- 1921 Earthquake Museum of Taiwan, National Museum of Natural Science, Taichung, Taiwan
- 2Institute of Oceanography, National Taiwan University, Taipei, Taiwan
- 3Geological Survey of Japan, National Institute of Advanced Industrial Science and Technology, Tsukuba, Japan
By examining bathymetric and seismic reflection data in the shelf-slope region offshore northeast of Taiwan, the morphology of the Huapinghsu Channel/Mienhua Canyon System was refined and the occurrence of axial incision in the major erosional trough of the Mienhua Canyon was identified. The Huapinghsu Channel extends from its head at a water depth of 120 m seaward across the shelf-slope region and merges with the Mienhua Canyon before finally emptying into the Southern Okinawa Trough. This review with a new idea illustrates this channel/canyon system as a sediment conduit transporting sediments from western Taiwan rivers to the Southern Okinawa Trough. Within the proximal reach of the Mienhua Canyon, in situ suspended particles were found associated with bottom nepheloid layers, which transport resuspended particles along the canyon course, and eventually into the Southern Okinawa Trough even during sea-level highstand. The shelf area around the channel head is subject to the influence of frequent occurrences of large typhoons and seasonal migrations of oceanic currents. Numerical examinations indicate that the flow velocities related with large waves or currents satisfy the critical values to the threshold of the motion of fine sand, implying gravity flows occur around the channel head, sweep unconsolidated sediments in the channel head, or even incise the channel floor at the present day. In this paper, sea level changes from the Last Glacial Maximum (LGM, ∼21 ka BP) to the present and sediment input to the head of the Huapinghsu Channel head are considered the major factors in the control of the Huapinghsu Channel/Mienhua Canyon system, which functions as a sediment conduit in the study area. Tectonics and climate are the minor factors that contribute to shelf sediment transport to the Southern Okinawa Trough via this channel/canyon system.
1 Introduction
Submarine canyons and channels are important conduits for transporting modern sediments from shelf to deep sea during both sea-level highstands and lowstands (Gardner, 1989; Covault et al., 2007; Piper and Normark, 2009; Canals et al., 2013; Normandeau et al., 2015; Puig et al., 2017; Chiang et al., 2020). In regional source-to-sink schemes, submarine canyons and channels are significant morpho-dynamic segments, serving not only as major sediment conduits but also as temporary sediment sinks (Sømme et al., 2009). It is reasonable to expect that channels and canyons on the shelf-slope region off northeastern Taiwan (Figure 1) are good candidates for conduits of East China Sea shelf (ECS shelf) sediments to the Southern Okinawa Trough (SOT).
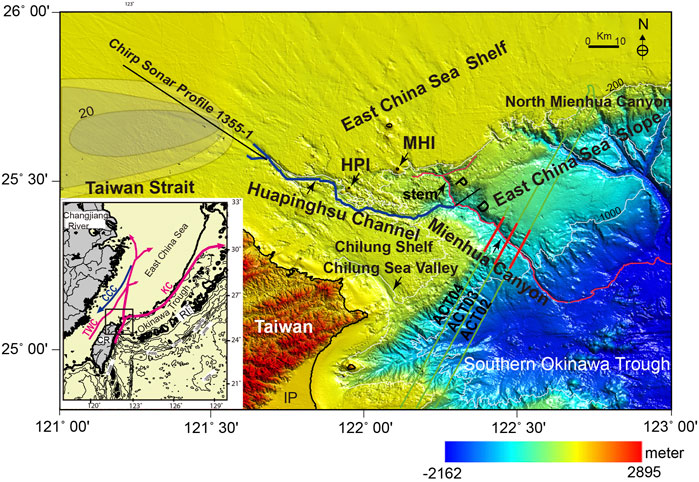
FIGURE 1. A map showing the main physiographic provinces off northeastern Taiwan. The inset in the lower left corner shows the regional geologic setting and the major Taiwan Warm Current and the Kuroshio Current (Zhang et al., 2019; Liu et al., 2020). Note that the Huapinghsu Channel crosses the Chilung Shelf and joins the western branch of the Mienhua Canyon on the slope before finally emptying into the SOT. The Mienhua Canyon can be divided into two parts by the western branch: proximal (P) and distal (D). The CHIRP sonar profile (1355-1) crosses the shelf northwest of Taiwan and extends southeastward to the head of the Huapinghsu Channel. Close to the channel head, a deltaic sediment body (>20 m in thickness) marked in gray is mainly composed of sand with 88% sand-sized grains (Liu et al., 2008). Three parallel track lines of seismic reflection profiles (in green) lie perpendicular to the distal part of the Mienhua Canyon. Three short segmented seismic profiles in red are displayed as interpreted seismic sections in Figure 4. HPI, Huapinghsu Islet; MHI, Mienhua Islet; RI, Ryukyu Islands; IP, Ilan Plain; KC, Kuroshio Current; TWC, Taiwan Warm Current; CCC, China Coastal Current; CR, Choshui River.
Submarine sea valleys, channels, and canyons offshore northeastern Taiwan have been known for more than 40 years (Wang and Hilde, 1973; Boggs et al., 1979; Kimura, 1983; Marsset et al., 1987; Song and Chang, 1993; Yu and Hong, 1993; Song et al., 2000; Chang et al., 2021), but their morphologies, sedimentary processes, sediment dispersals, and evolution have not been fully investigated. Few are well understood as data acquired by modern marine geophysical and geological techniques have been insufficient. Especially, the sediment transport regime and the sediment conduit for delivering the ECS shelf sediments to SOT are still unknown.
We propose a hypothesis that a channel/canyon system is serving as the sediment conduit transporting sediments from western Taiwan rivers to the SOT. This new idea regarding this channel/canyon system as a sediment conduit has not been mentioned in source-to-sink studies in the region offshore northeast of Taiwan before. This hypothesis pays attention to the possibility of a connection of the head of the Huapinghsu Channel to the sediments derived from Taiwanese rivers on the ECS shelf and to the nature of the channel/canyon transition where sediments within the channel course are delivered via the Mienhua Canyon to the SOT (Figure 1).
2 Background
2.1 Geology and Geomorphology
The island of Taiwan (Figure 1) was formed by oblique collision of the passive Chinese margin and the Luzon Volcanic Arc in the Late Miocene–Early Pliocene (Suppe, 1981; Teng, 1990; Dadson et al., 2003). The West Taiwan Basin developed approximately 6.5 Ma ago by lithospheric flexure of the Chinese continental margin in front of migrating thrust and fold loads in the Taiwan orogenic belt (Yu and Chou, 2001; Lin and Watts, 2002). The basin is occupied by shallow sea and was subaerially exposed during the late Pleistocene, when the East China Sea was up to 140 m below its present level (Boggs et al., 1979). Small mountainous rivers draining Taiwan collectively can discharge ∼200 Mt/year sediment into the Taiwan Strait, mostly during typhoon-generated floods (Dadson et al., 2003). The Choshui River, the largest river in Taiwan, occupies only ∼2% of the watershed area of the Yangtze (Figure 1) (Xu et al., 2009). The mountain belt in central-southern Taiwan is still actively uplifting, while the northern Taiwan mountain belt is beginning to collapse (Teng, 1996; Lo and Hsu, 2005). As a result, the sea floor immediately off northern Taiwan is situated in a transition zone among the post-collision Taiwan mountain belt, the passive Chinese margin, and the N-S extension of the rifting SOT (Sibuet et al., 1998; Tsai et al., 2018).
Regionally, the continental margin off northeastern Taiwan consists mainly of the broad ECS shelf, the narrow East China Sea slope (ECS slope), and the deep SOT (Figure 1). The ECS shelf is relatively flat and wide, measuring about 400 km in width, and has a northeast trending edge about 140 m in water depth. The ECS slope near Taiwan is characterized by irregular sea floor features with an average slope angle of about 1.5° (Song et al., 2000; Tsai et al., 2018). Linear depressions such as sea valleys, canyons, channels, and gullies are prominent morphological features of the shelf-slope region off northeastern Taiwan. The Okinawa Trough (OT), lying between Japan and Taiwan, is a back arc basin formed by extension within the continental lithosphere behind the Ryukyu trench-arc system (Kimura, 1985; Sibuet et al., 1998; Chen et al., 2019). The SOT near northeastern Taiwan is bounded by the ECS slope to the north and by the Ryukyu Islands to the south. It extends from the southwest Kyushu Island to the Ilan Plain of Taiwan (Sibuet et al., 1998) (Figure 1). The floor of SOT is at a water depth of less than 2,300 m (Sibuet et al., 1998). The water depth is nearly 200 m close to the Taiwan coast. The SOT is actively rifting with the current N-S extension of the shelf-slope areas near the Huapinghsu Channel and the Mienhua Canyon. The areas surrounding the SOT are characterized not only by active tectonics but also by frequent typhoons and earthquakes (Hsu et al., 2004; Huh et al., 2004; Liang et al., 2019). Submarine slides and mass-wasting features are also observed in this region (Tsai et al., 2018; Chang et al., 2021).
Locally, a relatively small portion of sea floor immediately off the northern coast of Taiwan, known as the Chilung Shelf, shows marked differences from the ESC shelf in terms of tectonics and topography (Song et al., 2000). The Chilung Shelf looks like a platform with rugged surface approximately bounded by isobath 200 m west of the Huapinghsu Channel and the Mienhua Canyon (Figure 1). The noticeable Chilung Sea Valley occurs along the northern coast of Taiwan and is characterized by a relatively small and short linear trough with low reliefs about 20 m along its valley axis (Figure 1). It is about 65 km long and ends near the shelf edge (Song and Chang, 1993; Song et al., 2000). East of the Chilung Sea Valley, two canyons were named the Mienhua Canyon and the North Mienhua Canyon, respectively, by Song and Chang (1993), after an islet near the head of the canyon on the shelf. These two canyons barely indent the shelf, before crossing the slope and extending southeastward into the SOT (Figure 1). The North Mienhua Canyon is a slope-confined canyon with four distinct heads immediately below the shelf edge, coalescing to form a single canyon down-cutting the sea floor with its end in the lower slope about 900 m in water depth (Yu and Lee, 1998). The stem of the Mienhua Canyon mainly developed on the continental slope. The head of this canyon barely indents the shelf edge northward at about 200 m in water depth near the islet of Mienhua (Figure 1). This canyon displays a relatively straight southeastward course but with two noticeable branches at the head and ends in the lower slope at approximately 1,000 m isobath. Yu and Hong (1993) noticed that a broad trough-shaped channel occurs northwest of the Mienhua Canyon with its head close to the islet of Huapinghsu where the water depth is around 120 m. This channel extends from its head near the Huapinghsu Island seaward in a slightly southeasterly direction and merges into the western side of the Mienhua Canyon where a canyon branch occurs at a water depth of about 300 m. The apparent connection of the Huapinghsu Channel to the Mienhua Canyon led Yu and Hong (1993) to name this linear depression the Huapinghsu Channel/Mienhua Canyon System, although the channel/canyon transition has not been clearly determined by the limited bathymetric data (Figure 1).
2.2 Oceanography
The Kuroshio current is an oceanic warm current flowing toward north or northeast off the eastern coast of Taiwan (Figure 1). The current shows the maximum velocity with over 100 cm/s near the surface of its core and gradually reduces it with depth (Tang et al., 2000). Seasonal migration of the Kuroshio current around the shelf edge off north of Taiwan interacts with the northward flowing Taiwan Warm Current (TWC) (Chuang et al., 1993; Tang and Yang, 1993; Tang et al., 1999; Tang et al., 2000; Tseng and Shen, 2003; Yin et al., 2020; Zhao et al., 2021), which yields a counterclockwise circulation of about 100 km in diameter centered around the Mienhua Canyon.
This circulation leads intrusion of the subsurface Kuroshio water onto the northern shelf of Taiwan, a deep southwestward countercurrent along the northern wall of the Mienhua Canyon, and a seaward outflow of continental shelf water around the northern coast of Taiwan. The mean velocity of the southwestward countercurrent showed a nearly constant value of 0.23 m/s over the upper 380 m water volume (Yin et al., 2020). The southwestward countercurrent can be significantly enhanced by a stronger offshore Kuroshio Current northeast of Taiwan. The northward flow of TWC in the Taiwan Strait has been formed about 7.3 Ka BP and has maintained its pathway to the present (Hsiung and Saito, 2017).
Wave condition around the study area is usually moderate. Based on the wave statistics of the Central Weather Bureau, Taiwan, 0.6–2.0 m of the mean wave height and 4.7–6.1 s of the rush period are reported in December, 2015–2019, near the Huapinghsu Channel head (northeast Taiwan; 25.304°N, 121.534°E; 34 m water depth). However, strong typhoons could generate giant waves offshore with the wave height (Hs) > 10 m and a period (Tp) of >10 s (Ou et al., 2002; Shih et al., 2018).
2.3 Sediment Transport
The SOT has been considered to be an ideal place to study regional source-to-sink process. As a main sediment sink, the SOT receives abundant terrigenous sediment from multiple sources (Figures 1 and 2), including major rivers on the Chinese mainland and small mountain rivers in Taiwan (Kao et al., 2003; Wei et al., 2006; Bentahila et al., 2008; Diekmann et al., 2008; Liu et al., 2008; Dou et al., 2016; Li et al., 2016; Chen et al., 2017; Hsiung and Saito, 2017; Xu et al., 2019; Hu et al., 2020).
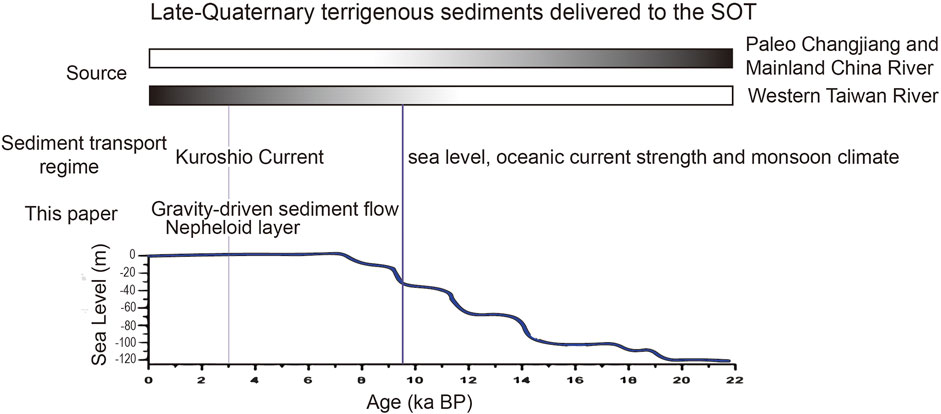
FIGURE 2. There are variations in sediment provenance in the SOT from the dominance of the paleo-Changjiang to western Taiwan rivers with changes in sea level from 22 ka to the present (Wang et al., 2015). The gradient gray bar represents the relative sediment contributions of possible provenances to the SOT from 22 to 9.5 ka. Dark gray indicates high contribution and light gray low contribution. Gray represents the sediment contribution mainly from western Taiwan rivers from 9.5 ka to the present. The sea level curve is represented by the solid black line in the Western Pacific Ocean (Liu et al., 2004). Note that in this study, gravity-driven sediment flows and nepheloid layers were considered the main sediment transport regime for the Huapinghsu Channel/Mienhua Canyon System, delivering sediments across the shelf/slope region to the SOT.
Diekmann et al. (2008) have suggested that sediment supply increased from the northwestern Taiwan between 28 and 19.5 ka BP, rather than predominantly coming from East China. Other proxies, for instance, Pb and Sr isotopes (Bentahila et al., 2008), have indicated a mixture, with about 60% from Taiwan, 30% from Chinese loess, and 10% from Changjiang River (Yangtze River). The SOT has a very high sedimentation rate of 0.10–0.95 cm/year (Chung and Chang, 1995; Wei et al., 2005; Wei et al., 2006), providing a good archive for examining sedimentation history in the Late Quaternary. For instance, Dou et al. (2016) found changes in detrital sediment provenance in the SOT from a dominance of the paleo-Changjiang and/or continental shelf sediment during the late deglaciation and from western Taiwan rivers since 9.5 ka BP (Figure 2).
Hsiung and Saito (2017) linked the abrupt provenance shift in SOT sediments from mainland China to Taiwan with the birth of the TWC since 7.3 ka BP, which transports sediments derived from western Taiwan rivers to the ECS shelf. From Sr-Nd isotopes and geochemical measurements, Hu et al. (2020) proposed that terrigenous sediment supply has increased from western Taiwan to the SOT over the last 3 ka BP (Figure 2).
Under the present sea-level highstand, sediments on the sea floor in the Taiwan Strait are composed mainly of silt, whose coarse fractions (fine sand to medium silt) have mostly been derived from large hyperpycnal discharges from rivers in the western Taiwan (Xu et al., 2009; Hsiung and Saito, 2017; Jin et al., 2021). Much of the sediments were carried northward by the TWC through Taiwan Strait and formed a thick (>20 m) sediment body on the shelf (Liu et al., 2008; Hsiung and Saito, 2017). Furthermore, Hsiung and Saito (2017) suggested that western Taiwanese river sediments are transported northward by the TWC to the ECS shelf and finally to the SOT with the evidence from provenance studies of SOT. Liu et al. (2008) conducted a comprehensive study of post-glacial northwestern Taiwanese sediment distribution and transport in the Taiwan Strait. Geochemical evidence has led Chen et al. (2017) to suggest that some Changjiang River-derived sediment is carried by the China Coastal Current southward and subsequently joins the northward flowing Kuroshio along the western Taiwan coast to be transported to the SOT.
3 Data and Methods
Using bathymetric data and reflection seismic profiles, the general topography of the continental margin off northeastern Taiwan is revealed with focuses on the morpho-seismic features of the Huapinghsu Channel and the Mienhua Canyon on the shelf-slope region (Figure 1). In 1996, a French–Taiwanese cooperative research program (the Active Collision in Taiwan program) was carried out aboard the French R/V L’Atalante in the offshore areas of Taiwan, including the shelf-slope region off northeastern Taiwan (Lallemand et al., 1996). During this cruise, a six-channel streamer was deployed with two 75-cubic-inch GI guns at a pressure of 160 bars. The guns were fired in harmonic mode to generate a source signature centered on 20 Hz to enhance sub-bottom penetration of energy. Shot intervals were ∼50 m. Following standard procedures using the SIOSEIS and PROMAX software packages, the seismic data was processed to generate the seismic sections. Seismic reflection profiles collected during the 1996 cruise were stored in the Ocean Data Bank (ODB) at the National Taiwan University, Taiwan. Three seismic profiles (ACT 02–ACT04) across the distal reach of the Mienhua Canyon were selected for this study (Figure 1).
Bathymetric data was collectively acquired by R/V Researcher I and R/V Researcher II, which were operated by the National Taiwan University and the National Taiwan Ocean University, respectively. New bathymetric data were then integrated into the bathymetric databank at the National Center for Ocean Research of National Taiwan University to generate the bathymetric charts and bathymetric profiles for this study. A series of bathymetric profiles across the axis of the Huapinghsu Channel displayed the cross-sectional morphology of the channel. In addition, one CHIRP sonar image (profile 1355-1) was selected from the published literature (Liu et al., 2008) to link the possible shelf sediment supply to the head of the Huapinghsu Channel. The southeastern end of the profile close to the head of the Huapinghsu Channel shows a sediment body mainly derived from western Taiwan rivers about 20 m thick, which could be disturbed and mobilized by earthquakes or typhoon events (Figure 1). Our study primarily presents integrated interpretation of morpho-seismic characteristics including new bathymetric data and other published datasets across the course of the Huapinghsu Channel and the Mienhua Canyon and selected seismic profiles covering the distal reach of the Mienhua Canyon.
4 Results
4.1 Geomorphology
4.1.1 Planform Morphology
Based on bathymetric mapping, the Huapinghsu Channel planform characteristics include a relatively straight linear depression that runs slightly southeast parallel to the coastline of northern Taiwan (Figure 1). The head of the Huapinghsu Channel extends headwards into the shelf for about 82 km, showing a linear depression, representative of a typical shelf-indenting channel (Figures 3A,B). This channel head has two short branches, eastern and western, which converge down-channel at a 150-m water depth. The southeast end of the Huapinghsu Channel displays an ambiguous bathymetric expression, losing the confined channel course and forming a break that opens to the northeast, where the 200 m isobath along the northern side of the channel sharply bends to the north and then turns to the east along the ECS shelf edge (Figure 1). However, the Huapinghsu Channel continues down-slope and joins the western branch of the Mihenhua Canyon at the water depth of 215 m, forming a channel/canyon connection (Figures 1 and 3).
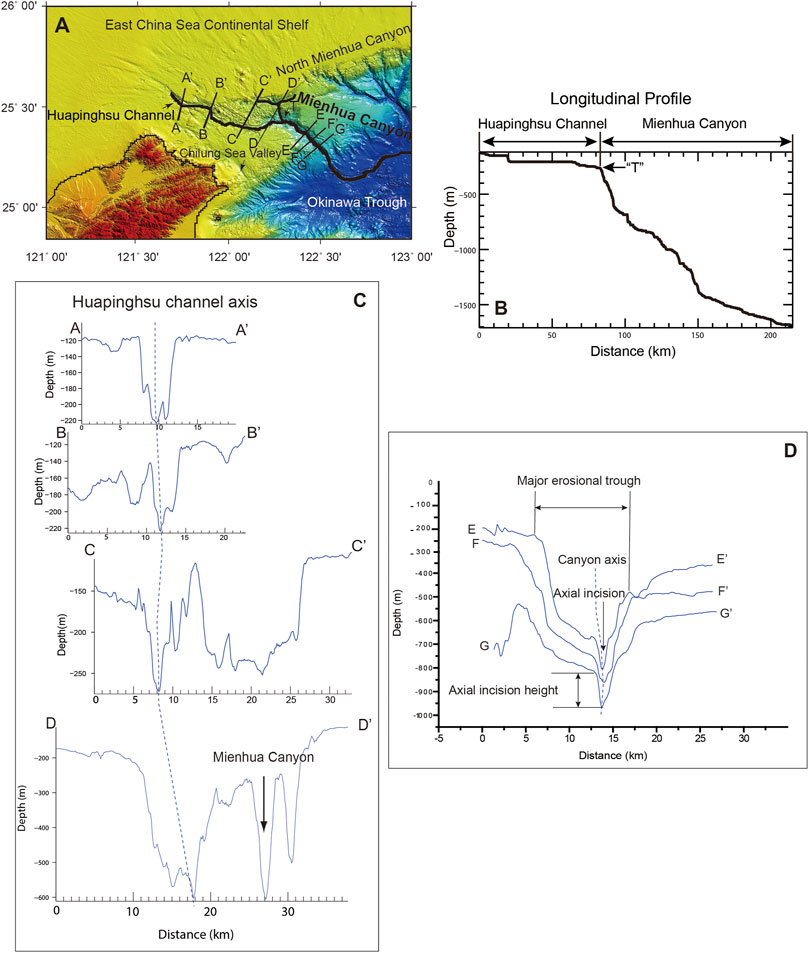
FIGURE 3. Locations of a series of bathymetric profiles across the Huapinghsu Channel and the distal part of the Mienhua Canyon (A). The length of the Huapinghsu Channel/Mienhua Canyon System is 215 km and can be separated into two parts by the reference point “T,” which is the turning point for the change in slope angle. The Huapinghsu Channel has a gentle slope angle of 0.15°, and the distal Mienhua Canyon has a steep slope angle of 0.98° (B). The stem of the Huapinghsu Channel is characterized by a V-shaped depression with a narrow width of about 5 km and an incision depth of around 120 m (profiles A-A′ through C-C′). The channel end increases in width up to 8 km and in incision depth up to 400 m. The thalwegs of the Huapinghsu Channel can be aligned into a continuous bathymetric depression as shown by the dotted lines (C). Profiles E-E′ through G-G′ show that the distal part of the Mienhua Canyon is characterized by an axial incision within the major erosional trough (D).
4.1.2 Channel Relief Morphology
A series of bathymetric profiles across the courses of the Huapinghsu Channel and Mienhua Canyon show the cross-sectional morphology of this channel/canyon system (Figure 3). The channel head segment is about 5-km wide and 100-m deep with steep channel walls of about 2.3° on both sides, revealing a V-shaped trough (Figure 3C, profile A-A′). The incision depth of the channel is the relief between channel bottom and edges on bathymetric profiles. Farther down-channel, bathymetric profile B-B′ shows that the width of the channel increases slightly to about 6 km with the depth maintained at about 100 m with steep channel walls and slope angles of about 2.5°. Apparently, the channel edge to the north is slightly shallower than that to the south, revealing an asymmetrical V-shaped trough. Profile C-C′, farther down-channel, shows that the channel is about 7-km wide and 120-m deep with steep channel walls and slope angles of about 2°, just like on profile B-B′. Apparently, the channel wall on the northern side has an irregular surface, in contrast with the smooth surface of the southern channel wall. In general, the Huapinghsu Channel is a relatively narrow (5–7-km wide) V-shaped trough with incision depths ranging from 100 to 120 m.
4.1.3 Canyon Axial Incision (Cross-Sectional View)
Crossing the western branch and the head segment of the Mienhua Canyon, profile D-D′ shows that the western canyon branch is a general V-shaped trough. The canyon width is about 11 km and the canyon depth is about 600 m with irregular bottom and no narrow V-shaped depression (Figure 3C). In contrast, the head segment of the Mienhua Canyon is characterized by the sharp V-shaped cross-sectional morphology typical of submarine canyons (Figure 3C, profile D-D′). The canyon edges of the head segment are about 180-m deep and about 4-km wide, with a canyon relief of about 320 m. Farther down-canyon, profiles E-E′, F-F′, and G-G′ show that the cross-sectional morphology of the canyon segment on the lower slope is quite different from that of the canyon head segment (Figure 3D). Profile E-E′ shows that the western canyon edge is about 240 m in water depth, which is shallower than the eastern canyon edge, resulting in an asymmetrical V-shaped trough. This canyon is about 10.5 km wide and about 810 m deep with canyon relief of around 570 m. The canyon gradually narrows downwards. Near the bottom, the width decreases substantially to form a sharp V-shaped axial incision of about 120 m in height and 1.5 km in width (Figure 3D). Farther down-canyon, the cross-sectional morphology on profile F-F′ resembles that on profile E-E′. The cross section of the canyon shows an asymmetrical V-shaped trough with a width of about 13 km. The canyon bottom is about 860 m deep with canyon relief of about 600 m. This canyon segment is also characterized by an axial incision about 100 m high and 2.5 km wide (Figure 3D). Profile G-G′, farther down-canyon, shows that the canyon displayed as a V-shaped trough is about 13.5 km wide and 970 m deep with canyon relief of about 440 m. An axial incision about 140 m high and 3.6 km wide can be seen at the canyon bottom (Figure 3D). It is noted that the cross-sectional morphology on profiles E-E′, F-F′, and G-G′ is characterized not only by a general V-shaped trough of typical submarine canyons but also by the presence of a sharp V-shaped axial incision. This distinct cross-sectional canyon morphology has been reported in the Danube submarine canyon in the northwestern Black Sea (Popescu et al., 2004) and submarine canyons of the western Gulf of Lion (Baztan et al., 2005). Morphologically, the canyon from both edges to the bottom is referred to as a major erosional trough (Popescu et al., 2004). A V-shaped notch or depression on cross sections where there is no sediment accumulation in the deepest part of the canyon is referred to as an axial incision (Baztan et al., 2005; Micallef et al., 2014; Normandeau et al., 2015). Note that the major erosional trough described in this paper follows the terminology of Popescu et al. (2004) rather than that of Shepard (1981).
4.1.4 Longitudinal Profile of the Huapinghsu Channel/Mienhua Canyon System
The longitudinal profile of the Huapinghsu Channel/Mienhua Canyon System shows a relatively flat channel and generally convex upward canyon (Figure 3B). The thalweg length of the Huapinghsu Channel/Mienhua Canyon System is 215 km and can be separated into two parts at reference point “T,” which marks the turning point of change in slope angle. The Huapinghsu Channel at the shelf is characterized by a relatively gentle longitudinal profile with minor variations in channel depth along the course and an average slope angle of around 0.15° in accordance with that of the surrounding shelf floor (Song et al., 2000). In contrast, the longitudinal profile of the Mienhua Canyon is a steep continuous inclination. Moreover, the western branch head of the Mienhua Canyon is at about 215 m in water depth, dropping to 1,000 m for about 46 km along the canyon course with an average slope angle of 0.98° (Figure 3B). Apparently, the reference point “T” divides this channel/canyon system into a channel segment with a gentle slope angle of 0.15° and a canyon segment with a steep slope angle of 0.98°.
4.2 Morpho-Seismic Features
This section describes major seismic characteristics and morpho-sedimentary features from three seismic reflection profiles crossing the segment of the Mienhua Canyon at a water depth of more than 600 m. Seismic profiles penetrate downwards into the slope strata below the present canyon floor, allowing for examinations of characteristic features of the infilled sediments accumulated during the preceding canyon developing stages and interpretations of sedimentary processes related to the present Mienhua Canyon. Seismic profile ACT-04 (Figure 4A) shows distinct morpho-sedimentary features of a major erosional trough with steep flanks and flat-bottom cut by an axial incision. The main body of the major erosional trough consists of layered parallel to sub-parallel reflectors with high to low amplitudes. These seismic reflectors show variations in lateral continuity. Tilting and chaotic seismic facies are also present. The surface of the southwestern flank of the Mienhua Canyon is an inclined seismic reflector that extends down-slope and is intercepted by the canyon floor, which is a relatively smooth and flat seismic reflector. The shallow successions below the canyon floor are characterized by stratified, continuous, and parallel reflectors, which form layers that terminate at the inclined reflector below the canyon floor, forming an onlap seismic configuration (Figure 4A). Moreover, the northeastern flank of the Mienhua Canyon is an inclined seismic reflector with an irregular surface associated with several steps extending down-slope to the flat canyon bottom. It is noted that sub-parallel reflections below the northeast canyon flank terminate against the inclined reflection, implying that the northwestern canyon flank is an erosional surface. The axial incision is a sharp V-shaped depression without sediment accumulation at the thalweg. Apparently, layers of reflections below the canyon floor are truncated by both flanks of the axial incision, indicating down-cutting of the canyon bottom. Below the axial incision, chaotic seismic reflections occur, overlain by high-amplitude bedded facies that are probably incised axially. Ancient axial incisions likely formed during the early stages and were infilled by sediment later, resulting in axial incision-like features. Farther down-canyon, seismic profile ACT-03 (Figure 4B), parallel to seismic profile ACT-04, shows two distinct morpho-seismic features of a major erosional trough, flat bottom, and entrenched axial thalweg, closely resembling those on profile ACT-04. However, there are noticeable differences in these two features. Both major erosional trough tops are well recognized on seismic section, showing a symmetrical V-shaped depression with relatively narrow canyon walls and broad flat canyon floors. In the middle of the canyon floor is an entrenched axial incision with similar dimensions to those on profile ACT-04. However, the axial incision is characterized by a narrow U-shaped depression rather than a V-shaped depression. Note that the axial incision on profile ACT-03 is partially infilled by sediment, resulting in a relatively flat reflector at the incision bottom with a U-shaped depression.
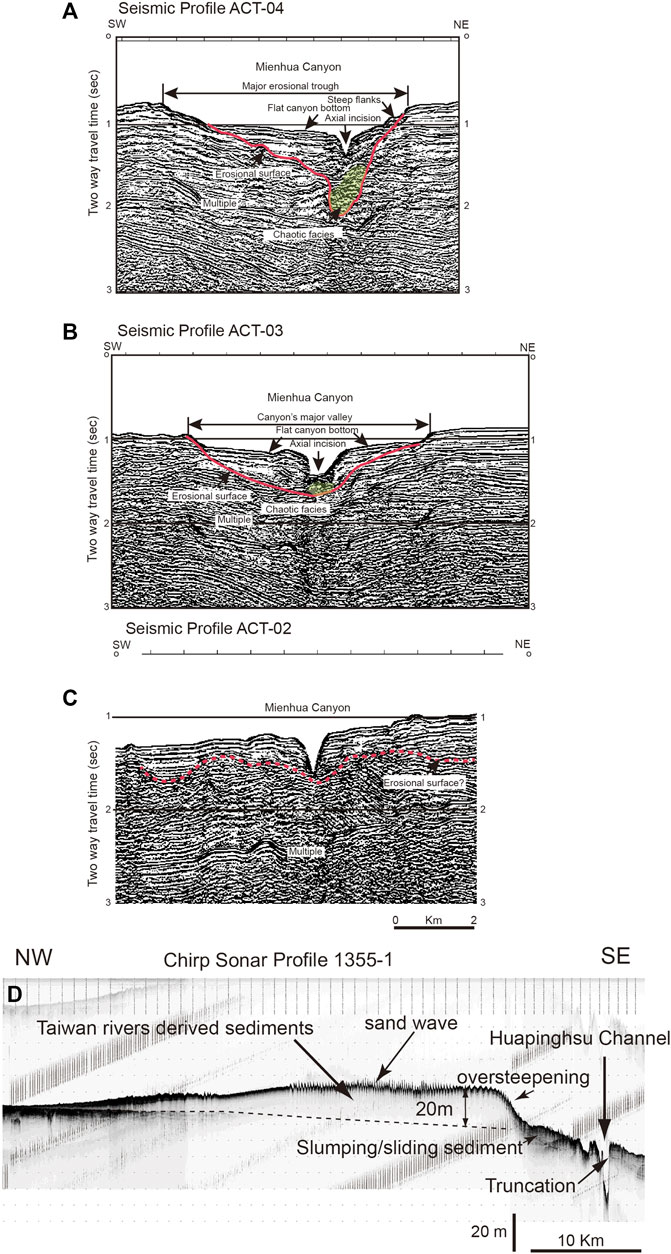
FIGURE 4. Seismic profiles (ACT-04 and ACT-03) crossing the distal canyon segment show two distinct morpho-seismic features, major erosional trough, and entrenched thalweg, of the Mienhua Canyon (A,B). Farther down-canyon, seismic profile ACT-02 shows a small V-shaped depression about 1-km wide, cutting downward into the flat canyon bottom (C). The major erosional trough was mainly formed over multiple episodes of erosion and partial sediment infilling. The occurrence of an axial incision implies down-cutting into the canyon floor by erosive sediment flows with high sediment supply. The presumed basal erosional surface is denoted by the red dotted line (C). The southeast trending CHIRP sonar profile (1355-1) across the East China Sea shelf shows that the head of the Huapinghsu Channel is immediately in front of the local depocenter of shelf sediments (about 20-m thick) sourced from western Taiwan rivers (D). Note that the shelf sediments have a steep flank facing the head of the Huapinghsu Channel, characterized by a narrow V-shaped entrenched channel floor. The shelf sediments flanked by over-steeping slope most likely collapse and re-mobilize, with subsequent feeding into the head of the Huapinghsu Channel. The closely spaced spikes are sand waves on the surface of the sediment body (Boggs et al., 1979; Liu et al., 2008). The presence of sand wave indicates that bottom currents are fast enough to move sand-sized sediment. The location of the chirp sonar profile is shown in Figure 1 (Liu et al., 2008).
Farther down-canyon, seismic profile ACT-02 (Figure 4C) crossing the Mienhua Canyon shows a small V-shaped depression about 2 km wide and 190 m deep, cutting downward into the slope strata. Apparently, the canyon shape changes drastically from a well-defined major erosional trough with a distinct axial incision to a hardly recognizable major erosional trough with only a thalweg incision down-cutting the present canyon floor.
5 Discussion
5.1 Huapinghsu Channel
In general, channels and canyons are formed by downslope erosion of the sea floor on both active and passive margins. The major difference is that tectonics control the location and orientation, while sedimentary processes contribute to the excavation and enlargement of channels and canyons (Hagen et al., 1994; Yu and Hong, 2006; Bernhardt et al., 2015; Chiang et al., 2020). This section focuses on the sedimentary processes related to the role of the Huapinghsu Channel as a modern sediment conduit. Moreover, the orientation and location of the Huapinghsu Channel are related to tectonic influence on the development of this channel.
5.1.1 Tectonic Control
Yu and Hong (1993) proposed that base level change (i.e., changes in sea level) mainly controlled erosion and deposition within the Huapinghsu Channel but did not consider tectonic influence on its development. In contrast, Song et al. (1997) and Song et al. (2000) examined the tectonics offshore northeastern Taiwan and found regional topographic lineaments and lateral strike-slip faults on the Chilung Shelf. The occurrence of the Chilung Sea Valley is closely related to topographic lineaments and associated faults, which led Song et al. (2000) to propose a fault-controlled origin for the Chilung Sea Valley. This alternative tectonic origin for the Chilung Sea Valley can explain the low slope gradient of about 1/500 along its course, which is very close to that of the Chilung Shelf.
The Huapinghsu Channel and the Chilung Sea Valley characteristically share similar orientation and location mainly due to the influences of tectonics. We considered that the Chilung Sea Valley is a modern analogy for the Huapinghsu Channel, paying attention to the tectonic influence on the development of the Huapinghsu Channel. The development of channels and canyons under structural control is common (e.g., Laursen and Normark, 2002; Chiang and Yu, 2006; Noda et al., 2008; Chiang et al., 2012; Micallef et al., 2014; Wiles et al., 2019). Therefore, we proposed that the Huapinghsu Channel developed under the effects of not only changes in sea level but also tectonics and gravity-driven sediment flows. The relative contributions to the development of the Huapinghsu Channel are not able to be completely determined based on the currently available data.
5.1.2 Sedimentary Processes of the Huapinghsu Channel
At the head of the Huapinghsu Channel close to the islet of Huapinghsu, the water depth is around 120 m. This shallow shelf channel is characterized by a relatively narrow (5–7 km wide) V-shaped trough with incision depths ranging from 100 to 120 m. Using models of submarine canyon evolution (Shepard, 1981; Farre et al., 1983), Yu and Hong (1993) speculated that there was initiation of the Huapinghsu Channel at the paleo-shelf edge at 15 ka BP when the sea level dropped to about 140 m below the present level. This may have been followed by significant slope failures and mass-wasting, with channel head erosion progressively up-slope and indentation into the shelf for about 60 km. The channel head did not extend far into the shelf probably due to the low gradient of the shelf and the absence of fluvial sediment input from Taiwan or mainland China, resulting in low capacity for generation of erosive sediment flows within the channel. The rising sea level stopped the headward erosion of the Huapinghsu Channel. Cut off from its main sediment source, this channel/canyon system no longer acted as a modern conduit transporting sediment to the sea (Yu and Hong, 1993, p. 307).
The results of this study suggest that the Huapinghsu Channel acts as an active sediment conduit during the present sea-level highstand, which is in contrast to the findings of Yu and Hong (1993). Yu and Hong (1993) considered that the rise and fall of relative sea level (i.e., base level change) is the key control of sedimentary processes including erosion, transportation, and deposition in the Huapinghsu Channel. However, recent advanced studies have suggested that other factors such as tectonics, climate, and sediment input are as important as relative sea-level changes in channel and canyon activities (Walsh and Nittrouer, 2003; Covault et al., 2007; Covault and Graham, 2010). In particular, sediment input to the heads of channels and canyons has been emphasized for maintaining the activity of channels and canyons and transport of sediment down-slope during the sea-level highstand (Covault et al., 2007; Covault and Graham, 2010). For example, the presence of littoral sediment cells or accumulations of sediment brought by longshore currents or other oceanographic processes close to the head of channels or canyons and the shelf sediments near the head are captured or intercepted by the head segment, generating gravity sediment flows and allowing sediment transport along the channel/canyon floor farther down-slope (Covault et al., 2007; Bernhardt et al., 2015; Maier et al., 2019). If there is adequate sediment input to the Huapinghsu Channel head, this channel can maintain transport of sediments down-slope along the channel course even during the present sea-level highstand. Therefore, we explored the sediment input to the Huapinghsu Channel head. To date, there has been no published report on the presence of littoral sediment cells on the shelf around the head of the Huapinghsu Channel. However, we found thick deposits of sediment mainly derived from northwestern Taiwan rivers on the shelf off northwestern Taiwan near the Huapinghsu Channel head (Figure 1). In addition, a southeastern trending CHIRP sonar profile (1355-1) across the ECS shelf showed that the location of the head of the Huapinghsu Channel is immediately southeast of the 20-m-thick shelf deposits sourced from Taiwan rivers (Figure 4D). Core samples indicate that coarse-grained sediments are dominant near the end of the Huapinghsu channel, whereas coarse-grained rock fragments and mud clasts are transported farther down channel to the Mienhua Canyon (Yu and Hong, 1993). These mud clast conglomerates are interpreted to be the product of gravity flow due to the presence of sub-angular to rounded clasts that range in size from granular to pebble. The sub-angular clasts probably result from slumping or sliding due to failures of steepened walls. The coarse-grained sediments indicate that the distal part of the channel has experienced strong (high-velocity) currents that enable to resuspend fine-grained sediments during the present high-stand condition. It is suggested that the present-day Huapinghsu Channel remains active mainly due to erosive mass-wasting events in response to slumping and slope failure at the steep flanks.
Oceanographic studies have indicated that occurrences of upwelling, counter-currents, branching, and frontal eddies are associated with turning of the Kuroshio Current in this region (Lin et al., 1992; Chern and Wang, 1994; Yin and Huang, 2019). The shelf sediments close to the Huapinghsu Channel head could be disturbed and removed by local currents, resulting in a connection between the channel head and nearby shelf sediments. Seasonal typhoons and earthquake events occur around the channel head areas, re-remobilizing the shelf sediments and feeding sediments to the head of the Huapinghsu Channel. The high sediment supply to the Huapinghsu Channel head is favorable to the generation of turbidity flows, transporting trapped shelf sediment in the head segment farther down channel to the SOT via the Mienhua Canyon. Here, we considered that the present Huapinghsu Channel remains active due to the erosion of channel bottom and transportation of sediments down-channel to the western branch of the Mienhua Canyon mainly due to a continuous sediment supply from the nearby shelf into the channel head. Furthermore, both the Chilung Sea Valley and the nearby Huapinghsu Channel are located on the Chilung Shelf under similar tectonism and on relatively flat sea floor. There exist morphological differences between these two linear troughs in terms of relief or incision depth. The former has an incision depth of around 20 m and the latter has an incision depth of about 100–120 m. Our explanation for the differences in incision depth is that the Chilung Sea Valley has no apparent sediment input to its head at present, resulting in low capacity for generation of gravity sediment flows that erode the valley bottom. In contrast, the Huapinghsu Channel head is supplied by nearby modern shelf sediments, allowing the generation of gravity sediment flows to down cut the flat sea floor (gradient of 0.15°) with an incision depth of up to 120 m and to transport sediment to the SOT during sea-level highstand.
5.2 Factors on Generating Density Currents Around the Channel Head
V-shaped channel bottom indicates that the channel is not filled by sediments, implying gravity flows sweep unconsolidated sediments in the channel or even actively incises the channel floor. Here, we discuss the possibility of the occurrence of such density currents around the channel head.
Large waves during typhoons can often resuspend seafloor sediments on shelves and generate density current (e.g., Fan et al., 2004; Puig et al., 2004; Palanques et al., 2008). Because the study area is a place where intensive typhoons repeatedly attack every year, density flows may be formed by resuspended sediments from the seafloor during and after the events. Recent typhoons Soudelor (August, 2015) and Megi (September, 2016) are examples that caused heavy damages and numbers of fatalities in Taiwan. Both typhoons recorded large wave heights and long wave periods, whose maximum significant waves and the periods were 14.2 m and 15.1 s for the Soudelor and 8.2 m and 11.9 s for the Megi, respectively, at the Fugui Cape Buoy (Central Weather Bureau, Taiwan).
Based on the Airy wave theory (e.g., Komar, 1998), the maximum orbital velocities Uw for the maximum significant wave heights reach 139 cm/s for the Soudelor and 44 cm/s for the Megi at the water depth of 80 m around the head of the Mienhua Canyon (Figure 5A). Here, the threshold velocities for the motion of sand by the bottom orbital velocity Uw are obtained from the equations of Komar and Miller (1975),
where s is the ratio of densities of grain and sea water (1,650 kg/m3), g is the gravitational acceleration (9.81 m/s2), D is the grain size, and do is the orbital diameter by wave at the seafloor. Combining the depth-dependent orbital velocities (Figure 5A) with the threshold grain size under the waves (Figure 5B) yields a diagram showing the relationship between water depth and the threshold grain size (Figure 5C). This suggests that the typhoon waves are large enough to entrain coarse sand (1 mm) shallower than 80 m water depth and fine sand (0.1 mm) at ∼120 m water depth, although the normal wave base for coarse sand and fine sand are 16 and 27 m, respectively (Figure 5C). Because the wave-induced bottom shear stress is commonly enhanced by tides and surges (e.g., Shih et al., 2018), it is likely that sediment resuspension and generation of sediment gravity flows around the channel head occur during typhoon events.
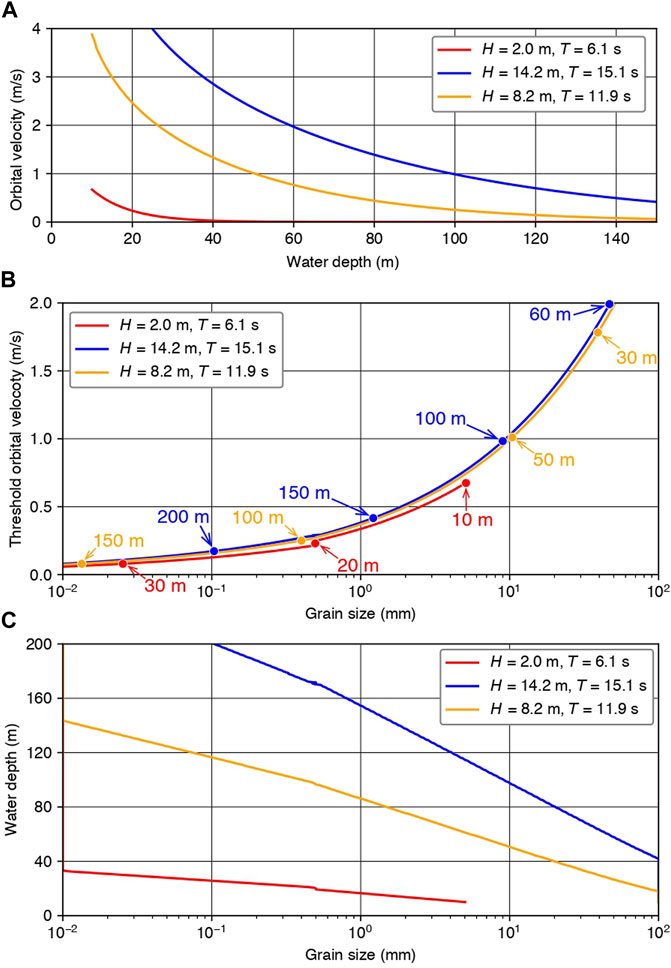
FIGURE 5. Orbital velocities under usual (red) and typhoon (blue and orange) conditions. The maximum significant wave height (H) and period (T) were recorded by the typhoons of Soudelor (2015) (blue) and Megi (2016) (orange) (A). Relationships between threshold orbital velocity and grain sizes for three cases (B). Plots of water depth versus grain size. Under usual conditions, wave base for fine sand is less than 40 m in water depth, while typhoons can shift the base to more than 100 m (C).
Another possibility for shelf-to-channel flows at the edge of the shelf northeast of Taiwan may be also caused by the seasonal migration of the Kuroshio current (Chuang et al., 1993; Tang et al., 1999; Tang et al., 2000; Tseng and Shen, 2003). More than 30 cm/s of the flow velocity were reported along the shelf margin of 100–300 m water depth (Tang et al., 1999; Tang et al., 2000; Yin et al., 2020). For the case of unidirectional steady flows, threshold grain size can be estimated by using the following equations (p. 100 in Soulsby, 1997),
where h is the height from the seafloor and ν is the kinematic viscosity of water (10−6 m2/s). This equation indicates that the threshold current velocities at 1 m above the seafloor are 0.29 m/s for fine sand (0.1 mm) and 0.41 m/s for coarse sand (1 mm), respectively. The observed flow velocities of the currents satisfy the critical values to threshold of motion of fine sand. Therefore, such southwestward countercurrent in this region may also cause density currents from the outer shelf where unconsolidated sediments are supplied.
5.3 Mienhua Canyon
Based on bathymetry, seismic profiles, and previous studies, we focused on the Mienhua Canyon as a main sediment conduit for receiving shelf sediments from the Huapinghsu Channel and transporting them down slope towards the SOT. The stem of the Mienhua Canyon is a slope-confined canyon characterized by a head terminating at the upper slope immediately below the shelf-break (Figure 1). Therefore, in the present sea-level highstand, the stem of the Mienhua Canyon has no apparent sediment input from fluvial sources to the canyon head. Hsu et al. (2004) clearly illustrates developments of intermediate and bottom nepheloid layers that were responsible for suspended sediment transportation from the shelf to the trough. Hung et al. (2003) also suggests the suspended particles might be sourced from resuspended bottom sediments. Submarine canyons can act as preferential pathways for sediment escaping from the continental shelf to the deep sea (Puig et al., 2014; Wilson et al., 2015; Haalboom et al., 2021), In other words, the present Mienhua Canyon acts as a sediment conduit, delivering mainly in situ sediments generated within proximal reach with minor amounts of ECS shelf sediments farther down canyon.
Instead, the western canyon branch and distal part function as a main sediment conduit delivering sediments sourced from Taiwan rivers to the SOT. Our results showed that the distal reach of the Mienhua Canyon is characterized by distinct morpho-seismic features of a major erosional trough with steep flanks and flat bottom cut by an axial incision (Figures 4A,B). The major erosional trough and axial incision observed in the Mienhua Canyon closely resemble the morpho-seismic features found in other submarine canyons (Popescu et al., 2004; Baztan et al., 2005). For the Mienhua Canyon, the major erosional trough and the filling of the canyon below the flat canyon bottom have resulted from multiple episodes of erosion and deposition throughout its evolution, in agreement with the findings of previous studies on canyon development (Shepard, 1981; Pratson and Coakley, 1996; Popescu et al., 2004; Baztan et al., 2005). For example, the stratified sediments of flat canyon floor overlie the steep canyon flanks to form an onlap seismic configuration (Figure 4A), indicating that the major erosional trough is subsequently and partially filled with sediment. In general, the occurrences and formation of axial incision in submarine canyons are mainly formed by erosive sediment flows generated by high sediment input into the canyon head areas. High sediment supply to the canyon is usually related to paleo-fluvial drainage during sea-level lowstand. Specifically, present-day axial incisions in submarine canyons (Black Sea and Gulf of Lion) have resulted from erosive sediment flows down-cut into the flat canyon bottom during the last sea-level lowstand. By analogy, the axial incisions in the Mienhua Canyon were formed by erosive sediment flows during the last sea-level lowstand 18 ka BP. Subsequent transgression took place some 9 to 9.5 ka BP (Figure 2). The ECS shelf north of the Mienhua Canyon head became a sediment-starved shelf, and the sediment supply to the canyon head was cut off with reduced canyon erosion and sediment transport. The Mienhua Canyon remained active, transporting sediment down-canyon even without high sediment supply from the head areas during the sea-level highstand. Due to the high sediment supply from the western canyon branch, the stem of the canyon head kept the canyon segment south of the head areas active during the subsequent transgression and sea-level highstand. In addition, intensities or magnitudes of erosion and transportation of sediment flows within the Mienhua Canyon may have been enhanced by episodic floods and earthquakes (Hsu et al., 2004). As long as shelf sediments are adequately and continuously supplied to the western canyon branch via the Huapinghsu Channel, the Mienhua Canyon maintains an active sediment conduit, delivering river-borne sediments from western Taiwan to the SOT even during sea-level highstand.
5.4 Development of a Modern Sediment Conduit: The Huapinghsu Channel/Mienhua Canyon System
In this section, we intend to integrate the shelf sediments sourced from western Taiwan rivers, Huapinghsu Channel, Mienhua Canyon, and the SOT into a unified source-to-sink scheme. We propose a simplified model for the Huapinghsu Channel/Mienhua Canyon System as a modern sediment conduit with the main controls of changes in sea level, sediment supply to the heads of channel and canyon, sedimentary processes, and tectonics. This simplified model is described as follows:
At about 18 ka BP, a series of subaerial rivers and submarine channels and canyons developed in northeastern Taiwan (Boggs et al., 1979). No names were given to these linear troughs on the exposed shelf and submerged channels and canyons, which were assumed to be the seaward extensions of subaerial rivers. These linear troughs are generally oriented in a NW-SE direction and normal to the paleo-shelf edge of the ECS shelf (Figure 6A). Some of these linear troughs are considered the precursors of the Chilung Sea Valley, Huapinghsu Channel, and Mienhua Canyon. During the last sea-level lowstand, most of the present-day Huapinghsu Channel was exposed subaerially as river channel. However, the distal part at a water depth of >140 m might have remained a submarine channel. The stem of the Mienhua Canyon and the Huapinghsu Channel, close to the paleo-shoreline, possessed the capacity for receiving nearby fluvial and shelf sediments. Fluvial sediment input and shelf sediments, together with in situ sediments, resulted from slope failures of both canyon walls in the canyon head areas with transport down-canyon and final delivery to the SOT. Dou et al. (2016) suggested that sediments sourced from paleo-Changjiang and mainland Chinese rivers transported to the SOT were prevalent before 9.5 ka BP (Figure 2). These results indicated that the paleo-Changjiang and mainland Chinese rivers dominated the early Holocene deposition in the OT before 9.5 ka BP, despite the paleo-Changjiang shoal and mainland Chinese rivers being submerged and estuaries retreating landward to the Chinese coasts due to sea-level rise (Li et al., 2002). Judging from the canyon setting, we suggest that the Mienhua Canyon and the Huapinghsu Channel can be considered viable sediment conduits for delivery of shelf sediments to the sediment sink of the SOT before 9.5 ka BP. At the end of the Younger Dryaswas (11.55–12.7 ka BP), there was a sudden sea-level rise from about −60 to −45 m below the present level, followed by another rapid 15-m rise between 9.5 and 9.2 ka BP. The Yangtze mouth shifted close to its present position in response to these sea-level rises (Liu et al., 2004). In addition, the paleo ESC shelf-break might have rapidly retreated landward, resulting in less sediment input to these canyons and sediment sourced from Chinese rivers deposited in the SOT (Xu et al., 2019). It is noted that local depocenter of post-glacial fluvial sediments is located near the head of the Huapinghsu Channel (Figure 4D). These deposits could be formed in the past 10 ka BP (Liu et al., 2008). Assumed continuous sediment input to the head of the Huapinghsu Channel from these deposits might have kept this channel/canyon system actively transporting sediments to the SOT via the distal Mienhua Canyon since the 9.5 ka BP. Shen et al. (2021) noted that these sediments derived by Taiwanese rivers are carried northward by TWC and deposited near the head of the Huapinghsu Channel. The deposits were formed after the 7 ka BP (Jin et al., 2021). However, continuous sediment input to the head of the Huapinghsu Channel from the deposits has kept this channel/canyon system actively transporting sediments to the SOT via the distal Mienhua Canyon since the 7 ka BP. The Mienhua Canyon might have become less active 9.5–7 ka BP due to the lack of connection between the head and fluvial sediments.
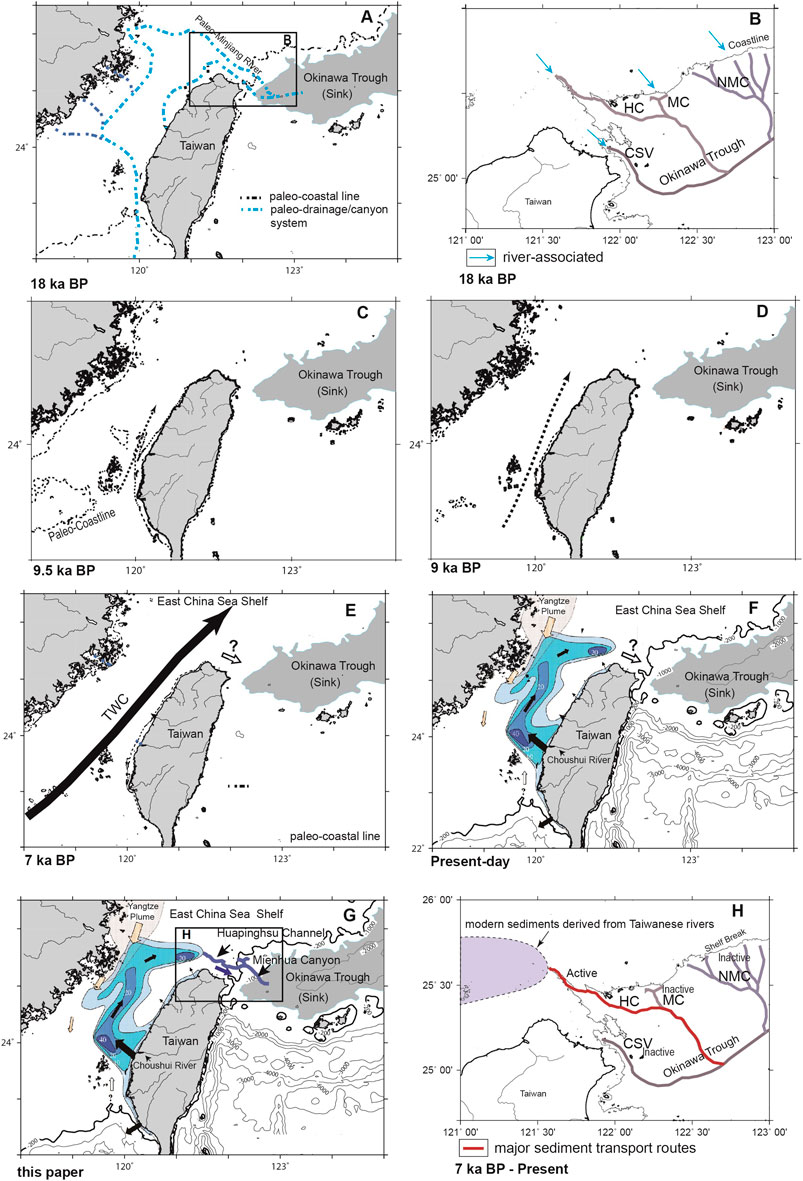
FIGURE 6. The hypothesized distributions of paleo-rivers, channels, and canyons in the Taiwan Strait and the shelf and slope region of East China Sea 18 ka BP. Note that the postulated southeast flowing river/channel/canyon drainages are indicated by blue dotted lines crossing the shelf and slope and extending to the SOT (A) (Boggs et al., 1979). Relatively large amounts of fluvial and near-shore sediments were transported from the ECS shelf to the SOT via the paleo-canyons, including the Mienhua Canyon, in a transverse transport direction during the LGM (B). By about 9.5 ka BP, rising sea level had reached about −35 m below the present level (C). The paleo-Taiwan Strait was restricted to a narrow seaway, allowing ocean currents as indicated by dotted line to flow northwards to the ECS. Shortly after the rise of sea level to −15 m (below the present level) about 9.0 Ka BP, the Taiwan Strait has been submerged nearly to the present shorelines and the north flowing ocean increased in strength and continued to transport sediments derived western Taiwan rivers (D). The birth of the Taiwan Warm Current followed the sea-level rise about 7.0 ka BP (Hsiung and Saito, 2017). After that, it began to transport sediments derived from western Taiwan rivers to the ECS shelf northwest of Taiwan (E). Isopach of the post-glacial sediments mainly derived from Taiwan rivers, shown in blue, is distributed in the Taiwan Strait west of Taiwan. The black arrows represent input and transport of the sediments derived from western Taiwan rivers. The southward flow of the China Coastal Current is denoted by yellow arrows. The open arrow with question mark (?) east of the tip of the isopach map represents unknown transport route of the river-borne sediments from Taiwan (F) (Liu et al., 2008). The results of this study suggested that the head of the Huapinghsu Channel is connected to the temporary sediment sink, with high sediment input, allowing for generation of sediment flows and transportation of river-borne sediments from western Taiwan to the SOT via the channel/canyon system during the present sea-level highstand (G). The variations in sediment provenance in the SOT from the dominance of the paleo-Changjiang to western Taiwan rivers can be explained by the lateral shifting sediment input associated with sea level changes, activities of sediment conduits, and the Taiwan Warm Current (H). HC, Huapinghsu Channel; MC, Mienhua Canyon; NMC, North Mienhua Canyon; CSV, Chilung Sea Valley.
With the subsequent transgression some 5–7 ka BP, when the sea level rose to close to the present position (Figure 2), the entire Huapinghsu Channel was a submerged submarine channel, while the stem of the Mienhua Canyon was a slope-confined canyon far from the present shoreline (Figure 1). As shown in Figure 1, there exists bathymetric continuity of the deepest part of the sea floor along the courses of the Huapinghsu Channel and the distal part of the Mienhua Canyon, allowing inter-connections along their axes to form a continuous bathymetric trough across the shelf and slope region. It is worth noting that the Mienhua Canyon preferentially developed along the western canyon branch, which connects to the distal part of the Huapinghsu Channel to form a channel/canyon transition pathway, allowing shelf sediments to bypass the Huapinghsu Channel and be transported laterally to the Mienhua Canyon. Finally, the modern sediment conduit developed in response to the progressive changes in sea level from lowstand to highstand with shifting sediment input from the head of the Mienhua Canyon (18–9.5 ka BP) to the head of the Huapinghsu Channel from 9.5 ka BP to the present. The shifting of high-sediment input to the Huapinghsu Channel head led to generation of gravity-driven sediment flows, with transport of river-borne sediments from western Taiwan to the ultimate sink of the SOT via the Huapinghsu Channel/Mienhua Canyon System. High sediment input to the head of Huapinghsu Channel during the present sea-level highstand has been supported by a provenance study (Hu et al., 2020). Hu et al. (2020) showed that terrigenous sediment supply from western Taiwan to the SOT progressively increased from 3 ka BP to the present (Figure 2).
Wide continental shelves are commonly considered as an important sediment sink for terrestrial sediments due to very low slope gradient (Nittrouer and Demaster, 1986; Thomas et al., 2004; Shen et al., 2021). In the modern day, the East China Sea shelf with a width more than 500 km is then considered as a sediment sink as much of Yangtze River-derived fine-grained sediments are transported southward by the China Coast Current and deposited along the Zhejiang–Fujian coast. Less sediments from the Yangtze River can be transported across shelf east of the coast of about 120–150 km where the TWC is present. The TWC hinders most cross-shelf coastal sediment transport (Milliman et al., 1989; Bian et al., 2013; Chen et al., 2021). Then, the modern sediments from the Yangtze River are unlikely transported to the outer shelf of East China Sea. On the other hand, the northern Taiwan Strait has high energy with strong currents and high-sediment-yield environment, where sediments derived from Taiwanese rivers, mainly the Choushui River, are carried northward by the north-flowing Taiwan Warm Current and tidal currents and deposited near northern Taiwan island in outer East China Shelf (Liao et al., 2008; Liu et al., 2008; Shen et al., 2021). This study recognized the head of the Huapinghsu Channel being immediately in front of the local depocenter of shelf sediments about 20 m thick sourced from western Taiwan rivers (Figure 1). It is noted that the Huapinghsu Channel may play an important role as a sediment conduit transporting sediment derived from Taiwan rivers along the channel course, with merging into the Mienhua Canyon and eventually into the Southern Okinawa Trough. Nepheloid layers are significant contributors to the cross-shelf transport of particle from the ECS shelf through the Mienhua Canyon (Hung et al., 2003). The linkages between the north-flowing currents in the northern Taiwan Strait, the deposition of sediments derived from western Taiwan rivers, and the sediment conduits of the Huapinghsu Channel and Mienhua Canyon may explain the increase of terrestrial sediment supply from western Taiwan rivers to the final sediment sink of Southern Okinawa Trough over the last 3 ka BP.
6 Implications
More than 30 years ago, Boggs et al. (1979) hypothesized that sediments derived from the northwestern Taiwan drainage basins and Chinese rivers were transported northward by paleo-river channels along the coastline to the offshore areas around northeastern Taiwan where some paleo-channels connect to submarine canyons, flowing southeastward and transporting sediments down to the slope and beyond during the sea-level lowstand about 18 ka BP ago (Figure 6A). In addition, it has been hypothesized that a paleo-river (Minjiang) flowed from the mainland China coast, crossed the entire ECS shelf, and joined an unnamed canyon on the continental slope, delivering terrestrial sediments to the deep sea (Boggs et al., 1979), as shown in Figures 6A, B. With the sea level lowered by 120 m during the Last Glacial Maximum (LGM) (∼21 ka BP), there were direct connections between river mouths and canyons, enhancing delivery of sediment to head areas of channel and canyon (Pratson and Coakley, 1996; Baztan et al., 2005; Deptuck et al., 2007; Jobe et al., 2015; Ribeiro et al., 2021). In the Gulf of Lions, Baztan et al. (2005) suggested that the direct input of fluvial sediments into the canyon head during the Last Glacial Maximum resulted in the formation of narrow (300 m wide) axial incisions, about 100 m deep, cutting across the thalweg of the major erosional trough. However, the Huapinghsu Channel head shows relatively small V-shaped trough with no sediment accumulation. Bathymetric and seismic data indicate that axial incision exists in the Mienhua Canyon. By about 18 ka BP, rising sea level had reached about −100 m below the present level. It is postulated that the axial incision down-cutting the flat floor in the Mienhua Canyon is likely due to erosive sediment flows generated by high-sediment input to the canyon head areas during the last sea-level lowstand about 18 ka BP (Figure 6B). Beginning ∼9.8 ka BP, sea level again rose rapidly, from −36 to −16 m (below the present level) in 800 years (Liu et al., 2004). By about 9.5 ka BP, rising sea level had reached about −35 m below the present level (Figure 6C). The paleo-Taiwan Strait was restricted to a narrow seaway, allowing ocean currents indicated as dotted line to flow northwards to the ECS. Shortly after the rise of sea level to −16 m (below the present level) about 9.0 ka BP, the Taiwan Strait has been submerged nearly to the present shoreline, and the north-flowing ocean currents increased in strength and continued to transport sediments derived from western Taiwan rivers (Figure 6D). The inferred presence of north-flowing ocean currents in the Taiwan Strait during 9.5–9.0 ka BP is based on the early Holocene sediment accumulation near the head of Huapinghsu Channel shown on CHIRP sonar profiles (Liu et al., 2008). The inferred north-flowing ocean currents formed during 9.5–9.0 Ka BP could be considered as the precursor of the Taiwan Warm Current (Hsiung and Saito, 2017).
Hsiung and Saito (2017) pointed out that in the Taiwan Strait, the birth of the TWC occurred following the sea-level rise at 7.3 Ka BP, after which the TWC began to transport sediments derived from western Taiwan rivers to the ECS shelf northwest of Taiwan. They further postulated that these shelf sediments would be transported eastward downslope to the SOT (Figure 6E). It is noted that the sharp provenance change in SOT sediments from mainland China (Figure 6B) to western Taiwan can be linked to the presence of north-flowing TWC (Figure 6E). On the other hand, early sedimentological data indicate that modern terrigenous materials from the Yangtze River are transported not only along the Zhejiang–Fujian coast of the East China Sea but also across the continental shelf (Zhang et al., 2019). The stable position of the fine-grained sediment zone and the coincidence of the coarse-grained area south of its base with the nearshore branch of the Kuroshio Current indicate that the Kuroshio intrusion plays an important role in triggering the cross-shelf flows (Zhang et al., 2019). However, how the modern terrigenous sediments from western Taiwan rivers are carried northward and accumulated on the outer ECS shelf and subsequently are transported to the SOT is not clearly understood (Figure 6F). Several pertinent hypotheses have been proposed. Oceanographic studies have suggested that transport of terrigenous material, mainly made up of fine particles, from the ECS shelf to the SOT takes place via strong wind-driven bottom flows and other current flows (Hu, 1994; Yanagi et al., 1996; Tang et al., 1999; Iseki et al., 2003; Tseng and Shen, 2003). For example, Tang et al. (1999) found intruded Kuroshio water, upwelled water, and shelf water mixed in the Mienhua Canyon head areas with outflow through the canyon, possibly facilitating transportation of particles as well. Using drifter measurements, Tseng and Shen (2003) found that the general flow paths include a deflected eastward Kuroshio Current from the ECS shelf directly passing through the Mienhua Canyon axis downslope. Drifter observations (Tseng and Shen, 2003) together with simulation modelling in the Taiwan Strait (Jan et al., 2004) and cyclonic eddies in northeastern Taiwan (Liu et al., 2003) led Kao et al. (2008) to imply the potential transport of Taiwan muddy sediment to the SOT via the flow path of surface currents. Several studies have suggested that fine-grained sediment discharged from western Taiwan rivers is transported northward into the SOT by local ocean currents (Kao et al., 2008; Liu et al., 2008; Xu et al., 2009; Dou et al., 2016; Hu et al., 2020). These studies have paid attention to the currents or ocean circulations in the sediment transport regime with crossing of the ECS slope and transporting of river-borne sediments from mainland China and Taiwan on the outer shelf to the SOT. However, it has been unclear how submarine canyons as a conduit are serving as a link in the transport of material from shelf edge to the SOT, because evidence of remobilization of shelf edge sediments into the canyon head and then farther transported down-canyon to the SOT are not available.
This study attempts to deal with the important subject relevant for tracking the source and sink of the shelf sediments sourced from northwestern Taiwan rivers delivered to the western end of the SOT (Figure 6G). The Huapinghsu Channel/Mienhua Canyon System as a modern sediment conduit may be supported by bathymetric mapping and seismic profiles, as well as our interpretations. From this, a complete sediment-routing system linking the Choushui River drainage basin (source) to the SOT (sink) can be established. As shown in Figure 6G, the proposed sediment-routing system consists of 1) the Choushui River drainage basin, the main sediment source, in central-western Taiwan, which provides much sediment to the Taiwan Strait; 2) a longitudinal sediment transport route parallel to the strike of the adjacent Taiwan mountain belt, river-borne sediments transported northward mainly by TWC and Kuroshio Current, and formation of a temporary sink of river-borne sediments with sediment thickness up to 20 m on the shelf and close to the Huapinghsu Channel head; 3) the Huapinghsu Channel/Mienhua Canyon System, a distinct morpho-sedimentary feature on the sea floor, a modern sediment conduit receiving sediments from the temporary sink in the head areas and transporting sediments along the channel/canyon course transversely across the shelf and slope region to the deep sea basin; and 4) the SOT as the ultimate sediment sink for deposition of river-borne sediments from western Taiwan rivers particularly during the past 3 ka BP.
This modern sediment conduit of the Huapinghsu Channel/Mienhua Canyon system has developed today in response to the progressive changes in sea level from lowstand to highstand and shifting sediment input from the head of Mienhua Canyon (from 18 to 7 ka BP) to the Huapinghsu Channel head from 7 ka BP to the present (Figure 6H). Subsequent transgression from 7 ka BP to the present has led to a shift in sediment input from the head of the Mienhua Canyon to the head of the Huapinghsu Channel and caused the proximal Mienhua Canyon to be inactive due to cut-off of sediment supply. Continuous sediment input to the head of the Huapinghsu Channel from the local depocenter of shelf sediments has enabled this channel/canyon system to actively transport sediments to the SOT via the distal Mienhua Canyon during the present sea-level highstand (Figure 6H). The variations in sediment provenance in the SOT from the dominance of the paleo-Changjiang to western Taiwan rivers can be explained by the lateral shifting sediment input associated with sea level changes, activities of sediment conduits, and the TWC.
7 Conclusion
Integrated interpretation of morpho-seismic characteristics including new bathymetric data and other published dataset in the region offshore northeast Taiwan has led us to propose a hypothesis that the Huapinghsu Channel/Mienhua Canyon System possibly functions as a sediment conduit for transport of sediments derived from western Taiwan to the SOT (Figure 7).
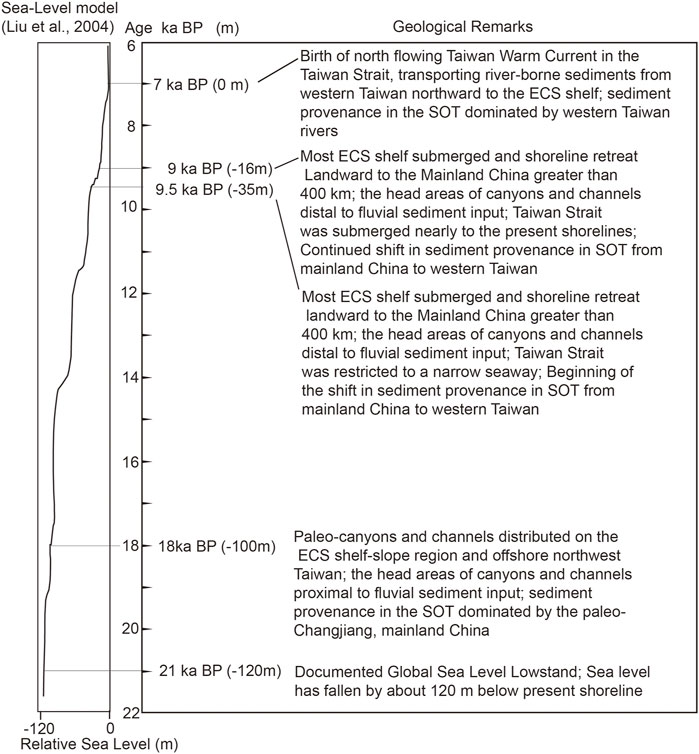
FIGURE 7. Since the last glaciation about 22 ka BP, sea level has risen by about 120 m (Boggs et al., 1979; Liu et al., 2004). As a result, in the study areas, the shoreline has migrated landward, inundating large areas of the ECS shelf. The sea level changes with ages have caused lateral sediment input from mainland China to western Taiwan and consequently the shifting sediment provenance in the SOT from the dominance of the paleo-Changjiang to western Taiwan rivers.
The Huapinghsu Channel extends from its head at the water depth of 120 m seaward across the shelf-slope region and merges with the Mienhua Canyon before finally emptying into the SOT. The river-borne sediment on the shelf edge may be a source of gravity-driven sediment flows into the head of the Huapinghsu Channel because our numerical examination indicates that large waves during huge typhoons or strong oceanic currents satisfy the critical values to threshold of motion of fine sand even deposited on the shelf edge. Within the proximal reach of the Mienhua Canyon, in situ resuspensed particles were found associated to nepheloid layers, which transport resuspended particles along the canyon course and eventually into the SOT even during sea-level highstand. The refined morpho-sedimentary features and presented scientific literature of this channel/canyon system may shed light on the alternative explanation for the regional sediment dispersal along the vast ECS shelf since the last sea-level lowstand. A summary in Figure 7 is presented to show that in the study areas, the sea level changes since 22 ka BP with ages have caused concordant sedimentary processes, including lateral sediment input from mainland China to western Taiwan with the birth of the Taiwan Warm Current and consequently the shifting sediment provenance in the SOT from the dominance of the paleo-Changjiang to western Taiwan rivers.
Data Availability Statement
The original contributions presented in the study are included in the article/Supplementary Material; further inquiries can be directed to the corresponding author.
Author Contributions
C-SC made significant contributions in the conceptualization, data processing, interpretation, methodology, and original draft preparation. H-SY made significant contributions in the morpho-seismic interpretation and manuscript reviewing. AN made significant contributions in the seismic interpretation and numerical examinations. TT made significant contributions in the seismic interpretation. All authors contributed to the article and approved the submitted version.
Funding
This study was financially supported in part by the Ministry of Science and Technology (MOST 109-2116-M-178-003), Taiwan.
Conflict of Interest
The authors declare that the research was conducted in the absence of any commercial or financial relationships that could be construed as a potential conflict of interest.
Publisher’s Note
All claims expressed in this article are solely those of the authors and do not necessarily represent those of their affiliated organizations, or those of the publisher, the editors and the reviewers. Any product that may be evaluated in this article, or claim that may be made by its manufacturer, is not guaranteed or endorsed by the publisher.
Acknowledgments
We greatly appreciate the use of bathymetric and seismic reflection profiles from the Ocean Data Bank of the National Center for Ocean Research, Taiwan. Constructive comments and valuable suggestions from the editor and two reviewers greatly improved the quality of this paper.
References
Baztan, J., Berné, S., Olivet, J.-L., Rabineau, M., Aslanian, D., Gaudin, M., et al. (2005). Axial Incision: The Key to Understand Submarine Canyon Evolution (In the Western Gulf of Lion). Mar. Pet. Geology. 22, 805–826. doi:10.1016/j.marpetgeo.2005.03.011
Bentahila, Y., Ben Othman, D., and Luck, J.-M. (2008). Strontium, Lead and Zinc Isotopes in marine Cores as Tracers of Sedimentary Provenance: A Case Study Around Taiwan Orogen. Chem. Geology. 248, 62–82. doi:10.1016/j.chemgeo.2007.10.024
Bernhardt, A., Melnick, D., Jara-Muñoz, J., Argandoña, B., González, J., and Strecker, M. R. (2015). Controls on Submarine Canyon Activity during Sea-Level Highstands: The Biobío Canyon System Offshore Chile. Geosphere 11, 1226–1255. doi:10.1130/GES01063.1
Bian, C., Jiang, W., Quan, Q., Wang, T., Greatbatch, R. J., and Li, W. (2013). Distributions of Suspended Sediment Concentration in the Yellow Sea and the East China Sea Based on Field Surveys during the Four Seasons of 2011. J. Mar. Syst. 121-122, 24–35. doi:10.1016/j.jmarsys.2013.03.013
Boggs, S. J., Wang, W. C., Lewis, F. S., and Chen, J. C. (1979). Sediment Properties and Water Characteristics of the Taiwan Shelf and Slope. Acta Oceanogr Taiwanica 10, 10–49.
Canals, M., Company, J. B., Martín, D., Sànchez-Vidal, A., and Ramírez-Llodrà, E. (2013). Integrated Study of Mediterranean Deep Sea Canyons: Novel Results and Future Challenges. Prog. Oceanography 118, 1–27. doi:10.1016/j.pocean.2013.09.004
Chang, J.-H., Yang, E. Y.-C., Hsu, H.-H., Chen, T.-T., Liu, C.-S., and Chiu, S.-D. (2021). Igneous Activity and Structural Development of the Mianhua Terrace, Offshore North Taiwan. Minerals 11, 303. doi:10.3390/min11030303
Chen, C.-T. A., Kandasamy, S., Chang, Y.-P., Bai, Y., He, X., Lu, J.-T., et al. (2017). Geochemical Evidence of the Indirect Pathway of Terrestrial Particulate Material Transport to the Okinawa Trough. Quat. Int. 441, 51–61. doi:10.1016/j.quaint.2016.08.006
Chen, S.-C., Lin, J.-Y., Su, C.-C., and Doo, W.-B. (2019). Introduction to the Special Issue on Tectonic Environment and Seabed Resources of the Southern Okinawa Trough. Terr. Atmos. Ocean. Sci. 30, 605–611. doi:10.3319/TAO.2019.08.27.01
Chen, K., Kuang, C., Wang, Y., Wang, T., and Bian, C. (2021). Cross-Shelf Sediment Transport in the Yangtze Delta Frontal Zone: Insights from Field Observations. J. Mar. Syst. 219, 103559. doi:10.1016/j.jmarsys.2021.103559
Chern, C.-S., and Wang, J. (1994). Influence of the Seasonal Thermocline on the Intrusion of Kuroshio across the continental Shelf Northeast of Taiwan. J. Oceanogr. 50, 691–711. doi:10.1007/bf02270500
Chiang, C.-S., and Yu, H.-S. (2006). Morphotectonics and Incision of the Kaoping Submarine canyon, SW Taiwan Orogenic Wedge. Geomorphology 80, 199–213. doi:10.1016/j.geomorph.2006.02.008
Chiang, C.-S., Yu, H.-S., Noda, A., TuZino, T., and Su, C.-C. (2012). Avulsion of the Fangliao Submarine canyon off Southwestern Taiwan as Revealed by Morphological Analysis and Numerical Simulation. Geomorphology 177-178, 26–37. doi:10.1016/j.geomorph.2012.07.011
Chiang, C.-S., Hsiung, K.-H., Yu, H.-S., and Chen, S.-C. (2020). Three Types of Modern Submarine Canyons on the Tectonically Active Continental Margin Offshore Southwestern Taiwan. Mar. Geophys. Res. 41, 4. doi:10.1007/s11001-020-09403-z
Chuang, W.-S., Li, H.-W., Tang, T. Y., and Wu, C.-K. (1993). Observations of the Countercurrent on the Inshore Side of the Kuroshio Northeast of Taiwan. J. Oceanogr. 49, 581–592. doi:10.1007/BF02237464
Chung, Y., and Chang, W. C. (1995). Pb-210 Fluxes and Sedimentation Rates on the Lower Continental Slope between Taiwan and the South Okinawa Trough. Continental Shelf Res. 15, 149–164. doi:10.1016/0278-4343(94)E0023-F
Covault, J. A., and Graham, S. A. (2010). Submarine Fans at All Sea-Level Stands: Tectono-Morphologic and Climatic Controls on Terrigenous Sediment Delivery to the Deep Sea. Geology 38, 939–942. doi:10.1130/G31081.1
Covault, J. A., Normark, W. R., Romans, B. W., and Graham, S. A. (2007). Highstand Fans in the California Borderland: The Overlooked Deep-Water Depositional Systems. Geology 35, 783–786. doi:10.1130/G23800A.1
Dadson, S. J., Hovius, N., Chen, H., Dade, W. B., Hsieh, M.-L., Willett, S. D., et al. (2003). Links between Erosion, Runoff Variability and Seismicity in the Taiwan Orogen. Nature 426, 648–651. doi:10.1038/nature02150
Deptuck, M. E., Sylvester, Z., Pirmez, C., and O’Byrne, C. (2007). Migration-Aggradation History and 3-D Seismic Geomorphology of Submarine Channels in the Pleistocene Benin-Major Canyon, Western Niger Delta Slope. Mar. Pet. Geology. 24, 406–433. doi:10.1016/j.marpetgeo.2007.01.005
Diekmann, B., Hofmann, J., Henrich, R., Fütterer, D. K., Röhl, U., and Wei, K.-Y. (2008). Detrital Sediment Supply in the Southern Okinawa Trough and its Relation to Sea-Level and Kuroshio Dynamics during the Late Quaternary. Mar. Geology. 255, 83–95. doi:10.1016/j.margeo.2008.08.001
Dou, Y., Yang, S., Shi, X., Clift, P. D., Liu, S., Liu, J., et al. (2016). Provenance Weathering and Erosion Records in Southern Okinawa Trough Sediments since 28 Ka: Geochemical and Sr-Nd-Pb Isotopic Evidences. Chem. Geology. 425, 93–109. doi:10.1016/j.chemgeo.2016.01.029
Fan, S., Swift, D. J. P., Traykovski, P., Bentley, S., Borgeld, J. C., Reed, C. W., et al. (2004). River Flooding, Storm Resuspension, and Event Stratigraphy on the Northern California Shelf: Observations Compared with Simulations. Mar. Geology. 210, 17–41. doi:10.1016/j.margeo.2004.05.024
Farre, J. A., McGregor, B. A., Ryan, W. B. F., and Robb, J. M. (1983). “Breaching the Shelfbreak: Passage from Youthful to Mature Phase in Submarine Canyon Evolution,” in The Shelf Break. Editors DJ Stanley, and GT Moore (Tulsa, OK: SEPM Spec. Publ), 33, 25–39. doi:10.2110/pec.83.06.0025
Gardner, W. D. (1989). Periodic Resuspension in Baltimore Canyon by Focusing of Internal Waves. J. Geophys. Res. 94, 18185–18194. doi:10.1029/JC094iC12p18185
Haalboom, S., de Stigter, H., Duineveld, G., van Haren, H., Reichart, G.-J., and Mienis, F. (2021). Suspended Particulate Matter in a Submarine canyon (Whittard Canyon, Bay of Biscay, NE Atlantic Ocean): Assessment of Commonly Used Instruments to Record Turbidity. Mar. Geology. 434, 106439. doi:10.1016/j.margeo.2021.106439
Hagen, R. A., Bergersen, D. D., Moberly, R., and Coulbourn, W. T. (1994). Morphology of a Large Meandering Submarine canyon System on the Peru-Chile Forearc. Mar. Geology. 119, 7–38. doi:10.1016/0025-3227(94)90138-4
Hsiung, K.-H., and Saito, Y. (2017). Sediment Trapping in Deltas of Small Mountainous Rivers of Southwestern Taiwan and its Influence on East China Sea Sedimentation. Quat. Int. 455, 30–44. doi:10.1016/j.quaint.2017.02.020
Hsu, S.-C., Lin, F.-J., Jeng, W.-L., Chung, Y.-c., Shaw, L.-M., and Hung, K.-W. (2004). Observed Sediment Fluxes in the Southwesternmost Okinawa Trough Enhanced by Episodic Events: Flood Runoff from Taiwan Rivers and Large Earthquakes. Deep Sea Res. Oceanographic Res. Pap. 51, 979–997. doi:10.1016/J.DSR.2004.01.009
Hu, S., Zeng, Z., Fang, X., Yin, X., Chen, Z., Li, X., et al. (2020). Increasing Terrigenous Sediment Supply from Taiwan to the Southern Okinawa Trough over the Last 3000 Years Evidenced by Sr Nd Isotopes and Geochemistry. Sediment. Geology. 406, 105725. doi:10.1016/j.sedgeo.2020.105725
Hu, D. (1994). “Possible Mechanisms for Material Flux in the Yellow Sea and East China Sea from Hydrographic Viewpoint,” in Global Fluxes of Carbon and its Related Substances in the Coastal Sea-Ocean-Atmosphere System. Editors S Tsunogai, K Iseki, I Koike, and T Oba (Yokohama, Japan: M & J. International).
Huh, C.-A., Su, C.-C., Liang, W.-T., and Ling, C.-Y. (2004). Linkages between Turbidites in the Southern Okinawa Trough and Submarine Earthquakes. Geophys. Res. Lett. 31, L12304. doi:10.1029/2004GL019731
Hung, J.-J., Lin, C.-S., Chung, Y.-C., Hung, G.-W., and Liu, W.-S. (2003). Lateral Fluxes of Biogenic Particles through the Mien-Hua canyon in the Southern East China Sea Slope. Continental Shelf Res. 23, 935–955. doi:10.1016/S0278-4343(03)00085-2
Iseki, K., Okamura, K., and Kiyomoto, Y. (2003). Seasonality and Composition of Downward Particulate Fluxes at the continental Shelf and Okinawa Trough in the East China Sea. Deep Sea Res. Part Topical Stud. Oceanography 50, 457–473. doi:10.1016/S0967-0645(02)00468-X
Jan, S., Chern, C.-S., Wang, J., and Chao, S.-Y. (2004). The Anomalous Amplification of M2tide in the Taiwan Strait. Geophys. Res. Lett. 31, L07308. doi:10.1029/2003GL019373
Jin, L., Shan, X., Shi, X., Fonnesu, M., Qiao, S., Kandasamy, S., et al. (2021). Hybrid Event Beds Generated by Erosional Bulking of Modern Hyperpycnal Flows on the Choshui River delta Front, Taiwan Strait. Sedimentology 68, 2500–2522. doi:10.1111/sed.12862
Jobe, Z. R., Sylvester, Z., Parker, A. O., Howes, N., Slowey, N., and Pirmez, C. (2015). Rapid Adjustment of Submarine Channel Architecture to Changes in Sediment Supply. J. Sediment. Res. 85, 729–753. doi:10.2110/jsr.2015.30
Kao, S. J., Lin, F. J., and Liu, K. K. (2003). Organic Carbon and Nitrogen Contents and Their Isotopic Compositions in Surficial Sediments from the East China Sea Shelf and the Southern Okinawa Trough. Deep Sea Res. Part Topical Stud. Oceanography 50, 1203–1217. doi:10.1016/S0967-0645(03)00018-3
Kao, S.-J., Jan, S., Hsu, S.-C., Lee, T.-Y., and Dai, M. (2008). Sediment Budget in the Taiwan Strait with High Fluvial Sediment Inputs from Mountainous Rivers: New Observations and Synthesis. Terr. Atmos. Ocean. Sci. 19, 525–546. doi:10.3319/tao.2008.19.5.525(oc)
Kimura, M. (1983). Submarine Topography Around Ryukyu Arc. Scale 1: 180,000., 1 Sheet. Naha City, Japan: Okinawa Times Company.
Kimura, M. (1985). Back-arc Rifting in the Okinawa Trough. Mar. Pet. Geology. 2, 222–240. doi:10.1016/0264-8172(85)90012-1
Komar, P. D., and Miller, M. C. (1975). On the Comparison between the Threshold of Sediment Motion Under Waves and Unidirectional Currents with a Discussion of the Practical Evaluation of the Threshold: REPLY. J. Sediment. Petro. 45, 362–367. doi:10.1306/212F6D66-2B24-11D7-8648000102C1865D
Lallemand, S. E., Liu, C. S., Angelier, J., Collot, J. Y., Deffontaines, B., Dominguez, S., et al. (1996). ACT Cruise Report. Marseille, France: Instituts National des Sciences de l'Univers.
Laursen, J., and Normark, W. R. (2002). Late Quaternary Evolution of the San Antonio Submarine Canyon in the central Chile Forearc (∼33°S). Mar. Geology. 188, 365–390. doi:10.1016/S0025-3227(02)00421-8
Li, C., Wang, P., Sun, H., Zhang, J., Fan, D., and Deng, B. (2002). Late Quaternary Incised-Valley Fill of the Yangtze Delta (China): Its Stratigraphic Framework and Evolution. Sediment. Geology. 152, 133–158. doi:10.1016/s0037-0738(02)00066-0
Li, C., Francois, R., Yang, S., Barling, J., Darfeuil, S., Luo, Y., et al. (2016). Constraining the Transport Time of Lithogenic Sediments to the Okinawa Trough (East China Sea). Chem. Geology. 445, 199–207. doi:10.1016/j.chemgeo.2016.04.010
Liang, W.-T., Lee, J.-C., and Hsiao, N.-C. (2019). Crowdsourcing Platform Toward Seismic Disaster Reduction: The Taiwan Scientific Earthquake Reporting (TSER) System. Front. Earth Sci. 7, 79. doi:10.3389/feart.2019.00079
Liao, H.-R., Yu, H.-S., and Su, C.-C. (2008). Morphology and Sedimentation of Sand Bodies in the Tidal Shelf Sea of Eastern Taiwan Strait. Mar. Geology. 248 (3–4), 161–178. doi:10.1016/j.margeo.2007.10.013
Lin, A. T., and Watts, A. B. (2002). Origin of the West Taiwan Basin by Orogenic Loading and Flexure of a Rifted Continental Margin. J. Geophys. Res. 107, 2185. doi:10.1029/2001JB000669
Lin, C.-Y., Shyu, C.-Z., and Shih, W.-H. (1992). The Kuroshio Fronts and Cold Eddies off Northeastern Taiwan Observed by NOAA-AVHRR Imageries. Terr. Atmos. Ocean. Sci. 3, 225–242. doi:10.3319/tao.1992.3.3.225(keep)
Liu, K.-K., Peng, T.-H., Shaw, P.-T., and Shiah, F.-K. (2003). Circulation and Biogeochemical Processes in the East China Sea and the Vicinity of Taiwan: An Overview and a Brief Synthesis. Deep Sea Res. Part Topical Stud. Oceanography 50, 1055–1064. doi:10.1016/S0967-0645(03)00009-2
Liu, J. P., Milliman, J. D., Gao, S., and Cheng, P. (2004). Holocene Development of the Yellow River's Subaqueous delta, North Yellow Sea. Mar. Geology. 209, 45–67. doi:10.1016/j.margeo.2004.06.009
Liu, J. P., Liu, C. S., Xu, K. H., Milliman, J. D., Chiu, J. K., Kao, S. J., et al. (2008). Flux and Fate of Small Mountainous Rivers Derived Sediments into the Taiwan Strait. Mar. Geology. 256, 65–76. doi:10.1016/J.MARGEO.2008.09.007
Liu, X., Li, A., Fike, D. A., Dong, J., Xu, F., Zhuang, G., et al. (2020). Environmental Evolution of the East China Sea Inner Shelf and its Constraints on Pyrite Sulfur Contents and Isotopes since the Last Deglaciation. Mar. Geology. 429, 106307. doi:10.1016/j.margeo.2020.106307
Lo, C.-L., and Hsu, S.-K. (2005). Earthquake-Induced Gravitational Potential Energy Change in the Active Taiwan Orogenic belt. Geophys. J. Int. 162, 169–176. doi:10.1111/j.1365-246X.2005.02634.x
Maier, K. L., Gales, J. A., Paull, C. K., Rosenberger, K., Talling, P. J., Simmons, S. M., et al. (2019). Linking Direct Measurements of Turbidity Currents to Submarine Canyon-Floor Deposits. Front. Earth Sci. 7, 144. doi:10.3389/feart.2019.00144
Marsset, B., Sibuet, J. C., Letouzey, J., and Maze, J. P. (1987). Bathymetric Map of the Okinawa Trough. Brest, France: IFREMER. Scale: 1:1000000.
Micallef, A., Mountjoy, J. J., Barnes, P. M., Canals, M., and Lastras, G. (2014). Geomorphic Response of Submarine Canyons to Tectonic Activity: Insights from the Cook Strait canyon System, New Zealand. Geosphere 10, 905–929. doi:10.1130/GES01040.1
Milliman, J. D., Qin, Y., and Park, Y. (1989). “Sediments and Sedimentary Processes in the Yellow and East China Seas,” in Sedimentary Facies in the Active Plate Margin. Editors A. Taira, and F. Masuda (Tokyo: Terra Scientific Publishing Company), 233–249.
Nittrouer, C. A., and Demaster, D. J. (1986). Sedimentary Processes on the Amazon continental Shelf - Past, Present and Future-Research. Cont. Shelf Res. 6 (1–2), 5–30. doi:10.1016/0278-4343(86)90051-8
Noda, A., TuZino, T., Furukawa, R., Joshima, M., and Uchida, J.-I. (2008). Physiographical and Sedimentological Characteristics of Submarine Canyons Developed upon an Active Forearc Slope: the Kushiro Submarine Canyon, Northern Japan. Geol. Soc. America Bull. 120, 750–767. doi:10.1130/B26155.1
Normandeau, A., Lajeunesse, P., and St-Onge, G. (2015). Submarine Canyons and Channels in the Lower St. Lawrence Estuary (Eastern Canada): Morphology, Classification and Recent Sediment Dynamics. Geomorphology 241, 1–18. doi:10.1016/j.geomorph.2015.03.023
Ou, S.-H., Liau, J.-M., Hsu, T.-W., and Tzang, S.-Y. (2002). Simulating Typhoon Waves by SWAN Wave Model in Coastal Waters of Taiwan. Ocean Eng. 29, 947–971. doi:10.1016/s0029-8018(01)00049-x
Palanques, A., Guillén, J., Puig, P., and Durrieu de Madron, X. (2008). Storm-Driven Shelf-To-canyon Suspended Sediment Transport at the Southwestern Gulf of Lions. Continental Shelf Res. 28, 1947–1956. doi:10.1016/j.csr.2008.03.020
Piper, D. J. W., and Normark, W. R. (2009). Processes that Initiate Turbidity Currents and Their Influence on Turbidites: A marine Geology Perspective. J. Sediment. Res. 79, 347–362. doi:10.2110/jsr.2009.046
Popescu, I., Lericolais, G., Panin, N., Normand, A., Dinu, C., and Le Drezen, E. (2004). The Danube Submarine Canyon (Black Sea): Morphology and Sedimentary Processes. Mar. Geology. 206, 249–265. doi:10.1016/j.margeo.2004.03.003
Pratson, L. F., and Coakley, B. J. (1996). A Model for the Headward Erosion of Submarine Canyons Induced by Downslope-Eroding Sediment Flows. Geol. Soc. Am. Bull. 108, 225–234. doi:10.1130/0016-7606(1996)108<0225:amfthe>2.3.co;2
Puig, P., Ogston, A. S., Mullenbach, B. L., Nittrouer, C. A., Parsons, J. D., and Sternberg, R. W. (2004). Storm-induced Sediment Gravity Flows at the Head of the Eel Submarine Canyon, Northern California Margin. J. Geophys. Res. 109, C03019. doi:10.1029/2003JC001918
Puig, P., Palanques, A., and Martín, J. (2014). Contemporary Sediment-Transport Processes in Submarine Canyons. Annu. Rev. Mar. Sci. 6, 53–77. doi:10.1146/annurev-marine-010213-135037
Puig, P., Durán, R., Muñoz, A., Elvira, E., and Guillén, J. (2017). Submarine Canyon-Head Morphologies and Inferred Sediment Transport Processes in the Alías-Almanzora Canyon System (SW Mediterranean): On the Role of the Sediment Supply. Mar. Geology. 393, 21–34. doi:10.1016/j.margeo.2017.02.009
Ribeiro, R. F., Dominguez, J. M. L., Santos, A. A., and Rangel, A. G. D. A. N. (2021). Continuous Canyon-River Connection on a Passive Margin: The Case of São Francisco Canyon (Eastern Brazil). Geomorphology 375, 107549. doi:10.1016/j.geomorph.2020.107549
Shen, X., Jian, X., Li, C., Liu, J. T., Chang, Y.-P., Zhang, S., et al. (2021). Submarine Topography-Related Spatial Variability of the Southern Taiwan Strait Sands (East Asia). Mar. Geology. 436, 106495. doi:10.1016/j.margeo.2021.106495
Shepard, F. P. (1981). Submarine Canyons: Multiple Causes and Long-Time Persistence. AAPG Bull. 65, 1062–1077. doi:10.1306/03b59459-16d1-11d7-8645000102c1865d
Shih, H.-J., Chen, H., Liang, T.-Y., Fu, H.-S., Chang, C.-H., Chen, W.-B., et al. (2018). Generating Potential Risk Maps for Typhoon-Induced Waves along the Coast of Taiwan. Ocean Eng. 163, 1–14. doi:10.1016/j.oceaneng.2018.05.045
Sibuet, J.-C., Deffontaines, B., Hsu, S.-K., Thareau, N., Le Formal, J.-P., and Liu, C.-S. (1998). Okinawa Trough Backarc Basin: Early Tectonic and Magmatic Evolution. J. Geophys. Res. 103, 30245–30267. doi:10.1029/98JB01823
Sømme, T. O., Helland-Hansen, W., Martinsen, O. J., and Thurmond, J. B. (2009). Relationships between Morphological and Sedimentological Parameters in Source-To-Sink Systems: A Basis for Predicting Semi-Quantitative Characteristics in Subsurface Systems. Basin Res. 21, 361–387. doi:10.1111/j.1365-2117.2009.00397.x
Song, G. S., and Chang, Y. C. (1993). Comment on Naming of the Submarine Canyons off Northeastern Taiwan: A Note by Ho-Shing Yu (1992). Acta Oceanogr Taiwanica 30, 77–84.
Song, G. S., Chang, Y. C., and Ma, C. P. (1997). Characteristics of Submarine Topography Off Northern Taiwan. Terr. Atmos. Ocean. Sci. 8 (4), 461–480. doi:10.3319/TAO.1997.8.4.461(O)
Song, G.-S., Ma, C.-P., and Yu, H.-S. (2000). Fault-Controlled Genesis of the Chilung Sea Valley (Northern Taiwan) Revealed by Topographic Lineaments. Mar. Geology. 169, 305–325. doi:10.1016/S0025-3227(00)00084-0
Suppe, J. (1981). Mechanics of Mountain Building and Metamorphism in Taiwan. Mem. Geol. Soc. China 4, 67–89.
Tang, T. Y., and Yang, Y. J. (1993). Low Frequency Current Variability on the Shelf Break Northeast of Taiwan. J. Oceanogr. 49, 193–210. doi:10.1007/BF02237288
Tang, T. Y., Hsueh, Y., Yang, Y. J., and Ma, J. C. (1999). Continental Slope Flow Northeast of Taiwan. J. Phys. Oceanogr. 29, 1353–1362. doi:10.1175/1520-0485(1999)029<1353:csfnot>2.0.co;2
Tang, T. Y., Tai, J. H., and Yang, Y. J. (2000). The Flow Pattern North of Taiwan and the Migration of the Kuroshio. Continental Shelf Res. 20, 349–371. doi:10.1016/S0278-4343(99)00076-X
Teng, L. S. (1990). Geotectonic Evolution of Late Cenozoic Arc-Continent Collision in Taiwan. Tectonophysics 183, 57–76. doi:10.1016/0040-1951(90)90188-E
Teng, L. S. (1996). Extensional Collapse of the Northern Taiwan Mountain Belt. Geology 24, 949–952. doi:10.1130/0091-7613(1996)024<0949:ecotnt>2.3.co;2
Thomas, H., Bozec, Y., Elkalay, K., and De Baar, H. J. W. (2004). Enhanced Open Ocean Storage of CO 2 from Shelf Sea Pumping. Science 304, 56731005–56731008. doi:10.1126/science.1095491
Tsai, C.-H., Huang, C.-L., Hsu, S.-K., Doo, W.-B., Lin, S.-S., Wang, S.-Y., et al. (2018). Active Normal Faults and Submarine Landslides in the Keelung Shelf off NE Taiwan. Terr. Atmos. Ocean. Sci. 29, 31–38. doi:10.3319/TAO.2017.07.02.01
Tseng, R.-S., and Shen, Y.-T. (2003). Lagrangian Observations of Surface Flow Patterns in the Vicinity of Taiwan. Deep Sea Res. Part Topical Stud. Oceanography 50, 1107–1115. doi:10.1016/S0967-0645(03)00012-2
Walsh, J. P., and Nittrouer, C. A. (2003). Contrasting Styles of Off-Shelf Sediment Accumulation in New Guinea. Mar. Geology. 196, 105–125. doi:10.1016/S0025-3227(03)00069-0
Wang, C., and Hilde, T. W. C. (1973). Geomagnetic Interpretation of the Geologic Structure in the Northeast Offshore Region of Taiwan. Acta Oceanogr. Taiwanica 3, 141–156.
Wang, J., Li, A., Xu, K., Zheng, X., and Huang, J. (2015). Clay Mineral and Grain Size Studies of Sediment Provenances and Paleoenvironment Evolution in the Middle Okinawa Trough since 17ka. Mar. Geology. 366, 49–61. doi:10.1016/j.margeo.2015.04.007
Wave Statistics at Pengjiayu Buoy (2020). Taiwan: Central Weather Bureau. Available at https://www.cwb.gov.tw/V8/E/C/MMC_STAT/sta_wave.html.
Wei, K. Y., Mii, H. S., and Huang, C. Y. (2005). Age Model and Oxygen Isotope Stratigraphy of Site ODP1202 in the Southern Okinawa Trough, Northwestern Pacific. Terr, Atmos. Ocean Sci. 16, 1–17. doi:10.3319/tao.2005.16.1.1(ot)
Wei, G., Li, X.-H., Liu, Y., Shao, L., and Liang, X. (2006). Geochemical Record of Chemical Weathering and Monsoon Climate Change since the Early Miocene in the South China Sea. Paleoceanography 21, PA4214. doi:10.1029/2006PA001300
Wiles, E., Green, A., Watkeys, M., Botes, R., and Jokat, W. (2019). Submarine Canyons of NW Madagascar: a First Geomorphological Insight. Deep Sea Res. Part Topical Stud. Oceanography 161, 5–15. doi:10.1016/j.dsr2.2018.06.003
Wilson, A. M., Raine, R., Mohn, C., and White, M. (2015). Nepheloid Layer Distribution in the Whittard Canyon, NE Atlantic Margin. Mar. Geology. 367, 130–142. doi:10.1016/j.margeo.2015.06.002
Xu, K., Milliman, J. D., Li, A., Paul Liu, J., Kao, S.-J., and Wan, S. (2009). Yangtze- and Taiwan-Derived Sediments on the Inner Shelf of East China Sea. Continental Shelf Res. 29, 2240–2256. doi:10.1016/j.csr.2009.08.017
Xu, Z., Lim, D., Li, T., Kim, S., Jung, H., Wan, S., et al. (2019). REEs and Sr-Nd Isotope Variations in a 20 Ky-Sediment Core from the Middle Okinawa Trough, East China Sea: An In-Depth Provenance Analysis of Siliciclastic Components. Mar. Geology. 415, 105970. doi:10.1016/j.margeo.2019.105970
Yanagi, T., Takahashi, S., Hoshika, A., and Tanimoto, T. (1996). Seasonal Variation in the Transport of Suspended Matter in the East China Sea. J. Oceanogr. 52, 539–552. doi:10.1007/BF02238320
Yin, W., and Huang, D. (2019). Short‐Term Variations in the Surface Upwelling off Northeastern Taiwan Observed via Satellite Data. J. Geophys. Res. Oceans 124, 939–954. doi:10.1029/2018JC014537
Yin, Y., Liu, Z., Hu, P., Hou, Y., Lu, J., and He, Y. (2020). Impact of Mesoscale Eddies on the Southwestward Countercurrent Northeast of Taiwan Revealed by ADCP Mooring Observations. Continental Shelf Res. 195, 104063. doi:10.1016/j.csr.2020.104063
Yu, H. S., and Hong, E. (2006). Shifting Submarine Canyons and Development of a Foreland Basin in SW Taiwan: Controls of Foreland Sedimentation and Longitudinal Sediment Transport. J. Asian Earth Sci. 27, 922–932. doi:10.1016/j.jseaes.2005.09.007
Yu, H.-S., and Chou, Y.-W. (2001). Characteristics and Development of the Flexural Forebulge and Basal Unconformity of Western Taiwan Foreland Basin. Tectonophysics 333, 277–291. doi:10.1016/S0040-1951(00)00279-1
Yu, H.-S., and Hong, E. (1993). The Huapinghsu Channel/canyon System off Northeastern Taiwan: Morphology, Sediment Character and Origin. Terr. Atmos. Ocean. Sci. 4, 307–319. doi:10.3319/tao.1993.4.3.307(o)
Yu, H.-S., and Lee, M.-L. (1998). Morphological and Seismic Characteristics of the North Mien-Hua Submarine Cany on off Northeastern Taiwan. Terr. Atmos. Ocean. Sci. 9, 263–278. doi:10.3319/tao.1998.9.2.263(o)
Zhang, K., Li, A., Huang, P., Lu, J., Liu, X., and Zhang, J. (2019). Sedimentary Responses to the Cross-Shelf Transport of Terrigenous Material on the East China Sea continental Shelf. Sediment. Geology. 384, 50–59. doi:10.1016/j.sedgeo.2019.03.006
Keywords: Huapinghsu Channel, Mienhua Canyon, modern sediment conduit, morpho-seismic features, Okinawa Trough, Taiwan
Citation: Chiang C-, Yu H-, Noda A and TuZino T (2022) The Huapinghsu Channel/Mienhua Canyon System as a Sediment Conduit Transporting Sediments From Offshore North Taiwan to the Southern Okinawa Trough. Front. Earth Sci. 9:792595. doi: 10.3389/feart.2021.792595
Received: 10 October 2021; Accepted: 14 December 2021;
Published: 10 February 2022.
Edited by:
Xiting Liu, Ocean University of China, ChinaReviewed by:
Kan-Hsi Hsiung, Japan Agency for Marine-Earth Science and Technology (JAMSTEC), JapanDeyong Li, Ocean University of China, China
Copyright © 2022 Chiang, Yu, Noda and TuZino. This is an open-access article distributed under the terms of the Creative Commons Attribution License (CC BY). The use, distribution or reproduction in other forums is permitted, provided the original author(s) and the copyright owner(s) are credited and that the original publication in this journal is cited, in accordance with accepted academic practice. No use, distribution or reproduction is permitted which does not comply with these terms.
*Correspondence: Cheng-Shing Chiang, d2luZEBtYWlsLm5tbnMuZWR1LnR3