- 1State Key Laboratory of Oil and Gas Reservoir Geology and Exploitation, Chengdu University of Technology, Chengdu, China
- 2Petrochina Southwest Oil and Gasfield Company, Chengdu, China
Though sedimentary mercury in marine sediments has been regarded as a geological indicator of volcanic activity based on volcanism activity is the dominant natural source of Hg to the oceans, the influence of diagenesis on mercury cannot be dismissed. Marine mudstone has been selected to explore the migration of mercury from the syn-sedimentary stage to the diagenetic stage in this review. Marine mudstones undergo a series of significant transformation processes, including the illitization of smectite and the formation of framboidal pyrite aggregates during the diagenetic stage. This process makes the adsorption capacity of minerals change significantly that clay minerals are weakened, while pyrites are enhanced. In this reason, it is inferred that the sedimentary mercury may re-migrate from clay minerals to pyrite. This at least means that the indication of volcanic activity by mercury enrichment in marine mudstone need to be re-evaluated.
Introduction
In the Phanerozoic Earth, there is a correlation between volcanic activity and several major biological extinction events, such as in the Ordovician/Silurian, Permian/Triassic and Cretaceous/Paleogene (Font et al., 2016; Grasby et al., 2016; Sial et al., 2016; Shen et al., 2019a; Shen et al., 2019b). Mercury has been widely recognized as tracer evidence of volcanic activity in sedimentary records from Large Igneous Provinces (LIPs), and so the enrichment of sedimentary mercury before and after biological extinctions has been used to assess regional/global volcanic activity (Grasby et al., 2019; Shen et al., 2020).
Mercury is mainly present in various inorganic species in marine sediments (>98% of total Hg; Morel et al., 1998; Boszke et al., 2003). The retention of mercury in sediments usually should require attachment to the mineral surfaces, including organic matter, clay minerals, sulfides, and other phases (Krupp, 1998; Oliveri et al., 2016; Ravichandran, 2004; Sanei et al., 2012; Selin, 2009). Organic matter mainly exists in form of organic-mercury complex (Ravichandran, 2004). Clay minerals can also adsorb mercury because of its high surface area, moderate to high cation exchange capacity and high negative surface charge (Farrah and Pickering, 1978; Horowitz, 1991). Sulfide minerals may be the main hosts of Hg in anoxic environments due to the high affinity of Hg for sulfide (Bower et al., 2008; Shen et al., 2019c). Other phases (such as metal oxides) also have certain adsorption capacity for mercury, but they do not play an absoulute role in mercury adsorption (Gobeil and Cossa, 1993; Feyte et al., 2010). According to the modern and ancient mercury sedimentary hosts, the main hosts of mercury include organic matter, clay minerals and pyrite (Shen et al., 2020).
In Mcneal and Rose (1974), mercury content in unconsolidated sediments was much higher than those in sedimentary rocks, suggesting that mercury is lost during diagenesis. Furthermore, when sediment undergoes redox changes because of organic matter mineralization (Froelich et al., 1979), the Hg bound to or-ganic matter can be released in pore water (Cossa and Gobeil, 2000; Gagnon et al., 1997). All of the above suggest that mercury re-migration may occur after entering the sediment.
Because most minerals are transformed during the diagenetic stage, the possibility that Hg may move after entering sediments cannot be ignored (Young et al., 1973). This problem has also led to intensive debate about whether the core profiles of Hg enrichment at the sediment surface reflect digenesis (Rasmussen, 1994). However, the specific change mechanism of mercury in the diagenetic stage is still unclear. For this reason, based on previous studies on diagenesis of marine mudstones, we propose a remobilization mechanism of mercury that mercury migration from clay minerals to pyrite. Finally, when this migration reaches a certain extent, mercury is no longer suitable as an effective tracer for the volcanic activity of the same fire province.
Properties of mercury
Mercury is a toxic heavy metal that exists in nature with a variety of sources, including soils and surface volatilization of water bodies, geothermal and volcanic activities, forest fires, spontaneous combustion of coal and degassing of the Earth’s crust (Lindberg and Stratton, 1998; Gustin, 2003; Obrist, 2007). Among which, volcanic eruption is the main natural source of mercury (Pirrone et al., 2010).
There are three main types of atmospheric mercury, including gaseous elemental mercury (Hg0), active mercury ion (Hg2+) and Hg2+ compounds associated with particles (Hg(P)) (Schroeder and Munthe, 1998). It usually settles to the sea surface, soils or plant surface through dry or wet deposition (dry deposition: settling or uptake the surface without precipitation; wet deposition: removel from the atmosphere through precipitation; Mason et al., 1994; Selin, 2009). Hg0 can remain in the atmosphere for a long-time period (0.5–1 year; Selin, 2009), and be responsible for long-distance transportation and exchange, based on its low water solubility and dry deposition rate (Lamborg et al., 2002; Selin, 2009), but also is the main occurrence state, accounting for 97%–99% of the total mercury content (Fitzgerald, 1995). Compared to Hg0, the content of Hg2+ is less than 3% and it is soluble in water (Lindberg and Stratton 1998).
In the atmosphere-ocean system (Figure 1), Hg0 can be oxidized to Hg2+, which is removed from the atmosphere by the oxidation by halogen, ozone and other free radicals (Schroeder and Munthe, 1998; Selin, 2009). Some Hg2+ arriving at the seawater surface could be transformed through photochemical reduction and then re-emitted to the atmosphere (Ravichandran, 2004). Under biotic and abiotic action, Hg2+ can convert into methylmercury (Me-Hg) and mercury sulfide complex (HgS), respectively (Ravichandran, 2004). Me-Hg can then be used as an intermediate to form organic mercury complex (Hg-OM), which is deposited into sediments or adsorbed by clay and pyrite, while HgS would be precipitated in an environment rich in hydrogen sulfide (Percival et al., 2015).
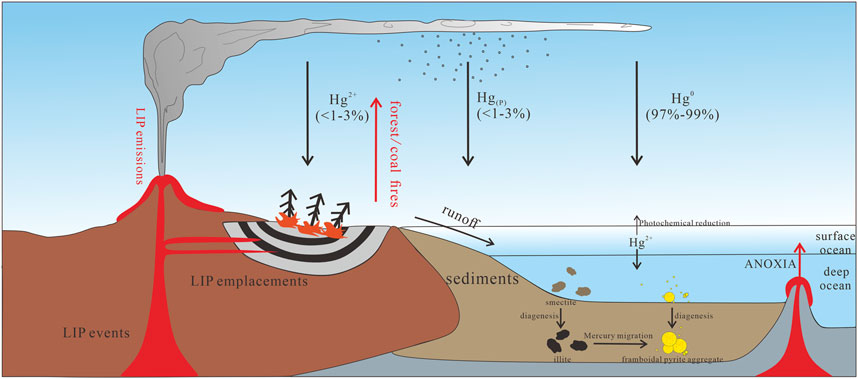
FIGURE 1. Circulation, transport and form of mercury in nature (modified from Percival et al., 2015).
Migration of mercury
Generally, the transportation of mercury from volcanic activity to sediments is the main focus, but the migration in the diagenetic stage has been rarely studied previously. Due to the influence of diagenesis, mercury may undergo significant migration (Smit et al., 2016). Based on the mobility of mercury in mineral transformation, we propose a migration mechanism from clay minerals to pyrite during diagenesis. Therefore, it is of interest to explore the migration processes of mercury in water bodies, and during syn-sedimentary, and diagenesis stages (Figure 2). This will affect the effectiveness of mercury as an indicator of volcanic activity.
In water bodies
The mercury derived from the atmosphere or terrigenous debris before entering the water mass is mainly Hg(P) and Hg2+ ions (Lin and Pehkonen, 1999). After entering a water body, particulate mercury can be directly adsorbed by porous media, such as organic matter, clay minerals and pyrite (Ravichandran, 2004; Holmes et al., 2009). Under the biotic and abiotic actions, most Hg2+ would form Me-Hg and be adsorbed by organic matter, clay minerals and framboidal pyrite (Amyot et al., 1997; Holmes et al., 2009). Some Hg2+ reacts with sulfide to form HgS in anoxic water which could be directly precipitated when the water column becomes saturated (Ravichandran, 2004). Unsaturated in concentration, it is methylated to form Me-Hg which would endure the transformation to migration to precipitation process (Niessen et al., 2003).
Syn-sedimentary stage
At this stage, there are mainly three kinds of porous media for mercury adsorption, including organic matter, clay minerals dominated by smectite and framboidal pyrite (Shen et al., 2020), although other hosts (iorn and manganese oxides) can also have an important role (Gobeil and Cossa, 1993; Feyte et al., 2010). Organic matter and clay minerals mainly come from terrestrial weathering products (Singer, 1984), while framboidal pyrite is a syn-sedimentary authigenic mineral formed in anoxic water columns (Wilkin and Barnes, 1997). The formation of sedimentary pyrite is mainly related to bacterial sulfate reduction (BSR) (Berner, 1984). In anoxic water, organic matter is used as reducing agent and energy source to form sulfide (mainly hydrogen sulfide) (Eq. 1), which combines with free active iron to form iron monosulfide microcrystals (Eq. 2) (Wilkin et al., 1996). The microcrystals react to form greigite (Fe3S4) and aggregate, and finally form framboidal pyrite (Wilkin et al., 1996). Then framboidal pyrite monomer can be accumulated and preserved in sediments after gathering (Wilkin and Barnes, 1997).
Diagenetic stage
There are three main groups of clay minerals, namely smectite, illite and kaolinite. During the diagenetic stage, transformations take place, including illitzation of smectite and kaolinite (Curtis, 1985). The transformation of smectite to illite is a most important diagenetic chemical transformation in mudstone with increasing burial depth (Pollastro, 1993; Schicker et al., 2021).
During diagenesis, the petroleum generation process can theoretically release a large quantity of organic acid and carbon dioxide (CO2) (Barth and Bjorlykke, 1993; Seewald, 2003). This process can promote the dissolution of minerals (mainly feldspar and mica), and then release a large quantity of potassium ions, which would enhance the transformation of smectite to illite (Eq. 3) (Berger et al., 1999). Among the clay minerals, smectite not only has the largest specific surface area (Passey et al., 2010), but also is the only mineral that has a significant correlation with mercury (Kongchum et al., 2011). Undergoing transformation of smectite to illite, the adsorption capacity of clay minerals is substantially reduced, which could lead to mercury that was adsorbed by smectite in the syn-sedimentary stage entering into pore water to find a new host (Loukola-Ruskeeniemi et al., 2003). However, because of the Hg-OM have relatively high stability constants (1022–1028) (Ravichandran, 2004) and the strong reducing environment of marine mudstones, the mineralization organic matter contributes little to the mercury content in pore water.
At the same time, pyrite also undergoes significant transformation in mudstone. The single particle (diameter <6 ㎛) framboidal pyrite monomer formed during syn-sedimentary deposition has a certain adsorption capacity (Wilkin et al., 1996; Wilkin and Barnes, 1997). Under certain conditions, because of a large quantity of sulfur and iron sources enriching, the individual framboidal pyrite crystals began to form framboidal pyrite aggregates, which could greatly improve the specific surface areas (Wilkin and Barnes, 1997). At this time, with the increase of the specific surface area, the adsorption capacity of pyrite can be greatly improved (Pugh et al., 1981). Furthermore, mercury is associated with abundant pyrite in Shen et al. (2019c), which commonly has a stonger affinity for Hg than other potential host phases (e.g., organic matter, clay minerals; Ravichandran, 2004; Bower et al., 2008). Therefore, free mercury being released by smectite can enter the framboidal pyrite aggregate.
Previous studies have demonstrated that clay minerals are one of the main hosts of mercury (Selin, 2009; Kalvoda et al., 2019; Shen et al., 2019d). However, in the Jiaoye section of the Ordovician/Silurian in South China, it is found that there is almost no mercury in the clay minerals, while a large amount of mercury is enriched in the framboidal pyrite aggregates (Shen et al., 2019c). This also demonstrates that the re-migration of mercury from clay minerals to pyrite during diagenesis. Furthermore, mercury isotope mass-independent fractionation (Hg-MIF) generally occurs in special photochemical processes, such as photoreduction or photooxidation (Blum et al., 2014). Therefore, the Hg-MIF can effectively identify the source of mercury. However, photochemical processes are not involved in the diagenesis process, so the isotopic mass-independent fractionation of mercury did not change significantly during this migration process. In other words, the isotopes do not distinguish whether the source of mercury is diagenetic re-migration. Therefore, when the amount of sedimentary mercury that migrates between layers is large enough, it means that the sedimentary mercury cannot be used as an effective indicator of volcanic activity in LIPs.
Conclusion
According to previous studies, it is found that the diagenetic transformation of the main host of mercury has a great influence on the retention of mercury. In addition, there is a phenomenon in the marine Ordovician/Silurian boundary in South China that a large quantity of mercury is enriched in framboidal pyrite aggregates, but almost lost in clay minerals. These aspects support the assumption of sedimentary mercury migration. For this reason, a diagenetic re-migration model of mercury based on mineral transformation is proposed that migration from clay minerals to pyrite. However, mercury is not suitable as an effective tracer of volcanic activity in large igneous provinces when the interlayer flow of mercury occurs and the mobility is sufficient to limit the reconstruction of the paleoenvironment by mercury. Therefore, inferences about volcanic mercury inputs associated with LIP may need to be re-evaluated. Finally, future research needs to better understand the influence of diagenesis on the migration of mercury are recommended.
Author contributions
BR and SL conceived and designed the study. HX and BR wrote the first draft of the manuscript. TS provided constructive suggestions for improving the work. CL, YL, and YZ edited and reviewed the manuscript.
Funding
The article is supported by the National Scientific Fund Project “Fundamental Study on Marine Deep-Layer Oil and Gas Enrichment Mechanism and Key Engineering Technologies” (No. U19B6003) and the National Natural Science Foundation of China (NSFC, Project NO. 42072129).
Conflict of interest
The authors CL, YL, and YZ were employed by Petrochina Southwest Oil and Gasfield Company.
The remaining authors declare that the research was conducted in the absence of any commercial or financial relationships that could be construed as a potential conflict of interest.
Publisher’s note
All claims expressed in this article are solely those of the authors and do not necessarily represent those of their affiliated organizations, or those of the publisher, the editors and the reviewers. Any product that may be evaluated in this article, or claim that may be made by its manufacturer, is not guaranteed or endorsed by the publisher.
References
Amyot, M., Gill, G. A., and Morel, F. M. (1997). Production and loss of dissolved gaseous mercury in coastal seawater. Environ. Sci. Technol. 31 (12), 3606–3611. doi:10.1021/es9703685
Barth, T., and Bjørlykke, K. (1993). Organic acids from source rock maturation: generation potentials, transport mechanisms and relevance for mineral diagenesis. Appl. Geochem. 8 (4), 325–337. doi:10.1016/0883-2927(93)90002-X
Berger, G., Velde, B., and Aigouy, T. (1999). Potassium sources and illitization in Texas Gulf Coast shale diagenesis. J. Sediment. Res. 69 (1), 151–157. doi:10.2110/jsr.69.151
Berner, R. A. (1984). Sedimentary pyrite formation: an update. Geochimica Cosmochimica Acta 48 (4), 605–615. doi:10.1016/0016-7037(84)90089-9
Blum, J. D., Sherman, L. S., and Johnson, M. W. (2014). Mercury isotopes in Earth and environmental sciences. Annu. Rev. Earth Planet. Sci. 42, 249–269. doi:10.1146/annurev-earth-050212-124107
Boszke, L., Kowalski, A., Glosifiska, G., Szarek, R., and Siepak, J. (2003). Environmental factors affecting speciation of mercury in the bottom sediments; an overview. Pol. J. Environ. Stud. 12, 5.
Bower, J., Savage, K. S., Weinman, B., Barnett, M. O., Hamilton, W. P., and Harper, W. F. (2008). Immobilization of mercury by pyrite (FeS2). Environ. Pollut. 156, 504–514. doi:10.1016/j.envpol.2008.01.011
Cossa, D., and Gobeil, C. (2000). Mercury speciation in the Lower st. Lawrence estuary. Can. J. Fish. Aquat. Sci. 57, 138–147. doi:10.1139/f99-237
Curtis, C. D. (1985). Clay mineral precipitation and transformation during burial diagenesis. Philosophical Trans. R. Soc. Lond. Ser. A. J. Math. Phys. Sci. 315 (1531), 91–105. doi:10.1098/rsta.1985.0031
Farrah, H., and Pickering, W. F. (1978). The sorption of mercury species by clay minerals. Water Air Soil Pollut. 9 (1), 23–31. doi:10.1007/bf00185744
Feyte, S., Tessier, A., Gobeil, C., and Cossa, D. (2010). In situ adsorption of mercury, methylmercury and other elements by iron oxyhydroxides and organic matter in lake sediments. Appl. Geochem. 25 (7), 984–995. doi:10.1016/j.apgeochem.2010.04.005
Fitzgerald, W. F. (1995). Is mercury increasing in the atmosphere? The need for an atmospheric mercury network (AMNET). Water Air Soil Pollut. 80 (1), 245–254. doi:10.1007/BF01189674
Font, E., Adatte, T., Sial, A. N., de Lacerda, L. D., Keller, G., and Punekar, J. (2016). Mercury anomaly, Deccan volcanism, and the end-Cretaceous mass extinction. Geology 44 (2), 171–174. doi:10.1130/G37451.1
Froelich, P. M., Klinkhammer, G. P., Bender, M. L., Lurdtke, N. A., Heath, G. R., Cullen, D., et al. (1979). Early oxidation of organic matter in pelagic sediments of the eastern equatorial atlantic: suboxic diagenesis. Geochimica Cosmochimica Acta 43, 1075–1090. doi:10.1016/0016-7037(79)90095-4
Gagnon, C., Pelletier, E., and Mucci, A. (1997). Behaviour of anthropogenic mercury in coastal marine sediments. Mar. Chem. 59, 159–176. doi:10.1016/S0304-4203(97)00071-6
Gobeil, C., and Cossa, D. (1993). Mercury in sediments and sediment pore water in the Laurentian Trough. Can. J. Fish. Aquat. Sci. 50 (8), 1794–1800. doi:10.1139/f93-201
Grasby, S. E., Beauchamp, B., Bond, D. P., Wignall, P. B., and Sanei, H. (2016). Mercury anomalies associated with three extinction events (Capitanian crisis, latest Permian extinction and the Smithian/Spathian extinction) in NW Pangea. Geol. Mag. 153 (2), 285–297. doi:10.1017/S0016756815000436
Grasby, S. E., Them, T. R., Chen, Z., Yin, R., and Ardakani, O. H. (2019). Mercury as a proxy for volcanic emissions in the geologic record. Earth-Science Rev. 196, 102880. doi:10.1016/j.earscirev.2019.102880
Gustin, M. S. (2003). Are mercury emissions from geologic sources significant? A status report. Sci. Total Environ. 304 (1-3), 153–167. doi:10.1016/S0048-9697(02)00565-X
Holmes, C. D., Jacob, D. J., Mason, R. P., and Jaffe, D. A. (2009). Sources and deposition of reactive gaseous mercury in the marine atmosphere. Atmos. Environ. 43 (14), 2278–2285. doi:10.1016/j.atmosenv.2009.01.051
Kalvoda, J., Kumpan, T., Qie, W., Frýda, J., and Bábek, O. (2019). Mercury spikes at the devonian-carboniferous boundary in the eastern part of the rhenohercynian zone (central europe) and in the South China block. Palaeogeogr. Palaeoclimatol. Palaeoecol. 531, 109221. doi:10.1016/j.palaeo.2019.05.043
Kongchum, M., Hudnall, W. H., and Delaune, R. D. (2011). Relationship between sediment clay minerals and total mercury. J. Environ. Sci. Health Part A 46 (5), 534–539. doi:10.1080/10934529.2011.551745
Krupp, R. (1988). Physicochemical aspects of mercury metallogenesis. Chem. Geol. 69 (3-4), 345–356. doi:10.1016/0009-2541(88)90045-9
Lamborg, C. H., Fitzgerald, W. F., O’Donnell, J., and Torgersen, T. (2002). A non-steady-state compartmental model of global-scale mercury biogeochemistry with interhemispheric atmospheric gradients. Geochimica Cosmochimica Acta 66 (7), 1105–1118. doi:10.1016/S0016-7037(01)00841-9
Lin, C. J., and Pehkonen, S. O. (1999). The chemistry of atmospheric mercury: a review. Atmos. Environ. 33 (13), 2067–2079. doi:10.1016/S1352-2310(98)00387-2
Lindberg, S. A., and Stratton, W. J. (1998). Atmospheric mercury speciation: Concentrations and behavior of reactive gaseous mercury in ambient air. Environ. Sci. Technol. 32 (1), 49–57. doi:10.1021/es970546u
Loukola-Ruskeeniemi, K., Kantola, M., Halonen, T., Seppänen, K., Henttonen, P., Kallio, E., et al. (2003). Mercury-bearing black shales and human Hg intake in eastern Finland: impact and mechanisms. Env. Geol. 43 (3), 283–297. doi:10.1007/s00254-002-0625-9
Mason, R. P., Fitzgerald, W. F., and Morel, F. M. (1994). The biogeochemical cycling of elemental mercury: anthropogenic influences. Geochimica Cosmochimica Acta 58 (15), 3191–3198. doi:10.1016/0016-7037(94)90046-9
Mcneal, J. M., and Rose, A. W. (1974). The geochemistry of mercury in sedimentary rocks and soils in Pennsylvania. Geochimica Cosmochimica Acta 38 (12), 1759–1784. doi:10.1016/0016-7037(74)90160-4
Morel, F. M., Kraepiel, A. M., and Amyot, M. (1998). The chemical cycle and bioaccumulation of mercury. Annu. Rev. Ecol. Syst. 29, 543–566. doi:10.1146/annurev.ecolsys.29.1.543
Niessen, S., Foucher, D., Clarisse, O., Fischer, J. C., Mikac, N., Kwokal, Z., et al. (2003). Influence of sulphur cycle on mercury methylation in estuarine sediment (seine estuary, france). J. Phys. IV Fr. 107, 953–956. doi:10.1051/jp4:20030456
Obrist, D. (2007). Atmospheric mercury pollution due to losses of terrestrial carbon pools? Biogeochemistry 85 (2), 119–123. doi:10.1007/s10533-007-9108-0
Oliveri, E., Manta, D. S., Bonsignore, M., Cappello, S., Tranchida, G., Bagnato, E., et al. (2016). Mobility of mercury in contaminated marine sediments: biogeochemical pathways. Mar. Chem. 186, 1–10. doi:10.1016/j.marchem.2016.07.002
Passey, Q. R., Bohacs, K. M., Esch, W. L., Klimentidis, R., and Sinha, S. (2010). “From oil-prone source rock to gas-producing shale reservoir–geologic and petrophysical characterization of unconventional shale-gas reservoirs,” in International oil and gas conference and exhibition in China, Beijing, China, 8-10 June 2010. doi:10.2118/131350-MS
Percival, L. M. E., Witt, M. L. I., Mather, T. A., Hermoso, M., Jenkyns, H. C., Hesselbo, S. P., et al. (2015). Globally enhanced mercury deposition during the end-pliensbachian extinction and toarcian OAE: A link to the karoo–ferrar Large igneous province. Earth Planet. Sci. Lett. 428, 267–280. doi:10.1016/j.epsl.2015.06.064
Pirrone, N., Cinnirella, S., Feng, X., Finkelman, R. B., Friedli, H. R., Leaner, J., et al. (2010). Global mercury emissions to the atmosphere from anthropogenic and natural sources. Atmos. Chem. Phys. 10 (13), 5951–5964. doi:10.5194/acp-10-5951-2010
Pollastro, R. M. (1993). Considerations and applications of the illite/smectite geothermometer in hydrocarbon-bearing rocks of Miocene to Mississippian age. Clays Clay Minerals 41 (2), 119–133. doi:10.1346/CCMN.1993.0410202
Pugh, C. E., Hossner, L. R., and Dixon, J. B. (1981). Pyrite and marcasite surface area as influenced by morphology and particle diameter. Soil Sci. Soc. Am. J. 45 (5), 979–982. doi:10.2136/sssaj1981.03615995004500050033x
Rasmussen, P. E. (1994). Current methods of estimating atmospheric mercury fluxes in remote areas. Environ. Sci. Technol. 28 (13), 2233–2241. doi:10.1021/es00062a006
Ravichandran, M. (2004). Interactions between mercury and dissolved organic matter––a review. Chemosphere 55 (3), 319–331. doi:10.1016/j.chemosphere.2003.11.011
Sanei, H., Grasby, S. E., and Beauchamp, B. (2012). Latest Permian mercury anomalies. Geology 40 (1), 63–66. doi:10.1130/G32596.1
Schicker, A., Gier, S., Schieber, J., and Krois, P. (2021). Diagenesis of the malmian mikulov formation source rock, vienna basin: Focus on matrix and pores. Mar. Petroleum Geol. 129, 105082. doi:10.1016/j.marpetgeo.2021.105082
Schroeder, W. H., and Munthe, J. (1998). Atmospheric mercury—An overview. Atmos. Environ. 32 (5), 809–822. doi:10.1016/S1352-2310(97)00293-8
Seewald, J. S. (2003). Organic–inorganic interactions in petroleum-producing sedimentary basins. Nature 426 (6964), 327–333. doi:10.1038/nature02132
Selin, N. E. (2009). Global biogeochemical cycling of mercury: a review. Annu. Rev. Environ. Resour. 34 (1), 43–63. doi:10.1146/annurev.environ.051308.084314
Shen, J., Algeo, T. J., Planavsky, N. J., Yu, J., Feng, Q., Song, H., et al. (2019a). Mercury enrichments provide evidence of Early Triassic volcanism following the end-Permian mass extinction. Earth-Science Rev. 195, 191–212. doi:10.1016/j.earscirev.2019.05.010
Shen, J., Chen, J., Algeo, T. J., Yuan, S., Feng, Q., Yu, J., et al. (2019b). Evidence for a prolonged Permian–Triassic extinction interval from global marine mercury records. Nat. Commun. 10 (1), 1563–1569. doi:10.1038/s41467-019-09620-0
Shen, J., Algeo, T. J., Chen, J., Planavsky, N. J., Feng, Q., Yu, J., et al. (2019c). Mercury in marine Ordovician/Silurian boundary sections of South China is sulfide-hosted and non-volcanic in origin. Earth Planet. Sci. Lett. 511, 130–140. doi:10.1016/j.epsl.2019.01.028
Shen, J., Yu, J., Chen, J., Algeo, T. J., Xu, G., Feng, Q., et al. (2019d). Mercury evidence of intense volcanic effects on land during the Permian-Triassic transition. Geology 47 (12), 1117–1121. doi:10.1130/G46679.1
Shen, J., Feng, Q., Algeo, T. J., Liu, J., Zhou, C., Wei, W., et al. (2020). Sedimentary host phases of mercury (Hg) and implications for use of Hg as a volcanic proxy. Earth Planet. Sci. Lett. 543, 116333. doi:10.1016/j.epsl.2020.116333
Sial, A. N., Chen, J., Lacerda, L. D., Frei, R., Tewari, V. C., Pandit, M. K., et al. (2016). Mercury enrichment and Hg isotopes in Cretaceous–Paleogene boundary successions: Links to volcanism and palaeoenvironmental impacts. Cretac. Res. 66, 60–81. doi:10.1016/j.cretres.2016.05.006
Singer, A. (1984). The paleoclimatic interpretation of clay minerals in sediments—A review. Earth-Science Rev. 21 (4), 251–293. doi:10.1016/0012-8252(84)90055-2
Smit, J., Koeberl, C., Claeys, P., and Montanari, A. (2016). Mercury anomaly, Deccan volcanism, and the end-Cretaceous mass extinction:Comment. Geology 44 (3), 381. doi:10.1130/G37683C.1
Wilkin, R. T., and Barnes, H. L. (1997). Formation processes of framboidal pyrite. Geochimica Cosmochimica Acta 61 (2), 323–339. doi:10.1016/S0016-7037(96)00320-1
Wilkin, R. T., Barnes, H. L., and Brantley, S. L. (1996). The size distribution of framboidal pyrite in modern sediments: an indicator of redox conditions. Geochimica Cosmochimica Acta 60 (20), 3897–3912. doi:10.1016/0016-7037(96)00209-8
Keywords: mercury, diagenesis, smectite, illite, framboidal pyrite
Citation: Xu H, Ran B, Liu S, Sun T, Luo C, Li Y and Zhu Y (2023) A review of possible mechanisms for mercury migration in diagenesis: Clay to pyrite. Front. Earth Sci. 10:1011598. doi: 10.3389/feart.2022.1011598
Received: 04 August 2022; Accepted: 08 November 2022;
Published: 18 January 2023.
Edited by:
Jun Wang, Yunnan University, ChinaReviewed by:
Ramanathan Alagappan, Jawaharlal Nehru University, IndiaDawei Lv, Shandong University of Science and Technology, China
Copyright © 2023 Xu, Ran, Liu, Sun, Luo, Li and Zhu. This is an open-access article distributed under the terms of the Creative Commons Attribution License (CC BY). The use, distribution or reproduction in other forums is permitted, provided the original author(s) and the copyright owner(s) are credited and that the original publication in this journal is cited, in accordance with accepted academic practice. No use, distribution or reproduction is permitted which does not comply with these terms.
*Correspondence: Bo Ran, UmFuYm8wOEBjZHV0LmNu