- 1School of Geosciences, China University of Petroleum, Qingdao, China
- 2Xianhe Oil Production Plant of Shengli Oilfield Company, SINOPEC, Dongying, China
- 3Shengli Oil Production Plant of Shengli Oilfield Company, SINOPEC, Dongying, China
A suite of source rock consists of mudstone and shale, with great thickness and continuous deposition was found in the well LK-1 in Lingshan island in Ri-Qing-Wei basin. In order to evaluate the hydrocarbon generation prospects of these source rock and find the mechanism of organic matter enrichment, shale samples were selected from the core for TOC (total organic carbon) and element geochemistry analysis. The results show that organic matter abundance of the source rocks are generally high with average TOC content of 1.26 wt%, suggesting they are good source rocks. The geochemical features show that the sedimentary environment is mostly anoxic brackish water to salt water environment with arid to semiarid climate condition. The enrichment mechanism of organic matter varied with the evolution of the basin, which was divided into three stages according to the sedimentary characteristics. In the initial-middle period of rifting evolution (stage 1 and early stage 2), paleoproductivity is the major factor of OM-enrichment reflecting by high positive correlation between the TOC contents and paleoproductivity proxies. While with the evolution of the rift basin, redox condition and terrigenous clastic input became more and more important until they became the major factor of OM enrichment in the middle stage of rift evolution (stage 2). In the later stage of rift evolution (latest stage 2 and stage 3), besides terrigenous clastic input, the effect of paleoclimate on OM-enrichment increased gradually from a minor factor to a major factor.
Introduction
As the most important type of source rock, shale is widely distributed in almost every depositional setting in different tectonic settings (Verma and Armstrong-Altrin, 2013). Whatever depositional setting the shale belongs to, the Total Organic Carbon (TOC) content is the primary indicator to judge the oil and gas potential. Almost each big oil and gas field all over the world owes a suite of shale or mudstone as source rock, with huge thickness and high TOC content. Previous researches (Yan et al., 2018; Gallego-Torres et al., 2007; Mort et al., 2007) indicate that restricted basin sedimentary conditions are good for organic matter (OM) enrichment and there are two typical models for OM enrichment: the paleoproductivity model and the preservation model. The paleoproductivity model emphasizes that large amount of OM enriched for the higher productivity in the sedimentary water. Large amount of OM consume oxygen in the water, leading to an anoxic environment which is good for preservation of OM (Sageman et al., 2003; 8; Tang et al., 2020). While the preservation model stresses the importance of the sea level fluctuation and the restricted effect of basin, proving an anoxic environment for OM accumulation (Arthur et al., 1998; Mort et al., 2007; Tang et al., 2020). In addition, the terrigenous clastic input is another important factor controlling the OM enrichment in recent study (Tang et al., 2020). Changes in sedimentary environment would affect the OM enrichment. The geochemistry of shale could reflect the sedimentary environment such as Ba, P, Cu for paleoproductivity (Paytan and Griffith, 2007; Algeo et al., 2011), Mo, U, V for redox condition and Al, Ti, Zr for terrigenous clastic input (Tribovillard et al., 2006).
There were a lot of Meso-Cenozoic basins developed in eastern China as the respond to the subduction of western Pacific plate. With the development of petroleum exploration, more and more petroliferous basins were found such as Bohai Bay basin, Songliao basin (Wang et al., 2020). However, the oil and gas potential of Jiaolai basin which is the best record with relatively complete Cretaceous strata, is not ideal. Recently, the Lingshan island scientific drilling (Well LK-1) uncovered there was a rifting basin in the central Sulu orogenic belt, beside the Jiaolai basin. Although a lot of researchers have studied Lingshan island in different aspects, the oil and gas potential is still unclear. This study focuses on the core sample of LK-1, using TOC content and element contents of shale samples to reveal the mechanism of organic matter enrichment.
Geological Setting
Ri-Qing-Wei basin is located in the central Sulu orogenic belt, eastern coastal Shandong, China. It is a long and narrow late Mesozoic rift basin and is distributed along the NE-SW direction. The northwest boundary is Wulian-Qingdao faults and Jimo-Muping faults, while the southeast boundary is Qianliyan faults. Lingshan island is located in the south Ri-Qing-Wei basin (Figure 1). The strata in Lingshan island represents the stratigraphic sequence of Ri-Qing-Wei basin. The stratigraphic sequence is divided into two groups: 1) Laiyang group, the older one which is consisted by a series of gravity flows’ deposition (Lü et al., 2011; Zhong et al., 2012; Yang and van Loon, 2016; Yang et al., 2017; Liang et al., 2018; Yang et al., 2018), mainly developed in the west island, including several outcrops (Figure 2A) such as Beilaishi, Qiancengya, Chuanchang, Dengta et al., and 2) Qingshan group, the younger one which is mainly dominated by volcanic deposition and a suite of terrestrial clastic deposits (Wang et al., 2015; Zhou et al., 2017; Zhou et al., 2018).
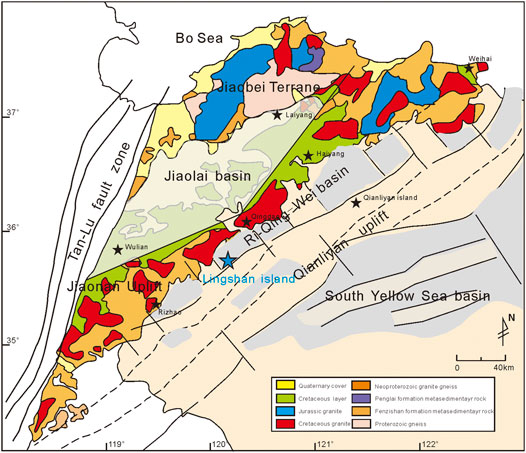
FIGURE 1. Geological map of Ri-Qing-Wei basin and related area (modifiedfrom Zhou et al., 2015; Liu et al., 2019).
In order to uncover the main layer sequence of the Laiyang group, we set up the Lingshan Island Scientific Drill (Well LK-1). The depth of LK-1 is 1,350 m, and the total thickness of layers of shale and mudstone is about 550 m. This discovery further confirms the existence of Ri-Qing-Wei basin. In the layers of Laiyang group which are presented by the core of LK-1 (Figure 2B), various kinds of gravity flows and large amounts of soft sediments deformation structures are found. Also, a lot of markers representing deep-water sedimentary environment are observed, such as iron nodule, vitrain and plant debris. According to the lithology and sedimentary characteristics of LK-1, Yuan et al. (2019) show that the sedimentary strata of Laiyang group developed fan delta, subaqueousfan, turbidite fan and delta from the bottom to the top. In addition, the evolution of Ri-Qing-Wei basin could be divided into four stages (Yuan et al., 2019): initial stage of the basin rifting, the stage of basin expansion and subsidence, the stable stage of deep subsidence and the stage of basin uplift.
Materials and Methods
Eighty-two samples of shale of LK-1 were selected to do TOC (total organic carbon) analysis. Thirty samples of the batch above and other 22 samples were selected to do major and trace element analysis.
TOC (total organic carbon) content analyses were done in Northeast Petroleum University. The TOC contents were measeured with acid-dissolution method. Diluted hydrochloric acid were used to removing the inorganic carbon compound of the samples’ powder. After, samples were burned in the high temperature oxygen. Carbon content of CO2 produced during the burning process are all from the organic carbon of samples. The contents of CO2 were measured by thermal conductivity detector, to get the TOC contents. The detailed analytical method is described by Liu et al. (2019).
Chemical analyses were conducted in the laboratory of Eighth Institute of Geology and Mineral Exploration of Shandong Province, China. Fresh rock pieces without cracks were crushed to 200 meshes before experiment. Major oxides were analyzed by X-ray fluorescence spectrometry (XRF; equipment model is Axios) with the relative standard derivation (RSD) of major oxides less than 5%. Trace elements were analyzed by ICP-MS (equipment model is ICAP Qc), and detailed procedures for trace elements analysis are as described by Liang et al. (2000). The relative standard deviations (RSD) of trace elements are within 5%. The procedures for major and trace element analyses are described by Kimura (1998) and Liang et al. (2000), respectively.
Results
The TOC, major element, trace element and rare element contents of LK-1 shales analyzed in this study are listed in the Supplementary Table S1.
TOC Contents
The TOC contents of collected samples of Laiyang group from LK-1 are generally high, with a mean TOC content at about 1.26 wt%. More than 70% of samples’ TOC content are greater than 1 wt%. The TOC contents are different within different depth of LK-1. In the depth ranges below, TOC content of most samples are greater than 1 wt%: 145–246 m (average TOC, 1.39 wt%), 392–453 m (1.74 wt%), 589–665 m (1.35 wt%), 696–776 m (1.46 wt%), 833–919 m (1.85 wt%), 1,031–1,136 m (1.40 wt%), 1,175–1,301 m (1.45 wt%).
Major Elements
The element composition of the shales of Laiyang group are dominated by SiO2 (51.90–75.12 wt%, average 61.09 wt%), Al2O3 (8.68–18.58 wt%, average 14.86 wt%) and CaO (1.21–17.56 wt%, average 7.12 wt%). Besides, these shales also contain relative low concentrations of Fe2O3 (average 5.22 wt%), K2O (average 3.59 wt%), MgO (average 3.42 wt%), Na2O (average 3.22 wt%). Other major element contents (MnO, P2O5, TiO2) are all lower than 1 wt%.
The contents of SiO2, Al2O3, Fe2O3, K2O and MnO of our samples are close to PAAS (Taylor and Mclennan, 1985), while the contents of CaO and P2O5 show much higher than the corresponding contents in PASS (Figure 3). The higher concentration of CaO may be related to the calcite veins which widely spread in the core. The higher content of P2O5 may reflect the higher paleoproductivity in the sedimentary water in Laiyang period, because P is an important nutrient element to life.
A negative correlation can be seen between SiO2 and Al2O3 contents, and between Al2O3 and CaO contents (Figure 4). No obvious correlation could be observed between Al2O3 and P2O5. These indicate Si, Ca, P of samples are mainly effected by sedimentary environment, while the effect of terrigenous clastic input is relative slight. However, samples show a positive correlation between Al2O3 and TiO2 contents. This indicates Ti in samples were mainly controlled by terrigenous clastic input.
Trace Elements
Different trace element owes different sensitivities to specific geological event, so sedimentary environment could be reconstructed by suitable trace elements. Selecting authigenic element composition could help reflecting the sedimentary water conditions better and also its variation during the geological period.
Element enrichment factors were used to see the enrichment or depletion of an element through comparing with selected standard value. In this study, the trace elemental compositions of the samples were compared with the composition of PAAS (Taylor and Mclennan, 1985) through Al-normalization to identify significant deviations. Element enrichment factors of X (XEF) were caluculated by XEF = (X/Al)Sample/(X/Al)PAAS. XEF > 1 represents the element X was relative enriched in Sample compared to X in PAAS, while XEF < 1 represents the situation of depletion. The XEF of Co, Pb, Ba, Sr, Hf, U are obviously larger than 1 showing these trace elements are enriched in samples than PAAS. While the XEF of V, Cr, Ni, Sc, Cu, Cs are much lower than 1, indicating these elements are depleted. As to the elements Li, Rb, Th, Nb and Zr, behaved similar to PAAS for their XEF values are close to 1 (Figure 3).
Rare Earth Elements
Both sedimentary water condition and terrigenous clastic input affect the rare earth elements in shale. Because of the stability of rare earth elements after they were absorbed from the sedimentary water and preserved in sedimentary rock, they are often used to reflect the paleo-environment conditions (Sholkovitz et al., 1994; Xia et al., 2015). The∑REE of shales of Laiyang group from LK-1 range from 115.69 to 334.03 × 10−6, with an average value of 225.19 × 10−6, larger than ∑REE contents in UCC (148.14 × 10−6) and PAAS (184.77 × 10−6). The ratios of ∑LREE/∑HREE of our samples are 3.48–6.25 with a mean value of 5.10. These show shales of Laiyang group from LK-1 are enriched in LREE, relative depleted in REE.
The trends of variation of LREE and HREE contents match each other in vertical direction of well LK-1 (Supplementary Table S1). At the beginning, from the depth 1,312–1,032 m, the ratio of ∑LREE/∑HREE varies from 4.72 to 5.69, does not change much. The ratio show much variation (3.48–6.25) from the depth 887–412 m. From the depth of 412–24 m, the ratio does not show much fluctuation, demonstrating the similar situation as the beginning. The different variation of ∑LREE/∑HREE may be related to the terrigenous clastic input and sedimentation rate.
Discussion
The calculations of proxies are represented in Supplementary Table S1.
Paleoproductivity Proxies
The enrichement of organic matter depends on whether there were enough organic matter sources in the geological period. The sources of organic matter mainly come from two parts: besides the partly organic matter provided by terrigenous clastic input, the biological productivity in sedimentary water is another key factor for organic matter enrichment (Authur and Sageman, 1994). The higher biological productivity in sedimentary water, the better sedimentary condition for organic matter enrichment. Elements such as Ba, P, Al and Ni were widely used as paleoproductivity proxies. In this study, we select P and biogenic Ba(Babio) content to discuss the paleoproductivity of Laiyang group.
Element P is necessary for organisms to survive and reproduce in water and the content of P element could reflect the paleoproductivity. In addition, the P of organisms will transfer into sediments after their death (Shen et al., 2015). In this study, P of samples are divided by Al and Ti to avoid the effect of terrigenous clastic input, so P/Al and P/Ti could reflect the paleoproductivity more precisely. The P/Ti and P/Al of shales of Laiyang group from LK-1 vary with the depth, behaving similar trends. The ratio of P/Ti ranges from 0.14 to 0.71 with a mean value of 0.28 and the ratio of P/Al ranges from 0.007 to 0.035 with a mean value of 0.012. These two ratios are generally larger than the ones of PAAS (P/Ti = 0.12, P/Al = 0.007) according to Figure 5, indicating the high level of paleoproductivity in Laiyang period.
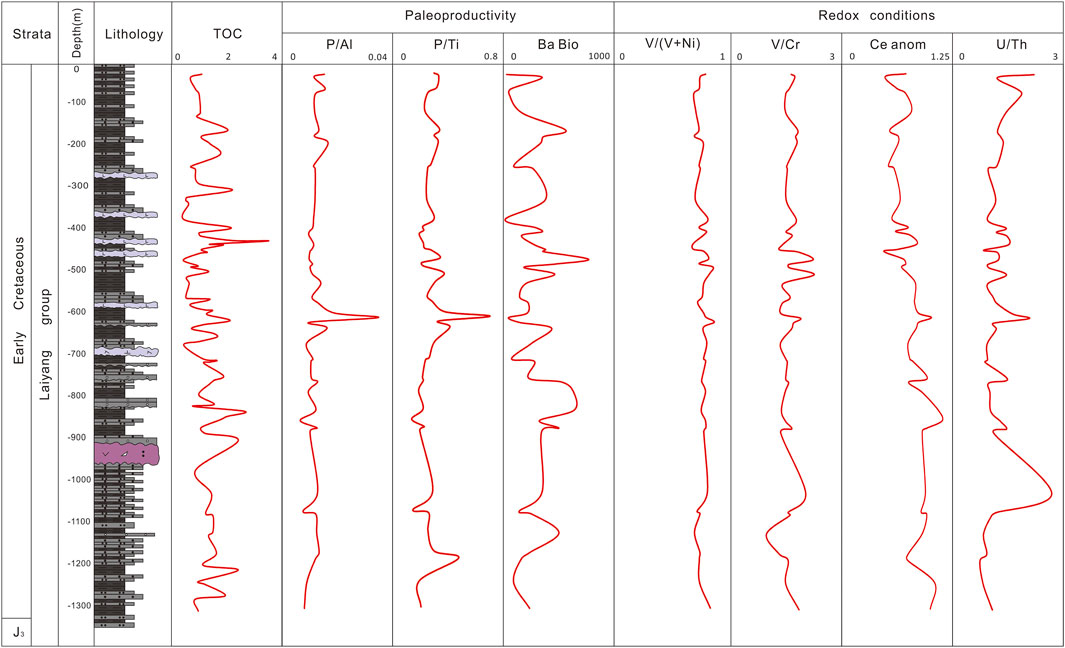
FIGURE 5. Stratigraphic distribution of TOC, paleoproductivity proxies, redox proxies, in well LK-1.
Biogenic Ba content (Babio) has been widely used as an important paleoproductivity proxy. Also the Ba of samples were affected by terrigenous clastic as other elements, so avoiding the effects of terrigenous clastic is the first step to do. Murray and Leinen (1996) proposed the method to calculate the biogenic Ba content (Babio): Babio = BaSample − AlSample × 0.0077. The Babio values of shales of Laiyang group from LK-1 range from 11.85 to 767.05 ppm with a mean value of 283.99 ppm. The trend of Babio does not match the trends of P/Ti and P/Al perfectly because the content of Ba may be affected by the redox condition and the hydrothermal activity.
Redox Proxies
Redox condition of sedimentary water is directly related to the preservation of organic matter, so the study of redox condition is very important. V/(V + Ni) and Ceanom are widely used to judge the redox condition (Abanda and Hannigan, 2006; Raiswell, 1988; Wang et al., 2014). V/(V + Ni) < 0.46 represents oxic environment, 0.46–0.57 represents semi-anoxic environment, >0.57 represents anoxic environment. Moreover, when the value of V/(V + Ni) is larger than 0.54 but less than 0.82, anoxic and a less strongly stratified water column is indicated. If the value of V/(V + Ni) is larger than 0.84, it indicates the presence of H2S in a strongly stratified water column (Hatch and Leventhal, 1992). To avoid the potential analytical errors of single element concentration, other reliable indices such as V/Cr, Ceanom and U/Th (German and Elderfield, 1990; Jones and Manning, 1994) are also used in this study. Values of V/Cr above two represent anoxic of depositional conditions, and the closer this value gets to 1, the more oxic the depositional condition it was. Ce anomaly was defined and applied by German and Elderfield (1990) as a paleoredox indicator: Ce anomaly (Ce/Ce*) = 3(Ce/Ceshale)/[(2La/Lashale)+(Nd/Ndshale)], the value of REE of the shale as normalized standard is from De Baar et al. (1985). As Figure 5 shows, the values of V/(V + Ni) of our samples do not show obvious fluctuation with the depth, ranging from 0.68 to 0.86 with a mean value of 0.75. This indicates the depositional condition is anoxic environment with a less strongly stratified water column. The values of Ceanom of our samples range from 1.09 to 1.23 with a mean value of 1.16. According to the application of Ceanom from German and Elderfield (1990), the depositional conditions in Laiyang period is an anoxic environment. The values of U/Th range from 0.70 to 2.69 with a mean value of 1.26, indicating the depositional conditions varied between anoxic and dysoxic environment (Jones and Manning, 1994). The values of V/Cr of shales form LK-1 range from 0.97 to 2.26 with a mean value of 1.58. Although the ratio of V/Cr might be affected by the carbonate content (Jones and Manning, 1994), its trend with the depth shows similarity as the trend of V/(V + Ni). Combined those geochemical evidences above with widely distributed pyrite across the whole layers of Laiyang group, the redox condition of Laiyang period is mainly anoxic environment.
Paleosalinity and Paleoclimate Proxies
Paleosalinity is an important indicator to reflect the sedimentary water, and also could help to judge the marine and terrestrial environment. The ratio of Sr/Ba and the Value m (=100 × MgO/Al2O3) were selected as the indicators to reflect the paleosalinity. The ratio of Sr/Ba < 0.5 represents freshwater environment, 0.5 < Sr/Ba<1 represents brackish water and Sr/Ba>1 represents salt water (He et al., 2019). Zhang et al. (1988) proposed the value m (=100 × MgO/Al2O3) as an indicator to interpret the paleosalinity: m < 1 represents fresh water, 1 < m < 10 represents transitional environment and m > 10 represents marine environment.
The ratios of Sr/Ba of shales of Laiyang group from LK-1 (Figure 6) range from 0.22 to 1.89 with a mean value of 0.68 and the value m range from 10.43 to 43.57 with a mean value of 23.27. These indicate the paleosalinity condition of depositional water of Laiyang period is almost brackish water to salt water in a marine environment.
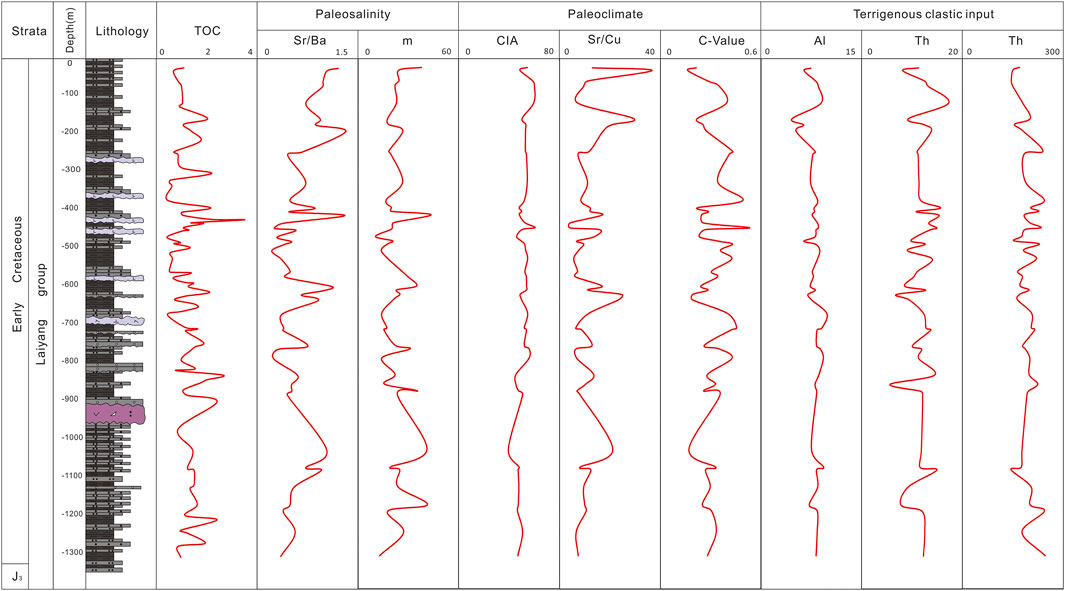
FIGURE 6. Stratigraphic distribution of TOC, paleosalinity proxies, paleoclimate proxies, and terrigenous clastic proxies, in well LK-1.
CIA (Chemical Index of Alteration), C-value and Sr/Cu were selected to interpret the paleoclimate condition of Laiyang period. Because the weathering degree of rock is different under different climate condition, the CIA were successfully applied to reflect the paleoclimate condition (McLennan, 1993): 50 to 65 (arid), 65 to 85 (semiarid to semimoist), 85 to 100 (moist). The element Sr, Cu, Mg, Ca are sensitive to the paleoclimate, so the ratios of Sr/Cu and Mg/Ca were used as indicators of paleoclimate in sedimentary period. The ratio of Sr/Cu > 10 was explained to stand for the arid and hot climate condition, while 1 < Sr/Cu < 10 indicates warm and moist climate condition. The trace elements Fe, Mn, Cr, V, Ni and Co have been proved to be relatively enriched in sediments formed under moist conditions. In contrast, the elements of Ca, Mg, K, Na, Sr and Ba exhibit higher concentrations under arid conditions because the evaporation prompts saline minerals to precipitate (Cao et al., 2012). The C-value [C-value = Σ(Fe + Mn + Cr + Ni + V + Co)/Σ(Ca + Mg + Sr + Ba + K + Na)] has been applied successfully in evaluating the palaeoclimatic conditions (Cao et al., 2012; Fu et al., 2016; He et al., 2019).
In this study (Figure 6), the CIA of shales of Laiyang group range from 39.36 to 60.44 with an mean value 51.26, indicating an arid paleoclimate in Laiyang period. The C-values range from 0.16 to 0.53, reflecting dominant arid to semiarid climatic conditions at the time of deposition. The ratio of Sr/Cu range from 8.46 to 73.59 with a mean value of 25.12, showing an arid to semiarid paleoclimate condition.
Terrigenous Clastic Proxies
The concentration of Al, Ti, Zr, Th in sediments are mainly affected by terrigenous clastic. The latter diagenesis and weathering had little effect on their preservation. So, these elements were used to reflect the situation of terrigenous clastic input during the deposition period (Murphy et al., 2000; Tribovillard et al., 2006). In sedimentary rock, element Ti, Zr and Th are persevered in the heavy minerals such as ilmenite, zircon or in the clay minerals, while Al are mainly persevered in the clay minerals (Young and Nesbitt, 1998). The contents of Al, Ti, Th and Zr vary in a wider range among the shales of Laiyang group: Al (4.62–9.84 wt% with a mean value of 7.97 wt%), Ti (0.18–0.51 wt% with a mean value of 0.36 wt%), Zr (144.51 × 10−6 to 246.31 × 10−6 with a mean value of 191.29 × 10−6) and Th (5.64 × 10−6 to 28.30 × 10−6 with a mean value of 12.04 × 10−6). From the trends of variation of Al, Zr, Th, the condition of terrigenous clastic input varied during the Laiyang period. In the first sedimentary period, the change in terrigenous clastic input was quite small. The condition of terrigenous clastic input raised and then reduced for two times, showing an intermediate level. In the second sedimentary period, the change became much more fluctuant and reached the peak in the middle of this period. This may be related to the continuous input water as the rift developed. The level of terrigenous clastic input reduced at the beginning of the third sedimentary period, then raised gradually.
Controlling Factors of OM Enrichment
From the 15 different indicators above, the basic change in sedimentary condition of Laiyang period in Lingshan island could be concluded. The sedimentary environment is almost brackish water to salt water in a marine environment with redox condition of mainly anoxic. The paleoclimate condition is dominated by arid to semiarid climatic conditions. Paleoproductivity kept at a relatively high level in the whole Laiyang period, compared to PAAS. In order to find the relationship between TOC contents and those indicators of different factors and also find the key indicators in different stages, correlation analysis method were applied (Figure 7).
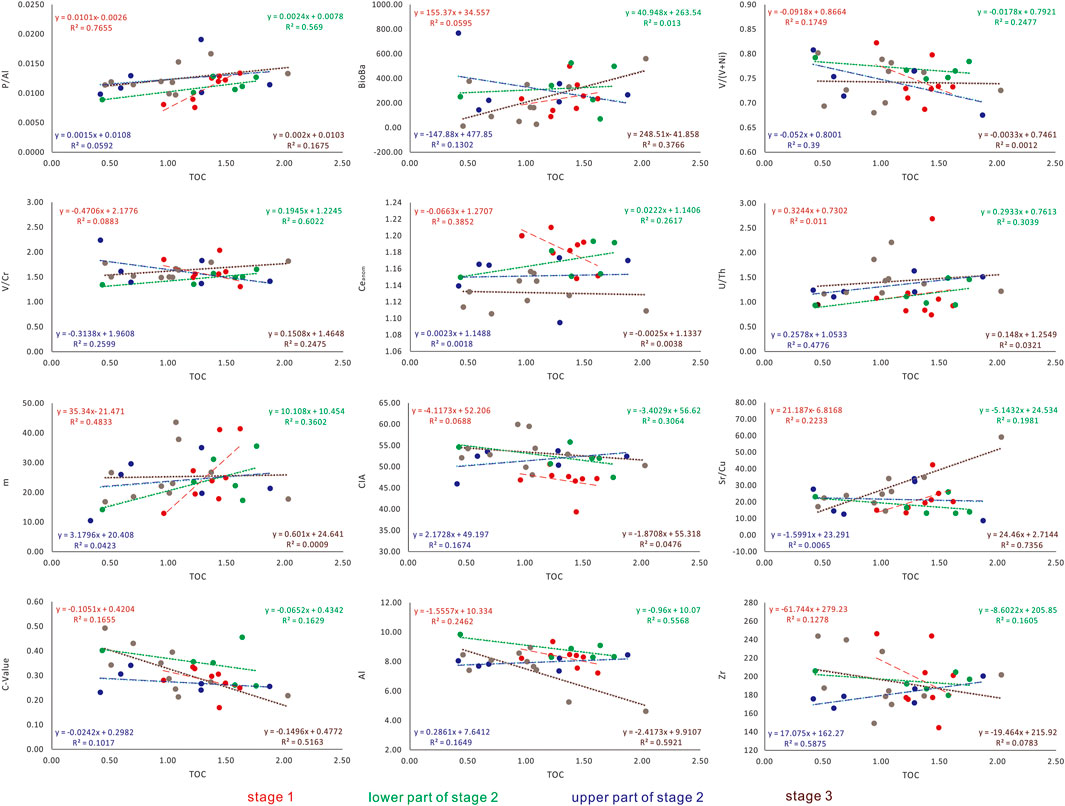
FIGURE 7. The relationship between paleoproductivity proxies, redox proxies, paleosalinity proxies, paleoclimate proxies, terrigenous clastic proxies andTOC contents of three sedimentary stages of Laiyang group, in well LK-1.
In the first stage, the index of P/Al is highly positive correlated to the TOC contents with R2 = 0.7655. This suggests the paleoproductivity is the key controlling factors of OM enrichment in the initial stage. Although Ceanom is slightly negative correlated with the TOC contents (R2 = 0.3852), the correlation coefficients of V/(V + Ni) and V/Cr are all less than 0.2. Thus, the redox condition may be a minor factor of OM enrichment. The paleosalinity condition should be another minor factor of OM enrichment as well, reflecting by its medium positive correlation between m and TOC contents (R2 = 0.4833). The correlation coefficients of all the other indexes versus TOC contents are less than 0.3, suggesting very little effect on OM enrichment. In the second stage, no obvious pattern were seen from the correlation analysis between the selected indexes with TOC contents if this period was regarded as a whole. This is because the variation of the factors of the upper part and the lower part of the second stage follow different trends, suggesting the controlling factors should be different between the upper and lower parts. In the lower part, the index of P/Al is medium positive correlated to the TOC contents (R2 = 0.569), suggesting the paleoproductivity is one of the major controlling factor of OM enrichment. The indicators of redox condition show correlation with the TOC content at different level (V/Cr with R2 = 0.6022, U/Th with R2 = 0.3039, Ceanom with R2 = 0.2617), together suggesting the redox condition is another major controlling factor of OM enrichment in this period. The effect of terrigenous clastic in the lower part is significant, because the Al content is medium negative correlated to the TOC content (R2 = 0.5568). The indicators of paleosalinity and paleoclimate show slightly correlated to the TOC contents (m with R2 = 0.3602 and CIA with R2 = 0.3064), suggesting both the paleosalinity and paleoclimate condition are minor factors of OM enrichment. While in the upper part, only the index of Zr is medium positive correlated to the TOC contents with R2 = 0.5875. But the indexes of Al and Th are almost not correlated to the TOC contents with R2 = 0.1649, 0.201, respectively. The indexes of redox condition show medium to slight correlation with the TOC contents: U/Th with R2 = 0.4776, V/(V + Ni) with R2 = 0.39 and V/Cr with R2 = 0.2599. These together indicate the terrigenous clastic input and redox condition are the major factors of OM enrichment of the upper part of the second stage of Laiyang period. In the third stage, the indexes of Sr/Cu, C-value and Al are highly to medium correlated to the TOC contents with R2 = 0.7356, 0.5163 and 0.5921, respectively, suggesting paleoclimate condition is the major factor and the terrigenous clastic input is the minor factor of OM enrichment. The paleoproductivity also show slightly to not positive correlated to the TOC contents, reflecting by the indexes of BioBa (R2 = 0.3766), P/Ti (R2 = 0.1836) and P/Al (0.1675). However, the other indicators are not obviously correlated to the TOC contents.
Above all, the controlling factors of OM enrichment varied during the three sedimentary periods (Table 1). This may be affected by the different sedimentary mircrofacies of the shale in different depth. Such as the geochemical features of shale from the “Te” part of turbidite may be closely related to the terrigenous clastic input, while the one from deep lake/marine should be much more affected by the paleoproductivity, redox condition and paleosalinity condition. In addition, the paleoclimate didn’t change much during Laiyang period. Thus, the evolution of the rift basin which controlled the sedimentary facies should be the primitive factor controlling the OM-enrichment of shale.
Conclusion
The TOC contents of shale samples of Laiyang group from the well LK-1 are generally higher than 1 wt%, indicating that they are good source rocks. The factors controlling OM-enrichment varied in different sedimentary period of Laiyang period, recorded by the geochemical features of the shale samples. In the early period of rifting evolution (the stage 1 and the early stage 2), paleoproductivity is the major factor of OM-enrichment. The role of redox condition and terrigenous clastic input became more and more important. When the rift evolution entered the late period (the late stage 2 and the stage 3), terrigenous clastic input is the most important factor controlling the OM-enrichment.(Mcmanus et al., 1998)., (Raiswell et al., 1988)
Data Availability Statement
The original contributions presented in the study are included in the article/Supplementary Material, further inquiries can be directed to the corresponding authors.
Author Contributions
All authors listed have made a substantial, direct, and intellectual contribution to the work and approved it for publication.
Funding
This study was supported by the Project of Department of Science and Technology of Sinopec (No. P20028) and the Fundamental Research Funds for the Central Universities grant (No. 18CX06019A).
Conflict of Interest
Author HZ is employed by the company Xianhe Oil Production Plant of Shengli Oilfield Company, SINOPEC; and Author ML is employed by the company Shengli Oil Production Plant of Shengli Oilfield Company, SINOPEC.
The remaining authors declare that the research was conducted in the absence of any commercial or financial relationships that could be construed as a potential conflict of interest.
Publisher’s Note
All claims expressed in this article are solely those of the authors and do not necessarily represent those of their affiliated organizations, or those of the publisher, the editors and the reviewers. Any product that may be evaluated in this article, or claim that may be made by its manufacturer, is not guaranteed or endorsed by the publisher.
Supplementary Material
The Supplementary Material for this article can be found online at: https://www.frontiersin.org/articles/10.3389/feart.2021.808916/full#supplementary-material
References
Abanda, P. A., and Hannigan, R. E. (2006). Effect of Diagenesis on Trace Element Partitioning in Shales. Chem. Geology. 230 (1-2), 42–59. doi:10.1016/j.chemgeo.2005.11.011
Algeo, T. J., Kuwahara, K., Sano, H., Bates, S., Lyons, T., Elswick, E., et al. (2011). Spatial Variation in Sediment Fluxes, Redox Conditions, and Productivity in the Permian–Triassic Panthalassic Ocean. Palaeogeogr. Palaeoclimatol. Palaeoecol. 308 (1-2), 65–83. doi:10.1016/j.palaeo.2010.07.007
Arthur, M. A., Dean, W. E., and Laarkamp, K. (1998). Organic Carbon Accumulation and Preservation in Surface Sediments on the Peru Margin. Chem. Geol. 152 (3), 273–286. doi:10.1016/s0009-2541(98)00120-x
Arthur, M. A., and Sageman, B. B. (1994). Marine Black Shales:Depositional Mechanisms and Environments of Ancient Deposits. Rev. Earth Plan. Sci. 22, 499–551. doi:10.1146/annurev.ea.22.050194.002435
De Baar, H. J. W., Bacon, M. P., Brewer, P. G., and Bruland, K. W. (1985). Rare Earth Elements in the Pacific and Atlantic Oceans. Geochimica Et Cosmochimica Acta 49 (9), 1943–1959. doi:10.1016/0016-7037(85)90089-4
Gallego-Torres, D., Martínez-Ruiz, F., Paytan, A., Jiménez-Espejo, F. J., and Ortega-Huertas, M. (2007). Pliocene-Holocene Evolution of Depositional Conditions in the Eastern Mediterranean: Role of Anoxia vs. Productivity at Time of Sapropel Deposition. Palaeogeogr. Palaeoclimatol. Palaeoecol. 246 (2), 424–439. doi:10.1016/j.palaeo.2006.10.008
German, C. R., and Elderfield, H. (1990). Application of the Ce Anomaly as a Paleoredox Indicator: The Ground Rules. Paleoceanography 5 (5), 823–833. doi:10.1029/pa005i005p00823
He, C., Ji, L., Su, A., Wu, Y., Zhang, M., Zhou, S., et al. (2019). Source-rock Evaluation and Depositional Environment of Black Shales in the Triassic Yanchang Formation, Southern Ordos Basin, north-central China. J. Pet. Sci. Eng. 173, 899–911. doi:10.1016/j.petrol.2018.10.089
Jones, B., and Manning, D. A. C. (1994). Comparison of Geochemical Indices Used for the Interpretation of Palaeoredox Conditions in Ancient Mudstones. Chem. Geology. 111, 111–129. doi:10.1016/0009-2541(94)90085-x
Kimura, T. (1998). Relationships between Inorganic Elements and Minerals in Coals from the Ashibetsu District, Ishikari Coal Field. Jpn. Fuel Process. Technol. 56 (1-2), 1–19. doi:10.1016/s0378-3820(97)00089-1
Leventhal, J. R., and Leventhal, J. S. (1992). Relationship between Inferred Redox Potential of the Depositional Environment and Geochemistry of the Upper Pennsylvanian (Missourian) Stark Shale Member of the Dennis Limestone, Wabaunsee County, Kansas, U.S.A. Chem. Geology. 99 (1-3), 65–82. doi:10.1016/0009-2541(92)90031-Y
Liang, Q., Jing, H., and Gregoire, D. C. (2000). Determination of Trace Elements in Granites by Inductively Coupled Plasma Mass Spectrometry. Talanta 51 (3), 507–513. doi:10.1016/s0039-9140(99)00318-5
Liang, Z., Zhou, Y., and van Loon, A. J. (2018). Soft-sediment Deformation Structures Induced by Rapid Sedimentation in Early Cretaceous Turbidites, Lingshan Island, Eastern China. Can. J. Earth Sci. 55 (2), 118–129. doi:10.1139/cjes-2017-0106
Liu, B., Wang, H., Fu, X., Bai, Y., Bai, L., Jia, M., et al. (2019). Lithofacies and Depositional Setting of a Highly Prospective Lacustrine Shale Oil Succession from the Upper Cretaceous Qingshankou Formation in the Gulong Sag, Northern Songliao Basin, Northeast China. Bulletin 103 (2), 405–432. doi:10.1306/08031817416
Lu, H. B., Wang, J., and Zhang, H. C. (2011). Discovery of the Late Mesozoic Slump Beds in Lingshan Island, Shandong, and a Pilot Research on the Regional Tectonics. Acta Geologica Sinica 85 (6), 938–946. (in Chinese with English abstract).
Mclennan, S. M., Hemming, S., Mcdaniel, D. K., and Hanson, G. N. (1993). “Geochemical Approaches to Sedimentation, Provenance, and Tectonics,” in Processes Controlling the Composition of Clastic Sediments (Boulder, Colorado: Geological Society of America Special Paper), 284. doi:10.1130/spe284-p21
Mcmanus, J., Berelson, W. M., Klinkhammer, G. P., Johnson, K. S., Coale, K. H., Anderson, R. F., et al. (1998). Geochemistry of Barium in marine Sediments: Implications for its Use as a Paleoproxy. Geochimica Et Cosmochimica Acta 62 (21-22), 3453–3473. doi:10.1016/s0016-7037(98)00248-8
Mort, H., Jacquat, O., Adatte, T., Steinmann, P., Föllmi, K., Matera, V., et al. (2007). The Cenomanian/Turonian Anoxic Event at the Bonarelli Level in Italy and Spain: Enhanced Productivity And/or Better Preservation? Cretaceous Res. 28 (4), 597–612. doi:10.1016/j.cretres.2006.09.003
Murphy, J. B., Strachan, R. A., Nance, R. D., Parker, K. D., and Fowler, M. B. (2000). Proto-Avalonia: A 1.2-1.0 Ga Tectonothermal Event and Constraints for the Evolution of Rodinia. Geology 28 (12), 1071–1074. doi:10.1130/0091-7613(2000)028<1071:paagte>2.3.co;2
Murray, R. W., and Leinen, M. (1996). Scavenged Excess Aluminum and its Relationship to Bulk Titanium in Biogenic Sediment from the central Equatorial Pacific Ocean. Geochimica Et Cosmochimica Acta 60 (20), 3869–3878. doi:10.1016/0016-7037(96)00236-0
Paytan, A., and Griffith, E. M. (2007). Marine Barite: Recorder of Variations in Ocean export Productivity. Deep Sea Res. Part. Top. Stud. Oceanogr. 54 (5-7), 687–705. doi:10.1016/j.dsr2.2007.01.007
Raiswell, R., Buckley, F., Berner, R., and Anderson, T. (1988). Degree of Pyritization of Iron as a Paleoenvironmental Indicator of Bottom-Water Oxygenation[J]. J. Sediment. Petrology 58 (5), 812–819.
Raiswell, R., Buckley, F., Berner, R. A., and Anderson, T. F. (1988). Degree of Pyritization of Iron as a Paleoenvironmental Indicator of Bottom-Water Oxygenation. J. Sediment. Petrology 58 (5), 812–819. doi:10.1306/212f8e72-2b24-11d7-8648000102c1865d
Sageman, B. B., Murphy, A. E., Werne, J. P., Straeten, C. A. V., Hollander, D. J., and Lyons, T. W. (2003). A Tale of Shales: the Relative Roles of Production, Decomposition, and Dilution in the Accumulation of Organic-Rich Strata, Middle-Upper Devonian, Appalachian basin. Chem. Geol. 195 (1-4), 229–273. doi:10.1016/s0009-2541(02)00397-2
Shen, J., Schoepfer, S. D., Feng, Q., Zhou, L., Yu, J., Song, H., et al. (2015). Marine Productivity Changes during the End-Permian Crisis and Early Triassic Recovery. Earth-Science Rev. 149, 136–162. doi:10.1016/j.earscirev.2014.11.002
Sholkovitz, E. R., Landing, W. M., and Lewis, B. L. (1994). Ocean Particle Chemistry: The Fractionation of Rare Earth Elements between Suspended Particles and Seawater. Geochimica Et Cosmochimica Acta 58 (6), 1567–1579. doi:10.1016/0016-7037(94)90559-2
Tang, S. H., Xi, Z. D., Zhu, W. P., Li, Y., and Yan, X. L. (2020). Factors Controlling Organic Matter Accumulation in the Upper Ordovician Wufeng Shale from Upper Yangtze Platform. J. China coal Soc. 45 (1), 285–295. (in Chinese with English abstract). doi:10.1016/j.marpetgeo.2020.104597
Taylor, S. R., and Mclennan, S. M. (1985). “The Continental Crust: its Composition and Evolution,” in An Examination of the Geochemical Record Preserved in Sedimentary Rocks (Hoboken, New Jersey, US: Blackwell Scientific Pub).
Tribovillard, N., Algeo, T. J., Lyons, T., and Riboulleau, A. (2006). Trace Metals as Paleoredox and Paleoproductivity Proxies: An Update. Chem. Geol. 232 (1-2), 12–32. doi:10.1016/j.chemgeo.2006.02.012
Verma, S. P., and Armstrong-Altrin, J. S. (2013). New Multi-Dimensional Diagrams for Tectonic Discrimination of Siliciclastic Sediments and Their Application to Precambrian Basins. Chem. Geology. 355, 117–133. doi:10.1016/j.chemgeo.2013.07.014
Wang, J., Chang, S.-C., Wang, K.-L., Lu, H.-B., and Zhang, H.-C. (2015). Geochronology and Geochemistry of Early Cretaceous Igneous Units from the central Sulu Orogenic belt: Evidence for Crustal Delamination during a Shift in the Regional Tectonic Regime. J. Asian Earth Sci. 112, 49–59. doi:10.1016/j.jseaes.2015.09.009
Wang, M., Chen, Y., Bain, W. M., Song, G., Liu, K., Zhou, Z., et al. 2020). Direct Evidence for Fluid Overpressure during Hydrocarbon Generation and Expulsion from Organic-Rich Shales. Geology, 48(4), 374–378.doi:10.1130/g46650.1
Wang, X. X., Zheng, R. C., Yan, G. Q., Wang, C. Y., and Chen, H. R. (2014). The Mudstone Sedimentary Environment and Provenance Analysis Based on the Geochemical Evidence of Rare Earth Elements: Take Chang 9 Oil-Bearing Layer in Longdong Area of Ordos Basin as an Example. Nat. Gas Geosci. 25 (9), 1387–1394. (in Chinese with English abstract). doi:10.11764/j.issn.1672-1926.2014.09.1387
Xia, W., Yu, B. S., and Sun, M. D. (2015). Depositional Setting and Enrichment Mechanism of Organic Matter of the Black Shales of Niutitang Formation at the Bottom of Lower Cambrian, in Well Yuke 1, Southeast Chongqing. J. Mineral. Petrol. 35 (2), 70–80. (in Chinese with English abstract). doi:10.19719/j.cnki.1001-6872.2015.02.009
Yan, C. N., Jin, Z. J., Zhao, J. H., Du, W., and Liu, Q. Y. (2018). Influence of Sedimentary Environment on Organic Matter Enrichment in Shale: A Case Study of the Wufeng and Longmaxi Formations of the Sichuan Basin. China.
Yang, R., Fan, A., Han, Z., and van Loon, A. J. T. (2017). A Marine or Continental Nature of the Deltas in the Early Cretaceous Lingshandao Formation-Evidences from Trace Elements. Acta Geologica Sinica - English Edition 91 (1), 367–368. doi:10.1111/1755-6724.13094
Yang, R., and van Loon, A. J. (2016). Early Cretaceous Slumps and Turbidites with peculiar Soft-Sediment Deformation Structures on Lingshan Island (Qingdao, China) Indicating a Tensional Tectonic Regime. J. Asian Earth Sci. 129, 206–219. doi:10.1016/j.jseaes.2016.08.014
Yang, T., Cao, Y., Friis, H., Liu, K., and Wang, Y. (2018). Origin and Evolution Processes of Hybrid Event Beds in the Lower Cretaceous of the Lingshan Island, Eastern China. Aust. J. Earth Sci. 65 (4), 517–534. doi:10.1080/08120099.2018.1433236
Young, G. M., and Nesbitt, H. W. (1998). Processes Controlling the Distribution of Ti and Al in Weathering Profiles, Siliciclastic Sediments and Sedimentary Rocks. J. Sediment. Res. 68 (3), 448–455. doi:10.2110/jsr.68.448
Zhang, S. S. (1988). Study on the Ratio of Mg and Al in Sedimentary Layer and its Application. Bull. Mineralogy,Petrology Geochem. 1(02), 112–113. (in Chinese with English abstract).
Zhong, J. H. (2012). Lingshan Island Mesozoic Sedimentary Rocks in Deep Water Far Source Turbidite or continental delta Deposits? A Discussion with Professor Lu Hongbo. Geol. Rev. 58 (6), 1180–1182. (in Chinese with English abstract). doi:10.1016/j.jop.2017.02.002
Zhou, Y.-Q., Peng, T.-M., Zhou, T.-F., Zhang, Z.-K., Tian, H., Liang, W.-D., et al. (2017). Soft-sediment Deformation Structures Related to Volcanic Earthquakes of the Lower Cretaceous Qingshan Group in Lingshan Island, Shandong Province, East China. J. Palaeogeogr. 6 (2), 162–181. doi:10.1016/j.jop.2017.02.002
Zhou, Y. Q., Zhou, T. F., Ma, C. Q., Zhang, Z. K., Dong, S. H., Gu, Y. J., et al. (2018). Transcrustal Magmatic System of Early Cretaceous (Qingshan Stage) in Eastern Shandong and the Basin Formation Related to “Thermal Upwelling-Detachment”. Earth Sci. 43 (10), 3373–3390. (in Chinese with English abstract). doi:10.3799/dqkx.2018.998
Keywords: Lingshan Island, organic matter enrichment, shale, Cretaceous, depositional setting
Citation: Zhou T, Zhou Y, Zhao H, Li M and Mu H (2022) Depositional Setting and Enrichment Mechanism of Organic Matter of Lower Cretaceous Shale in Ri-Qing-Wei Basin in the Central Sulu Orogenic Belt. Front. Earth Sci. 9:808916. doi: 10.3389/feart.2021.808916
Received: 04 November 2021; Accepted: 29 November 2021;
Published: 12 January 2022.
Edited by:
Yanyong Wang, Chengdu University of Technology, ChinaReviewed by:
Huiwen Yue, Helmholtz Association of German Research Centres (HZ), GermanyGuanhua Li, University of Alberta, Canada
Liang Zhao, Hebei GEO University, China
Copyright © 2022 Zhou, Zhou, Zhao, Li and Mu. This is an open-access article distributed under the terms of the Creative Commons Attribution License (CC BY). The use, distribution or reproduction in other forums is permitted, provided the original author(s) and the copyright owner(s) are credited and that the original publication in this journal is cited, in accordance with accepted academic practice. No use, distribution or reproduction is permitted which does not comply with these terms.
*Correspondence: Tengfei Zhou, emhvdXRmZ2Vvc2NpZW5jZUAxMjYuY29t; Yaoqi Zhou, emhvdXlxQHVwYy5lZHUuY24=