- 1Department of Oceanography, National Sun Yat-sen University, Kaohsiung, Taiwan
- 2Department of Geosciences, National Taiwan University, Taipei, Taiwan
- 3Research Center for Future Earth, National Taiwan University, Taipei, Taiwan
The declining trend of the δ13C of tropical corals over the last century was about −0.01‰ year−1, according to global coral records. The decrease was attributable to the significant input of anthropogenic CO2 (13C Suess effect) to the atmosphere. Previous studies of δ13C in corals suggested that the signal of the anthropogenic carbon in the Pacific and Indian Oceans were weaker than that in the Atlantic Ocean. However, biases relating to environments in which corals grew caused concerns. To investigate the anthropogenic carbon signal in the Western Pacific, foraminiferal records in a suite of 13 box cores with good age control were obtained from the continental slope off southwestern Taiwan between 2004 and 2006. δ18O values of planktonic foraminifera (Globigerinoides sacculifer or so-called Trilobatus sacculifer) in collected cores were relatively stable at −2.5‰ to −2‰ in the last century, but foraminiferal δ13C had a gradual secular decline after the 1900s. The decline trend of δ13C began to intensify after the 1960s, and its rate was similar to that observed in the Atlantic. Similar decline trends of δ13C were also found in coral records at regions where the human activity is high (Liuqiu) and low (Dongsha). Our findings indicate that the anthropogenic carbon signal in the Western Pacific was not weaker than that recorded in the Atlantic, and the nearshore sediment can supplement the lack of δ13C records in corals, which are deficient when the environment is not suitable to grow.
Introduction
Large quantities of CO2 have been emitted into the atmosphere since the late 18th century as a result of human activities like fossil fuel burning, deforestation, and cement manufacturing (Crutzen and Stoermer, 2000). The input of the anthropogenic carbon not only altered lateral carbon fluxes from the land to the ocean but also influenced the climate on continental scales (Khatiwala et al., 2009; Höök and Tang, 2013; Regnier et al., 2013; Hansen and Stone, 2016).
The pathway of anthropogenic CO2 entering the ocean is through gas exchanges across the air–sea interface (Suess, 1955; Keeling, 1979; Broecker and Maier-Reimer, 1992; Quay et al., 1992). The perturbation of carbon fluxes from the land to the ocean was calculated to be about 1.0 PgC year−1 since the Industrial Revolution (Regnier et al., 2013), and the uptake of anthropogenic CO2 by oceans was estimated up to 70% on the time scales of thousands of years (Archer et al., 1998; Raven and Falkowski, 1999). However, the current oceanic capacity only accounts for around one-third of the value because of the slow mixing rate (Field and Raupach, 2004; Sabine et al., 2004). Approximately 30% of the anthropogenic CO2 was found at water depths shallower than 200 m (Sabine et al., 2004).
The penetration and distribution of the anthropogenic CO2 concentration in oceans have been investigated by various instruments and proxies, among which carbon isotopes in sedimentary records are conventionally applied (Sabine et al., 2004; Khatiwala et al., 2009; Höök and Tang, 2013; Regnier et al., 2013). Fossil fuels stored in geological reservoirs contain high 12C (lighter carbon isotope than 13C) because the buried C3 plants discriminated against 13C in the photosynthesis occurring hundreds of millions to tens of millions of years ago (Farquhar et al., 1989; Graven et al., 2020). As a result, the combustion of fossil fuels releases the lighter carbon isotope and causes the 12C concentration to increase faster than 13C in the atmosphere. Consequently, the carbon isotopic composition of 13C (δ13C: the ratio of 13C/12C) depletes both in atmospheric and oceanic environments. The 13C depletion associated with anthropogenic combustions, which induce CO2 emission, is signified as the Suess effect (Keeling, 1979).
The 13C Suess effect is not only archived in the atmosphere (Suess, 1955; Friedli et al., 1986) but also imprinted in marine realms (Quay et al., 1992; Swart et al., 2010; Black et al., 2011; Mellon et al., 2019; Simon et al., 2020). The significant decline trend of the δ13C was measured in corals or sclerosponges pervasively distributed over the Atlantic, Indian, and Pacific Oceans (Damon et al., 1978; Nozaki et al., 1978; Druffel and Benavides, 1986; Wei et al., 2009; Swart et al., 2010). The decline rate of the δ13C in the Atlantic Ocean was found to be greater than that in the Indian and the Pacific Oceans because of physiological activities of corals, local bathymetric conditions, or different buffer capacities in marine regimes (Takahashi et al., 1993; Sabine et al., 2004; Swart et al., 2010). Therefore, there are constraints to demonstrate the temporal variability of stable carbon isotopes by using coral records.
The calcium carbonate deposits in marine sediments (e.g., foraminifera) are regarded as another potential research material due to the wide coverage without constraints (e.g., water depth, turbidity; Mcconnaughey, 1989; Grottoli and Wellington, 1999; Linsley et al., 2019). The foraminiferal δ13C records are often used to extract the environmental and metabolic information, though the offset exists because of physical and biogeological processes (Spero and Williams, 1988; Jonkers et al., 2013; Gaskell and Hull, 2019). For example, the fossil benthic foraminifera had indicated the negative excursion of the long-term δ13C variability occurring within this century, which was related to the anthropogenic CO2 emission (Al-Rousan et al., 2004; Mellon et al., 2019).
In addition to carbon isotopes, the composition of oxygen isotopes (δ18O: the ratio of 18O/16O) in the foraminiferal shell are widely used to estimate changes in the water temperature or glacier volumes (Shackleton, 1967; Thunell et al., 1999). In tropical and subtropical oceans, δ18O records in foraminifera and corals have been applied to reconstruct the history of the sea surface temperature and the sea surface salinity over the century scale (Qiu et al., 2014; Watanabe et al., 2014; Raza et al., 2017). The foraminiferal δ18O is also conventionally used to reflect the δ18O in the ambient seawater (Katz et al., 2010). Tao et al. (2013) indicated that the varying δ18O represented variabilities in hydrographic conditions such as the strength of the local upwelling and the freshwater input.
However, related studies are few due to low resolutions inhibited by the sedimentation rate in the open ocean. Furthermore, the near-shore realm with high sediment rates is usually intrigued by bioturbations, which can only be exempted in anoxic bottom conditions (Schimmelmann et al., 1990; Kennedy and Brassell, 1992; Black et al., 2007; Black et al., 2011). Therefore, the isotopic signal in sediments is crucial to be further studied. A suite of short box cores was collected from the continental slope off southwestern Taiwan in this study. Downcore sediment records were constrained by fallout radionuclides including 210Pb and 137Cs. The activity of radionuclides shows fairly constant hemipelagic accumulations and indicates that the near-shore realm was stable with high sedimentation rates. Therefore, our sediment cores provide good quality records in δ13C and δ18O to compare with coral isotope records collected in different areas such as Liuqiu and Dongsha. Liuqiu is located near our sampling sites, and Dongsha is around 424 km away from sampling sites in the northern South China Sea (SCS). Such precious materials provide insight information regarding the anthropogenic imprint in the Western Pacific for the last century.
Materials and methods
Sediment cores
FATES (Fate of Terrestrial/Nonterrestrial Sediments) Program was conducted to understand processes and responses of substances from the land to the marine sink. A suite of 13 short box cores were collected between 2004 and 2006 from the continental slope off SW Taiwan, northern SCS (Table 1; (Huh et al., 2009; Liu et al., 2009). Locations of sediment cores are shown on the bathymetric map (Figure 1), and information regarding geographic coordinates, water depths, and core lengths are listed in Table 1. Sediments were sampled at 5-cm intervals throughout sediment cores for foraminiferal isotope analyses. Planktonic shell sizes used for isotopic measurements were constrained by the sieving mash size of 300–355 μm for Globigerinoides sacculifer (so-called Trilobatus sacculifer) to minimize ontogenetic effects. Benthic foraminiferal shells of Uvigerina sp. were picked from a fraction greater than 150 μm. Stable isotopic analyses were done on groups of 10 specimens or less for each sample. The picked foraminiferal specimens were cleaned thoroughly in an ultrasonic bath with methanol to remove adhering fine particles, followed by soaking in sodium hypochlorite (NaOCl, 5%) at room temperature for more than 24 h to further remove any fine organic particles. Cleaning with deionized distilled water followed, and samples were then oven dried at 50°C. Stable isotope analyses for specimens were measured at the Stable Isotope Laboratory, National Taiwan University, Taiwan, following standard procedures with a precision better than 0.07‰ for δ18O and 0.04‰ for δ13C.
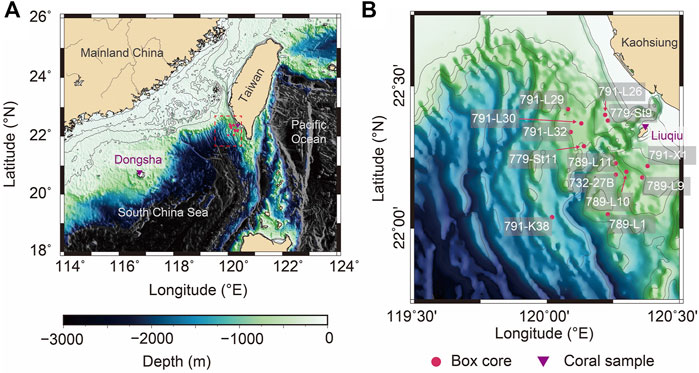
FIGURE 1. (A) The location of Taiwan and Dongsha in the Western Pacific. Red circles represent the sampling locations of the box core, and purple triangles represent the sampling locations of the coral core. (B) is the enlarged segment of (A) marked with the red dash square and shows the location and label of the collected box cores.
Samples used for the radionuclide isotope analysis were sliced into sections with 2-cm intervals from the top of the sediment core. Each section was sealed in a plastic bag and stored in the refrigerator at 4°C before being freeze dried. After the sample was dried, the water content in the sediment sample was determined. Dried samples were then transferred to plastic jars (inside diameter is 8.5 cm; height is 7.5 cm) for nondestructive gamma spectrometric assay of radionuclides. Analyzed radionuclides include 210Pb, 214Pb, and 137Cs, which were used as sediment chronometers (Huh et al., 2009) and were calculated according to a salt-free dry weight. The digital gamma-ray spectrometer was connected to HPGe detectors for counting radionuclides simultaneously based on photon peaks centering at 46.52 (210Pb), 351.99 (214Pb), and 661.62 (137Cs) keV, respectively. Afterward, the counting results were analyzed with GammaVision 32 software (Su and Huh, 2002; Huh et al., 2006; Huh et al., 2009).
214Pb is the precursor of 210Pb and used as the index of supported 210Pb (210Pbsup). An excess of 210Pb (210Pbex) can be obtained by subtracting the 214Pb active concentration from the recorded 210Pb (210Pbex = 210Pbmea − 210Pbsup; (Huh et al., 2006; Huh et al., 2009). 137Cs could be measured simultaneously with 210Pb by nondestructive gamma spectrometry, but it took a long time to obtain 137Cs data due to the lower activity. Therefore, only a portion of the cores were analyzed for 137Cs to constrain the 210Pb chronology. More details of the gamma spectrometry analysis are described in Huh et al. (2009).
Sedimentation rates derived from fallout radionuclides (210Pb and 137Cs) indicate constant hemipelagic accumulation, which implies that collected cores are suitable for reconstructing the recent paleoenvironment (Huh et al., 2009). The two out of 92 sediment cores (789-L1 and 791-K38) were selected as representatives in our study because of ample planktonic and benthic foraminiferal shells and the well age dating of box cores.
Ages of core samples at different depths were estimated by the sedimentation rate of each core. The linear regression of each core between δ13C values of foraminifera shells and ages of samples was performed by the least square method using FITLM function provided by MATLAB R2020 software (Street et al., 1988). The two datasets, age later than 1900s and later than 1960s, were screened for regression analyses to compare with published records. Results of regression are listed in Tables 2 and 3 (e.g., slope, intercept, r squared, p value, and number of samples).
Coral skeletal records from Dongsha (Ren et al., 2017) and Liuqiu were also included to compare the anthropogenic effects on the two reef sites (Figure 1). The coral skeletal core collected around Liuqiu (120.36°E, 22.35°N) was drilled from a living Porites sp. colony at a depth of about 10 m on June 29, 2017. The core was cut into two slabs, then scanned by x-rays to identify its maximum growth axis, and then subsampled with an automated three-axis saw machine. Skeletal pieces (11 mm × 1 mm × 2.5 mm) with about monthly resolution were cut along the maximum growth axis and crushed into powder. About 5% of the powder is used for δ13C and δ18O analyses with isotope ratio mass spectrometer in the Stable Isotope Laboratory at the Department of Earth Science in National Normal University. The remaining powder is saved for other analysis. Combined with annual growth bands in x-ray images, skeletal δ18O was compared with the extended reconstructed sea surface temperature (ERSSTv5) of the NOAA to establish the age model.
Results and discussion
The chronology of sediment cores
In general, 210Pb in marine sediments is influenced by two factors: 1) the decay of 226Ra of marine sediments, 210Pbsup, and 2) the decay of atmospheric 222Rn, which deposits into the ocean and is preserved in marine sediments by the removal of particles from the water column, expressed as 210Pbex (Hung and Chung, 1998). The depletion of 210Pbex causes the 210Pb activity to decrease exponentially or quasi-exponentially along the downcore until total 210Pb activity in the sediment is equal to 210Pbsup. In short, the 210Pbex profile represents the stable deposition if 210Pbex decays exponentially with the depth and can be explained by the steady-state advection-decay model (Huh et al., 2009).
Assuming the amount of the sediment and 210Pb flux at a given site are constant, and the mixing in the sediment is ignored. The downcore of 210Pbex profile is, thus, invariable with time and described by:
where C0 and Cz are 210Pbex at the sediment–water interface and depth Z, respectively. λ is the decay constant (0.0311 year−1) of 210Pb, and S is the sedimentation rate (cm year−1). Based on the regression analysis of 210Pbex (Eq. 1), the linear sedimentation rate can be calculated (Holmes, 1998; Lewis et al., 2002; Huh et al., 2009).
Most of the 210Pbex in the collected sediment cores decreased exponentially downcore with a few layers having low 210Pbex values (Figure 2). The characteristics of 210Pbex profiles were separated into Type I (sediment deposition in nonreworked settings; e.g., 789-L1), Type III (the existence of a physically and/or biological reworked surficial layer; e.g., 779-St11), and Type V (influenced by strong episodic events; e.g., 779-St9) according to the classification described by Xu et al. (2015). After excluding bio-interferences and event layers, the sedimentation rate and the preliminary age model of the 13 sediment cores were estimated.
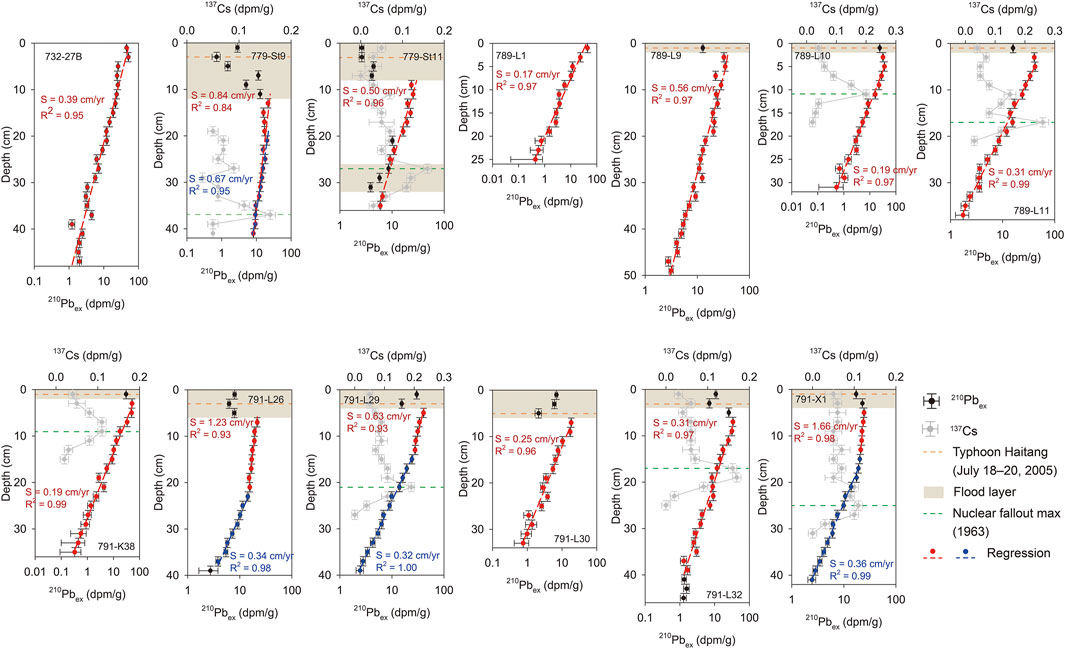
FIGURE 2. The 210Pbex profile in each sediment core (black dots) shows a good fit (R2 > 0.93) with a steady-state advection-decay model. The red and blue dots represent the selected fitting points, and the dashed line of each color represents the corresponding fitting results. The black dots that are not marked by color are not included in the fit, usually in the flood event layer. The 137Cs profile is represented by gray dots. The control points in the age model, the maximum value of 137Cs and Typhoon Haitang, are represented by green and orange dashed lines, respectively [The data of 210Pbex and 137Cs was from Huh et al., (2009)]. Brown patches in the core top indicate the event layer (flood layer).
137Cs was introduced as the control point of the 210Pbex-derived age model in collected sediment cores. The 137Cs profile had the typical maximum radioisotope activity in the subsurface (the anthropogenic nuclide in 1963 A.D.) in which 137Cs decreased gradually upward but sharply downward (Holmes, 1998; Huh et al., 2009; Figure 2). Since the 210Pb-derived age model matches the control point indicated by 137Cs, the quality of 210Pb dating is reliable.
Additionally, the interference by the typhoon was considered as another control point of the age model in our study. For example, the intense rainfall caused flood deposits in the downstream shelf during Super Typhoon Haitang occurring on July 18–20, 2005 (Huh et al., 2009). This resulted in a low 210Pbex layer occupying on the sediment core top collected afterward, especially for cores obtained in the same year of the typhoon event (e.g., 779-St9 and 779-St11 in Figure 2). The 210Pbex profile implies the typhoon event did not interfere with the 210Pb-derived age model severely in the long-term scale because the event layer (indicated by the minimum 210Pbex value) was gradually buried by accumulating pelagic or hemipelagic sediments. Eventually, the event signal with low 210Pbex was diluted and became weaker, though the signal was still observed in some of the collected sediment cores.
Isotopes in planktonic Foraminifera
Oxygen and carbon isotopes of G. sacculifer in 13 box cores were plotted in Figure 3. Of the δ18O data, 84% ranged between −2.5‰ and −2‰ for the last 150 years from 2010 (Figure 3A). The δ13C records, however, fluctuated around 1.5‰ and started to decline after the 1900s followed by the rapid decline trend after the 1960s (Figure 3B). Both oxygen and carbon isotopic compositions from coretops were consistent with modern shells collected by sediment traps in this regime (Lin, 2014). To decipher historical isotopic changes, two out of 13 cores, 789-L1 and 791-K38, were selected as the representatives. The isotopes generated from planktonic foraminifera G. sacculifer and benthic foraminifera Uvigerina sp. are shown in Figures 4A–D. The planktonic isotopes of the other 11 cores are shown in Supplementary Figure S1.
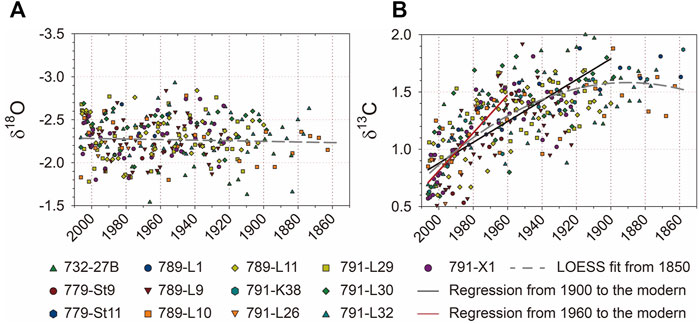
FIGURE 3. δ18O and δ13C derived from planktonic foraminifera G. sacculifer of 13 box cores are shown in (A) and (B), respectively. LOESS fit from 1850 to the present was plotted as the gray line. Linear regressions of δ13C from 1900 to the present and from 1960 to the present were plotted as blue and red lines, respectively. Different symbols with colors indicate box cores collected near Liuqiu.
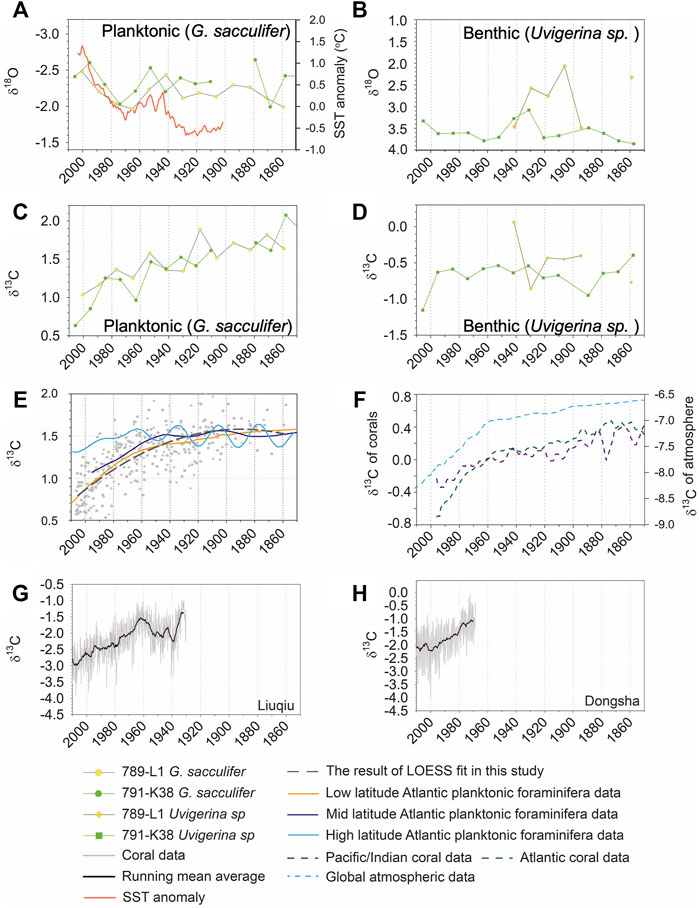
FIGURE 4. (A) The δ18O of G. sacculifer in core 789-L1 and 791-K38 combine with the anomaly of sea surface temperature around Taiwan (adopted from Shiu et al., 2009). (B) The δ18O of Uvigerina sp. in core 789-L1 and 791-K38. (C) The δ13C of G. sacculifer in core 789-L1 and 791-K38. (D) The δ13C of Uvigerina sp. in core 789-L1 and 791-K38. (E) The light gray symbol shows all the δ13C of planktonic foraminifers in this study, and the LOESS fit represent the situation in the mid-low latitude Pacific Ocean. The orange, purple, and light blue lines represent the situation of planktonic foraminifera in the Atlantic Ocean at low, mid, and high latitudes, respectively (adopted from Black et al., 2011; Mellon et al., 2019; Simon et al., 2020). The δ13C axis of these three data sets was shifted to facilitate comparison with our data. (F) The δ13C of corals in the Atlantic and the Pacific/Indian Oceans (green and purple lines; adopted from Swart et al., 2010), and the global δ13C of atmosphere (light blue line; Graven et al., 2017). (G) The δ13C of coral record from Liuqiu. (H) The δ13C of coral record from Dongsha. The gray line represents the raw data, and the black line is the 4-year running mean average.
The δ18O variability in core samples
The range and fluctuation pattern of planktonic δ18O of two selected downcore records were very similar, particularly the broad δ18O-enriched interval between 1950 and 1990 (Figure 4A). The deceasing of δ18O occurring between 1950 and 1970 followed by a rising trend until 1990 was also observed in local meteorological data (Shiu et al., 2009). However, the planktonic δ18O was consistent overall.
Unlike the relatively continuous planktonic record, the benthic δ18O for core 789-L1 was discrete due to insufficient foraminiferal shells at specific layers of the sediment core (Figure 4B). Despite the limited number of measured shells, δ18O values of Uvigerina sp. from core 789-L1 were generally lighter than that of core 791-K38. Mulitza et al. (2003) has described that the increase in δ18O is induced by the temperature drop, regardless of salinity effect. Therefore, the offset in benthic δ18O records (2.2‰ for 789-L1 vs. 2.9‰ for 791-K38 in average between 1910–1940) could be attributed to the different water temperatures between two coring sites (5.51°C at 911 m for 789-L1 vs. 3.45°C at 1,260 m for 791-K38 according to the hydrographic data). However, the variability of the regional upwelling should be another potential factor to change benthic δ18O (Wang et al., 2008).
Generally speaking, the planktonic δ18O corresponded well with the change in the local surface temperature, and benthic δ18O values were controlled by the temperature gradient at the sampled water depth or the regional ocean circulation. There is no further evidence indicating that human activities influenced δ18O values in marine sediments in the nearshore realm.
The δ13C trend in core samples and anthropogenic carbon effects
The carbon isotope composition of G. sacculifer of two selected cores are shown in Figure 4C. Unlike the coherent pattern in planktonic δ18O records (Figure 4A), the time-series of planktonic δ13C showed a 1‰–1.5‰ decline trend for the last century. Particularly, the decline trend since 1960 is a pervasive feature showing in all collected sediment cores (Figure 3B), which also followed the depletion trend of δ13C in the atmospheric CO2 (Figure 4H). Since the planktonic δ18O did not present a corresponding decline (or incline) trend during the same period (Figures 3A and 4A), the depletion of the planktonic δ13C was not attributed to the temperature change (Goericke and Fry, 1994; Dixit et al., 2015). The results highlight the creditability of foraminiferal records for identifying the 13C Suess effect in the nearshore off southwestern Taiwan.
The decline trend of δ13C in the surface ocean was caused by the input of radiocarbon-dead or anthropogenic-produced 13C-depleted carbons from the atmosphere through the air–sea exchange process (Suess, 1955; Keeling, 1979; Broecker and Maier-Reimer, 1992; Quay et al., 1992). The 13C-depleted carbon has been widely reported in coral skeleton and sclerosponge (Druffel and Benavides, 1986; Swart et al., 1996a; Swart et al., 1996b; Swart et al., 2010; Al-Rousan and Felis, 2013; Hou et al., 2019; Liu et al., 2021) and planktonic foraminifera (Al-Rousan et al., 2004; Black et al., 2011; Xu et al., 2014; Mellon et al., 2019; Simon et al., 2020), while the downcore variability of the benthic δ13C was rather flat and restricted within 0.51‰–1‰ in our study area (Figure 4D). This implies that the penetration of the anthropogenic carbon only occurred in the upper water column at our study site. Although other processes could influence the δ13C of benthic foraminifera (Mccorkle et al., 1990; Schmittner et al., 2017), our results were consistent as the previous finding by Sabine et al. (2004).
The similar magnitude of planktonic δ13C decline from the 1900s were around 0.6‰ and 0.9‰ for cores 789-L1 and 791-K38, respectively (the decline rate for each core was shown as the slope in Table 2). The similar range in the decline of δ13C was first noted by corals and sclerosponges with 0.5‰ between 1850 and 1975 from Bermuda (Nozaki et al., 1978) and Jamaica (Druffel and Benavides, 1986). The global average rate of change in the coral skeletal δ13C was estimated as −0.01‰ year−1 from 1900 to 1990 based on a compilation of coral records throughout the oceans (Swart et al., 2010). However, the decline rates of δ13C in the Indian, Pacific, and Atlantic Oceans were different during 1960–1990. The rate in the Atlantic Ocean was −0.019‰ year−1, but in the Pacific and the Indian Ocean, it was around −0.007‰ year−1 (Swart et al., 2010). The differences could be caused by physiological activities of corals or bathymetric conditions, which change the δ13CDIC [dissolved inorganic carbon (DIC)] in the ambient seawater (Swart et al., 2010; Watanabe et al., 2017; Fujii et al., 2020; Simon et al., 2020).
To compare with previous coral records (Swart et al., 2010), the δ13C used in this study was analyzed by the linear regression at two corresponding periods (1900 to the present and 1960 to the present, Tables 2 and 3). More than half of the sediment cores used in this study show the statistical significance in the depletion of δ13C (p < 0.05) toward the present (Table 2). From 1900 to the present, the average decline rate of the δ13C in planktonic foraminiferal records was about 0.008‰ year−1, which was similar to the findings of the global coral. From 1960 to the present, the average decline rate of the δ13C significantly increased to 0.016‰ year−1, which was higher than published coral records in the Pacific Ocean but was close to the value of coral measurements in the Atlantic Ocean (Swart et al., 2010). Although the decline rate was different from the previous record in the Pacific Ocean, our findings suggest that the δ13C Suess effect at the surface water of the Pacific Ocean might be as strong as that of the Atlantic Ocean, which is consistent with the global estimation by Eide et al. (2017).
Although there were isotopic offsets between precipitated calcium carbonate shells and the ambient seawater, Mellon et al. (2019) have indicated that decline trends of the δ13C recorded by different species of foraminiferal were still consistent. To compare δ13C among different planktonic foraminiferal records, data published in previous studies were digitalized and plotted with the long-term variability of measured planktonic δ13C in this study (Figure 4E). Our measurement was depicted by LOESS fit (Mellon et al., 2019) and showed a similar trend to planktonic foraminiferal records in the lower latitudes of the Atlantic, both in terms of the overall decline magnitude in, and the enhanced decline rate after, the 1960s. However, there were differences in the δ13C trend found in the higher latitudes of the Atlantic. Our planktonic records showed a larger decreasing range of δ13C than that found in mid to high latitude of the Atlantic, though the rapid decline trend also occurred after the 1960s. The differences have been described by Eide et al. (2017) indicating that the 13C Suess effect in the surface seawater is relatively uniform in the North Pacific and North Atlantic but slightly lower in the mid-high latitudes. In the Atlantic, the decline range of δ13C in foraminiferal records was less than 0.3‰ (−0.007‰ year−1) in high latitude, about 0.4‰ (−0.010‰ year−1) in the middle latitude, and close to 0.7‰ (−0.018‰ year−1) in the low latitude (Black et al., 2011; Mellon et al., 2019; Simon et al., 2020). The lower 13C Suess effect occurring in the higher latitudes of the Atlantic was attributed to local ocean circulations and primary productivities (Mellon et al., 2019; Simon et al., 2020).
The collected foraminiferal and coral records in sediment cores were further compared with the coral records obtained at Liuqiu (high human impact and close to the coring sites of foraminiferal records) and Dongsha (less human impact). The δ13C trends in foraminiferal and coral records were consistent, and its decline rates were similar (Figures 4C, F–H). The slopes of the Liuqiu and Dongsha coral records after the 1960s were −0.022 and −0.031‰ year−1, respectively (Table 3), which were also higher than that in the published records of Pacific corals. Obviously, the similar δ13C trend found in foraminiferal and coral records was not influenced by the local effect since the distance between Liuqiu and Dongsha is 412 km away (Figure 1), so global 13C Suess effect is considered to explain the extensive effect on the records of these two regions. This indicates that planktonic records of sediment cores collected off southwestern Taiwan are suitable to represent the anthropogenic signal of δ13C in the last century.
Conclusion
Our results show that the δ13C of planktonic foraminifera off southwestern Taiwan in the Western Pacific has decreased by 1‰–1.5‰ over the last century. From the 1900 to the present, the decline trend was about 0.008‰ year−1, which became steeper to 0.016‰ year−1 after the 1960s. The decline trend of δ13C found in our foraminiferal samples was higher than that in previous coral records in the Pacific and consistent with that in the Atlantic coral records, which presented a similar decline trend in global atmospheric CO2. The decreasing δ13C in planktonic foraminiferal records was attributed to the additional anthropogenic CO2 input, which is regarded as the Suess effect. Such anthropogenic carbon signal was only observed in the upper water column according to the planktonic and benthic foraminiferal records. Since the variability of δ13C in foraminiferal records were highly consistent with that in coral records collected at Liuqiu and Dongsha, the SCS, our findings suggest that carbon isotopes on a centennial scale could be reconstructed in the nearshore region with well age-controlled sediment cores. Therefore, the nearshore sediment core potentially complements the lack of δ13C records in specific areas where coral growth is restricted.
Data Availability Statement
The original contributions presented in the study are included in the article/Supplementary Material. Further inquiries can be directed to the corresponding author.
Author Contributions
JL and RY performed the simulation and prepared the manuscript. HL conceived and designed the study, handled the sedimentary records, and co-wrote the manuscript. YC and R-YC-L handled the coral records in Liuqiu and Dongsha. HR contributed to the interpretation of the coral records. JTL is the leader of the FATES Program and supervised the work.
Funding
This study was funded by the Ministry of Science and Technology grants (Nos. NSC 96-2611-M-110-009 and MOST 108-2611- M-110-012).
Conflict of Interest
The authors declare that the research was conducted in the absence of any commercial or financial relationships that could be construed as a potential conflict of interest.
The handling editor declared a past co-authorship with the authors RY and JTL.
Publisher’s Note
All claims expressed in this article are solely those of the authors and do not necessarily represent those of their affiliated organizations, or those of the publisher, the editors, and the reviewers. Any product that may be evaluated in this article, or claim that may be made by its manufacturer, is not guaranteed or endorsed by the publisher.
Acknowledgments
We are grateful to Dr. Salwood Lin at the Institute of Oceanography, National Taiwan University, for collecting the sediment cores applied in this research. Bo-Shian Wang, Tsai-Luen Yu, and Chiuan-Sheng Liu sampled the Liuqui (Xiaoliuqiu) coral core. Prof. Hong-Chun Li at the Department of Geosciences, National Taiwan University, and Prof. Horng-Sheng Mii at the Department of Earth Sciences, National Taiwan Normal University, are acknowledged for their help with the stable isotope analyses. Our sincere gratitude to Tai-Chun Lin for her assistance in the laboratory.
Supplementary Material
The Supplementary Material for this article can be found online at: https://www.frontiersin.org/articles/10.3389/feart.2021.795519/full#supplementary-material
References
Al-Rousan, S., and Felis, T. (2013). Long-term Variability in the Stable Carbon Isotopic Composition of Porites Corals at the Northern Gulf of Aqaba, Red Sea. Palaeogeogr. Palaeoclimatol. Palaeoecol. 381-382, 1–14. doi:10.1016/j.palaeo.2013.03.025
Al-Rousan, S., Patzold, J. r., Al-Moghrabi, S., and Wefer, G. (2004). Invasion of Anthropogenic CO2 Recorded in Planktonic Foraminifera from the Northern Gulf of Aqaba. Int. J. Earth Sci. (Geol Rundsch) 93, 1066–1076. doi:10.1007/s00531-004-0433-4
Archer, D., Kheshgi, H., and Maier-Reimer, E. (1998). Dynamics of Fossil Fuel CO2neutralization by marine CaCO3. Glob. Biogeochem. Cycles 12, 259–276. doi:10.1029/98gb00744
Black, D. E., Abahazi, M. A., Thunell, R. C., Kaplan, A., Tappa, E. J., and Peterson, L. C. (2007). An 8-century Tropical Atlantic SST Record from the Cariaco Basin: Baseline Variability, Twentieth-century Warming, and Atlantic hurricane Frequency. Paleoceanography 22, PA4204. doi:10.1029/2007pa001427
Black, D., Thunell, R., Wejnert, K., and Astor, Y. (2011). Carbon Isotope Composition of Caribbean Sea Surface Waters: Response to the Uptake of Anthropogenic CO2. Geophys. Res. Lett. 38, L16609. doi:10.1029/2011gl048538
Broecker, W. S., and Maier-Reimer, E. (1992). The Influence of Air and Sea Exchange on the Carbon Isotope Distribution in the Sea. Glob. Biogeochem. Cycles 6, 315–320. doi:10.1029/92gb01672
Damon, P. E., Lerman, J. C., and Long, A. (1978). Temporal Fluctuations of Atmospheric 14C: Causal Factors and Implications. Annu. Rev. Earth Planet. Sci. 6, 457–494. doi:10.1146/annurev.ea.06.050178.002325
Dixit, Y., Hodell, D. A., Sinha, R., and Petrie, C. A. (2015). Oxygen Isotope Analysis of Multiple, Single Ostracod Valves as a Proxy for Combined Variability in Seasonal Temperature and lake Water Oxygen Isotopes. J. Paleolimnol 53, 35–45. doi:10.1007/s10933-014-9805-3
Druffel, E. R. M., and Benavides, L. M. (1986). Input of Excess CO2 to the Surface Ocean Based on 13C/12C Ratios in a Banded Jamaican Sclerosponge. Nature 321, 58–61. doi:10.1038/321058a0
Eide, M., Olsen, A., Ninnemann, U. S., and Eldevik, T. (2017). A Global Estimate of the Full Oceanic 13 C Suess Effect since the Preindustrial. Glob. Biogeochem. Cycles 31, 492–514. doi:10.1002/2016GB005472
Farquhar, G. D., Ehleringer, J. R., and Hubick, K. T. (1989). Carbon Isotope Discrimination and Photosynthesis. Annu. Rev. Plant Physiol. Plant Mol. Biol. 40, 503–537. doi:10.1146/annurev.pp.40.060189.002443
Field, C. B., and Raupach, M. R. (2004). The Global Carbon Cycle: Integrating Humans, Climate, and the Natural World. Washington DC: Island Press.
Friedli, H., Lötscher, H., Oeschger, H., Siegenthaler, U., and Stauffer, B. (1986). Ice Core Record of the 13C/12C Ratio of Atmospheric CO2 in the Past Two Centuries. Nature 324, 237–238. doi:10.1038/324237a0
Fujii, T., Tanaka, Y., Maki, K., Saotome, N., Morimoto, N., Watanabe, A., et al. (2020). Organic Carbon and Nitrogen Isoscapes of Reef Corals and Algal Symbionts: Relative Influences of Environmental Gradients and Heterotrophy. Microorganisms 8, 1221. doi:10.3390/microorganisms8081221
Gaskell, D. E., and Hull, P. M. (2019). Symbiont Arrangement and Metabolism Can Explain High δ13C in Eocene Planktonic Foraminifera. Geology 47, 1156–1160. doi:10.1130/g46304.1
Goericke, R., and Fry, B. (1994). Variations of marine Plankton δ13C with Latitude, Temperature, and Dissolved CO2in the World Ocean. Glob. Biogeochem. Cycles 8, 85–90. doi:10.1029/93gb03272
Graven, H., Keeling, R. F., and Rogelj, J. (2020). Changes to Carbon Isotopes in Atmospheric CO 2 over the Industrial Era and into the Future. Glob. Biogeochem. Cycles 34, e2019GB006170. doi:10.1029/2019gb006170
Graven, H., Allison, C.-E., and Etheridge, D. M. (2017). Compiled Records of Carbon Isotopes in Atmospheric CO2 for Historical Simulations in CMIP6. Geosci. Model. Dev. 10(12), 4405–4417. doi:10.5194/gmd-10-4405-2017
Grottoli, A. G., and Wellington, G. M. (1999). Effect of Light and Zooplankton on Skeletal δ 13 C Values in the Eastern Pacific Corals Pavona Clavus and Pavona Gigantea. Coral Reefs 18, 29–41. doi:10.1007/s003380050150
Hansen, G., and Stone, D. (2016). Assessing the Observed Impact of Anthropogenic Climate Change. Nat. Clim Change 6, 532–537. doi:10.1038/nclimate2896
Holmes, C. W. (1998). “Short-lived Isotopic Chronometers: a Means of Measuring Decadal Sedimentary Dynamics,” in Fact Sheet. U.S. Geological Survey. doi:10.3133/fs07398
Höök, M., and Tang, X. (2013). Depletion of Fossil Fuels and Anthropogenic Climate Change-A Review. Energy Policy 52, 797–809. doi:10.1016/j.enpol.2012.10.046
Hou, A., Halfar, J., Adey, W., Wortmann, U. G., Zajacz, Z., Tsay, A., et al. (2019). Long-lived Coralline Alga Records Multidecadal Variability in Labrador Sea Carbon Isotopes. Chem. Geology. 526, 93–100. doi:10.1016/j.chemgeo.2018.02.026
Huh, C.-A., Lin, H.-L., Lin, S., and Huang, Y.-W. (2009). Modern Accumulation Rates and a Budget of Sediment off the Gaoping (Kaoping) River, SW Taiwan: A Tidal and Flood Dominated Depositional Environment Around a Submarine canyon. J. Mar. Syst. 76, 405–416. doi:10.1016/j.jmarsys.2007.07.009
Huh, C.-A., Su, C.-C., Wang, C.-H., Lee, S.-Y., and Lin, I.-T. (2006). Sedimentation in the Southern Okinawa Trough - Rates, Turbidites and a Sediment Budget. Mar. Geology. 231, 129–139. doi:10.1016/j.margeo.2006.05.009
Hung, G. W., and Chung, Y.-C. (1998). Particulate Fluxes, 210Pb and 210Po Measured from Sediment Trap Samples in a canyon off Northeastern Taiwan. Continental Shelf Res. 18, 1475–1491. doi:10.1016/s0278-4343(98)00032-6
Jonkers, L., Van Heuven, S., Zahn, R., and Peeters, F. J. C. (2013). Seasonal Patterns of Shell Flux, δ18O and δ13C of Small and largeN.Pachyderma(s) andG.Bulloidesin the Subpolar North Atlantic. Paleoceanography 28, 164–174. doi:10.1002/palo.20018
Katz, M. E., Cramer, B. S., Franzese, A., Honisch, B., Miller, K. G., Rosenthal, Y., et al. (2010). Traditional and Emerging Geochemical Proxies in Foraminifera. J. Foraminiferal Res. 40, 165–192. doi:10.2113/gsjfr.40.2.165
Keeling, C. D. (1979). The Suess Effect: 13Carbon-14Carbon Interrelations. Environ. Int. 2, 229–300. doi:10.1016/0160-4120(79)90005-9
Kennedy, J. A., and Brassell, S. C. (1992). Molecular Records of Twentieth-century El Niño Events in Laminated Sediments from the Santa Barbara basin. Nature 357, 62–64. doi:10.1038/357062a0
Khatiwala, S., Primeau, F., and Hall, T. (2009). Reconstruction of the History of Anthropogenic CO2 Concentrations in the Ocean. Nature 462, 346–349. doi:10.1038/nature08526
Lewis, R. C., Coale, K. H., Edwards, B. D., Marot, M., Douglas, J. N., and Burton, E. J. (2002). Accumulation Rate and Mixing of Shelf Sediments in the Monterey Bay National Marine Sanctuary. Mar. Geology. 181, 157–169. doi:10.1016/S0025-3227(01)00265-1
Lin, H.-L. (2014). The Seasonal Succession of Modern Planktonic Foraminifera: Sediment Traps Observations from Southwest Taiwan Waters. Continental Shelf Res. 84, 13–22. doi:10.1016/j.csr.2014.04.020
Linsley, B. K., Dunbar, R. B., Dassié, E. P., Tangri, N., Wu, H. C., Brenner, L. D., et al. (2019). Coral Carbon Isotope Sensitivity to Growth Rate and Water Depth with Paleo-Sea Level Implications. Nat. Commun. 10, 2056. doi:10.1038/s41467-019-10054-x
Liu, J. T., Huh, C.-A., and You, C.-F. (2009). Fate of Terrestrial Substances in the Gaoping (Kaoping) Shelf/Slope and in the Gaoping Submarine Canyon off SW Taiwan. J. Mar. Syst. 76, 367–368. doi:10.1016/j.jmarsys.2008.08.005
Liu, X., Deng, W., Cui, H., Chen, X., Cai, G., Zeng, T., et al. (2021). Change of Coral Carbon Isotopic Response to Anthropogenic Suess Effect since Around 2000s. Mar. Environ. Res. 168, 105328. doi:10.1016/j.marenvres.2021.105328
Mcconnaughey, T. (1989). 13C and 18O Isotopic Disequilibrium in Biological Carbonates: I. Patterns. Geochimica et Cosmochimica Acta 53, 151–162. doi:10.1016/0016-7037(89)90282-2
Mccorkle, D. C., Keigwin, L. D., Corliss, B. H., and Emerson, S. R. (1990). The Influence of Microhabitats on the Carbon Isotopic Composition of Deep-Sea Benthic Foraminifera. Paleoceanography 5, 161–185. doi:10.1029/PA005i002p00161
Mellon, S., Kienast, M., Algar, C., Menocal, P., Kienast, S. S., Marchitto, T. M., et al. (2019). Foraminifera Trace Anthropogenic CO 2 in the NW Atlantic by 1950. Geophys. Res. Lett. 46, 14683–14691. doi:10.1029/2019gl084965
Mulitza, S., Boltovskoy, D., Donner, B., Meggers, H., Paul, A., and Wefer, G. (2003). Temperature: δ18O Relationships of Planktonic Foraminifera Collected from Surface Waters. Palaeogeogr. Palaeoclimatol. Palaeoecol. 202(1), 143–152. doi:10.1016/S0031-0182(03)00633-3
Nozaki, Y., Rye, D. M., Turekian, K. K., and Dodge, R. E. (1978). A 200 Year Record of Carbon-13 and Carbon-14 Variations in a Bermuda Coral. Geophys. Res. Lett. 5, 825–828. doi:10.1029/GL005i010p00825
Qiu, X., Li, T., Chang, F., Nan, Q., Xiong, Z., and Sun, H. (2014). Sea Surface Temperature and Salinity Reconstruction Based on Stable Isotopes and Mg/Ca of Planktonic Foraminifera in the Western Pacific Warm Pool during the Last 155 Ka. Chin. J. Ocean. Limnol. 32, 187–200. doi:10.1007/s00343-014-3073-y
Quay, P. D., Tilbrook, B., and Wong, C. S. (1992). Oceanic Uptake of Fossil Fuel CO 2 : Carbon-13 Evidence. Science 256, 74–79. doi:10.1126/science.256.5053.74
Raven, J. A., and Falkowski, P. G. (1999). Oceanic Sinks for Atmospheric CO2. Plant Cel Environ 22, 741–755. doi:10.1046/j.1365-3040.1999.00419.x
Raza, T., Ahmad, S. M., Steinke, S., Raza, W., Lone, M. A., Beja, S. K., et al. (2017). Glacial to Holocene Changes in Sea Surface Temperature and Seawater δ18O in the Northern Indian Ocean. Palaeogeogr. Palaeoclimatol. Palaeoecol. 485, 697–705. doi:10.1016/j.palaeo.2017.07.026
Regnier, P., Friedlingstein, P., Ciais, P., Mackenzie, F. T., Gruber, N., Janssens, I. A., et al. (2013). Anthropogenic Perturbation of the Carbon Fluxes from Land to Ocean. Nat. Geosci 6, 597–607. doi:10.1038/ngeo1830
Ren, H., Chen, Y.-C., Wang, X. T., Wong, G. T. F., Cohen, A. L., Decarlo, T. M., et al. (2017). 21st-century Rise in Anthropogenic Nitrogen Deposition on a Remote Coral Reef. Science 356, 749–752. doi:10.1126/science.aal3869
Sabine, C. L., Feely, R. A., Gruber, N., Key, R. M., Lee, K., Bullister, J. L., et al. (2004). The Oceanic Sink for Anthropogenic CO 2. Science 305, 367–371. doi:10.1126/science.1097403
Schimmelmann, A., Lange, C. B., and Berger, W. H. (1990). Climatically Controlled Marker Layers in Santa Barbara Basin Sediments and fine-scale Core-To-Core Correlation. Limnology and Oceanography 35, 165–173.
Schmittner, A., Bostock, H. C., Cartapanis, O., Curry, W. B., Filipsson, H. L., Galbraith, E. D., et al. (2017). Calibration of the Carbon Isotope Composition (δ 13 C) of Benthic Foraminifera. Paleoceanography 32, 512–530. doi:10.1002/2016pa003072
Shackleton, N. (1967). Oxygen Isotope Analyses and Pleistocene Temperatures Re-assessed. Nature 215, 15–17. doi:10.1038/215015a0
Shiu, C.-J., Liu, S. C., and Chen, J.-P. (2009). Diurnally Asymmetric Trends of Temperature, Humidity, and Precipitation in Taiwan. J. Clim. 22, 5635–5649. doi:10.1175/2009jcli2514.1
Simon, M. H., Muschitiello, F., Tisserand, A. A., Olsen, A., Moros, M., Perner, K., et al. (2020). A Multi-Decadal Record of Oceanographic Changes of the Past ∼165 Years (1850-2015 AD) from Northwest of Iceland. PLOS ONE 15, e0239373. doi:10.1371/journal.pone.0239373
Spero, H. J., and Williams, D. F. (1988). Extracting Environmental Information from Planktonic Foraminiferal δ13C Data. Nature 335, 717–719. doi:10.1038/335717a0
Street, J. O., Carroll, R. J., and Ruppert, D. (1988). A Note on Computing Robust Regression Estimates via Iteratively Reweighted Least Squares. The Am. Statistician 42, 152–154. doi:10.1080/00031305.1988.10475548
Su, C.-C., and Huh, C.-A. (2002). 210Pb, 137Cs and 239,240Pu in East China Sea Sediments: Sources, Pathways and Budgets of Sediments and Radionuclides. Mar. Geology. 183, 163–178. doi:10.1016/s0025-3227(02)00165-2
Suess, H. E. (1955). Radiocarbon Concentration in Modern Wood. Science 122, 415–417. doi:10.1126/science.122.3166.415.b
Swart, P. K., Dodge, R. E., and Hudson, H. J. (1996a). A 240-year Stable Oxygen and Carbon Isotopic Record in a Coral from South Florida: Implications for the Prediction of Precipitation in Southern Florida. Palaios 11, 362–375. doi:10.2307/3515246
Swart, P. K., Greer, L., Rosenheim, B. E., Moses, C. S., Waite, A. J., Winter, A., et al. (2010). The13C Suess Effect in Scleractinian Corals Mirror Changes in the Anthropogenic CO2 inventory of the Surface Oceans. Geophys. Res. Lett. 37, L05604. doi:10.1029/2009gl041397
Swart, P. K., Healy, G. F., Dodge, R. E., Kramer, P., Hudson, J. H., Halley, R. B., et al. (1996b). The Stable Oxygen and Carbon Isotopic Record from a Coral Growing in Florida Bay: A 160 Year Record of Climatic and Anthropogenic Influence. Palaeogeogr. Palaeoclimatol. Palaeoecol. 123, 219–237. doi:10.1016/0031-0182(95)00078-x
Takahashi, T., Olafsson, J., Goddard, J. G., Chipman, D. W., and Sutherland, S. C. (1993). Seasonal Variation of CO2and Nutrients in the High-Latitude Surface Oceans: A Comparative Study. Glob. Biogeochem. Cycles 7, 843–878. doi:10.1029/93GB02263
Tao, K., Robbins, J. A., Grossman, E. L., and O'dea, A. (2013). Quantifying Upwelling and Freshening in Nearshore Tropical American Environments Using Stable Isotopes in Modern Gastropods. Bms 89, 815–835. doi:10.5343/bms.2012.1065
Thunell, R., Tappa, E., Pride, C., and Kincaid, E. (1999). Sea-surface Temperature Anomalies Associated with the 1997-1998 El Niño Recorded in the Oxygen Isotope Composition of Planktonic Foraminifera. Geol 27, 843–846. doi:10.1130/0091-7613(1999)027<0843:sstaaw>2.3.co;2
Wang, Y. H., Lee, I. H., and Liu, J. T. (2008). Observation of Internal Tidal Currents in the Kaoping Canyon off Southwestern Taiwan. Estuarine, Coastal Shelf Sci. 80, 153–160. doi:10.1016/j.ecss.2008.07.016
Watanabe, T., Kawamura, T., Yamazaki, A., Murayama, M., and Yamano, H. (2014). A 106 Year Monthly Coral Record Reveals that the East Asian Summer Monsoon Modulates winter PDO Variability. Geophys. Res. Lett. 41, 3609–3614. doi:10.1002/2014gl060037
Watanabe, T. K., Watanabe, T., Yamazaki, A., Pfeiffer, M., Garbe-Schönberg, D., and Claereboudt, M. R. (2017). Past Summer Upwelling Events in the Gulf of Oman Derived from a Coral Geochemical Record. Sci. Rep. 7, 4568. doi:10.1038/s41598-017-04865-5
Wei, G., Mcculloch, M. T., Mortimer, G., Deng, W., and Xie, L. (2009). Evidence for Ocean Acidification in the Great Barrier Reef of Australia. Geochimica Et Cosmochimica Acta 73, 2332–2346. doi:10.1016/j.gca.2009.02.009
Xu, B., Bianchi, T. S., Allison, M. A., Dimova, N. T., Wang, H., Zhang, L., et al. (2015). Using Multi-Radiotracer Techniques to Better Understand Sedimentary Dynamics of Reworked Muds in the Changjiang River Estuary and Inner Shelf of East China Sea. Mar. Geology. 370, 76–86. doi:10.1016/j.margeo.2015.10.006
Keywords: anthropogenic activity, corals, sediment cores, δ13C, δ18O, foraminifera
Citation: Lee J, Yang RJ, Lin H-L, Chen Y-C, Cai-Li R-Y, Ren H and Liu JT (2022) Sedimentary Anthropogenic Carbon Signals From the Western Pacific Margin for the Last Century. Front. Earth Sci. 9:795519. doi: 10.3389/feart.2021.795519
Received: 15 October 2021; Accepted: 14 December 2021;
Published: 28 January 2022.
Edited by:
Daidu Fan, Tongji University, ChinaReviewed by:
Xuefei Chen, Guangzhou Institute of Geochemistry (CAS), ChinaJeroen Groeneveld, University of Hamburg, Germany
Sui Wan, South China Sea Institute of Oceanology (CAS), China
Copyright © 2022 Lee, Yang, Lin, Chen, Cai-Li, Ren and Liu. This is an open-access article distributed under the terms of the Creative Commons Attribution License (CC BY). The use, distribution or reproduction in other forums is permitted, provided the original author(s) and the copyright owner(s) are credited and that the original publication in this journal is cited, in accordance with accepted academic practice. No use, distribution or reproduction is permitted which does not comply with these terms.
*Correspondence: Hui-Ling Lin, aGxsaW5AbWFpbC5uc3lzdS5lZHUudHc=
†These authors have contributed equally to this work and share first authorship