhow these factors influence absoption maximum. Fang et al. (2010) considered that the phenomenon of an adsorption anomaly in shale is related to clay mineral content, moisture content, organic matter and supercritical adsorption characteristics. Nie and Zhang, 2010 analyzed the cause of the inverted U phenomenon for adsorption curves from the standpoint of the adsorption experiment principle, microscopic pore structures, adsorbent, etc. And concluded that the phenomenon is attributed to the difference between the experimental adsorption amount and the actual adsorption quantity. Zhang and Cao, 2003 also noted that the gas compression rate increases with increasing pressure, thereby increasing the storage capacity of free gas and resulting in a decrease in the amount of adsorption gas content.
3 Influencing factors of gas adsorption behaviors
3.1 Organic matter in shale gas reservoirs
Organic matter is one of the factors that affect shale adsorption capacity. Ross and Bustin, 2009 noted that approximately 90% of methane adsorption content is related to organic matter, and approximately 10% is related to the minerals in shale. Organic matter mainly affects the adsorption capacity through organic carbon content, organic matter maturity and organic matter type.
(1) Total organic carbon content (TOC)
Current research has shown that as the TOC increases, so does the adsorption capacity of shale (Figure 3). Lu et al. (1995) demonstrated that organic matter was the primary storage media for adsorbed gas. Hill et al. (2007) showed that there is a positive linear correlation between TOC and methane adsorption capacity. Chalmers and Bustin, 2008 studied the adsorption capacity of the Lower Cretaceous shale in northeastern British Columbia and the Barnett and Barnett shale in Mitchell (T.P.Sims well); they all reached the same conclusion, that is, TOC is higher, and shale gas adsorption capacity is stronger. There are three main reasons to explain those observations. First, the TOC is higher, gas generation potential is greater, and shale gas content is higher; secondly, kerogen, with a large number of micro porosity channels and higher specific surface areas, provides more space for gas adsorption (Chalmers and Bustin, 2007; Ross and Bustin, 2009); finally, the kerogen surface is lipophilic, which favors gas adsorption. In addition, dissolution of gaseous hydrocarbons in amorphous and non-structured matrix asphalt bodies also has a significant contribution to the adsorption capacity (Wu et al., 2012; Lin et al., 2019).
(2) Organic matter maturity
In the field of shale gas adsorption, the current basic consensus is that increasing the thermal evolution degree of organic matter increases the gas adsorption capacity (Figure 4). Jiang et al. (2018) conducted isothermal adsorption tests on Barnett shale with different amounts of TOC ranging from 6.6% to 7.9% and found that adsorption capacity increased with an increase in organic maturity. This conclusion can be explained based on three properties of the TOC. The first is that thermally mature shale tends to exhibit larger micro pore volumes and larger specific surface areas than immature shale, which results in higher adsorption capacities (Chalmers and Bustin, 2007; Ross and Bustin, 2007; Chalmers and Bustin, 2008; Zhang, 2012). Second, during the stage of low-temperature evolution, a large amount of asphaltenes and colloids are generated and fill the pore space; the connectivity and porosity of pores decreases and leads to a decrease in specific surface area for gas adsorption (Franco et al., 2013). Lastly, with an increase in organic maturity, the amount of hydrogen in the organic matter decreases and enhances the aromatization of organic matter. Kerogen rich in aromatic structures has a stronger affinity for methane than kerogen with high aliphatic hydrocarbon content (Zhang, 2012). The conclusion is consistent with the gas adsorption capacity in coalbeds (Wang et al., 2018); namely, as the degree of coalification increases, the adsorption capacity of coalbed methane also increases.
(3) Organic matter type
Gas adsorption capacity in shale is complex, as shale contains a high degree of heterogeneity in its organic matter maceral composition. Maceral composition controls the organic matter type, influences the surface characteristics and the pore size distribution of shale, all of which affect the sorption capacity. Type III kerogen is mainly derived from terrestrial higher plants, and its microcosmic components are dominated by vitrinite (Hou et al., 2022). Type I kerogen is mainly derived from planktonic organisms and is mainly composed of chitin. Type III has more micro pores than type I, resulting in larger specific surface areas for gas adsorption, so adsorption capacity is stronger. The components of type II kerogen are a mixture of both types I and III. Zhang , 2012 conducted isothermal adsorption experiments with different types of kerogen and indicated that type III kerogen has the highest adsorption capacity, followed by type II and type I. Chen et al. (Chen et al., 2015) showed that the adsorption capacity of different kerogen types followed the following order: type II2> type II1> type I. Generally, the adsorption capacity order of different kerogen types is as follows: type II/III or type III> type I or type II (Chalmers and Bustin, 2008; Zhang, 2012; Jiang et al., 2016) (Figure 5). Chalmers and Bustin, 2007 (Chalmers and Bustin, 2008) and Wang et al. (2004) studied the adsorption capacity of the Late Cretaceous Fort St. John shale in the northeastern British Columbia and indicated that the high adsorption capacity of shale is related to the amount of vitrinite and inertinite. Vitrinite contains more micropores, i.e., more surface area, and has a positive effect on gas adsorption capacity, while liptinite contains more macropores than micropores, which has a negative effect on gas absorption (Perera et al., 2012). This means that type III kerogen has higher gas adsorption than that of type I. The kerogen types have less of an effect on adsorption compared with TOC content (Figure 5). The type I with higher TOC possibly has a larger adsorption capacity than that of type III.
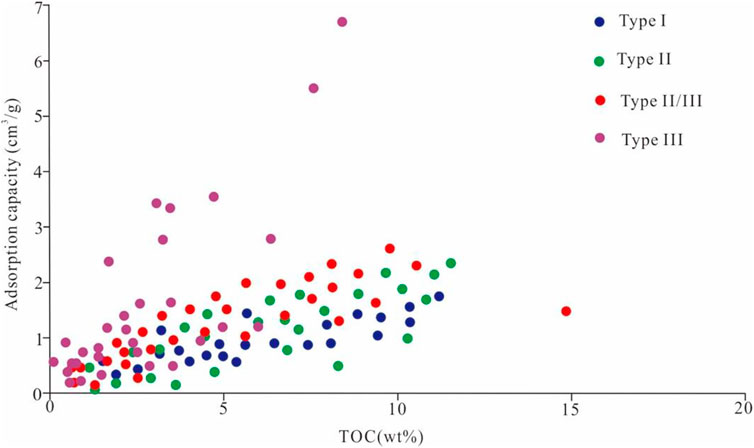
FIGURE 5. Relationship between kerogen types and methane adsorption capacity (Data from: Chalmers and Bustin, 2008; Zhang et al., 2012; Jiang et al., 2016).
3.2 Mineral and clay mineral
The mineral composition of shale is complex and includes the following: clay minerals such as illite, montmorillonite and kaolinite; brittle minerals such as quartz, calcite, feldspar, mica and other detrital minerals and authigenic minerals. These minerals can all affect the adsorption capacity. For instance, brittle minerals can reduce porosity, which leads to a decrease of adsorption capacity and storage space of the free gas (Figure 6A) (Li et al., 2007; Loucks and Ruppel, 2007; Chalmers and Bustin, 2008). However, the presence of clay minerals is favorable for gas adsorption (Figure 6B) (Lu et al., 1995; Cheng and Huang, 2004; Wang et al., 2004; Loucks and Ruppel, 2007; Ji et al., 2012a; Ji et al., 2012b). On the one hand, micro pores with apertures of 1 nm–2 nm often exist between the crystal layers of clay minerals, which have great micro-pore volumes, large specific surface areas and are the primary sites for gas adsorption (Cheng and Huang, 2004; Wang et al., 2004; Loucks and Ruppel, 2007; Ji et al., 2012a; Ji et al., 2012b; Niu et al., 2021). On the other hand, a layer of water film can form on clay mineral surfaces due to electrochemical characteristics of the clay mineral surface, which plays an important role in methane adsorption and might combine with methane to form gas hydrates (Park and Sposito, 2003). Of course, some scholars hold different views about the relationship between adsorption capacity and clay minerals; Liu and Wang, 2012 studied the adsorption characteristics in marine shale in southern China, and concluded that the maximum adsorption capacity is not related to clay mineral composition under the conditions of similar TOC contents.
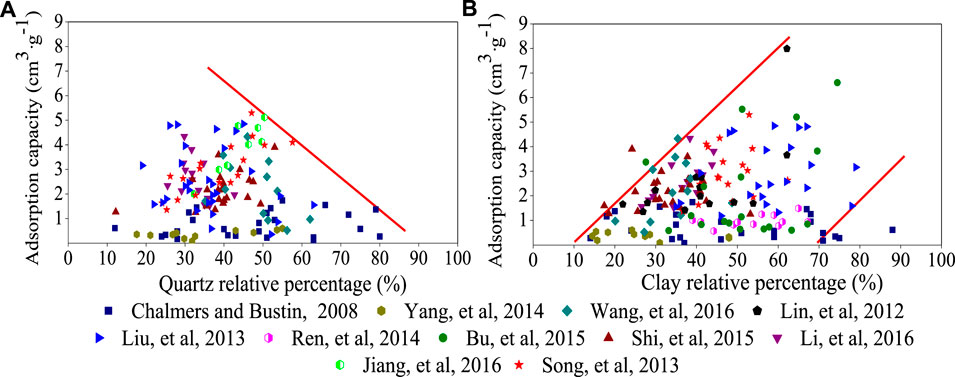
FIGURE 6. (A): Relationship between quartz and methane adsorption capacity and (B): Relationship between clay minerals and methane adsorption capacity.
Different clay minerals have different chemical compositions, crystal structures, particle sizes and petro physical properties, in which crystal spaces, inter-layer and gap sizes between particles, shapes and surface areas are different (Lu et al., 1995). Kennedy et al., 2002 found that the inner surface area of montmorillonite is much larger than that of kaolinite, approximately 90 times higher than kaolinite, which is favorable for gas adsorption. Ji et al., (2012b) showed that the methane adsorption capacity of different clay minerals differed, and the adsorption capacity order is as follows: montmorillonite > illite/montmorillonite mixed layer > kaolinite > chlorite > illite. The influence of clay minerals on methane adsorption capacity is also affected by their own digenetic evolution and rock formation.
3.3 Pore characterization
On the one hand, pore structure controls storage and output of shale gas. Heterogeneity of pore size distribution plays an important role in the adsorption process. Pore structure is more complex, porosity and surface area are larger, and the adsorption capacity increases (Heller and Zoback, 2014; Hinai et al., 2014; Chen et al., 2015; Li et al., 2016) (Figures 7A,B). Kim et al. (2017) studied the Horn River Basin shale in northeastern British Columbia and found that even in the case of low organic carbon content, adsorption capacity is still strong, as long as the specific surface area is large. Increasing the specific surface area and the number of micro pores with a pore size <2 nm increased the adsorption capacity (Lu et al., 1995; Brandon, 2005; Raut et al., 2007; Chalmers and Bustin, 2008; Weniger et al., 2010; Yan et al., 2013). Several groups compared macro pores with micro pores and found that micro pores had larger specific surface areas and adsorption capacities and concluded that specific surface area is the key factor for adsorption capacity (Dubinin, 1975; Cheng and Huang, 2004; Chalmers and Bustin, 2007; Loucks and Ruppel, 2007; Pan et al., 2019) (Figure 7B). Kim et al. (Kim et al., 2017) indicated that the micro pores account for 56.21%–80.95% of the specific surface area. Wu et al. (Wu et al., 2012) studied gas adsorption capacity in shale and its controlling factors from Well Yuye 1 of Longmaxi Formation of Lower Silurian at the southeast of Chongqing, which indicated that a saturated adsorption capacity has a negative correlation on pore volume; this might be related to smaller pore throat sizes in micro pores that could not reach the kinetic diameter of methane molecules. In contrast, mesopores and macro pores have relatively large pore throats and pore diameters compared to micro pores, making methane molecules more likely to flow into the pores. Therefore, mesopores and macro pores have additional effects on methane adsorption capacity (Yang et al., 2014b). Methane molecules are mainly adsorbed in narrow micro pores of solid asphalt. However, these relationships are complex. In micro pores, the methane adsorption phase density in the smallest pore size is large, and the methane adsorption capacity increases with a decrease in the volume of micro pores; however, for samples with larger pore sizes (pore size >1.1 nm), the amount of methane adsorbed molecules are modest as porosity increases, since CH4 has a lower density (Suárez-Ruiz et al., 2016). Qajar et al. (2015) considered that absorption gas mainly concentrates in pore sizes between 2 nm and 50 nm.
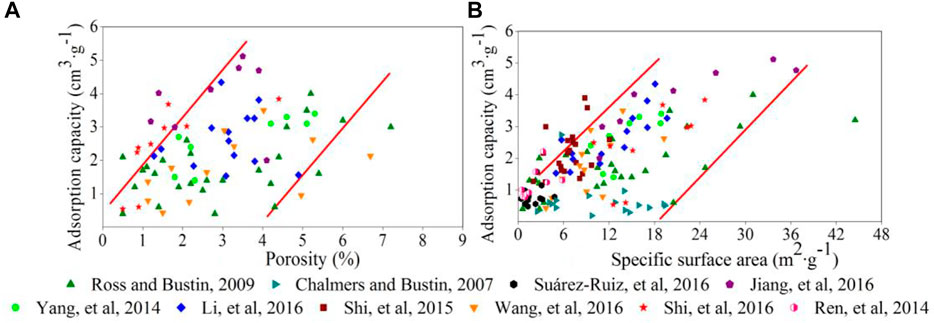
FIGURE 7. (A): Relationship between porosity and methane adsorption capacity and (B): Relationship between specific surface area and methane adsorption capacity.
One the other hand, pore types affect the gas adsorption. Pores in shale are mainly composed of organic matter pores and mineral pores. It is considered that gas is adsorbed on organic pore surfaces and is freely distributed in spaces of clay mineral pores (He et al., 2022). It can be seen that organic matter pores play a major role in methane adsorption (Ross and Bustin, 2009; Huang et al., 2015; Suárez-Ruiz et al., 2016). Hou et al. (2014) studied the Lower Paleozoic marine shale and Mesozoic continental shale in the western Yangtze basin and concluded that clay minerals with low thermal evolution can provide more specific surface area for methane adsorption and that organic matter pores gradually became the primary controlling factor in gas adsorption capacity as the maturity increased.
3.4 Moisture content
Moisture content has an influence on the adsorption capacity of shale. Water occupies more pore space with higher moisture content and reduces the surface position of free hydrocarbon gas shelter volume and adsorption sites on mineral surfaces. Namely, the adsorption capacity is smaller with higher moisture content (Figure 8). Ross and Bustin, 2008 found that when water content is higher (moisture content >4%), adsorption capacity decreases, and the adsorption capacity of saturated water samples is 40% lower than for dried samples. However, Chalmers and Bustin, 2008 studied the adsorption of shale with water content from 1.5% to 11% and found that there is no correlation between water content and adsorption capacity, and that samples with high water content also might have high methane adsorption capacity. Research on gas adsorption abilities in coal with different water content has a certain reference value for understanding the effect of water content on shale gas adsorption capacity. Effective adsorption sites on the inner surface of coal that could be provided for residence of gaseous molecules are limited. As the moisture content in coal increases, the amount of effective absorption sites decreases, and fewer effective absorption sites remain for methane molecules, which decreases the methane adsorption capacity. However, effective adsorption points occupied by water are related to the number of oxygen-containing functional groups. The more oxygen-containing functional groups there are, the greater the water content is, which affects the adsorption capacity. The moisture content is called equilibrium moisture, and once the content exceeds the equilibrium moisture, an increase in moisture content will no longer affect the adsorption capacity (Figure 8).
3.5 Temperature, pressure and the burial depth of shale
Under reservoir conditions, the gas adsorption capacity will be influenced by not only the above factors but also temperature and pressure. Currently, most exploration depths for shale gas has exceeded 1,000 m; this means the reservoir pressure in most areas is more than 5 Mpa which is greater than the critical pressure of methane. Therefore, under the high pressure and reservoir temperature conditions, whether adsorption capacity in shale still follows the adsorption capacity curve law that changes are subject to the Langmuir equation. The gas adsorption process is exothermic. With a temperature increase, gas adsorption capacity decreases (Figure 9). Liu and Wang, 2012 analyzed factors that affected the adsorption capacity in marine mudstone in southern China and found that temperature had a significant effect on adsorption capacity; the maximum adsorption gas content decreased by 1.46 cm3/g from 30°C to 120°C. Chalmers and Bustin, 2007 found that the gas adsorption curve shows a negative exponential state reduction with a temperature increase and has a greater influence on adsorption capacity than organic carbon. Loucks et al. (2009) thought that adsorption gas was negligible and that free gas was the primary gas at higher temperatures.
Adsorption capacity in shale does not change monotonically (monotonically increased) with the change of burial depth because of the combined effect of temperature and pressure, and it appears at the maximum value. Ji et al. (2015) studied methane adsorption capacity in marine shale from the Longmaxi Formation of the Lower Silurian in southern China and found that the maximum methane adsorption value was in the depth range of 800−1,350 m.
4 Challenges of gas adsorption on shale
In the field of shale gas adsorption, although researchers have carried out a great deal of research on many aspects, such as affecting factors of adsorption, adsorption characteristics, adsorption theory, and shale gas adsorption, research in this area still faces some challenges.
(1) Organic matter in shale has complex physical and chemical structures that play important roles in gas adsorption. In the past, the influence of organic matter on adsorption was studied mainly from the aspects of physical structures. However, chemical structures with different kerogen types or different thermal evolution degrees also have effects on gas adsorption. For example, the surface energy of organic matter with different structures is different, resulting in different gas adsorption capacities.
(2) Porosity provides space for shale gas occurrence. Adsorbed gas mainly occurs on the surface of the matrix layer. Currently, there are arguments on the influence of pore size on gas adsorption behavior. On the one hand, micro pores provide more specific surface area, but many pore diameters are smaller than the kinetic diameter of methane molecules. Can the micro pores truly become the main pore space for gas adsorption? On the other hand, clay minerals provide micro pore volumes for gas adsorption that account for 60%–80% of the total pore volumes in shale. It is unclear whether the important contribution to adsorption is via organic matter pore volumes or clay mineral pore volumes?
(3) Organic matter and clay minerals are the main adsorbents for shale gas adsorption. The contribution of clay minerals to gas adsorption is still controversial. Some researchers believe that clay minerals contribute little to gas adsorption; however, the contribution of organic matter and clay minerals to gas adsorption is not clear. The reason is that under actual geological conditions, 90% of organic matter and clay minerals exist in the form of organic-clay complexes; only a small fraction of organic matter is associated with mineral particles. Therefore, the interaction between organic matter and clay minerals and its effects on adsorption still need further research.
(4) Moisture content is another factor that influences shale adsorption behavior, but its impact on shale adsorption is also controversial. Whether the effect of moisture content on gas adsorption in shale is equal to that of coal is a question worth exploring, especially regarding how the presence of expansive minerals impacts adsorption.
(5) With changes in pressure, shale adsorption behavior does not entirely obey the monolayer adsorption. In the supercritical state, gas adsorption will be affected not only by adsorption phase volume but also by the shale’s morphological characteristics, structural features, composition, etc. The impact of these many variables on the mechanism of shale adsorption needs additional study.
(6) Under varying temperature and pressure conditions, adsorption characteristics in shale are almost blank, making an adsorption profile difficult to establish in a geological reservoir. Research has shown that in the coalbed methane field, the adsorption capacity in coal increased with an increase in pressure and decreased with an increase in temperature. Under geological conditions, the influence of pressure on the adsorption capacity plays a dominant role when the burial depth is less than 800–1,500 m, while temperature plays a leading role when the buried depth is more than 800–1,500 m. The influence of burial depth is different in different regions because of different effective stress. In other words, when burial depth is approximately 800–1,500 m, the coal seam has the largest adsorption capacity. The relationship between gas adsorption capacity and pressure is complex. With the influence of temperature, the relationship between the maximum adsorption capacity and depth is even more complex. Research in these areas is currently lacking and needs to be examined in greater detail.
5 Conclusion
Shale adsorption behavior, in addition to its own nature, is greatly impacted by external factors such as pressure and temperature. This paper presents a review of gas adsorption behaviors that can be applied to evaluate the gas content in shale reservoirs.
The main variables of shale gas adsorption are organic matter and clay minerals; other variables include moisture content, TOC, organic matter type and organic matter maturity. It is generally believed that higher shale TOC, vitrinite content or organic matter maturity contributes to a higher gas adsorption capacity. The reason is that there are many of pores in kerogen, especially type III kerogen that provide a comparatively large area for gas occurrence, and pores in organic matter gradually increase with the increase of thermal evolution degree. Brittle minerals will lead to a lower adsorption capacity. Clay minerals are good materials for gas adsorption due to their micro pores, large specific surface area and special electrochemical properties that provide more places for adsorbed gas. There are differences in shale gas adsorption capacities due to differences in chemical composition, crystal structure, particle size and petro physical properties of different clay mineral types. However, the influence of clay minerals on adsorption properties is still controversial. Generally, there exists a negative correlation between moisture content and gas adsorption capacity, but some researchers held the opposite view.
Under reservoir conditions, in addition to the abovementioned factors, temperature and pressure both increase with the increase of burial depth that have an influence on gas adsorption behavior. Gas adsorption capacity decreased with an increase in temperature. As the pressure increases, gas adsorption characteristics mainly show two characteristics—an adsorption curve that obeyed the Langmuir equation and another where the adsorption maximum value appeared as a “single peak” adsorption phenomenon with an inverted U shape at a pressure value distribution in the range of 5–12 MPa. The latter characteristic is difficult to describe with the Langmuir equation. In addition to the influence of adsorbed volume phase, composition, structure and morphology also have influences on shale.
There are still many problems in the study of adsorption behaviors in shale that pose a great challenge for research in this field. These problems include chemical structures of organic matter in shale; pore size distribution; clay minerals; interaction between clay minerals and organic matter; and especially the factor of moisture content which is rich in clay minerals. All of these variables need to be further studied. Under reservoir temperature and pressure conditions, the adsorption characteristics are difficult to describe by the single molecular layer adsorption theory. The mechanism of adsorption behavior changes and the adsorption model involving a variety of factors are problems that urgently need to be solved, with a particular emphasis on studying the variation of temperature and pressure with the influence of other factors that directly affect the estimation of adsorption content in shale under reservoir conditions.
Author contributions
XL is responsible for the overall conception of the paper and the writing of Sections 1, 3.1, 3.2.3.3, 4 and 5, CL is responsible for the information search and writing of Section 2, and ZW is responsible for the writing of Sections 3.4 and 3.5.
Funding
We acknowledge the National Natural Science Foundation Projects of China (42272199), the Natural Science Foundation Projects of Henan Province (222300420556), the Cultivate Funds for Young Teachers of Zhenghou University (JC202042035) for providing research funding.
Conflict of interest
The authors declare that the research was conducted in the absence of any commercial or financial relationships that could be construed as a potential conflict of interest.
Publisher’s note
All claims expressed in this article are solely those of the authors and do not necessarily represent those of their affiliated organizations, or those of the publisher, the editors and the reviewers. Any product that may be evaluated in this article, or claim that may be made by its manufacturer, is not guaranteed or endorsed by the publisher.
References
Aranovich, G. L., Sangwichien, C., and Donohue, M. D. (2000). Intermolecular repulsions in adsorbed layers. J. Colloid Interface Sci. 227 (2), 553–560. doi:10.1006/jcis.2000.6893
Bernaola-Galvan, P., Ivanov, P. C., Nunes Amaral, L. A., and Stanley, H. E. (2001). Scale invariance in the nonstationarity of human heart rate. Phys. Rev. Lett. 87 (16), 168105. doi:10.1103/PhysRevLett.87.168105
Bowker, K. A. (2007). Barnett shale gas production, Fort worth basin: Issues and discussion. Am. Assoc. Pet. Geol. Bull. 91 (4), 523–533. doi:10.1306/06190606018
Brandon, C. N. (2005). Analysis of devonian black shales in Kentucky for potential carbon dioxide sequestration and enhanced natural gas production quarterly report. United States, United States. doi:10.2172/842849
Chalmers, G. R. L., and Bustin, R. M. (2008). Lower cretaceous gas shales in northeastern British Columbia, Part I: Geological controls on methane sorption capacity. Bull. Can. Petroleum Geol. 56 (1), 1–21. doi:10.2113/gscpgbull.56.1.1
Chalmers, G. R. L., and Bustin, R. M. (2007). The organic matter distribution and methane capacity of the lower Cretaceous strata of northeastern British Columbia, Canada. Int. J. Coal Geol. 70 (1-3), 223–239. doi:10.1016/j.coal.2006.05.001
Chareonsuppanimit, P., Mohammad, S. A., Robinson, R. L., and Gasem, K. A. M. (2012). High-pressure adsorption of gases on shales: Measurements and modeling. Int. J. Coal Geol. 95, 34–46. doi:10.1016/j.coal.2012.02.005
Chen, Y., Zou, C., Maria, M., Zhu, R., Yang, Z., et al. (2015). Porosity and fractal characteristics of shale across a maturation gradient. Nat. Gas. Geosci. 26 (9), 1646–1656. doi:10.11764/j.issn.1672-1926.2015.09.1646
Cheng, A. L., and Huang, W. L. (2004). Selective adsorption of hydrocarbon gases on clays and organic matter. Org. Geochem. 35 (4), 413–423. doi:10.1016/j.orggeochem.2004.01.007
Chilev, C., Lamari, F. D., Kirilova, E., and Pentchev, I. (2012). Comparison of gas excess adsorption models and high pressure experimental validation. Chem. Eng. Res. Des. 90 (11), 2002–2012. doi:10.1016/j.cherd.2012.03.012
Day, S., Duffy, G., Sakurovs, R., and Weir, S. (2008). Effect of coal properties on CO2 sorption capacity under supercritical conditions. Int. J. Greenh. Gas Control 2 (3), 342–352. doi:10.1016/s1750-5836(07)00120-x
Do, D. D., and Do, H. D. (2005). Adsorption of argon from sub- to supercritical conditions on graphitized thermal carbon black and in graphitic slit pores: A grand canonical Monte Carlo simulation study. J. Chem. Phys. 123 (8), 084701. doi:10.1063/1.1996573
Dong, D., Zou, C., Yang, H., Wang, Y., and Li, X. (2012). Progress and prospects of shale gas exploration and development in China. Acta Pet. Sin. 33 (S1), 107–114. doi:10.7623/syxb2012S1013
Dreisbach, F., Losch, H. W., and Harting, P. (2002). Highest pressure adsorption equilibria data: Measurement with magnetic suspension balance and analysis with a new adsorbent/adsorbate-volume. Adsorpt. Bost. 8 (2), 95–109. doi:10.1023/a:1020431616093
Dubinin, M. M. (1975). Physical adsorption of gases and vapors in micropores (Elsevier), 1–70. D.A, C., J.F, D., M.D, R. doi:10.1016/B978-0-12-571809-7.50006-1
Fang, J., Zhu, Y., Wei, W., Du, Z., and Chen, S. (2010). Preliminary study of abnormalities of shale isothermal adsorption experiment. Tuha Oil Gas 15 (4), 317–320.
Franco, C. A., Nassar, N. N., Ruiz, M. A., Pereira-Almao, P., and Cortes, F. B. (2013). Nanoparticles for inhibition of asphaltenes damage: Adsorption study and displacement test on porous media. Energy fuels. 27 (6), 2899–2907. doi:10.1021/ef4000825
Gensterblum, Y., Merkel, A., Busch, A., and Krooss, B. M. (2013). High-pressure CH4 and CO2 sorption isotherms as a function of coal maturity and the influence of moisture. Int. J. Coal Geol. 118, 45–57. doi:10.1016/j.coal.2013.07.024
He, J., Cai, J., Lei, T., Song, M., Liu, H., and Wang, X. (2022). Characteristics comparison and occurrence mode of different types of soluble organic matter in lacustrine shale in the Dongying Sag, eastern China. Int. J. Coal Geol. 254, 103971. doi:10.1016/j.coal.2022.103971
Heller, R., and Zoback, M. (2014). Adsorption of methane and carbon dioxide on gas shale and pure mineral samples. J. Unconv. Oil Gas Resour. 8, 14–24. doi:10.1016/j.juogr.2014.06.001
Hill, R. J., Jarvie, D. M., Zumberge, J., Henry, M., and Pollastro, R. M. (2007). Oil and gas geochemistry and petroleum systems of the Fort Worth Basin. Am. Assoc. Pet. Geol. Bull. 91 (4), 445–473. doi:10.1306/11030606014
Hinai, A. A., Rezaee, R., Esteban, L., and Labani, M. (2014). Comparisons of pore size distribution: A case from the western Australian gas shale formations. J. Unconv. Oil Gas Resour. 8, 1–13. doi:10.1016/j.juogr.2014.06.002
Hou, H., Shao, L., Li, Y., Liu, L., Liang, G., Zhang, W., et al. (2022). Effect of paleoclimate and paleoenvironment on organic matter accumulation in lacustrine shale: Constraints from lithofacies and element geochemistry in the northern Qaidam Basin, NW China. J. Pet. Sci. Eng. 208, 109350. doi:10.1016/j.petrol.2021.109350
Hou, Y., He, S., Yi, J., Zhang, B., Chen, X., Wang, Y., et al. (2014). Effect of pore structure on methane sorption potential of shales. Petroleum Explor. Dev. 41 (2), 272–281. doi:10.1016/S1876-3804(14)60033-1
Huang, X., Zhang, J., Li, X., Sun, R., Peng, J., and Long, S. (2015). Pore types and characteristics of continental shale and discussion on the process of oil and gas accumulation:A case study of the western sag of liaohe depression. Nat. Gas. Geosci. 26 (7), 1422–1432. doi:10.11764/j.issn.1672-1926.2015.07.1422
Ji, L., Qiu, J., and Xia, Y. (2012a). Micro-pore characteristics and methane adsorption properties of common clay minerals by electron microscope scanning. Acta Pet. Sin. 33 (2), 249–256. doi:10.7623/syxb201202009
Ji, L., Qiu, J., Zhang, T., and Xia, Y. (2012b). Experiments on methane adsorption of common clay minerals in shale. Earth Sci. J. China Univ. Geosci. (China) 37 (5), 1043–1050. doi:10.3799/dqkx.2012.111
Ji, W., Song, Y., Jiang, Z., Chen, L., Li, Z., Yang, X., et al. (2015). Estimation of marine shale methane adsorption capacity based on experimental investigations of Lower Silurian Longmaxi formation in the Upper Yangtze Platform, south China. Mar. Pet. Geol. 68, 94–106. doi:10.1016/j.marpetgeo.2015.08.012
Jiang, Z., Tang, X., Li, Z., Huang, H., Yang, P., Yang, X., et al. (2016). The whole-aperture pore structure characteristics and its effect on gas content of the Longmaxi Formation shale in the southeastern Sichuan basin. Earth Sci. Front. 23 (2), 126–134. doi:10.13745/j.esf.2016.02.013
Jiang, Z., Zhao, L., and Zhang, D. (2018). Study of adsorption behavior in shale reservoirs under high pressure. J. Nat. Gas. Sci. Eng. 49, 275–285. doi:10.1016/j.jngse.2017.11.009
Kennedy, M. J., Pevear, D. R., and Hill, R. J. (2002). Mineral surface control of organic carbon in black shale. Science 295 (5555), 657–660. doi:10.1126/science.1066611
Kim, J., Kim, D., Lee, W., Lee, Y., and Kim, H. (2017). Impact of total organic carbon and specific surface area on the adsorption capacity in Horn River shale. J. Pet. Sci. Eng. 149, 331–339. doi:10.1016/j.petrol.2016.10.053
Kinley, T. J., Cook, L. W., Breyer, J. A., Jarvie, D. M., and Busbey, A. B. (2008). Hydrocarbon potential of the Barnett shale (mississippian), Delaware basin, west Texas and southeastern new Mexico. Am. Assoc. Pet. Geol. Bull. 92 (8), 967–991. doi:10.1306/03240807121
Li, A., Ding, W., He, J., Dai, P., Yin, S., and Xie, F. (2016). Investigation of pore structure and fractal characteristics of organic-rich shale reservoirs: A case study of lower cambrian qiongzhusi formation in malong block of eastern yunnan Province, south China. Mar. Pet. Geol. 70, 46–57. doi:10.1016/j.marpetgeo.2015.11.004
Li, X., Suyun, H. U., and Cheng, K. (2007). Suggestions from the development of fractured shale gas in North America. Petroleum Explor. Dev. 34 (4), 392–400. doi:10.3321/j.issn:1000-0747.2007.04.002
Lin, L. M., Zhang, J. C., Xuan, T., and Hua, Z. (2012). Gas-bearing characteristics of paleozoic gas shale reservoir in south China. J. Jilin Univ. Sci. Ed. 42, 88–94.
Lin, X., Huang, M., Chen, H., Wang, J., and Wang, R. (2019). Influence of different polar solvent extraction on pore structure in shale. Acta Pet. Sin. 40 (12), 1485–1494. doi:10.7623/syxb201912007
Liu, H., and Wang, H. (2012). Adsorptivity and influential factors of marine shales in South China. Nat. Gas. Ind. 32 (9), 5–9. doi:10.3787/j.issn.1000-0976.2012.09.002
Loucks, R. G., Reed, R. M., Ruppel, S. C., and Jarvie, D. M. (2009). Morphology, Genesis, and distribution of nanometer-scale pores in siliceous mudstones of the Mississippian Barnett Shale. J. Sediment. Res. 79 (11-12), 848–861. doi:10.2110/jsr.2009.092
Loucks, R. G., and Ruppel, S. C. (2007). Mississippian Barnett shale: Lithofacies and depositional setting of a deep-water shale-gas succession in the Fort worth basin, Texas. Am. Assoc. Pet. Geol. Bull. 91 (4), 579–601. doi:10.1306/11020606059
Lu, X. C., Li, F. C., and Watson, A. T. (1995). Adsorption measurements in Devonian shales. Fuel 74 (4), 599–603. doi:10.1016/0016-2361(95)98364-k
Malbrunot, P., Vidal, D., Vermesse, J., Chahine, R., and Bose, T. K. (1992). Adsorption measurements of argon, neon, krypton, nitrogen, and methane on activated carbon up to 650 MPa. Langmuir 8 (2), 577–580. doi:10.1021/la00038a044
Marecka, A., and Mianowski, A. (1998). Kinetics of CO2 and CH4 sorption on high rank coal at ambient temperatures. Fuel 77 (14), 1691–1696. doi:10.1016/s0016-2361(98)00071-4
Mavor, M. (2003). Barnett shale gas-in-place volume including sorbed and free gas volume. Texas, USA: AAPG Southwest Section Meeting.
Menon, P. G. (1965). Adsorption of carbon monoxide on alumina at high Pressures1. J. Am. Chem. Soc. 87 (14), 3057–3060. doi:10.1021/ja01092a006
Montgomery, S. L., Jarvie, D. M., Bowker, K. A., and Pollastro, R. M. (2005). Mississippian Barnett Shale, Fort Worth basin, north-central Texas: Gas-shale play with multi-trillion cubic foot potential. Am. Assoc. Pet. Geol. Bull. 89 (2), 155–175. doi:10.1306/09170404042
Nandi, S. P., and Walker, P. L. (1975). Activated diffusion of methane from coals at elevated pressures. Fuel 54 (2), 81–86. doi:10.1016/0016-2361(75)90061-7
Nie, H., and Zhang, J. (2010). Shale gas reservoir distribution geological law, characteristics and suggestions. J. Cent. South Univ. Sci. Technol. (China) 41 (2), 700–708.
Niu, Q. H., Cao, L., Sang, S., Wang, W., Zhou, X., Yuan, W., et al. (2021). Experimental study on the softening effect and mechanism of anthracite with CO2 injection. Int. J. Rock Mech. Min. Sci. 138, 104614. doi:10.1016/j.ijrmms.2021.104614
Pan, J. N., Lv, M. M., Hou, Q. L., Han, Y. Z., and Wang, K. (2019). Coal microcrystalline structural changes related to methane adsorption/desorption. Fuel 239, 13–23. doi:10.1016/j.fuel.2018.10.155
Park, S. H., and Sposito, G. (2003). Do montmorillonite surfaces promote methane hydrate formation? Monte Carlo and molecular dynamics simulations. J. Phys. Chem. B 107 (10), 2281–2290. doi:10.1021/jp021427q
Perera, M. S. A., Ranjith, P. G., Choi, S. K., Airey, D., and Weniger, P. (2012). Estimation of gas adsorption capacity in coal: A review and an analytical study. Int. J. Coal Prep. Util. 32 (1), 25–55. doi:10.1080/19392699.2011.614298
Qajar, A., Daigle, H., and Prodanovic, M. (2015). Methane dual-site adsorption in organic-rich shale-gas and coalbed systems. Int. J. Coal Geol. 149, 1–8. doi:10.1016/j.coal.2015.07.006
Rani, S., Prusty, B. K., and Pal, S. K. (2015). Methane adsorption and pore characterization of Indian shale samples. J. Unconv. Oil Gas Resour. 11, 1–10. doi:10.1016/j.juogr.2015.03.003
Raut, U., Fama, M., Teolis, B. D., and Baragiola, R. A. (2007). Characterization of porosity in vapor-deposited amorphous solid water from methane adsorption. J. Chem. Phys. 127 (20), 204713. doi:10.1063/1.2796166
Ren, Z., Liu, L., Gao, X., Xiao, F., Wang, Y., Wu, K., et al. (2014). Adsorption capacity and its influence factors of the jurassic shalein the northeastern kuqa depression. Nat. Gas. Geosci. 25 (4), 632–640. doi:10.11764/j.issn.1672-1926.2014.04.0632
Ross, D. J. K., and Bustin, R. M. (2008). Characterizing the shale gas resource potential of Devonian-Mississippian strata in the Western Canada sedimentary basin: Application of an integrated formation evaluation. Am. Assoc. Pet. Geol. Bull. 92 (1), 87–125. doi:10.1306/09040707048
Ross, D. J. K., and Bustin, R. M. (2007). Impact of mass balance calculations on adsorption capacities in microporous shale gas reservoirs. Fuel 86 (17-18), 2696–2706. doi:10.1016/j.fuel.2007.02.036
Ross, D. J. K., and Bustin, R. M. (2009). The importance of shale composition and pore structure upon gas storage potential of shale gas reservoirs. Mar. Pet. Geol. 26 (6), 916–927. doi:10.1016/j.marpetgeo.2008.06.004
Salem, M. M. K., Braeuer, P., Szombathely, M. v., Heuchel, M., Harting, P., Quitzsch, K., et al. (1998). Thermodynamics of high-pressure adsorption of argon, nitrogen, and methane on microporous adsorbents. Langmuir 14 (12), 3376–3389. doi:10.1021/la970119u
Siemons, N., and Busch, A. (2007). Measurement and interpretation of supercritical CO2 sorption on various coals. Int. J. Coal Geol. 69 (4), 229–242. doi:10.1016/j.coal.2006.06.004
Suárez-Ruiz, I., Juliao, T., Suárez-García, F., Marquez, R., and Ruiz, B. (2016). Porosity development and the influence of pore size on the CH4 adsorption capacity of a shale oil reservoir (Upper Cretaceous) from Colombia. Role of solid bitumen. Int. J. Coal Geol. 159, 1–17. doi:10.1016/j.coal.2016.03.020
Tang, S., Yang, Q., and Tang, D. (2003). Comparison between the experimental results of isothermal adsorption of binary mixed gas and the predicted values of the extended Langmuir equation. Geol. Sci. Technol. Inf. 2, 68–70. doi:10.3969/j.issn.1000-7849.2003.02.014
Wang, C. C., Juang, L. C., Lee, C. K., Hsu, T. C., Lee, J. F., and Chao, H. P. (2004). Effects of exchanged surfactant cations on the pore structure and adsorption characteristics of montmorillonite. J. Colloid Interface Sci. 280 (1), 27–35. doi:10.1016/j.jcis.2004.07.009
Wang, Z. Z., Pan, J., Hou, Q., Yu, B., Li, M., and Niu, Q. (2018). Anisotropic characteristics of low-rank coal fractures in the Fukang mining area, China. Fuel 211, 182–193. doi:10.1016/j.fuel.2017.09.067
Weniger, P., Kalkreuth, W., Busch, A., and Krooss, B. M. (2010). High-pressure methane and carbon dioxide sorption on coal and shale samples from the Parana Basin, Brazil. Int. J. Coal Geol. 84 (3-4), 190–205. doi:10.1016/j.coal.2010.08.003
Wu, J., Yu, B., and Li, Y. (2012). Adsorption capacity of shale gas and controlling factors from the well Yuye 1 at the southeast of chongqing. J. Southwest Petroleum Univ. 34 (4), 40–48. doi:10.3863/j.issn.1674-5086.2012.04.005
Yan, J., Zhang, T., Li, Y., Lu, H., and Zhang, X. (2013). Effect of the organic matter characteristics on methane adsorption in shale. J. China Coal Soc. 38 (5), 805–811.
Yang, F., Ning, Z., Wang, Q., Liu, H., and Kong, D. (2014a). Thermodynamic analysis of methane adsorption on gas shale. J. Central South Univ. Sci. Technol. 45 (8), 2871–2877.
Yang, F., Ning, Z. F., and Liu, H. Q. (2014b). Fractal characteristics of shales from a shale gas reservoir in the Sichuan Basin, China. Fuel 115, 378–384. doi:10.1016/j.fuel.2013.07.040
Yu, H., Fan, W., Sun, M., and Ye, J. (2004). Study on fitting models for methane isotherms adsorption of coals. J. China Coal Soc. 29 (4), 463–467. doi:10.3321/j.issn:0253-9993.2004.04.019
Yu, C., Nie, H., Zeng, C., Cheng, L., and Shao, X. (2014). Shale reservoir space characteristics and the effect on gas content in lower palaeozoic erathem of the eastern Sichuan Basin. Acta Geol. Sin. 88 (7), 1311–1320. doi:10.3969/j.issn.0001-5717.2014.07.008
Zhang, Q., and Cao, L. (2003). Study of data processing in coal orption isotherm testing. J. China Coal Soc. 28 (2), 131–135. doi:10.1115/1.1564062
Zhang, J., Wang, Z., Nie, H., Xu, B., Deng, F., Zhang, P., et al. (2008). Shale gas and its significance for exploration. Geoscience 22 (4), 640–646. doi:10.3787/j.issn.1000-0976.2008.06.040
Zhang, T., Ellis, G., Ruppel, S., Milliken, K., and Yang, R. (2012). Effect of organic-matter type and thermal maturity on methane adsorption in shale-gas systems. Org. Geochem. 47, 120–131. doi:10.1016/j.orggeochem.2012.03.012
Zhang, Z., Liu, G., Zhang, X., and Yang, X. (2009). Adsorption-disorption experiments of CH_4 and CO_2 with different consistency. J. China Coal Soc. 34 (04), 551–555.
Zhou, C., Hall, F., Gasem, K., and Robinson, R. L. (1994). Predicting gas adsorption using two-dimensional equations of state. Ind. Eng. Chem. Res. 33 (5), 1280–1289. doi:10.1021/ie00029a026
Zou, C., Li, J., Dong, D., and Li, X. (2010). Abundant nanoscale pores with founding in shale gas reservoirs for the first time in China. Petroleum Explor. Dev. 37 (05), 508–509.
Zou, C., Dong, D., Yang, H., Wang, Y., and Huang, J. (2011). Formation conditions and shale gas exploration in China. Nat. Gas. Ind. 31 (12), 26–39+125. doi:10.3787/j.issn.1000-0976.2011.12.005
Keywords: shale gas, adsorption behavior, organic matter, pore characteristics, moisture content, clay minerals, temperature, pressure
Citation: Lin X, Liu C and Wang Z (2023) The influencing factors of gas adsorption behaviors in shale gas reservoirs. Front. Earth Sci. 10:1021983. doi: 10.3389/feart.2022.1021983
Received: 18 August 2022; Accepted: 22 September 2022;
Published: 09 January 2023.
Edited by:
Zhenzhi Wang, Henan Polytechnic University, ChinaReviewed by:
Haihai Hou, Liaoning Technical University, ChinaRun Chen, China University of Mining and Technology, China
Daping Xia, Henan Polytechnic University, China
Copyright © 2023 Lin, Liu and Wang. This is an open-access article distributed under the terms of the Creative Commons Attribution License (CC BY). The use, distribution or reproduction in other forums is permitted, provided the original author(s) and the copyright owner(s) are credited and that the original publication in this journal is cited, in accordance with accepted academic practice. No use, distribution or reproduction is permitted which does not comply with these terms.
*Correspondence: Xiaoying Lin, bGlueGlhb3lpbmdAenp1LmVkdS5jbg==