- 1State Key Laboratory of Tibetan Plateau Earth System Science, Resources and Environment (LTPESRE), Institute of Tibetan Plateau Research, Chinese Academy of Sciences, Beijing, China
- 2University of Chinese Academy of Sciences, Beijing, China
- 3School of Environmental and Geographical Science, Shanghai Normal University, Shanghai, China
- 4Department of Geosciences, University of Tübingen, Tübingen, Germany
Late Cenozoic drying of the Asian inland has not only exerted a profound impact on the regional environment but also affected global climate as an important source of global atmospheric dust. Continuous and accurately dated sediment records from the Asian interior are pivotal to a better understanding of the evolutionary history of Asian inland drying and the associated driving mechanisms. In this study, we present a continuous record of climate change in the Asian interior spanning the past 7.3 Myr, reconstructed by the redox evolution of a paleolake in the western Qaidam Basin, NE Tibetan Plateau. The paleolake redox conditions are linked to the oxygen concentration of lake bottom water and lake level, and were revealed by the manganese (Mn) concentration in the carbonate fraction (leached by the diluted acetic acid) of the carbonate-rich lacustrine sediments retrieved from two drill-cores (SG-1 and SG-1b). The reconstructed regional climate in the western Qaidam Basin shows long-term fluctuations, consistent with the secular evolution of the coeval global climate, especially the sea surface temperature variation in the high latitude North Atlantic. Three transitions of the paleolake hydrochemical system occurred at 6.2, 5.3, and 2.6 Ma, with a short drying stage at 6.2–5.3 Ma and prolonged Quaternary drying since 2.6 Ma. We argue that drying of the Asia interior has been dominantly forced by global cooling, in particular, the high-latitude cooling of the Northern Hemisphere.
Introduction
As the largest temperate arid region, the Asian inland has attracted broad interest due to its strong impacts on regional and global climate change. The Asian inland drying is generally regarded as a coupling consequence of land-sea-atmosphere interaction, which could be dated back to the early Eocene (e.g., Li et al., 2018) and significantly enhanced since the late Oligocene-early Miocene (Guo et al., 2002; Sun et al., 2010a; Lu et al., 2019; Sun et al., 2020b). The driving mechanism appears to correspond closely with the uplift of the Tibetan Plateau (e.g., Manabe and Terpstra, 1974; Kutzbach et al., 1989; An et al., 2001; Guo et al., 2002), the retreat of the Para-Tethys Sea (Ramstein et al., 1997; Fluteau et al., 1999; Zhang et al., 2007; Meijer et al., 2019), and global cooling with Cenozoic development of bipolar glaciations and continental ice sheets (e.g., Ding et al., 2005; Dupont-Nivet et al., 2007; Sun et al., 2014; Li et al., 2018; Fang et al., 2019) or a combination of them (e.g., Guo et al., 2002; Lu et al., 2019). Especially during the Neogene-Quaternary, the regional drying and eolian dust activity have been greatly enhanced in the Asian interior (e.g., Rea et al., 1998; Guo et al., 2002; Sun and Liu, 2006; Kent-Corson et al., 2009; Sun et al., 2010a; Zhuang et al., 2011; Li et al., 2014; Guo et al., 2018; Jia et al., 2021). However, intensive tectonic activity since the late Oligocene-Early Miocene in the northern Tibetan Plateau and the Central Asian orogenic belt (Li et al., 2014; Lu et al., 2018; Wang et al., 2020; Yang et al., 2021 and reference therein) yields much coarser lithology with frequent changes in sediment facies in basin sequences, which hinders a better understanding of the full range of aridification and its driving force using the basin sediment records.
The Qaidam Basin, an inland arid area in the northeast Tibetan Plateau, is dominantly under the influence of the middle-latitude westerlies, with its southeast corner being impacted by the East Asian monsoons (Figure 1), thus providing the ideal area to reveal tectonic-climate linkages in terms of aridification of the Asian interior. The up to 10–15 km thick Cenozoic fluvial-lacustrine deposits in the basin contain crucial information on the evolution of the paleoenvironment and paleoclimate in the basin. Previous studies reconstructed the regional climate evolution using a variety of proxy records, e.g., pollen (Lu et al., 2010; Miao et al., 2011; Cai et al., 2012; Miao et al., 2016; Koutsodendris et al., 2019; Jia et al., 2021), biomarker (Zhuang et al., 2014; Zhuang et al., 2019; Sun et al., 2020c; Wu et al., 2019; Wu et al., 2021), clay minerals (Fang et al., 2016; Ye et al., 2016; Ye et al., 2018; Fang et al., 2019; Ye et al., 2020), and geochemical records (e.g., Zhuang et al., 2011; Yang et al., 2013a; Yang et al., 2013b; Yang et al., 2014; Yang et al., 2015; Yang et al., 2016; Song et al., 2017; Bao et al., 2019; Han et al., 2020; Song et al., 2020). However, the late-Miocene-Quaternary is a transition period of the Qaidam Basin from a dominant fresh-brackish lake environment in mid-Miocene to a widely spread salt lake and dry land in the Quaternary (e.g., Guo et al., 2018). Continuous, high-resolution and well-dated lake sediment records are pivotal to revealing the transformation of regional climates.
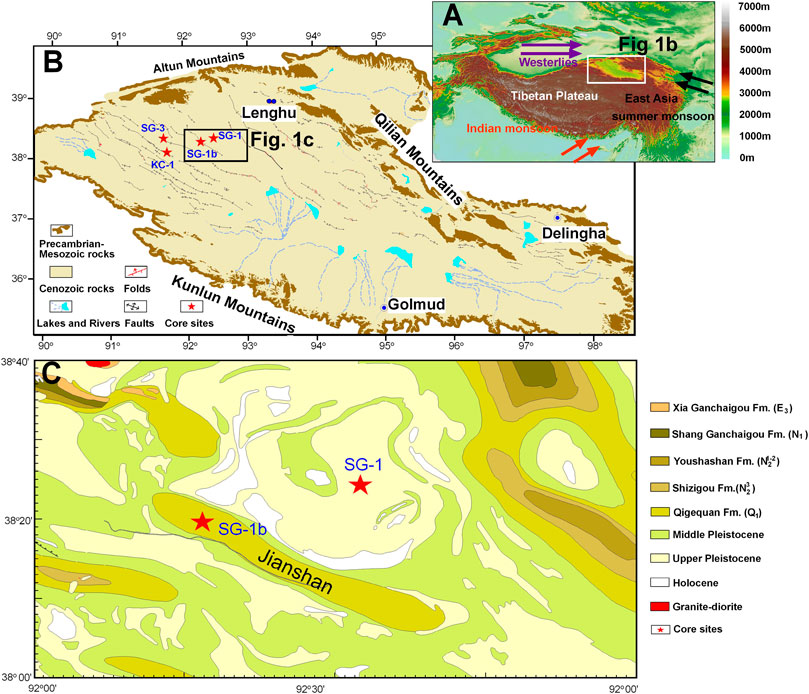
FIGURE 1. (A) Topographic map of the Tibetan Plateau and Central Asia indicating the Qaidam Basin area. (B) Map of the Qaidam Basin and adjacent region showing the surrounding mountains, major structures, and the study area (modified from Zhang et al., 2012a). (C) Geologic map of the study area in the western Qaidam Basin showing the locations of the SG-1b and SG-1 drill-sites (red asterisks) (modified from Zhang et al., 2012b).
We present a long-term hydrochemical record of the western Qaidam paleolake covering the past ∼7.3 Myr, based on analysis on two accurately dated drill-cores (SG-1 and SG-1b). Dissolved manganese (Mn) concentration in lake water reacts strongly to changes in redox conditions of lake bottom water and the lake level fluctuations in a closed basin (Yang et al., 2013a). Dissolved Mn in lake water can be incorporated in the authigenic carbonate minerals, thus leaving the hydrochemical imprint into the past sediment archives. Previous studies have revealed that Mn in carbonates (diluted acetic acid dissolved fraction) of SG-1 lake sediments (2.8–0.1 Ma) in the western Qaidam Basin is a sensitive indicator of paleolake evolution and regional climate (Yang et al., 2013a). We thus integrated new data of carbonate Mn concentration from the SG-1b core (7.3–1.6 Ma) with the previous SG-1 Mn records to reconstruct a continuous history of paleolake and climate evolution in the western Qaidam Basin since 7.3 Ma.
Materials and Methods
The Qaidam Basin, with an average elevation of 3000 m, forms the largest inland basin on the northeastern Tibetan Plateau. The basin is surrounded by the East Kunlun Shan to the south, the Qilian Shan to the northeast, and the Altyn Mountain to the northwest (Figure 1). The closed basin contains more than 10,000 m thick Cenozoic fluvial and lacustrine deposits derived from the surrounding mountains (Xia et al., 2001). The western Qaidam Basin has a hyper-arid environment, with mean annual precipitation less than 100 mm and potential evaporation greater than 2000 mm, and the surface is covered with a strongly indurated decimeter-thick salt crust.
The SG-1b drilling campaign was performed at the top of the Jianshan Anticline in the western Qaidam Basin, 100 km northeast of Lenghu Town, and about 20 km east of the SG-1 borehole (Zhang et al., 2014a, Figure 1). The drilling extended 723m in depth with an average recovery rate of 93%. The sedimentary sequence is mainly featured by deep-water, fine-grained lacustrine sediments comprising clay, clay-silt, siltstone, and calcareous mudstone (Zhang et al., 2014b). From 723 to 232 m, the sediments are characterized by well-bedded grey and/or blue-grey clay or mudstone. The sediments in the upper 232 m are characterized by grey (or dark grey) and blue-grey clay, clay-silt, and siltstone, with small amounts of gypsum crystals or thin gypsum layers (Figure 2, Zhang et al., 2014a; Lu et al., 2015). The SG-1b drill-core was dated at about 7.3–1.6 Ma with magnetostratigraphy (Zhang et al., 2014b), refined by orbital tuning in the period of 3.3–2.1 Ma (Kaboth-Bahr et al., 2020). The sediment accumulation rate in the SG-1 core is almost three times higher than in the SG-1b core (Figure 2), probably because the SG-1 drill-site is located close to the depocenter while the SG-1b core was recovered on an anticline (Zhang et al., 2014a).
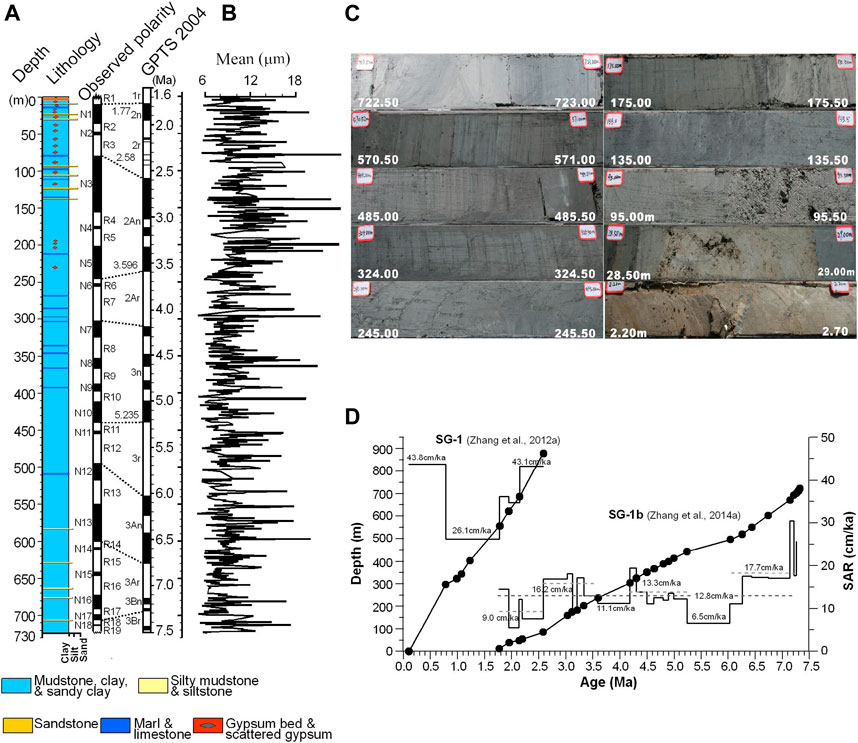
FIGURE 2. (A) SG-1b core lithology and magnetostratigraphy (after Zhang et al., 2014b). (B) Variations in mean grain size fractions in the SG-1b (derived from Lu et al., 2015). (C) Photographs of selected sliced cores between 723 m and 0 m (∼7.3–1.6 Ma). (D) Depth–age plot for the cores SG-1 (Zhang et al., 2012b) and SG-1b (Zhang et al., 2014a) based on the correlation of the polarity sequence with the geomagnetic polarity timescale of Gradstein et al. (2004).
Leaching experiments of loess suggest that 1M acetic acid (HOAc) mainly dissolves the Mn (II) of carbonate rather than manganese oxide (Liu et al., 2002; Liang et al., 2009). Meanwhile, leaching experiments of soil and loess show that the dissolution of clay by 1M HOAc is very weak (Yang et al., 2000; Liu et al., 2002). We thus used the 1M HOAc to leach the sediments in order to obtain the Mn(II) concentrations in the lacustrine sediments. We didn’t use a two-step procedure (water and HOAc leaching) like in the previous study of the SG-1 core (Yang et al., 2013a); instead, we used 1M HOAc leaching to directly react with bulk sediment. Therefore, the carbonate fraction and water-soluble salts are both incorporated in the HOAc leachates of the sediments. One reason for the modified leaching procedure is that only a small amount of gypsum occurred in the upper part of the SG-1b core, while evaporite minerals are abundant in the SG-1 core. Another reason is that multiple leaching experiments showed that a negligible amount of Mn exists in the water-soluble salts of the lacustrine sediments (Yang et al., 2016). Accordingly, diluted acetic acid leaching in our study can extract Mn only from carbonates, which could be well compared with the Mn concentration in the SG-1 core (Yang et al., 2013a).
A total of 619 bulk samples of the SG-1b core were selected for geochemical analysis. Samples were oven-dried at 40°C and ground into fine powder (<200 mesh). Approximately 0.5 g of each sample was leached by 10 ml 1M HOAc at room temperature for 24 h to extract Mn in the carbonate fraction of the samples. Concentrations of Mn, Ca, Mg, and Sr in the acetic acid leachate were analyzed by inductively coupled plasma-optical emission spectrometry (ICP-OES) (Leeman Labs Prodigy-H). Replicate analyses showed a relative standard deviation for all cations of less than 2%. All of the above treatments and measurements were conducted at the Institute of Tibetan Plateau Research, Chinese Academy of Sciences (ITP-CAS), Beijing. To obtain the major minerals (especially carbonate minerals in the core), we chose seven representative samples of SG-1b core for X-ray diffraction (XRD) analysis using a Rigaku D/MAX-2000 diffractometer (Cu, Kα, 1.5406 Å, 40 kV, 100 mA, 3–35°, step 0.02°, 10°/min) at the Micro Structure Analytical Laboratory, Peking University.
Results
The XRD results show that major minerals in the sediments of SG-1b core include quartz, albite, orthoclase, carbonate minerals (calcite, aragonite, dolomite, and ankerite), mica or illite, and chlorite (Figure 3). There is a minor amount of evaporite minerals, e.g., halite, gypsum, and celestite in the core except for abundant gypsum and halite observed in sample 1.1 m at the top of the SG-1b core.
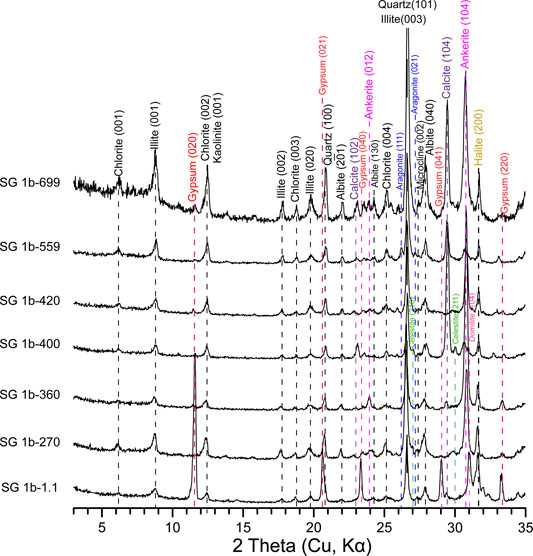
FIGURE 3. X-ray diffraction patterns of sedimentary minerals from representative samples of the SG-1b core. Note that mica minerals and illite are marked as illite.
Figure 4 shows the results of Mn, Ca, Mg, and Sr concentrations from the HOAc leaching of the SG-1b core sediments (data can be found in Supplementary Material). The concentration of Ca in acetic acid leachates (CaHOAc) mainly represents the calcium carbonate and some Ca-bearing salt minerals (e.g., gypsum) content in the sediments. The small amount of gypsum that only occurs in the upper 232 m suggests that a small amount of Ca is likely from the calcium sulfate. Consequently, the measured Ca concentrations reflect the calcium carbonate content below 232 m and an upper limit of the calcium carbonate content above 232 m. The average CaHOAc is around 10.43%, with slight fluctuations throughout the drill-core, yielding a rough estimate of 25% CaCO3 in the SG-1b core sediments. CaHOAC shows no long-term trend and remains at a relatively high level. The Mg/Ca and Sr/Ca ratios generally remain at ∼0.08 and 0.0085, respectively, and only fluctuate in some layers (Figure 4). MnHOAc values range from 40.7 μg/g to 1315.6 μg/g, with an average of 366.8 μg/g. The MnHOAc variation reveals an upward decrease above ∼240 m and a long-term relative stable content with several fluctuations below ∼240 m (Figure 4). The Mn/Ca ratios present a likewise variation as the Mn content along the whole core.
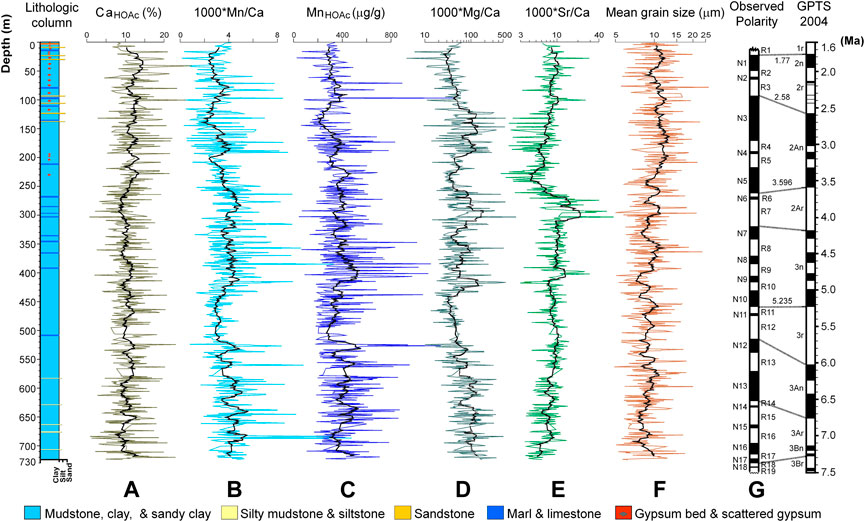
FIGURE 4. Depth profiles of Mn, Ca, Mg, and Sr variations of acetic acid leachate (HOAc) in the SG-1b core. (A) Ca concentrations in the HOAc leachate (CaHOAc); (B) The ratio of 1000*Mn/Ca in the HOAc leachate; (C) Mn concentrations in the HOAc leachate (MnHOAc); (D) The ratio of Mg/Ca in the HOAc leachate; (E) The ratio of 1000*Sr/Ca in the HOAc leachate; (F) Mean grain size from Lu et al. (2015); (G) Observed magnetic polarity sequence of the SG-1b core and its correlation with the Geomagnetic Polarity Time Scale (GPTS, Gradstein et al., 2004) (Zhang et al., 2014a). The lithology column is simplified according to Zhang et al. (2014b). Thin lines indicate the raw data for (A–F). Bold solid lines in the records provide 13-point running averages for (A–F).
Discussion
Mn in Carbonate as a Redox Proxy
Manganese is a metallic element reacting sensitively to changes in reduction-oxidation (redox) conditions. Redox conditions of bottom water in a closed lake are generally associated with the lake level. In arid regions, the lake level in a closed catchment is highly sensitive to changes in rainfall (Narisma et al., 2007). Numerous studies have studied the redox behaviour of Mn in lakes (e.g., Dean et al., 1981; Davison, 1993; Hamilton-Taylor and Davison, 1995; Wetzel, 2001). In brief, Mn (II) is soluble in reduced phases while Mn (IV) is insoluble in oxidized phases, both of which are the main valence states of manganese in lake environment and readily converted into each other in the vicinity of a redox boundary (Davison, 1993; Wetzel, 2001). However, Mn (IV) is easily reduced but Mn (II) is not readily oxidized, which can lead to a large field of stability for dissolved Mn (II) (Maynard, 2004). Moreover, Mn sulfide is very soluble in reducing environment (Algeo and Maynard, 2004), and dissolved Mn is not readily taken up by any organic or mineral phase (Huerta-Diaz and Morse, 1992). The above Mn properties result in a diffuse and homogeneous distribution of Mn (II) throughout the lake water body (Hamilton-Taylor and Davison, 1995), thus providing an ideal tool to reflect the lake hydrochemistry in a broad area. The Mn (II) can be sequestered by carbonate formation from low Eh to slightly oxic conditions (Calvert and Pedersen, 1993; Hild and Brumsack, 1998; Caplan and Bustin, 1999; Stevens et al., 2000; Maynard, 2004; Tribovillard et al., 2006). Hence, Mn concentration in carbonate is sensitive to lake-water redox conditions linked to the oxygen content of the bottom water and water depth (e.g., Schaller and Wehrli, 1997; Stevens et al., 2000). Mn concentration in carbonate has successfully been used in the SG-1 core to address the lake redox conditions and regional climate at long-term and glacial-interglacial scales (Yang et al., 2013a; Yang et al., 2016).
Controlling Factors
Provenance change along with detrital Mn input unlikely controls MnHOAc variations. Mn-rich bedrocks, such as rhodochrosite- or pyrolusite-rich rocks, are not found in the surrounding mountains of the western Qaidam Basin (RGMRGD, 1985). Exposed rocks in the catchment area are variable, with many intermediate and acid rocks. These are mainly grey gneiss, siliciclastic rocks, dolostone, quartzite, phyllite, marble, carbonatite, peridotite, serpentinite, augite, diorite, and granite of Precambrian to Early Cretaceous ages (Zhang, 1987; Wang et al., 2008). Carbonate analyses of the nearby Lenghu and Ganchaigou lacustrine sediments show that the average proportions of detrital carbonates to total carbonates are 11 and 13%, respectively (Hanson, 1999; Graham et al., 2005). Therefore, any Mn signal inherited from detrital carbonates should be relatively small. The lack of Mn-rich rocks in the catchment suggests that the provenance change that potentially follows regional uplift in the western Qaidam Basin cannot exert a dominant control on Mn concentration in lake water and carbonates, although anticline development following the uplift since 3.6 Ma could alter the clastic sedimentation (Lu et al., 2015).
Diagenesis of carbonate minerals can significantly alter Mn concentrations in carbonates during recrystallization. The Mn partitioning coefficients for sedimentary carbonate minerals are larger than 1, and Mn is thus preferentially incorporated into crystals rather than solutions (Brand and Veizer, 1980; Rimstidt et al., 1998). Lithological investigation of the SG-1 core (Wang et al., 2012) and the SG-1b core (Lu et al., 2015; Lu et al., 2021) didn’t show an obvious diagenetic imprint for carbonate minerals. Carbon and oxygen isotopic results from sedimentary carbonates in the SG-1 core (Han et al., 2014) and in Neogene lacustrine strata from adjacent areas, such as Lenghu and Laomangnai (Kent-Corson et al., 2009), demonstrate that the carbonate minerals are only diagenetically altered to a minor degree if any.
The high contents of carbonate minerals in the SG-1b core suggest that, similar to the SG-1 core (Yang et al., 2013a), the paleolake water was saturated with calcium carbonate. It means that sufficient bicarbonate existed in the paleolake during which Mn concentrations in carbonate phases will be in proportion with dissolved Mn in lake water, thus not relying on the amount of carbonate. It can be supported by the similar changes between Mn/Ca ratio and Mn concentrations (Figure 4). Furthermore, despite the location difference of the two cores within the basin sedimentation system (Zhang et al., 2014b), the well-matched MnHOAc variations and concentrations in the overlapped duration (2.8–1.6 Ma) of the two cores also confirm a homogeneous dissolved Mn distribution throughout the lake water body. Based on these observations, MnHOAc in the SG-1b core (7.3–1.6 Ma, this study) and in the SG-1 core (2.8–0.1 Yang et al., 2013a) could be integrated to reconstruct the evolution of dissolved Mn concentrations in the paleolake of the western Qaidam paleolake since 7.3 Ma (Figure 5).
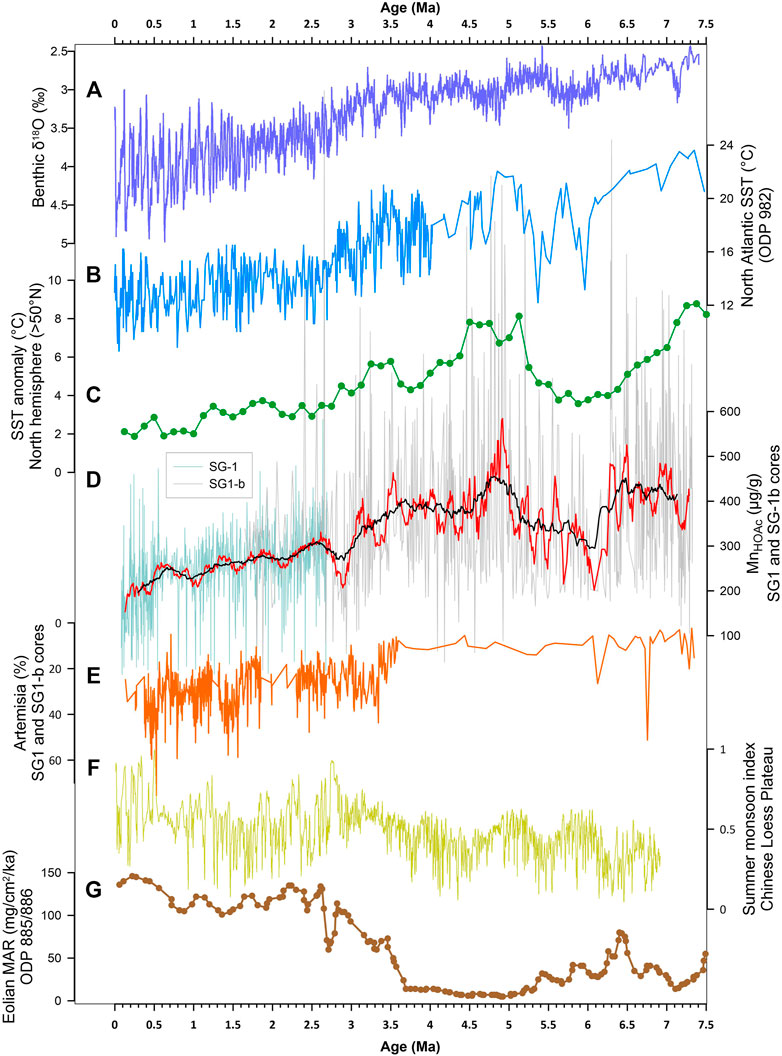
FIGURE 5. Integrated MnHOAc record of the SG-1b and SG-1 drill-cores since 7.3 Ma in the Qaidam Basin compared with other records. (A) Global marine benthic foraminiferal δ18O record (‰) (Zachos et al., 2001). (B) Alkenone-derived sea surface temperature (SST) record from the North Atlantic Ocean at ODP Site 982 (Herbert et al., 2016). (C) Stacked high latitude SST anomalies in North Hemisphere (Herbert et al., 2016). (D) MnHOAc record over the past 7.3 Myr (raw data, grey lines for the SG-1b core and light blue-green lines for the SG-1 core; red and black lines are 100- and 400-kyr smoothing of the raw data, respectively). (E) Artemisia content of the SG-1b and SG-1 cores (Koutsodendris et al., 2019). (F) Summer monsoon index in the Chinese Loess Plateau (Sun et al., 2010b). (G) Mass accumulation rate (MAR) of eolian dust at Site 885/886 in the northern Pacific Ocean (Rea et al., 1998).
Dissolved Mn concentration in the paleolake is mainly supplied by weathering of Mn-bearing minerals from the source area and further modulated by the lake bottom water redox conditions. To be more specific, as Yang et al. (2013a) proposed, the high MnHOAc concentration in the integrated record represents an increased input of dissolved Mn and Mn oxide-hydroxide from the source area with an intense reducing capacity that transformed Mn oxide-hydroxide to dissolved Mn(II). This scenario reflects a high lake level, probably with a stable thermocline and oxygen-deficient bottom water in a warm and humid climate. Conversely, the low MnHOAc concentration represents a decreased input of dissolved Mn and Mn oxide-hydroxide from the source area with a weakened reducing capacity that transformed Mn oxide-hydroxide to dissolved Mn(II). This scenario thus indicates a low lake level together with a less developed thermocline, probably well mixed and oxygen-deficient bottom water in a cold and dry climate. In this sense, the MnHOAc concentrations present long-term fluctuations of regional climate.
Driving Mechanism
Comparisons of the integrated MnHOAc record from the SG-1b core (this study) and SG-1 core (Yang et al., 2013a) with the benthic δ18O record (Zachos et al., 2001) and alkenone sea-surface temperatures (SST) of the North Atlantic Ocean (Herbert et al., 2016) show that the variation of MnHOAc is in general agreement with the global change and the climate of the high-latitude Northern Hemisphere (Figure 5). This becomes evident from the scatter plot of the MnHOAc record with benthic δ18O and OPD 982 Site SST (Figure 6). The integrated MnHOAc record is especially more synchronous with the North Atlantic Ocean SST (Figure 5), suggesting that the reduction of westerlies moisture caused by high-latitude cooling of the Northern Hemisphere directly affects the aridification of the Qaidam Basin. In addition, cooling in the Northern Hemisphere could also lead to cooler and drier conditions in the Qaidam Basin through enhancement of the Siberian High (Porter and An, 1995; Fang et al., 1999). A cooler and drier climate would reduce catchment Mn input from the catchment and elevate the oxygen content in lake bottom water, thus collectively leading to a decline in contents of dissolved Mn in lake water and MnHOAc in carbonate. However, it should be noted here that cooling since the late Miocene occurred synchronously in both hemispheres (Herbert et al., 2016). Given the close relationship among the high-latitude SST (especially in the North Atlantic), the formation of deep water, and the deep water δ18O (e.g., Zachos et al., 2001; Lozier, 2010), we here don’t distinguish the high latitude cooling in the North Hemisphere from a global context.
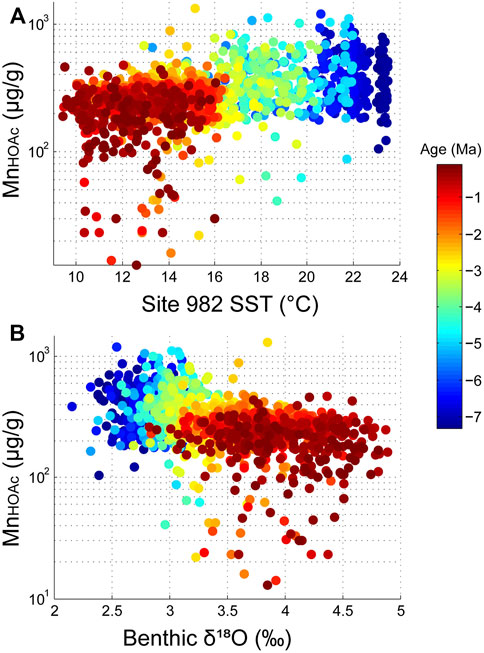
FIGURE 6. Cross-plots of the MnHOAc content in the SG-1 and SG-1b cores with the SST in ODP Site 982 (A) and benthic δ18O (B) since 7.3 Ma (ages are indicated by colour code). Power fits (LnY=BX + A) of MnHOAc versus SST and δ18O, yield correlation coefficients of 0.707 for (A) and −0.707 for (B), respectively (p < 0.0001). SST and δ18O data were interpolated at the same resolution as MnHOAc.
In order to detect possible nonlinear dynamical transitions in the regional climate record in the Qaidam Basin and to facilitate an evaluation of the underlying regulating driving mechanism(s), we performed a recurrence analysis (Marwan et al., 2007) of the MnHOAc time series (Figure 7). The recurrence plot is a binary plot, in which periodic processes are expressed as areas with higher-density points (i.e., longer lines and less isolated recurrence points), while chaotic/stochastic climate fluctuations are expressed by only isolated recurrence points or very short lines. Transition/shifts between different states can capture key transitions of system regime. The recurrence analysis has been successfully used to determine the climate state transitions using high-resolution proxy records, e.g., the Cenozoic climate (Westerhold et al., 2020) and the Quaternary climate in the Qaidam Basin (Han et al., 2020). The result of recurrence analysis of the integrated MnHOAc record shows three major transitions at 6.2 Ma, 5.3 Ma, and 2.6 Ma. Compared with the dry/wet cycles as suggested by the MnHOAc record (Figure 7), the 6.2 Ma marks the initiation of a short drying stage between 6.2–5.3 Ma, which accords with the concomitant rise in benthic δ18O and decline in SST in North Atlantic (Figures 5A,B). The 5.3 Ma change, i.e., at about the Miocene/Pliocene boundary, is a transition stage from the prior drying stage to the subsequent wetting stage in the Qaidam Basin, which is consistent with global warming at that time (Figure 5). The system change at the Pliocene/Quaternary boundary (2.6 Ma) marks the onset of prolonged and extensive Quaternary drying in the Qaidam Basin, which is contemporaneous with the intensification of the North Hemisphere glaciation. The three major transitions determined by the recurrence analysis thus indicate the major dry/wet changes in the Asian interior. All these transitions well match the global climate and the high latitude climate of the North Hemisphere, implying that global change modulated the Asian inland climate since 7.3 Ma at long-term scales.
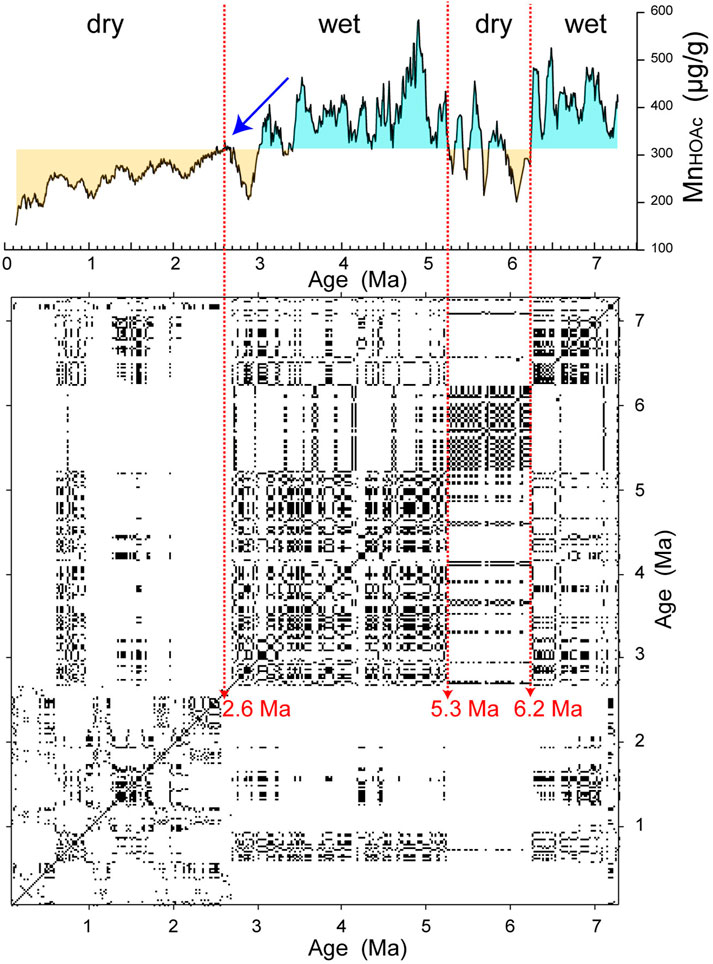
FIGURE 7. Plots of MnHOAc 100-kyr sliding window means (top) and recurrence analysis of MnHOAc variation (bottom) for the integrated SG-1 and SG-1b data, showing major climate transitions during the past 7.3 Myr. Red arrows show the three major transitions at around 6.2 Ma, 5.3 Ma and 2.6 Ma, which correspond to the major boundaries between dry and wet stages as suggested by the MnHOAc data in the upper panel. The blue arrow marks the beginning of the Pliocene drying since ∼3.6 Ma.
Further spectral analysis of the MnHOAc concentrations shows significant periodicities at 23, 41, 100, and 405 kyr (Figure 8), which are consistent with the orbital cycles in benthic δ18O record (Zachos et al., 2001) and in OPD 982 Site SST (Lawrence et al., 2009). In particular, the enhanced eccentricity band at 405 and 100 kyr during the Quaternary compared with the dominant obliquity band before 2.7 Ma (Figure 8) confirms an orbital change at the Mid-Pleistocene Transition (MPT, 1.2-0.8 Ma) from ∼40 to ∼100 kyr (e.g., Clark et al., 2006). Therefore, global change and northern hemisphere high latitude processes could modulate the Asian inland climate at orbital time scales, which is also supported with other studies of high-resolution Quaternary records in the SG-1 core (e.g., Han et al., 2020).
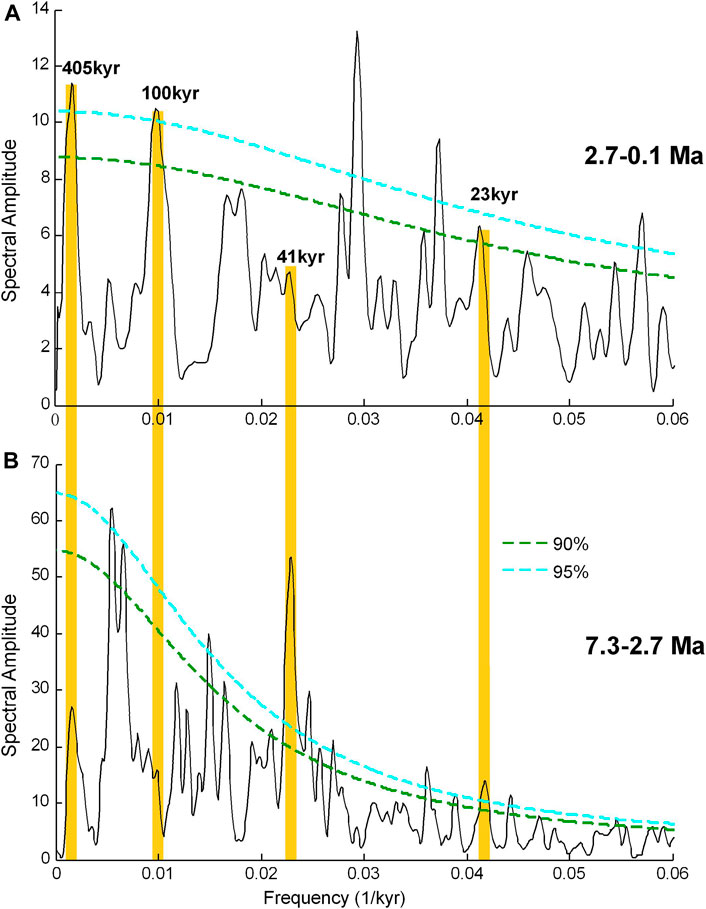
FIGURE 8. Spectral analysis of the carbonate Mn record after 2.7 Ma (A) and before 2.7 Ma (B). Green and blue dashed lines are 90 and 95% significance levels, respectively.
The MnHOAc results also record a dramatic environmental turnover from ∼3.6 to 2.6 Ma, during which the regional climate shifted from the dominant wetting stage to the prolonged Quaternary cooling (Figure 5). This climate turnover since 3.6 Ma could be witnessed by the rise in the Artemisia content of the pollen record (Koutsodendris et al., 2019) (Figure 5E) and the rise in eolian accumulation rate in the North Pacific (Rea et al., 1998). This 3.6 Ma-event is a widely observed drying event in East and Central Asia (Ge et al., 2013), but corresponds to an East Asian monsoon enhancement as shown by the summer monsoon index in the Chinese Loess Plateau (Figure 5F, Sun et al., 2010b; Nie et al., 2014; Ye et al., 2018). This Asian inland drying coupled with East Asian wetting is a typical environmental impact of the uplift of the northern Tibetan Plateau (e.g., An et al., 2001; Zhang R. et al., 2012; Tada et al., 2016). There is abundant observational evidence of the tectonic movements in the northern Tibetan Plateau at ∼3.6 Ma. The anticline development since 3.6 Ma was also suggested by the grain-size records of the SG-1b core (Lu et al., 2015). During 3.6–2.6 Ma, huge conglomerate layers widely formed in the basins at the northeastern edge of the Tibetan Plateau, such as the Qaidam basin (Fang et al., 2003), Linxia basin (Fang et al., 2003), Guide basin (Fang et al., 2005a), Jiuxi basin (Fang et al., 2005b). Hence, the rapid drying at ∼3.6 Ma in this study is probably caused by the Tibetan Plateau uplift superimposed on the global cooling.
In addition, the westward retreat of the Para-Tethys Sea seems unlikely to affect the regional drying in the Qaidam Basin since 7.3 Ma, although it is thought to have caused the Asian interior aridification from the middle Eocene to the Eocene-Oligocene transition at ∼34–33 Ma based on evidence from the Xining, Tajik, and Tarim Basins (Bosboom et al., 2014; Carrapa et al., 2015; Sun et al., 2016; Kaya et al., 2019; Meijer et al., 2019; Sun et al., 2020a) and the modelling work (see Ramstein et al., 1997; Zhang et al., 2007). The Para-Tethys and the Neo-Tethys seas before the late-Miocene has retreated to a place (e.g., Zhang et al., 2014a; Sun et al., 2021) that cannot have a significant impact on the Qaidam Basin. In particular, the moisture barrier of the mid-latitude westerlies has established before the late-Miocene, for example, the Pamir uplift (e.g., Blayney et al., 2019; Wang et al., 2020).
Conclusion
The integrated MnHOAc records since 7.3 Ma from the two deep drilling cores (SG-1b and SG-1) in the Qaidam Basin revealed the evolution of Qaidam paleolake and regional paleoenvironmental change during the late Cenozoic. The region climate exhibited long-term fluctuations, similar to the global change, especially the North Atlantic high latitude sea surface temperature. Three transitions of the paleolake hydrochemical system occurred at 6.2, 5.3, and 2.6 Ma, which deliminate a short drying stage at 6.2–5.3 Ma and a prolonged Quaternary drying stage since 2.6 Ma. This Quaternary drying may be related to the enhanced aridification of the Asian interior since 3.6 Ma. We argue that the drying history has been caused by global cooling, in particular, the high-latitude cooling of the Northern Hemisphere with some contributions from the growth of the northern Tibetan Plateau at 3.6 Ma.
Data Availability Statement
The original contributions presented in the study are included in the article/Supplementary Material, further inquiries can be directed to the corresponding author.
Author Contributions
YY, XF, and EA designed the research. YY, RY, and CY performed the analyses. YY and YL interpreted the data, and YL and YY prepared the manuscript with contributions from all co-authors.
Funding
This work was co-supported by the National Natural Science Foundation of China (Grant Nos 41771236, 41620104002, and 42071111), the Strategic Priority Research Program of the Chinese Academy of Sciences (Grant No. XDA20070201) and the Basic Science Center for Tibetan Plateau Earth System (CTPES, 41988101-01), the Second Tibetan Plateau Scientific Expedition and Research (STEP) program (Grant No. 2019QZKK0707), YY is supported by the Youth Innovation Promotion Association (2018095) of the Chinese Academy of Sciences.
Conflict of Interest
The authors declare that the research was conducted in the absence of any commercial or financial relationships that could be construed as a potential conflict of interest.
Publisher’s Note
All claims expressed in this article are solely those of the authors and do not necessarily represent those of their affiliated organizations, or those of the publisher, the editors and the reviewers. Any product that may be evaluated in this article, or claim that may be made by its manufacturer, is not guaranteed or endorsed by the publisher.
Acknowledgments
We appreciate Wenxia Han for providing the cross-correlation, the recurrence and spectral analyses of the data. We thank Xiaohua Teng and Xiaoming Liu for sample analyses.
Supplementary Material
The Supplementary Material for this article can be found online at: https://www.frontiersin.org/articles/10.3389/feart.2021.813727/full#supplementary-material
References
Algeo, T. J., and Maynard, J. B. (2004). Trace-element Behavior and Redox Facies in Core Shales of Upper Pennsylvanian Kansas-type Cyclothems. Chem. Geology. 206, 289–318. doi:10.1016/j.chemgeo.2003.12.009
An, Z. S., Kutzbach, J. E., and Prell, S. C. (2001). Evolution of Asian Monsoons and Phased Uplift of the Himalaya-Tibetan Plateau since Late Miocene Times. Nature 411, 62–66. doi:10.1038/35075035
Bao, J., Song, C., Yang, Y., Fang, X., Meng, Q., Feng, Y., et al. (2019). Reduced Chemical Weathering Intensity in the Qaidam Basin (NE Tibetan Plateau) during the Late Cenozoic. J. Asian Earth Sci. 170, 155–165. doi:10.1016/j.jseaes.2018.10.018
Blayney, T., Dupont‐Nivet, G., Najman, Y., Proust, J. N., Meijer, N., Roperch, P., et al. (2019). Tectonic Evolution of the Pamir Recorded in the Western Tarim Basin (China): Sedimentologic and Magnetostratigraphic Analyses of the Aertashi Section. Tectonics 38 (2), 492–515. doi:10.1029/2018TC005146
Brand, U., and Veizer, J. (1980). Chemical Diagenesis of a Multicomponent Carbonate System-1: Trace Elements. J. Sedimen. Res. 50, 1219–1236. doi:10.1306/212F7BB7-2B24-11D7-8648000102C1865D
Bosboom, R. E., Abels, H. A., Hoorn, C., van den Berg, B. C. J., Guo, Z., and Dupont-Nivet, G. (2014). Aridification in continental Asia after the Middle Eocene Climatic Optimum (MECO). Earth Planet. Sci. Lett. 389, 34–42. doi:10.1016/j.epsl.2013.12.014
Cai, M., Fang, X., Wu, F., Miao, Y., and Appel, E. (2012). Pliocene-Pleistocene Stepwise Drying of Central Asia: Evidence from Paleomagnetism and Sporopollen Record of the Deep Borehole SG-3 in the Western Qaidam Basin, NE Tibetan Plateau. Glob. Planet. Change 94-95, 72–81. doi:10.1016/j.gloplacha.2012.07.002
Calvert, S. E., and Pedersen, T. F. (1993). Geochemistry of Recent Oxic and Anoxic marine Sediments: Implications for the Geological Record. Mar. Geology. 113, 67–88. doi:10.1016/0025-3227(93)90150-T
Caplan, M. L., and Bustin, R. M. (1999). Devonian-Carboniferous Hangenberg Mass Extinction Event, Widespread Organic-Rich Mudrock and Anoxia: Causes and Consequences. Palaeogeogr. Palaeoclimatol. Palaeoecol. 148, 187–207. doi:10.1016/S0031-0182(98)00218-1
Carrapa, B., DeCelles, P. G., Wang, X., Clementz, M. T., Mancin, N., Stoica, M., et al. (2015). Tectono-climatic Implications of Eocene Paratethys Regression in the Tajik basin of central Asia. Earth Planet. Sci. Lett. 424, 168–178. doi:10.1016/j.epsl.2015.05.034
Clark, P. U., Archer, D., Pollard, D., Blum, J. D., Rial, J. A., Brovkin, V., et al. (2006). The Middle Pleistocene Transition: Characteristics, Mechanisms, and Implications for Long-Term Changes in Atmospheric pCO2. Quat. Sci. Rev. 25 (23-24), 3150–3184. doi:10.1016/j.quascirev.2006.07.008
Davison, W. (1993). Iron and Manganese in Lakes. Earth-Science Rev. 34, 119–163. doi:10.1016/0012-8252(93)90029-7
Dean, W. E., Moore, W. S., and Nealson, K. H. (1981). Manganese Cycles and the Origin of Manganese Nodules, Oneida Lake, New York, U.S.A. Chem. Geology. 34, 53–64. doi:10.1016/0009-2541(81)90071-1
Ding, Z. L., Derbyshire, E., Yang, S. L., Sun, J. M., and Liu, T. S. (2005). Stepwise Expansion of Desert Environment across Northern China in the Past 3.5 Ma and Implications for Monsoon Evolution. Earth Planet. Sci. Lett. 237, 45–55. doi:10.1016/j.epsl.2005.06.036
Dupont-Nivet, G., Krijgsman, W., Langereis, C. G., Abels, H. A., Dai, S., and Fang, X. (2007). Tibetan Plateau Aridification Linked to Global Cooling at the Eocene-Oligocene Transition. Nature 445 (7128), 635–638. doi:10.1038/nature05516
Fang, X., Galy, A., Yang, Y., Zhang, W., Ye, C., and Song, C. (2019). Paleogene Global Cooling-Induced Temperature Feedback on Chemical Weathering, as Recorded in the Northern Tibetan Plateau. Geology 47 (10), 992–996. doi:10.1130/G46422.1
Fang, X., Garzione, C., Van der Voo, R., Li, J., and Fan, M. (2003). Flexural Subsidence by 29 Ma on the NE Edge of Tibet from the Magnetostratigraphy of Linxia basin, China. Earth Planet. Sci. Lett. 210 (3-4), 545–560. doi:10.1016/s0012-821x(03)00142-0
Fang, X., Li, M., Wang, Z., Wang, J., Li, J., Liu, X., et al. (2016). Oscillation of mineral Compositions in Core SG-1b, Western Qaidam Basin, NE Tibetan Plateau. Sci. Rep. 6 (1), 1–7. doi:10.1038/srep32848
Fang, X. M., Li, J. J., and Van der Voo, R. (1999). Rock Magnetic and Grain Size Evidence for Intensified Asian Atmospheric Circulation since 800,000 Yrs B.P. Related to Tibetan Uplift. Earth Planet. Sci. Lett. 165, 129e144. doi:10.1016/S0012-821X(98)00259-3
Fang, X., Yan, M., Van der Voo, R., Rea, D. K., Song, C., Parés, J. M., et al. (2005a). Late Cenozoic Deformation and Uplift of the NE Tibetan Plateau: Evidence from High-Resolution Magnetostratigraphy of the Guide Basin, Qinghai Province, China. Geol. Soc. America Bull. 117 (9-10), 1208–1225. doi:10.1130/b25727.1
Fang, X., Zhao, Z., Li, J., Yan, M., Pan, B., Song, C., et al. (2005b). Magnetostratigraphy of the Late Cenozoic Laojunmiao Anticline in the Northern Qilian Mountains and its Implications for the Northern Tibetan Plateau Uplift. Sci. China Ser. D 48 (7), 1040. doi:10.1360/03yd0188
Fluteau, F., Ramstein, G., and Besse, J. (1999). Simulating the Evolution of the Asian and African Monsoons during the Past 30 Myr Using an Atmospheric General Circulation Model. J. Geophys. Res. 104, 11995–12018. doi:10.1029/1999JD900048
Ge, J., Dai, Y., Zhang, Z., Zhao, D., Li, Q., Zhang, Y., et al. (2013). Major Changes in East Asian Climate in the Mid-pliocene: Triggered by the Uplift of the Tibetan Plateau or Global Cooling? J. Asian Earth Sci. 69, 48–59. doi:10.1016/j.jseaes.2012.10.009
Gradstein, F., Ogg, J., and Smith, A. (2004). A Geologic Time Scale 2004 Cambridge Univ. Press, 589.
Graham, S. A., Chamberlain, C. P., and Yue, Y. (2005). Stable Isotope Records of Cenozoic Climate and Topography, Tibetan Plateau and Tarim Basin. Am. J. Sci. 305, 101–118. doi:10.1016/j.apsusc.2005.12.12010.2475/ajs.305.2.101
Guo, P., Liu, C., Huang, L., Yu, M., Wang, P., and Zhang, G. (2018). Palaeohydrological Evolution of the Late Cenozoic saline lake in the Qaidam Basin, NE Tibetan Plateau: Tectonic vs. Climatic Control. Glob. Planet. Change 165, 44–61. doi:10.1016/j.gloplacha.2018.03.012
Guo, Z. T., Ruddiman, W. F., Hao, Q. Z., Wu, H. B., Qiao, Y. S., Zhu, R. X., et al. (2002). Onset of Asian Desertification by 22 Myr Ago Inferred from Loess Deposits in China. Nature 416, 159–163. doi:10.1038/416159a
Hamilton-Taylor, J., and Davison, W. (1995). “Redox-driven Cycling of Trace Elements in Lakes,” in Physics and Chemistry of Lakes. Editors A. Lerman, D. M. Imboden, and J. R. Gat (Berlin: Springer-Verlag), 217–263. doi:10.1007/978-3-642-85132-2_8
Han, W., Appel, E., Galy, A., Rösler, W., Fang, X., Zhu, X., et al. (2020). Climate Transition in the Asia Inland at 0.8-0.6 Ma Related to Astronomically Forced Ice Sheet Expansion. Quat. Sci. Rev. 248, 106580. doi:10.1016/j.quascirev.2020.106580
Han, W., Fang, X., Ye, C., Teng, X., and Zhang, T. (2014). Tibet Forcing Quaternary Stepwise Enhancement of westerly Jet and central Asian Aridification: Carbonate Isotope Records from Deep Drilling in the Qaidam Salt Playa, NE Tibet. Glob. Planet. Change 116, 68–75. doi:10.1016/j.gloplacha.2014.02.006
Hanson, A. D. (1999). “Organic Geochemistry and Petroleum Geology, Tectonics, and basin Analysis of Southern Tarim and Northern Qaidam, Northwest China,” (Ph. D. thesis. Stanford University).
Herbert, T. D., Lawrence, K. T., Tzanova, A., Peterson, L. C., Caballero-Gill, R., and Kelly, C. S. (2016). Late Miocene Global Cooling and the Rise of Modern Ecosystems. Nat. Geosci 9 (11), 843–847. doi:10.1038/ngeo2813
Hild, E., and Brumsack, H.-J. (1998). Major and Minor Element Geochemistry of Lower Aptian Sediments from the NW German Basin (Core Hohenegglesen KB 40). Cretaceous Res. 19, 615–633. doi:10.1006/cres.1998.0122
Huerta-Diaz, M. A., and Morse, J. W. (1992). Pyritization of Trace Metals in Anoxic marine Sediments. Geochimica et Cosmochimica Acta 56, 2681–2702. doi:10.1016/0016-7037(92)90353-k
Jia, Y., Wu, H., Zhang, W., Li, Q., Yu, Y., Zhang, C., et al. (2021). Quantitative Cenozoic Climatic Reconstruction and its Implications for Aridification of the Northeastern Tibetan Plateau. Palaeogeogr. Palaeoclimatol. Palaeoecol. 567, 110244. doi:10.1016/j.palaeo.2021.110244
Kaboth-Bahr, S., Koutsodendris, A., Lu, Y., Nakajima, K., Zeeden, C., Appel, E., et al. (2020). A Late Pliocene to Early Pleistocene (3.3-2.1 Ma) Orbital Chronology for the Qaidam Basin Paleolake (NE Tibetan Plateau) Based on the SG-1b Drillcore Record. nos 53 (4), 479–496. doi:10.1127/nos/2020/0555
Kaya, M. Y., Dupont‐Nivet, G., Proust, J. N., Roperch, P., Bougeois, L., Meijer, N., et al. (2019). Paleogene Evolution and Demise of the proto‐Paratethys Sea in Central Asia (Tarim and Tajik Basins): Role of Intensified Tectonic Activity at Ca. 41 Ma. Basin Res. 31 (3), 461–486. doi:10.1111/bre.12330
Kent-Corson, M. L., Ritts, B. D., Zhuang, G., Bovet, P. M., Graham, S. A., and Page Chamberlain, C. (2009). Stable Isotopic Constraints on the Tectonic, Topographic, and Climatic Evolution of the Northern Margin of the Tibetan Plateau. Earth Planet. Sci. Lett. 282 (1-4), 158–166. doi:10.1016/j.epsl.2009.03.011
Koutsodendris, A., Allstädt, F. J., Kern, O. A., Kousis, I., Schwarz, F., Vannacci, M., et al. (2019). Late Pliocene Vegetation Turnover on the NE Tibetan Plateau (Central Asia) Triggered by Early Northern Hemisphere Glaciation. Glob. Planet. Change 180, 117–125. doi:10.1016/j.gloplacha.2019.06.001
Kutzbach, J. E., Guetter, P. J., Ruddiman, W. F., and Prell, W. L. (1989). Sensitivity of Climate to Late Cenozoic Uplift in Southern Asia and the American West: Numerical Experiments. J. Geophys. Res. 94, 18393–18407. doi:10.1029/JD094iD15p18393
Lawrence, K. T., Herbert, T. D., Brown, C. M., Raymo, M. E., and Haywood, A. M. (2009). High-amplitude Variations in North Atlantic Sea Surface Temperature during the Early Pliocene Warm Period. Paleoceanography 24 (2), a–n. doi:10.1029/2008PA001669
Li, J., Fang, X., Song, C., Pan, B., Ma, Y., and Yan, M. (2014). Late Miocene-Quaternary Rapid Stepwise Uplift of the NE Tibetan Plateau and its Effects on Climatic and Environmental Changes. Quat. Res. 81, 400–423. doi:10.1016/j.yqres.2014.01.002
Li, J. X., Yue, L. P., Roberts, A. P., Hirt, A. M., Pan, F., Guo, L., et al. (2018). Global Cooling and Enhanced Eocene Asian Mid-latitude interior Aridity. Nat. Commun. 9, 3026. doi:10.1038/s41467-018-05415-x
Liang, M., Guo, Z., Kahmann, A. J., and Oldfield, F. (2009). Geochemical Characteristics of the Miocene Eolian Deposits in China: Their Provenance and Climate Implications. Geochem. Geophys. Geosyst. 10 (4), a–n. doi:10.1029/2008GC002331
Liu, L., Wang, H., Chen, Y., and Chen, J. (2002). Chemical Leaching of Loess Deposits in China and its Implications for Carbonate Composition. Acta Petrol. Mineral. 21 (1), 69–75. doi:10.3969/j.issn.1000-6524.2002.01.009
Lozier, M. S. (2010). Deconstructing the Conveyor Belt. Science 328 (5985), 1507–1511. doi:10.1126/science.1189250
Lu, H., Tian, X., Yun, K., and Li, H. (2018). Convective Removal of the Tibetan Plateau Mantle Lithosphere by ∼26 Ma. Tectonophysics 731-732, 17–34. doi:10.1016/j.tecto.2018.03.006
Lu, H., Wang, X., Wang, X., Chang, X., Zhang, H., Xu, Z., et al. (2019). Formation and Evolution of Gobi Desert in central and Eastern Asia. Earth-Science Rev. 194, 251–263. doi:10.1016/j.earscirev.2019.04.014
Lu, J. F., Song, B. W., Chen, R. M., Zhang, J. Y., and Ye, H. (2010). Palynological Assemblage of Eocene-Oligocene Pollen and Their Biostratigraphic Correlation in Dahonggou, Daqaidam Area, Qaidam Basin. Earth Sci. J. China Univ. Geosci. (Wuhan) 35 (5), 839–848. doi:10.3799/dqkx.2010.097
Lu, Y., Fang, X., Appel, E., Wang, J., Herb, C., Han, W., et al. (2015). A 7.3-1.6Ma Grain Size Record of Interaction between Anticline Uplift and Climate Change in the Western Qaidam Basin, NE Tibetan Plateau. Sediment. Geology. 319, 40–51. doi:10.1016/j.sedgeo.2015.01.008
Lu, Y., Marco, S., Wetzler, N., Fang, X., Alsop, G. I., and Hubert‐Ferrari, A. (2021). A Paleoseismic Record Spanning 2‐Myr Reveals Episodic Late Pliocene Deformation in the Western Qaidam Basin, NE Tibet. Geophys. Res. Lett. 48, e2020GL090530. doi:10.1029/2020GL090530
Manabe, S., and Terpstra, T. B. (1974). The Effects of Mountains on the General Circulation of the Atmosphere as Identified by Numerical Experiments. J. Atmos. Sci. 31, 3–42. doi:10.1175/1520-0469(1974)031<0003:TEOMOT>2.0.CO;2
Marwan, N., Carmenromano, M., Thiel, M., and Kurths, J. (2007). Recurrence Plots for the Analysis of Complex Systems. Phys. Rep. 438 (5–6), 237–329. doi:10.1016/j.physrep.2006.11.001
Maynard, J. B. (2003). “Manganiferous Sediments, Rocks, and Ores,” in Treatise on Geochemistry. Editors H. D. Holland, and K. K. Turekian, 7, 289–308. doi:10.1016/B0-08-043751-6/07099-7
Meijer, N., Dupont-Nivet, G., Abels, H. A., Kaya, M. Y., Licht, A., Xiao, M., et al. (2019). Central Asian Moisture Modulated by Proto-Paratethys Sea Incursions since the Early Eocene. Earth Planet. Sci. Lett. 510, 73–84. doi:10.1016/j.epsl.2018.12.031
Miao, Y., Fang, X., Herrmann, M., Wu, F., Zhang, Y., and Liu, D. (2011). Miocene Pollen Record of KC-1 Core in the Qaidam Basin, NE Tibetan Plateau and Implications for Evolution of the East Asian Monsoon. Palaeogeogr. Palaeoclimatol. Palaeoecol. 299, 30–38. doi:10.1016/j.palaeo.2010.10.026
Miao, Y., Fang, X., Liu, Y.-S., Yan, X., Li, S., and Xia, W. (2016). Late Cenozoic Pollen Concentration in the Western Qaidam Basin, Northern Tibetan Plateau, and its Significance for Paleoclimate and Tectonics. Rev. Palaeobotany Palynology 231, 14–22. doi:10.1016/j.revpalbo.2016.04.008
Narisma, G. T., Foley, J. A., Licker, R., and Ramankutty, N. (2007). Abrupt Changes in Rainfall during the Twentieth century. Geophys. Res. Lett. 34, L06710. doi:10.1029/2006GL028628
Nie, J., Stevens, T., Song, Y., King, J. W., Zhang, R., Ji, S., et al. (2014). Pacific Freshening Drives Pliocene Cooling and Asian Monsoon Intensification. Sci. Rep. 4 (5474). doi:10.1038/srep05474
Porter, S. C., and Zhisheng, A. (1995). Correlation between Climate Events in the North Atlantic and China during the Last Glaciation. Nature 375, 305–308. doi:10.1038/375305a0
Ramstein, G., Fluteau, F., Besse, J., and Joussaume, S. (1997). Effect of Orogeny, Plate Motion and Land-Sea Distribution on Eurasian Climate Change over the Past 30 Million Years. Nature 386, 788–795. doi:10.1038/386788a0
Rea, D. K., Snoeckx, H., and Joseph, L. H. (1998). Late Cenozoic Eolian Deposition in the North Pacific: Asian Drying, Tibetan Uplift, and Cooling of the Northern Hemisphere. Paleoceanography 13, 215–224. doi:10.1029/98PA00123
RGMRGD (1985). “(Regional Geology and Mineral Resources Geological Division of Ministry of Geology and Mineral Resources),” in Compilation on the Geology of Manganese Deposit in China (Beijing: Geological Publishing House), 1–11.
Rimstidt, J. D., Balog, A., and Webb, J. (1998). Distribution of Trace Elements Between Carbonate Minerals And Aqueous Solutions. Geochim. Cosmochim. Acta 62, 1851–1863. doi:10.1016/S0016-7037(98)00125-2
Schaller, T., and Wehrli, B. (1997). Geochemical-focusing of Manganese in lake Sediments ? an Indicator of Deep-Water Oxygen Conditions. Aquat. Geochem. 2 (4), 359–378. doi:10.1007/bf00115977
Song, B., Ji, J., Wang, C., Xu, Y., and Zhang, K. (2017). Intensified Aridity in the Qaidam Basin during the Middle Miocene: Constraints from Ostracod, Stable Isotope, and Weathering Records. Can. J. Earth Sci. 54 (3), 242–256. doi:10.1139/cjes-2016-0052
Song, B., Yang, Y., Yang, R., Galy, A., Zhang, K., Ji, J., et al. (2020). Miocene 87Sr/86Sr Ratios of Ostracods in the Northern Qaidam Basin, NE Tibetan Plateau, and Links with Regional Provenance, Weathering and Eolian Input. Palaeogeogr. Palaeoclimatol. Palaeoecol. 552, 109775. doi:10.1016/j.palaeo.2020.109775
Stevens, L., Ito, E., and Olson, D. (2000). Relationship of Mn-Carbonates in Varved lake-sediments to Catchment Vegetation in Big Watab Lake, MN, USA. J. Paleolimnol. 24, 199–211. doi:10.1023/A:1008169526577
Sun, J., and Liu, T. (2006). The Age of the Taklimakan Desert. Science 312 (5780), 1621. doi:10.1126/science.1124616
Sun, J., Ni, X., Bi, S., Wu, W., Ye, J., Meng, J., et al. (2014). Synchronous Turnover of flora, Fauna and Climate at the Eocene-Oligocene Boundary in Asia. Sci. Rep. 4 (1), 1–6. doi:10.1038/srep07463
Sun, J., Talebian, M., Jin, C., Liu, W., Zhang, Z., Cao, M., et al. (2021). Timing and Forcing Mechanism of the Final Neotethys Seawater Retreat from Central Iran in Response to the Arabia-Asia Collision in the Late Early Miocene. Glob. Planet. Change 197, 103395. doi:10.1016/j.gloplacha.2020.103395
Sun, J., Windley, B. F., Zhang, Z., Fu, B., and Li, S. (2016). Diachronous Seawater Retreat from the Southwestern Margin of the Tarim Basin in the Late Eocene. J. Asian Earth Sci. 116, 222–231. doi:10.1016/j.jseaes.2015.11.020
Sun, J., Ye, J., Wu, W., Ni, X., Bi, S., Zhang, Z., et al. (2010a). Late Oligocene-Miocene Mid-latitude Aridification and Wind Patterns in the Asian interior. Geology 38 (6), 515–518. doi:10.1130/G30776.1
Sun, J., Zhang, Z., Cao, M., Windley, B. F., Tian, S., Sha, J., et al. (2020a). Timing of Seawater Retreat from Proto-Paratethys, Sedimentary Provenance, and Tectonic Rotations in the Late Eocene-Early Oligocene in the Tajik Basin, Central Asia. Palaeogeogr. Palaeoclimatol. Palaeoecol. 545, 109657. doi:10.1016/j.palaeo.2020.109657
Sun, Y., An, Z., Clemens, S. C., Bloemendal, J., and Vandenberghe, J. (2010b). Seven Million Years of Wind and Precipitation Variability on the Chinese Loess Plateau. Earth Planet. Sci. Lett. 297, 525–535. doi:10.1016/j.epsl.2010.07.004b
Sun, Y., Liu, J., Liang, Y., Ji, J., Liu, W., Aitchison, J. C., et al. (2020b). Cenozoic Moisture Fluctuations on the Northeastern Tibetan Plateau and Association with Global Climatic Conditionsfluctuations on the Northeastern Tibetan Plateau and Association with Global Climatic Conditions. J. Asian Earth Sci. 200, 104490. doi:10.1016/j.jseaes.2020.104490
Sun, Y., Yan, Y., Nie, J., Li, G., Shi, Z., Qiang, X., et al. (2020c). Source-to-sink Fluctuations of Asian Aeolian Deposits since the Late Oligocene. Earth-Science Rev. 200, 102963. doi:10.1016/j.earscirev.2019.102963
Tada, R., Zheng, H., and Clift, P. D. (2016). Evolution and Variability of the Asian Monsoon and its Potential Linkage with Uplift of the Himalaya and Tibetan Plateau. Prog. Earth Planet. Sci. 3 (1), 4. doi:10.1186/s40645-016-0080-y
Tribovillard, N., Algeo, T. J., Lyons, T., and Riboulleau, A. (2006). Trace Metals as Paleoredox and Paleoproductivity Proxies: An Update. Chem. Geology. 232, 12–32. doi:10.1016/j.chemgeo.2006.02.012
Wang, C. N., Guo, X. H., Ma, M. Z., Li, J. D., and Li, J. (2008). Ore-forming Geological Background of K–Mg Salt in Qarhan Salt Lake. Northwest. Geology. 41 (1), 97–106. doi:10.3969/j.issn.1009-6248.2008.01.012
Wang, J., Fang, X., Appel, E., and Song, C. (2012). Pliocene-Pleistocene Climate Change at the NE Tibetan Plateau Deduced from Lithofacies Variation in the Drill Core SG-1, Western Qaidam basin, China. J. Sediment. Res. 82 (12), 933–952. doi:10.2110/jsr.2012.76
Wang, X., Carrapa, B., Sun, Y., Dettman, D. L., Chapman, J. B., Caves Rugenstein, J. K., et al. (2020). The Role of the Westerlies and Orography in Asian Hydroclimate since the Late Oligocene. Geology 48 (7), 728–732. doi:10.1130/G47400.1
Xia, W., Zhang, N., Yuan, X. P., Fan, L. S., and Zhang, B. S. (2001). Cenozoic Qaidam Basin, China: a Stronger Tectonic Inversed, Extensional Rifted basin. Bulletin 85, 715–736. doi:10.1306/8626C98D-173B-11D7-8645000102C1865D
Westerhold, T., Marwan, N., Drury, A. J., Liebrand, D., Agnini, C., Anagnostou, E., et al. (2020). An Astronomically Dated Record of Earth's Climate and its Predictability over the Last 66 Million Years. Science 369 (6509), 1383–1387. doi:10.1126/science.aba6853
Wetzel, R. G. (2001). “Iron, Sulfur, and Silica Cycles,” in Limnology: Lake and River Ecosystems. 3rd ed. (San Diego: Academic Press), 289–330. doi:10.0000/13518479833729010.1016/b978-0-08-057439-4.50018-6
Wu, M., Zhuang, G., Hou, M., and Liu, Z. (2021). Expanded Lacustrine Sedimentation in the Qaidam Basin on the Northern Tibetan Plateau: Manifestation of Climatic Wetting during the Oligocene Icehouse. Earth Planet. Sci. Lett. 565, 116935. doi:10.1016/j.epsl.2021.116935
Wu, M., Zhuang, G., Hou, M., and Miao, Y. (2019). Ecologic Shift and Aridification in the Northern Tibetan Plateau Revealed by Leaf Wax N-Alkane δ2H and δ13C Records. Palaeogeogr. Palaeoclimatol. Palaeoecol. 514, 464–473. doi:10.1016/j.palaeo.2018.11.005
Yang, J., Chen, J., An, Z., Shields, G., Tao, X., Zhu, H., et al. (2000). Variations in 87Sr/86Sr Ratios of Calcites in Chinese Loess: a Proxy for Chemical Weathering Associated with the East Asian Summer Monsoon. Palaeogeogr. Palaeoclimatol. Palaeoecol. 157, 151–159. doi:10.1016/S0031-0182(99)00159-5
Yang, Y., Fang, X., Appel, E., Galy, A., Li, M., and Zhang, W. (2013a). Late Pliocene-Quaternary Evolution of Redox Conditions in the Western Qaidam Paleolake (NE Tibetan Plateau) Deduced from Mn Geochemistry in the Drilling Core SG-1. Quat. Res. 80, 586–595. doi:10.1016/j.yqres.2013.07.007
Yang, Y., Fang, X., Galy, A., Appel, E., and Li, M. (2013b). Quaternary Paleolake Nutrient Evolution and Climatic Change in the Western Qaidam basin Deduced from Phosphorus Geochemistry Record of Deep Drilling Core SG-1. Quat. Int. 313-314 (nov.5), 156–167. doi:10.1016/j.quaint.2013.06.004
Yang, Y., Fang, X., Galy, A., Li, M., Appel, E., and Liu, X. (2014). Paleoclimatic Significance of Rare Earth Element Record of the Calcareous Lacustrine Sediments from a Long Core (SG-1) in the Western Qaidam Basin, NE Tibetan Plateau. J. Geochemical Exploration 145, 223–232. doi:10.1016/j.gexplo.2014.06.013
Yang, Y., Fang, X., Koutsodendris, A., Ye, C., Yang, R., Zhang, W., et al. (2016). Exploring Quaternary Paleolake Evolution and Climate Change in the Western Qaidam basin Based on the Bulk Carbonate Geochemistry of lake Sediments. Palaeogeogr. Palaeoclimatol. Palaeoecol. 446, 152–161. doi:10.1016/j.palaeo.2016.01.021
Yang, Y., Fang, X., Li, M., Galy, A., Koutsodendris, A., and Zhang, W. (2015). Paleoenvironmental Implications of Uranium Concentrations in Lacustrine Calcareous Clastic-Evaporite Deposits in the Western Qaidam basin. Palaeogeogr. Palaeoclimatol. Palaeoecol. 417, 422–431. doi:10.1016/j.palaeo.2014.10.002
Yang, Y., Galy, A., Fang, X., Yang, R., Zhang, W., Song, B., et al. (2021). Neodymium Isotopic Constraints on Cenozoic Asian Dust Provenance Changes Linked to the Exhumation History of the Northern Tibetan Plateau and the Central Asian Orogenic Belt. Geochimica et Cosmochimica Acta 296, 38–55. doi:10.1016/j.gca.2020.12.026
Ye, C., Yang, Y., Fang, X., Hong, H., Wang, C., Yang, R., et al. (2018). Chlorite Chemical Composition Change in Response to the Eocene-Oligocene Climate Transition on the Northeastern Tibetan Plateau. Palaeogeogr. Palaeoclimatol. Palaeoecol. 512, 23–32. doi:10.1016/j.palaeo.2018.03.014
Ye, C., Yang, Y., Fang, X., and Zhang, W. (2016). Late Eocene clay boron-derived Paleosalinity in the Qaidam Basin and its Implications for Regional Tectonics and Climate. Sediment. Geology. 346, 49–59. doi:10.1016/j.sedgeo.2016.10.006
Ye, C., Yang, Y., Fang, X., Zhang, W., Song, C., and Yang, R. (2020). Paleolake Salinity Evolution in the Qaidam Basin (NE Tibetan Plateau) between ∼42 and 29 Ma: Links to Global Cooling and Paratethys Sea Incursions. Sediment. Geology. 409, 105778. doi:10.1016/j.sedgeo.2020.105778
Zachos, J., Pagani, M., Sloan, L., Thomas, E., and Billups, K. (2001). Trends, Rhythms, and Aberrations in Global Climate 65 Ma to Present. Science 292, 686–693. doi:10.1126/science.1059412
Zhang, R., Jiang, D., Liu, X., and Tian, Z. (2012). Modeling the Climate Effects of Different Subregional Uplifts within the Himalaya-Tibetan Plateau on Asian Summer Monsoon Evolution. Chin. Sci. Bull. 57, 4617–4626. doi:10.1007/s11434-012-5284-y
Zhang, W., Appel, E., Fang, X., Yan, M., Song, C., and Cao, L. (2012a). Paleoclimatic Implications of Magnetic Susceptibility in Late Pliocene-Quaternary Sediments from Deep Drilling Core SG-1 in the Western Qaidam Basin (NE Tibetan Plateau). J. Geophys. Res. 117, B06101. doi:10.1029/2011JB008949
Zhang, W., Appel, E., Fang, X., Song, C., and Cirpka, O. (2012b). Magnetostratigraphy of Deep Drilling Core SG-1 in the Western Qaidam Basin (NE Tibetan Plateau) and its Tectonic Implications. Quat. Res. 78, 139–148. doi:10.1016/j.yqres.2012.03.011
Zhang, W., Appel, E., Fang, X., Song, C., Setzer, F., Herb, C., et al. (2014a). Magnetostratigraphy of Drill-Core SG-1b in the Western Qaidam Basin (NE Tibetan Plateau) and Tectonic Implications. Geophys. J. Int. 197 (1), 90–118. doi:10.1093/gji/ggt439
Zhang, Z., Ramstein, G., Schuster, M., Li, C., Contoux, C., and Yan, Q. (2014b). Aridification of the Sahara Desert Caused by Tethys Sea Shrinkage during the Late Miocene. Nature 513 (7518), 401–404. doi:10.1038/nature13705
Zhongshi, Z., Huijun, W., Zhengtang, G., and Dabang, J. (2007). Impacts of Tectonic Changes on the Reorganization of the Cenozoic Paleoclimatic Patterns in China. Earth Planet. Sci. Lett. 257 (3-4), 622–634. doi:10.1016/j.epsl.2007.03.024
Zhuang, G., Brandon, M. T., Pagani, M., and Krishnan, S. (2014). Leaf Wax Stable Isotopes from Northern Tibetan Plateau: Implications for Uplift and Climate since 15 Ma. Earth Planet. Sci. Lett. 390, 186–198. doi:10.1016/j.epsl.2014.01.003
Zhuang, G., Hourigan, J. K., Koch, P. L., Ritts, B. D., and Kent-Corson, M. L. (2011). Isotopic Constraints on Intensified Aridity in Central Asia Around 12Ma. Earth Planet. Sci. Lett. 312, 152–163. doi:10.1016/j.epsl.2011.10.005
Keywords: late cenozoic, Asian inland drying, Qaidam Basin, carbonate, manganese
Citation: Liu Y, Yang Y, Ye C, Yang R, Appel E and Fang X (2021) Global Change Modulated Asian Inland Climate Since 7.3 Ma: Carbonate Manganese Records in the Western Qaidam Basin. Front. Earth Sci. 9:813727. doi: 10.3389/feart.2021.813727
Received: 12 November 2021; Accepted: 06 December 2021;
Published: 21 December 2021.
Edited by:
Davide Tiranti, Agenzia Regionale per la Protezione Ambientale (ARPA), ItalyCopyright © 2021 Liu, Yang, Ye, Yang, Appel and Fang. This is an open-access article distributed under the terms of the Creative Commons Attribution License (CC BY). The use, distribution or reproduction in other forums is permitted, provided the original author(s) and the copyright owner(s) are credited and that the original publication in this journal is cited, in accordance with accepted academic practice. No use, distribution or reproduction is permitted which does not comply with these terms.
*Correspondence: Yibo Yang, eWFuZ3lpYm9AaXRwY2FzLmFjLmNu