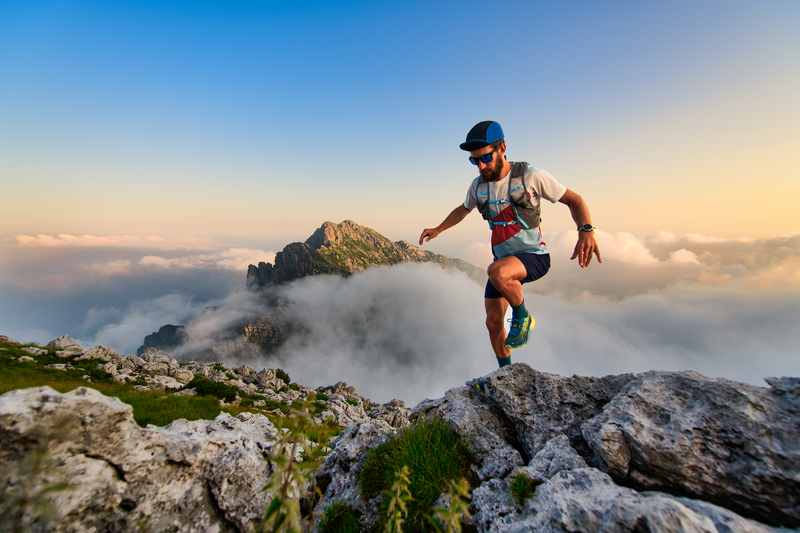
95% of researchers rate our articles as excellent or good
Learn more about the work of our research integrity team to safeguard the quality of each article we publish.
Find out more
REVIEW article
Front. Earth Sci. , 21 January 2022
Sec. Structural Geology and Tectonics
Volume 9 - 2021 | https://doi.org/10.3389/feart.2021.786915
This article is part of the Research Topic Tectonic Faults: Types, Characteristics and Behavior View all 7 articles
Polygonal faults (PFs) have been widely found in over 100 sedimentary basins worldwide, mainly in marine setting on continental margins and intracratonic sedimentary basins. PFs are characterized by layer-bound minor normal faults arranged in polygonal patterns and multi-direction strikes, most of which were developed in fine-grained sediments rich in claystone. The genesis mechanisms of PFs have been recognized as non-tectonic, mainly including shear failure, syneresis, density inversion, low coefficients of friction and gravity sliding theory. Previous studies often regarded PFs as conduits playing a role in favouring fluid migration, which were also regarded as fluid source expelled during PF generation. However, some researchers proposed that the PF-bearing layer is generally impermeable and may act as a seal. To better understand the role of PFs in fluid migration and their contrition to the shallower hydrocarbon accumulation, a geophysical review of global PFs case studies is conducted in this paper. A comprehensive analysis is carried out based on abundant case studies, with key parameters analyzed including the PFs characteristics (e.g., buried depth, lithology, size, etc.), formation mechanisms of PFs, tectonic-sedimentary setting, and the spatial relationship of PFs, nearby major fluid migration pathways and high amplitude anomalies. The compilation of global PF case studies of shows that hydrocarbon fluids from the deep is more likely to migrate upward through normal big faults, gas chimneys or unconformities surface around PFs. In most cases, PFs play limited roles in fluid migration, such as in South China Sea where hydrocarbon accumulations have been observed under the PFs and the PF-bearing layer may act as a seal trapping the free gas underneath. Generally, the role of PFs in fluid migration should be determined through a comprehensive analysis of the characteristics of PFs, surrounding amplitude anomalies, hydrocarbon distribution, and even the sealing ability of PFs which needs further study. It is more likely that the PFs play a limited role in vertical fluid migration and related shallower hydrocarbon accumulation and distribution.
Polygonal faults (PFs) are the network of layer-bound minor normal faults, which are generally characterized by polygonal planform geometry and variable strike directions (Lonergan et al., 1998a; Berndt et al., 2012; Laurent et al., 2012; Ding et al., 2013; Li et al., 2020). PFs were first found in the Lower Tertiary sediments in Belgium, but the polygonal planform pattern was not determined at the beginning due to the absence of 3D seismic data (Henriet et al., 1991). Cartwright (1994 ab) adopted the time slice technique to first characterize the planform pattern of the PFs in the North Sea Basin. Since then, PFs have gained attention as one of the most debated and mystical geological structures from researchers, and have been recognized in over 100 sedimentary basins worldwide till now (e.g., Seebeck et al., 2015; Ireland et al., 2021; Figure 1). PFs were proposed to be extensional non-tectonic in genesis occurring at comparatively shallow depth (Cartwright, 1994 ab, 2011). They are commonly featured by small fault throw (usually10-50 m), slight fault displacement (mostly< 100 m), steep in dip angles (typically 40–70°), short horizontal extension in a single fault (commonly < 1500 m), and multi-direction strikes without preferential direction (Cartwright, 1994a, 2011; Cartwright and Dewhurst, 1998; He et al., 2010; Berndt et al., 2012; Ding et al., 2013; Figure 2). In addition to PFs, non-tectonic fractures can also occur in shale, formed during shale diagenesis and hydrocarbon generation and expulsion (Meng et al., 2021). This kind of fractures can occur at deeper depth, caused by the fluid pressure buildup, local stress distortion, and abrupt changes in matrix porosity and rock mechanical properties due to the intrinsic properties of shales. But they may be invisible in seismic due to their small scale and the resolution limitation of seismic data.
FIGURE 1. Global distribution of the polygonal faults discovered all over the world (modified after Cartwright, 2011). In this study PF occurrences discovered after 2010 (yellow dots) are added which is a supplement of previous compilation (red dots; Cartwright, 2011).
FIGURE 2. (A) A seismic profile in east-west direction in the Pearl River Mouth Basin, northern South China Sea showing the distribution of PFs overlying the high amplitude reflections which are interpreted to be free gas; (B) A coherence map of a seismic reflection crosscutting the PFs in the Pearl River Mouth Basin, showing the plane form of polygonal faults and normal faults; (C) Enlarged sketch of the black rectangle in Figure 2B, showing the map-view random polygonal fault patterns.
Previous studies often regarded PFs as dominant pathways for fluid migration, the presence of which was often interpreted to have facilitated vertical migration of oil and gas, therefore playing a vertical role in the shallower hydrocarbon accumulations (Larter et al., 2000; Gay et al., 2006; Hustoft et al., 2007; Ding et al., 2013; Alrefaee et al., 2018; Velayatham et al., 2021). For example, Alrefaee et al. (2018) mentioned that the PFs in the Northern Carnarvon Basin acted as the conduits for the hydrocarbon migration, and Ding et al. (2013) stated that the PFs in the Sanzhao sag of the Songliao Basin in China played a significant role in oil migration. However, no direct detailed evidence has been provided in these publications to prove the roles of PFs in facilitating vertical fluid migration. On the contrary, high amplitude seismic reflections indicating free gas distribution was observed underneath the PF-bearing layer in the continental margin of the South China Sea, suggesting that the PF-bearing layer may act as a seal and the PFs may not be favorable fluid migration conduits at present (Sun et al., 2010). After careful examination of lots of PF case studies, it has been recognized that the evidence for the role of PFs in fluid migration is not solid enough in most cases, and more comprehensive studies should be carried out to evaluate the role of PFs in fluid migration. In this paper we focus on the role of PFs in fluid migration by conducting an examination of enormous global cases of PFs, and key parameters are analyzed such as the genesis mechanism and characteristics of the PFs (e.g., buried depth, lithology, size, etc.), surrounding amplitude anomalies, hydrocarbon distribution and even the permeability of the faults and nearby sediment layers. Based on above analysis the general role of PFs in fluid migration is evaluated, as well as the contribution to the shallower hydrocarbon or gas hydrate accumulation.
A compilation of the genesis mechanisms of PFs is summarized in this paper based on publications of global PF case studies, with five main mechanisms recognized including density inversion, gravity sliding theory, low coefficients of friction, syneresis, and shear failure (Table 1). ‘Gravity sliding theory’ was first proposed by Higgs and McClay (1993), according to which the gravitational stresses produce a strong alignment of fault strikes during a downslope sliding of deposits (Cartwright et al., 1994 ab; Goulty, 2008). However, many PF-bearing layers are smooth with an extremely small dip of almost zero, suggesting that this theory may not explain the formation mechanisms of many PF cases (Cartwright et al., 2003; Goulty, 2008).
The ‘low coefficients of residual friction’ mechanism was proposed by Goulty (2002), and it was proposed that the nucleated PFs can continue to grow by low coefficients of friction, creating compaction and repeated slip of sediments with increasing overburden stress (Goulty, 2008; Han et al., 2016). The mechanism of low friction coefficients along faults can only explain the formation of PFs after initial slip, but cannot account for fault nucleation (Goulty, 2002; Goulty and Swarbrick, 2005). In addition, this theory is not applicable for PFs developed in porous sandstones because shear band in sandstones is usually developed through a strain-hardening process that eventually weakens the strain (Antonellini et al., 1994; Aydin et al., 2006; Antonellini and Mollema, 2015).
‘Density inversion’ was interpreted to be caused by the differential compaction between the underlying over-pressured low-density strata and the overlying normally pressured layer (Henriet et al., 1991; Cartwright, 1994 ab). It was often accompanied by shale flowage and hydrofracturing, during which processes the PFs were formed. For example, the PFs in the Lake Hope region of South Australia was interpreted to be triggered by gravitational instability of a low-density over-pressured layer at the bottom of the PF-bearing sequence (Watterson et al., 2000). This has been proved by experimental results as the pattern of ridges defining the polygon boundaries is consistent to the experimental spoke and hub patterns formed at the boundaries between viscous materials with density inversion. The ‘density inversion’ theory has also been proved by Nicol et al. (2003), who proposed a density inversion model showing similar fault geometry, linkage and capture. However, this theory only suits the PFs developed in over-pressured low-density layers and cannot explain the PFs in normally pressured strata (Goulty, 2002; Ding et al., 2013).
‘Syneresis mechanism’ indicates the PF formation caused by dewatering contraction, probably due to spontaneous contraction of colloidal sediments which often occurs during early compaction (Dewhurst et al., 1999a; Dewhurst et al., 1999b; Berndt et al., 2012). This theory can better explain the map-view polygonal geometry as the small normal faults intersect with each other (Ding et al., 2013). The PFs found in the Tertiary strata of the North Sea is interpreted to be formed under this genesis mechanism (Dewhurst et al., 1999a). In this area, the lithology of the PF-bearing successions is dominantly composed of smectitic claystone and carbonate chalks, and the three-dimensional contraction of smectite-rich gels favors the PF formation, which is driven by the interparticle attractive forces between clay particles. At present the syneresis mechanism is widely accepted especially for PF occurrence areas rich in colloidal sediments.
‘Shear failure’, also known as ‘volumetric contraction’, is regarded to be caused by a decrease in horizontal stress due to mineral-specific dissolution associated mostly with opal A-opal CT-quartz transitions during sediment diagenesis (Davies and Ireland, 2011; Laurent et al., 2012; Li et al., 2020). This mechanism has been widely adopted to explain the formation of PFs (Cartwright, 2011; Ding et al., 2013), such as in the Sanzhao Sag in East China, Vøring Basin Offshore Norway and Great South Basin in New Zealand (Han et al., 2016; Li et al., 2020). However, the shear failure mechanism is not applicable for the PF formation in areas without biogenic silica and smectite, such as in the chalk layer of Cretaceous Khoman Formation near Farafra Oasis in the Western Desert of Egypt (Tewksbury et al., 2014).
Generally, no consensus on the genesis mechanism of PFs has been reached until present and it is possible that there are several different genesis mechanisms for the global PF occurrences which should be determined by the local lithological, diagenetic or stress conditions. “Density inversion”, ‘syneresis’ and ‘shear failure’ are the main formation mechanisms which are widely accepted as responsible for the formation of PFs in different areas. To determine the specific formation mechanism of PFs in certain areas, a comprehensive analysis should be carried out investigating key parameters such as lithology, pore fluid and strain conditions.
PFs are layer-bound deformation structures mainly formed in shallow soft sediments of marine settings such as passive continental margins (Tewksbury et al., 2014; Seebeck et al., 2015; Turrini et al., 2017; Hoffmann et al., 2019; Velayatham et al., 2021), some intracratonic sedimentary basins and foreland basins (Cartwright, 2011; Morgan et al., 2015; Li et al., 2020; Figure 1). Some PFs have also been observed in lacustrine environment (Cartwright et al., 2004; He et al., 2010; Han et al., 2016). Most PFs were found in strata of fine-grained sediments (e.g., mudstone, shale, claystone, or carbonate chalk), younger than Middle Cretaceous period (Table 2; Hansen et al., 2004; Andresen and Huuse, 2011; Tewksbury et al., 2014; Yang et al., 2017; Alrefaee et al., 2018). However, Sun et al. (2010) and Hansen et al. (2004) stated that PFs may also occur in comparatively older pre-Cretaceous sediments, but they cannot be imaged clearly in seismic data probably due to the limitation of seismic resolution. A case study in Arches National Park in south-eastern Utah displayed the outcrops of PFs in the upper sandstone layer of the Middle Jurassic (Table 2), and the preservation of PFs in a comparatively older strata was probably due to their shallow burial depth in an uplifted erosional environment (Antonellini and Mollema, 2015).
TABLE 2. A compilation of global case studies of PFs showing information on location, buried depth, geological time, lithology, thickness, size, related hydrocarbon reservoirs, and the role of PFs in fluid migration.
Hansen et al. (2004) used 3D seismic attribute maps to show the intersecting pattern between PFs and tectonic faults, suggesting that the propagation of PFs has been disturbed by the tectonic faults. Ostanin et al. (2012) also emphasized the tectonic impact on the growth of PFs, and the dominant strike direction of the PFs is consistent with the strike direction of the regional tectonic faults. Therefore, even though the PFs are non-tectonic, the tectonic influence on the growth and propagation of PFs cannot be ruled out. The development and distribution of PFs are primarily determined by the lithology of the PF-bearing layer and their buried depth, and the regional stress affects the propagation and dominant direction of both tectonic faults and PFs.
Based on publications of global PF case studies, a compilation of PFs was carried out with key parameters analysed including PF locations, geological time, buried depth, lithology, thickness, size, related hydrocarbon reservoirs, and their interpreted roles in fluid migration (Table 2).
PFs are often characterized by small fault throw (Figure 3A), small lateral extension and various displacement value (from a few meters to over 100 m), mainly developed in shallow-buried strata which are over 100 m thick and deposited after the Middle Cretaceous period (mainly Tertiary, Figure 3B, Figure 4B; Cartwright, 2007; He et al., 2010; Berndt et al., 2012; Ostanin et al., 2012; Li et al., 2020). They have neither dominant strike nor dominant tendency, existing as networks of minor normal faults (Watterson et al., 2000; Alrefaee et al., 2018; Figure 2). The throws of PFs developed in most of the PF areas are smaller than 50 m, such as in the Lower Congo Basin (10–20 m, Morgan et al., 2015), in the Hammerfest Basin (5–20 m, Morgan et al., 2015), in the Qiongdongnan Basin (10–40 m, Han et al., 2016), and in the Near Farafra Oasis, Egypt (2–3 m, Tewksbury et al., 2014). In addition, some PFs with larger throw of >100 m have also been found such as in the Faroe-Shetland Basin (Bureau et al., 2013). The extending length of PFs in most of the PF areas is generally smaller than 2 km, such as in the Northern Carnarvon Basin (70–620 m, Alrefaee et al., 2018), in the North Sea Basin (1,000–2000 m, Lonergan et al., 1998a), and in the Pearl River Mouth Basin (100–1400 m, Sun et al., 2012).
FIGURE 3. (A) Frequency of the throw of polygonal faults of the global PFs case; (B) Frequency of the thickness of sole layer with polygonal faults of the global PFs case.
FIGURE 4. (A) The buried depth below seabed of the PFs cases worldwide; (B) The development period of the global PFs case.
With the increase of burial depth, the dip angle of PFs decreases gradually, interpreted to be caused by compaction and flattening of the fault planes due to increasing vertical stress (Lonergan et al., 1998b; Stuevold et al., 2003; Shoulders et al., 2007). For example, some PFs from offshore Norway appear to have relatively steep dip angle of ∼80° at the shallower level, but they show strongly listric at deeper depth with comparatively smaller dip angle of ∼60° (Cartwright, 2011). PFs are layer-bound deformation structures mainly formed in shallow soft sediments of marine settings. Most of the PFs discovered worldwide are located at depth smaller than 1,500 m below the seabed (mbsb) with only a small proportion of PFs occur at depth ranging from 1,500 to 2000 mbsb, at which depth the early stages of compaction probably caused the initiation of PFs (Figure 4A). At even deeper depth, the PF-bearing layer experienced strong diagenesis which makes the PFs difficult to be imaged in seismic. What is more, small sized PFs have been found in a few meters below the present-day lakebed in the Western Lake Superior, with a thickness range of 10–15 m and fault throw of < 1 m (Cartwright et al., 2004). Two outcrops of PFs have been observed in the Jurassic layers in the Arches National Park in south-eastern Utah and the Cretaceous layers in the Near Farafra Oasis, Egypt (Tewksbury et al., 2014 Antonellini and Mollema, 2015). The layer-bound PFs are mainly developed in marine claystone, and therefore the thickness of the PF-bearing strata is mainly controlled by the sediment distribution deposited in certain sedimentary environment (Cartwright and Lonergan, 1997; Goulty, 2008). Most PFs around the world were developed in layers of < 700 m thick, with quite a few occurring in layers of only several meters thick (Table 2; Figure 3B). For example, the outcrops of PFs was observed in Arches National Park in south-eastern Utah, the layer thickness of which is < 2 m (Antonellini and Mollema, 2015).
PFs are usually found in layer-bound successions consisting mainly of fine-grained sediments (e.g., mudstone, shale, claystone, or carbonate chalk), which may be related with the genesis mechanism of PFs (Andresen and Huuse, 2011; Cartwright, 2011; Yang et al., 2017; Alrefaee et al., 2018; Ireland et al., 2021). “Syneresis” due to spontaneous contraction of colloidal sediments and “shear failure” caused by mineral-specific dissolution have been widely accepted to explain the PF formation mechanism in fine-grained sediments (Han et al., 2016; Li et al., 2020). Besides, small outcrops of PFs have been found in the Entrada sandstone strata of Middle Jurassic in the Arches National Park in south-eastern Utah (Antonellini and Mollema, 2015). Since sandstone are relatively coarser and permeable, genesis mechanisms such as “syneresis” and “shear failure” cannot explain the PF formation in sandstone layers. The “density inversion” was proposed to account for the PF formation in the Entrada Sandstone (Antonellini and Mollema, 2015). The over-pressured sequences (Carmel Formation) is overlain by normally pressured layer (Entrada Sandstone), which caused accompanied shale flowage and hydrofracturing therefore leading to the formation of PFs in sandstones. In addition, the geometry, distribution and intensity of PFs are mainly determined by lithological features such as grain size, hardness, diagenesis, clay mineralogy and organic matter content (Dewhurst et al., 1999 ab; Hansen et al., 2004; Turrini et al., 2017). When the PF-bearing layer is homogeneous in thickness and lithology, faults cross cut each other uniformly at an angle of 60° (Cartwright, 2011; Li et al., 2020). Otherwise, the geometry of the PFs is featured by irregular polygons when the PF-bearing layer is heterogeneous in thickness and lithology, which represents the situation of most global PF cases.
Although most PFs occur in fine-grained sediments, their formation and characteristics are also affected by nearby coarse sand bodies density (Victor and Moretti, 2006; Shoulders et al., 2007; Liu et al., 2008; He et al., 2010). In the south-east area of the Sanzhao sag, the PFs are well developed in areas of smaller sand/layer thickness ratio and poor physical property. On the contrary, the PFs are sparsely developed in the northern area of Sanzhao sag where is featured by larger sand/layer thickness ratio and good physical property (He et al., 2010). The sand bodies overlying the PFs favour the discharge of fluids from the PF-bearing mudstone layer, inhibiting the formation of overpressure and therefore causing sparsely developed PFs.
According to previous studies, the presence of PFs has been proposed to play a role in both favouring fluid migration and as a fluid source during the early stage of PF generation (Hustoft et al., 2007; Sun et al., 2010; Tewksbury et al., 2014). Many researchers commonly believed that PFs facilitate fluid migration by generating more failures and destroying the integrity of fine-grained layers, and regarded PFs as dominant channels for fluid migration and re-migration (Ding et al., 2013; Seebeck et al., 2015; Han et al., 2016). For example, Laurent et al. (2012) has proposed that the PFs in Gjallar Ridge offshore Norway can act as preferential fluid migration pathways when they are connected closely. Alrefaee et al. (2018) interpreted the PFs in Northern Carnarvon Basin in Australia as the fluid migration pathways based on seismic imaging of the discontinuity of the fine-grained sediments. In the Sanzhao Sag of the Songliao Basin, the oil source comparison of hydrocarbon reservoirs above and below PFs shows that they were from the same source rock, and this was regarded as the evidence that PFs acted as the fluid migration pathways (He et al., 2010; Ding et al., 2013). According to Sun et al. (2010), the development of PFs in the fine-grained Huangliu Formation and Meishan Formation in Qiongdongnan Basin destroyed the sealing property and increased the permeability, resulting in the upward secondary migration of oil and gas from the lower hydrocarbon reservoir.
Considering the dewatering process during the initial period of PF formation, the PF-bearing layer may be a fluid source where fluids were expelled to nearby sediments. For example, the sediment dewatering during PF generation in the Kai Formation and the underlying Brygge Formation in Mid-Norwegian Margin were regarded as responsible for providing the fluid source for the gas hydrate formation in the shallower Vøring margin (Berndt et al., 2003; Hustoft et al., 2007). PF generation is commonly associated with underlying troughs and overlying incised valleys, and it was proposed that the fluid produced by dewatering in the early stage of PFs formation may escape and migrate upward causing the troughs due to related consequent volume loss and subsequent collapse (Sun et al., 2010). The overlying incised valleys were caused by fluid escape which was expelled from underlying PF-bearing mudstone layer (Figure 5A).
FIGURE 5. (A) A representative seismic profile shows troughs below polygonal faults and fluidized sediments within incised valleys above polygonal faults in southeast direction in the Qiongdongnan Basin, South China Sea (modified from Sun et al. (2010)); (B) A seismic profile through a polygonal fault system and buried pockmark field in the Canterbury Basin, New Zealand (modified from Hoffmann et al. (2019)).
Sediment compaction and pore water drainage occur during PF formation, resulting in the formation of pockmark in Canterbury Basin (Berndt et al., 2003; Gay et al., 2004; Gay and Berndt, 2007; Goulty, 2008). But that is not the only factor for the pockmark formation because 6 out of 11 pockmarks are not spatially consistent with the PF locations (Figure 5B). Other possibilities cannot be excluded such as bottom currents and differential compaction. In addition, PFs are often laterally impermeable, which may prevent lateral fluid migration and may form fault-lithologic traps when combined with nearby sand bodies (He et al., 2010; Andresen and Huuse, 2011).
Previous studies often regarded PFs as fluid migration pathways for shallower hydrocarbon or gas hydrate accumulations (Ding et al., 2013; Alrefaee et al., 2018; Velayatham et al., 2021). However, other fluid conduits cannot be ruled out such as the major faults, gas chimneys unconformities surface, or lateral migration along permeable layers to the structural highs. According to Ostanin et al. (2012), the widely distributed PFs found in Hammerfest Basin within Campanian layers may act as pathways for vertical hydrocarbon migration. However, the localized high amplitude anomalies are more likely to be distributed along the Palaeocene-Early Eocene Faults and the major deep 1st order faults (Figure 6A). We propose that the hydrocarbon fluid from the deeper reservoirs may initially migrate along the 1st order faults and then continue to migrate along the PEEFs to form the high amplitude anomalies. These enhanced amplitude anomalies may be due to the presence of hydrocarbons or diagenesis effect, both of which are related to the fluid migration (O'Brien et al., 2005). Velayatham et al. (2021) proposed that the PFs in the Ceduna Sub-basin, offshore South Australia may act as fluid migration pathways due to the spatial association of the PFs with shallower pipes (Figure 6B). However, after careful examination of the seismic profiles and plan view maps in the Ceduna Sub-basin, it seems insufficient to determine the fluids that escaped along the pipes were from underneath PFs which provided the fluids (Velayatham et al., 2021). Other possibilities of fluid supply should not be excluded such the migration along the major deep faults or lateral permeable sedimentary layers. Based on comprehensive analysis of enormous global PF case studies, we propose that in most PF cases the PFs play very limited roles in facilitating vertical long-distance fluid migration.
FIGURE 6. (A) A seismic profile in northeast direction in the Hammerfest Basin (modified from Ostanin et al. (2012)), showing Amplitude anomalies, Acoustic blanking, Incoherent/chaotic reflectors, Polygonal faults, PEEFs (Paleocene-Early Eocene Faults) and 1st order faults. (B) A seismic profile in southwest direction in the Ceduna Sub-basin, offshore South Australia (modified from Velayatham et al. (2021)).
Many PFs occur in the fine-grained sediments layer of the Pearl River Mouth Basin in the northern South China Sea, but they may have played limited role in fluid migration (Sun et al., 2012; Yang et al., 2017). According to Sun et al. (2012) and Yang et al. (2017), the fluid migration system is mainly composed of gas chimneys and major large faults, and the enhanced seismic reflections representing shallow gas accumulation are mainly located on top of the gas chimneys (Figure 7). Meanwhile, the PF-bearing layer lacks local amplitude anomalies, and high amplitude gas reservoirs even exist underneath the PFs, both indicating that the PF-bearing layer should be impermeable at present and may hinder upward migration of the underlying fluids.
FIGURE 7. Seismic profiles in the Pearl River Mouth Basin, northern South China Sea showing the distribution of gas chimney, enhanced seismic reflections, large normal fault, and PFs. (A) modified from Sun et al. (2012). (B) modified from Yang et al. (2017).
In the Lower Congo Basin, Gay et al. (2006) stated that the PFs allow deeper fluid migration to reach the seabed because of the upward deflection of the base of the gas hydrate stability zone (indicated by bottom-simulating reflector BSR in seismic), which was interpreted to be caused by a localized positive heat flow anomaly related to the PFs (Figure 8A). However, it has been noticed in this study that the deflection of the BSR solely appears around the chimney zone rather than throughout the whole PF-bearing layer. In the same area, Andresen and Huuse (2011) described the high reflectivity zone between the two PF-bearing layers which represents free gas accumulation. On the contrary, the vertical configuration of the free gas zone and the PF-bearing layer suggests the limited role of PFs as fluid migration conduits. It is more possible that the PF-bearing layer act as a seal trapping the free gas underneath. Additionally, localized high amplitude anomalies only exist along the gas chimney, indicating the positive role of gas chimney in fluid migration (Andresen and Huuse, 2011; Figure 8B,C). In this study, we interpret the high reflectivity zone between the two PF-bearing layers as sandstone-rich porous reservoir bodies which deposited as turbidite sandstone body in a background of fine-grained marine sediments. In this condition, good configuration of reservoir-seal led to the accumulation of free gas zone, with gas chimney being the major conduits transporting free gas from the deep. Considering the role of sandstone bodies as a barrier to PF propagation, the distribution and geometric attributes of PFs can be used to delineate sandstone bodies in the PF-bearing layer, which can be applied in date-poor areas (Jackson et al., 2014; Turrini et al., 2017).
FIGURE 8. (A) A seismic profile and its interpretation in north-south direction across a single pockmark in the Lower Congo Basin (modified from Gay et al. (2006)). The highly-faulted interval is composed of numerous closely spaced polygonal faults as shown in Figure 8B,C. A seismic profile and its interpretation in southeast direction in the Lower Congo Basin (modified from Andresen and Huuse (2011)).
The activity of the PFs is intermittent which can last over several millions of years, and therefore the role of PFs in fluids migration may be spatially and temporally variable (Berndt et al., 2003; Gay and Berndt, 2007; Berndt et al., 2012). The integrity of a fine-grained seal can be destroyed by the initial dewatering of host sediments, slip activity of the PFs, or the reactivation of PFs due to the contraction during tectonic rifting period, which results in the transient fluid leakage (Gay et al., 2006; Gay and Berndt, 2007; Hustoft et al., 2007; Seebeck et al., 2015). However, the PFs should be inactive and therefore probably impermeable in a larger geological time-scale. Additionally, the PF-bearing layers are often composed of primarily very fine-grained smectitic claystones or carbonate chalks, with generally low permeability (<10−17 m2) (Hibsch et al., 2003; Hansen et al., 2004; Tewksbury et al., 2014; Antonellini and Mollema, 2015).
It is widely acknowledged that the existence of PFs potentially increases the overall vertical permeability of fine-grained strata, slightly higher than the permeability of the host lithology (Evans et al., 1997; Gay et al., 2004; Faulkner et al., 2010; Ilg et al., 2012). However, this may not be large enough to allow fluid to flow smoothly through the PF-bearing layer. For example, the PFs in offshore Australia exist in the cap rock above the potential CO2 reservoirs (Seebeck et al., 2015), suggesting the role of the PF-bearing layer as a seal. In contrast, the presence of PFs in coarse-grained sediments (e.g. porous sandstones) may reduce the permeability of PF-bearing layer, probably due to the deformation bands with cataclasis (Antonellini and Mollema, 2015). For example, the permeability of deformation band with cataclasis of the PFs is ∼10−15 m2 at Arches National Park, while the permeability of deformation bands without cataclasis can reach 10−14 to 10−13 m2 in the same area (Antonellini et al., 1994).
To summarize, the PFs developed in the sealing strata of fine-grained sediments may have improved the permeability for certain degree, but were still not favourable for fluid migration. Alternatively, the presence of PFs may even improve the sealing ability over time when clay smeared along the fault surfaces (Lonergan et al., 1998a; Turrini et al., 2017). In addition, Cartwright et al. (2007) proposed that the rate of fluid migration through PFs is relatively low on geological time scales, and therefore the presence of PFs alone cannot cause extended leakage from deeper hydrocarbon reservoirs. Generally, the role of PFs in fluid migration may be limited due to the PF-bearing layers act more as seals in most of the global PF cases.
A geophysical review of global PFs case studies was conducted in this paper to better understand the role of PFs in fluid migration and their contrition to the shallower hydrocarbon or gas hydrate accumulation. Five main conclusions are made based on a comprehensive analysis of the geophysical characteristics of the PFs Chen et al., 2011, Davies et al., 2009, Gay et al., 2007, Shin et al., 2008, Shin et al., 2010.
1. Three genesis mechanisms may account for the PF formation in most cases, including density inversion, syneresis and shear failure. A comprehensive analysis of lithology, pore fluid and strain conditions are needed to determine the specific PF formation mechanism for a specific study area.
2. PFs are non-tectonic and layer-bound, mainly developed in shallow-buried fine-grained strata of >100 m thickness and deposited after the Middle Cretaceous period. PFs are characterized by steep dip angles (40–70°), multi-direction strikes, small fault throws (most <50 m), small lateral extensions (most < 2 km) and various displacement values (10–100 m).
3. Examination of many case studies shows that the PFs may play a limited role in fluid migration based on the spatial relationship of the PFs, nearby major fluid migration pathways and high amplitude anomalies. On one hand, the PFs developed in the fine-grained strata may improve the permeability for certain degree, but may be not favourable for fluid migration. On the other hand, the presence of PFs may even improve the sealing ability when the clay smeared along the fault surfaces.
4. The sealing ability of PF-bearing layer can be destroyed by the initial dewatering of host sediments, slip activity of the PFs, or the reactivation of PFs, causing a transient period of fluid leakage. But in a larger geological time-scale, the PFs should be inactive and therefore probably impermeable.
5. Considering the limited role of PFs in fluid migration, it is proposed that the PFs may not contribute a lot to the shallower hydrocarbon or gas hydrate accumulation, and other major conduits are needed such as deep faults, gas chimneys, diapirs.
JY: Writing, Editing, Conceptualization, Methodology. YX: Software, Writing- Reviewing and Editing. ZY: Conceptualization, Supervision. SL and MW: Project administration, Investigation. SD: Data curation, Writing- Original draft preparation. YC and ML: Data curation, Writing- Reviewing and Editing.
This work was supported by Shandong Provincial Natural Science Foundation (ZR2019QD013), Foundation and key technology of marine gas hydrate trial production project (ZD2019-184-001), Fundamental Research Funds for the Central Universities (19CX02003A), and NSF of China (41406050).
Shandong Provincial Natural Science Foundation (ZR2019QD013), Foundation and key technology of marine gas hydrate trial production project (ZD2019-184-001), “14th Five-Year plan” forward-looking basic major science and technology project of CNPC (2021DJ4901), Fundamental Research Funds for the Central Universities (19CX02003A), and NSF of China (41406050) financially support this study.
ZY was employed by Engineering Technology Research Company Limited and China National Petroleum Corporation.
The remaining authors declare that the research was conducted in the absence of any commercial or financial relationships that could be construed as a potential conflict of interest.
All claims expressed in this article are solely those of the authors and do not necessarily represent those of their affiliated organizations, or those of the publisher, the editors, and the reviewers. Any product that may be evaluated in this article, or claim that may be made by its manufacturer, is not guaranteed or endorsed by the publisher.
Alrefaee, H. A., Ghosh, S., and Abdel-Fattah, M. I. (2018). 3D Seismic Characterization of the Polygonal Fault Systems and its Impact on Fluid Flow Migration: An Example from the Northern Carnarvon Basin, Australia. J. Pet. Sci. Eng. 167, 120–130. doi:10.1016/j.petrol.2018.04.009
Andresen, K. J., and Huuse, M. (2011). 'Bulls-eye' Pockmarks and Polygonal Faulting in the Lower Congo Basin: Relative Timing and Implications for Fluid Expulsion during Shallow Burial. Mar. Geology. 279, 111–127. doi:10.1016/j.margeo.2010.10.016
Antonellini, M. A., Aydin, A., and Pollard, D. D. (1994). Microstructure of Deformation Bands in Porous Sandstones at Arches National Park, Utah. J. Struct. Geology. 16, 941–959. doi:10.1016/0191-8141(94)90077-9
Antonellini, M., and Mollema, P. N. (2015). Polygonal Deformation Bands. J. Struct. Geology. 81, 45–58. doi:10.1016/j.jsg.2015.10.003
Aydin, A., Borja, R. I., and Eichhubl, P. (2006). Geological and Mathematical Framework for Failure Modes in Granular Rock. J. Struct. Geology. 28, 83–98. doi:10.1016/j.jsg.2005.07.008
Berndt, C., Bünz, S., and Mienert, J. (2003). Polygonal Fault Systems on the Mid-Norwegian Margin: a Long-Term Source for Fluid Flow. Geol. Soc. Lond. Spec. Publications 216, 283–290. doi:10.1144/gsl.sp.2003.216.01.18
Berndt, C., Jacobs, C., Evans, A., Gay, A., Elliott, G., Long, D., et al. (2012). Kilometre-scale Polygonal Seabed Depressions in the Hatton Basin, NE Atlantic Ocean: Constraints on the Origin of Polygonal Faulting. Mar. Geology. 332-334, 126–133. doi:10.1016/j.margeo.2012.09.013
Bureau, D., Mourgues, R., Cartwright, J., Foschi, M., and Abdelmalak, M. M. (2013). Characterisation of Interactions between a Pre-existing Polygonal Fault System and sandstone Intrusions and the Determination of Paleo-Stresses in the Faroe-Shetland basin. J. Struct. Geology. 46, 186–199. doi:10.1016/j.jsg.2012.09.003
Cartwright, J. A., and Dewhurst, D. N. (1998). Layer-bound Compaction Faults in fine-grained Sediments. Geol. Soc. Am. Bull. 110, 1242–1257. doi:10.1130/0016-7606(1998)110<1242:lbcfif>2.3.co;2
Cartwright, J. A. (1994a). Episodic basin-wide Fluid Expulsion from Geopressured Shale Sequences in the North Sea basin. Geol 22, 447–450. doi:10.1130/0091-7613(1994)022<0447:ebwfef>2.3.co;2
Cartwright, J. A. (1994b). Episodic basin-wide Hydrofracturing of Overpressured Early Cenozoic Mudrock Sequences in the North Sea Basin. Mar. Pet. Geology. 11, 587–607. doi:10.1016/0264-8172(94)90070-1
Cartwright, J. A., and Lonergan, L. (1996). Volumetric Contraction during the Compaction of Mudrocks: a Mechanism for the Development of Regional-Scale Polygonal Fault Systems. Basin. Res. 8, 183–193. doi:10.1046/j.1365-2117.1996.01536.x
Cartwright, J. A. (1996). Polygonal Fault Systems: a New Type of Fault Structure Revealed by 3-D Seismic Data from the North Sea Basin. AAPG. Stud. Geol. 42, 225–230.
Cartwright, J. (2011). Diagenetically Induced Shear Failure of fine-grained Sediments and the Development of Polygonal Fault Systems. Mar. Pet. Geology. 28, 1593–1610. doi:10.1016/j.marpetgeo.2011.06.004
Cartwright, J., Huuse, M., and Aplin, A. (2007). Seal Bypass Systems. Bulletin 91, 1141–1166. doi:10.1306/04090705181
Cartwright, J., James, D., and Bolton, A. (2003). The Genesis of Polygonal Fault Systems: a Review. Geol. Soc. Lond. Spec. Publications 216, 223–243. doi:10.1144/gsl.sp.2003.216.01.15
Cartwright, J., and Lonergan, L. (1997). Seismic Expression of Layer-Bound Fault Systems of the Eromanga and North Sea Basins. Exploration Geophys. 28, 323–331. doi:10.1071/eg997323
Cartwright, J. (2007). The Impact of 3D Seismic Data on the Understanding of Compaction, Fluid Flow and Diagenesis in Sedimentary Basins. J. Geol. Soc. 164, 881–893. doi:10.1144/0016-76492006-143
Cartwright, J., Wattrus, N., Rausch, D., and Bolton, A. (2004). Recognition of an Early Holocene Polygonal Fault System in Lake Superior: Implications for the Compaction of fine-grained Sediments. Geol 32, 253–256. doi:10.1130/g20121.1
Chen, D., Wu, S., Wang, X., and Lv, F. (2011). Seismic Expression of Polygonal Faults and its Impact on Fluid Flow Migration for Gas Hydrates Formation in Deep Water of the South China Sea. J. Geol. Res. 2011, 1–7. doi:10.1155/2011/384785
Clausen, J. A., Gabrielsen, R. H., Reksnes, P. A., and Nysæther, E. (1999). Development of Intraformational (Oligocene-Miocene) Faults in the Northern North Sea: Influence of Remote Stresses and Doming of Fennoscandia. J. Struct. Geology. 21, 1457–1475. doi:10.1016/s0191-8141(99)00083-8
Davies, R. J., Ireland, M. T., and Cartwright, J. A. (2009). Differential Compaction Due to the Irregular Topology of a Diagenetic Reaction Boundary: a New Mechanism for the Formation of Polygonal Faults. Basin. Res. 21, 354–359. doi:10.1111/j.1365-2117.2008.00389.x
Davies, R. J., and Ireland, M. T. (2011). Initiation and Propagation of Polygonal Fault Arrays by Thermally Triggered Volume Reduction Reactions in Siliceous Sediment. Mar. Geology. 289, 150–158. doi:10.1016/j.margeo.2011.05.005
Dewhurst, D. N., Cartwright, J. A., and Lonergan, L. (1999a). The Development of Polygonal Fault Systems by Syneresis of Colloidal Sediments. Mar. Pet. Geology. 16, 793–810. doi:10.1016/s0264-8172(99)00035-5
Dewhurst, D. N., Cartwright, J. A., and Lonergan, L. (1999b). Three-dimensional Consolidation of fine-grained Sediments. Can. Geotech. J. 36, 355–362. doi:10.1139/t98-101
Ding, X. J., Liu, G. D., Sun, M. L., and Wang, P. G. (2013). Origin of Polygonal Fault Systems: A Case from the Sanzhao Sag in the Songliao Basin, East China. Pet. Explor. Dev. 40, 309–319. doi:10.1016/s1876-3804(13)60040-3
Evans, J. P., Forster, C. B., and Goddard, J. V. (1997). Permeability of Fault-Related Rocks, and Implications for Hydraulic Structure of Fault Zones. J. Struct. Geology. 19, 1393–1404. doi:10.1016/s0191-8141(97)00057-6
Faulkner, D. R., Jackson, C. A. L., Lunn, R. J., Schlische, R. W., Shipton, Z. K., Wibberley, C. A. J., et al. (2010). A Review of Recent Developments Concerning the Structure, Mechanics and Fluid Flow Properties of Fault Zones. J. Struct. Geology. 32, 1557–1575. doi:10.1016/j.jsg.2010.06.009
Gay, A., and Berndt, C. (2007). Cessation/reactivation of Polygonal Faulting and Effects on Fluid Flow in the Vøring Basin, Norwegian Margin. J. Geol. Soc. 164, 129–141. doi:10.1144/0016-76492005-178
Gay, A., Lopez, M., Cochonat, P., Séranne, M., Levaché, D., and Sermondadaz, G. (2006). Isolated Seafloor Pockmarks Linked to BSRs, Fluid Chimneys, Polygonal Faults and Stacked Oligocene-Miocene Turbiditic Palaeochannels in the Lower Congo Basin. Mar. Geology. 226, 25–40. doi:10.1016/j.margeo.2005.09.018
Gay, A., Lopez, M., Cochonat, P., and Sermondadaz, G. (2004). Polygonal Faults-Furrows System Related to Early Stages of Compaction - Upper Miocene to Recent Sediments of the Lower Congo Basin. Basin Res. 16, 101–116. doi:10.1111/j.1365-2117.2003.00224.x
Gay, A., Lopez, M., Cochonat, P., Sermondadaz, G., and Seranne, M. (2007). Evidence of Early to Late Fluid Migration from an Upper Miocene Turbiditic Channel Revealed by 3D Seismic Coupled to Geochemical Sampling within Seafloor Pockmarks, Lower Congo Basin. Mar. Pet. Geol. 23, 387–399. doi:10.1016/j.marpetgeo.2006.02.004
Goulty, N. R. (2008). Geomechanics of Polygonal Fault Systems: a Review. Pet. Geosci. 14, 389–397. doi:10.1144/1354-079308-781
Goulty, N. R. (2002). Mechanics of Layer-Bound Polygonal Faulting in fine-grained Sediments. J. Geol. Soc. 159, 239–246. doi:10.1144/0016-764901-111
Goulty, N. R., and Swarbrick, R. E. (2005). Development of Polygonal Fault Systems: a Test of Hypotheses. J. Geol. Soc. 162, 587–590. doi:10.1144/0016-764905-004
Han, J., Leng, J., and Wang, Y. (2016). Characteristics and Genesis of the Polygonal Fault System in Southern Slope of the Qiongdongnan Basin, South China Sea. Mar. Pet. Geology. 70, 163–174. doi:10.1016/j.marpetgeo.2015.11.022
Hansen, D. M., Shimeld, J. W., Williamson, M. A., and Lykke-Andersen, H. (2004). Development of a Major Polygonal Fault System in Upper Cretaceous Chalk and Cenozoic Mudrocks of the Sable Subbasin, Canadian Atlantic Margin. Mar. Pet. Geology. 21, 1205–1219. doi:10.1016/j.marpetgeo.2004.07.004
He, C., Tang, L., Huang, D., and Shi, S. (2010). Polygonal Faults in the Sanzhao Sag of the Songliao basin: Their Significance in Hydrocarbon Accumulation. Mining Sci. Tech. (China) 20, 300–305. doi:10.1016/s1674-5264(09)60202-7
Henriet, J. P., De Batist, M., Van Vaerenburgh, W., and Verschuren, M. (1988). Seismic Facies and clay Tectonic Features of the Ypresian clay in the Southern North Sea. Bull. Belg. Geol. Soc. 97, 457–472.
Henriet, J. P., De Batist, M., and Verschuren, M. (1991). Early Fracturing of Paleogene Clays Southernmost North Sea: Relevance to Mechanisms of Primary Hydrocarbon Migration. Spec. Publ. - Eur. Assoc. Pet. Geol. Bull. 1, 217–227.
Higgs, W. G., and McClay, K. R. (1993). Analogue Sandbox Modelling of Miocene Extensional Faulting in the Outer Moray Firth. Geol. Soc. Lond. Spec. Publications 71, 141–162. doi:10.1144/gsl.sp.1993.071.01.07
Hoffmann, J. J. L., Gorman, A. R., and Crutchley, G. J. (2019). Seismic Evidence for Repeated Vertical Fluid Flow through Polygonally Faulted Strata in the Canterbury Basin, New Zealand. Mar. Pet. Geology. 109, 317–329. doi:10.1016/j.marpetgeo.2019.06.025
Hustoft, S., Mienert, J., Bünz, S., and Nouzé, H. (2007). High-resolution 3D-Seismic Data Indicate Focussed Fluid Migration Pathways above Polygonal Fault Systems of the Mid-Norwegian Margin. Mar. Geology. 245, 89–106. doi:10.1016/j.margeo.2007.07.004
Ilg, B. R., Hemmings-Sykes, S., Nicol, A., Baur, J., Fohrmann, M., Funnell, R., et al. (2012). Normal Faults and Gas Migration in an Active Plate Boundary, Southern Taranaki Basin, Offshore New Zealand. Bulletin 96, 1733–1756. doi:10.1306/02011211088
Ireland, M. T., Morley, C. K., and Davies, R. J. (2021). Systematic Spacing and Topological Variations in Layer‐bound Fault Systems. Basin. Res. 33, 2745–2762. doi:10.1111/bre.12582
Jackson, C. A. L., Carruthers, D. T., Mahlo, S. N., and Briggs, O. (2014). Can Polygonal Faults Help Locate Deep-Water Reservoirs? Bulletin 98, 1717–1738. doi:10.1306/03131413104
Larter, S., Aplin, A. C., Bowler, B., Lloyd, R., Zwach, C., Hansen, S., et al. (2000). A drain in My Graben: an Integrated Study of the Heimdal Area Petroleum System. J. Geochemical Exploration 69-70, 619–622. doi:10.1016/s0375-6742(00)00068-6
Laurent, D., Gay, A., Baudon, C., Berndt, C., Soliva, R., Planke, S., et al. (2012). High-resolution Architecture of a Polygonal Fault Interval Inferred from Geomodel Applied to 3D Seismic Data from the Gjallar Ridge, Vøring Basin, Offshore Norway. Mar. Geology. 332-334, 134–151. doi:10.1016/j.margeo.2012.07.016
Li, J., Mitra, S., and Qi, J. (2020). Seismic Analysis of Polygonal Fault Systems in the Great South Basin, New Zealand. Mar. Pet. Geology. 111, 638–649. doi:10.1016/j.marpetgeo.2019.08.052
Liu, Z. B., Ma, S. Z., Sun, Y., Zhang, J. G., and Lv, Y. F. (2008). High-resolution Sequence Stratigraphy Division and Depositional Characteristics of Putaohua Reservoir, Sanzhao Depression. Acta Sedimentol. Sin. 26, 399–406.
Lonergan, L., Cartwright, J., and Jolly, R. (1998a). The Geometry of Polygonal Fault Systems in Tertiary Mudrocks of the North Sea. J. Struct. Geology. 20, 529–548. doi:10.1016/s0191-8141(97)00113-2
Lonergan, L., Cartwright, J., Laver, R., and Staffurth, J. (1998b). Polygonal Faulting in the Tertiary of the central North Sea: Implications for Reservoir Geology. Geol. Soc. Lond. Spec. Publications 127, 191–207. doi:10.1144/gsl.sp.1998.127.01.14
Meng, Q., Hao, F., and Tian, J. (2021). Origins of Non-tectonic Fractures in Shale. Earth-Science Rev. 222, 103825. doi:10.1016/j.earscirev.2021.103825
Morgan, D. A., Cartwright, J. A., and Imbert, P. (2015). Perturbation of Polygonal Fault Propagation by Buried Pockmarks and the Implications for the Development of Polygonal Fault Systems. Mar. Pet. Geology. 65, 157–171. doi:10.1016/j.marpetgeo.2015.03.024
Nicol, A., Walsh, J. J., Watterson, J., Nell, P. A. R., and Bretan, P. (2003). The Geometry, Growth and Linkage of Faults within a Polygonal Fault System from South Australia. Geol. Soc. Lond. Spec. Publications 216, 245–261. doi:10.1144/gsl.sp.2003.216.01.16
O'Brien, G. W., Lawrence, G. M., Williams, A. K., Glenn, K., Barrett, A. G., Lech, M., et al. (2005). Yampi Shelf, Browse Basin, North-West Shelf, Australia: a Test-Bed for Constraining Hydrocarbon Migration and Seepage Rates Using Combinations of 2D and 3D Seismic Data and Multiple, Independent Remote Sensing Technologies. Mar. Pet. Geology. 22, 517–549. doi:10.1016/j.marpetgeo.2004.10.027
Ostanin, I., Anka, Z., di Primio, R., and Bernal, A. (2012). Identification of a Large Upper Cretaceous Polygonal Fault Network in the Hammerfest basin: Implications on the Reactivation of Regional Faulting and Gas Leakage Dynamics, SW Barents Sea. Mar. Geology. 332-334, 109–125. doi:10.1016/j.margeo.2012.03.005
Seebeck, H., Tenthorey, E., Consoli, C., and Nicol, A. (2015). Polygonal Faulting and Seal Integrity in the Bonaparte Basin, Australia. Mar. Pet. Geology. 60, 120–135. doi:10.1016/j.marpetgeo.2014.10.012
Shin, H., Santamarina, J. C., and Cartwright, J. A. (2008). Contraction-driven Shear Failure in Compacting Uncemented Sediments. Geol 36, 931–934. doi:10.1130/g24951a.1
Shin, H., Santamarina, J. C., and Cartwright, J. A. (2010). Displacement Field in Contraction-Driven Faults. J. Geophys. Res. 115, B07408. doi:10.1029/2009jb006572
Shoulders, S. J., Cartwright, J., and Huuse, M. (2007). Large-scale Conical sandstone Intrusions and Polygonal Fault Systems in Tranche 6, Faroe-Shetland Basin. Mar. Pet. Geology. 24, 173–188. doi:10.1016/j.marpetgeo.2006.12.001
Stuevold, L. M., Faerseth, R. B., Arnesen, L., Cartwright, J., and Möller, N. (2003). Polygonal Faults in the Ormen Lange Field, Møre Basin, Offshore Mid Norway. Geol. Soc. Lond. Spec. Publications 216, 263–281. doi:10.1144/gsl.sp.2003.216.01.17
Sun, Q., Wu, S., Cartwright, J., and Dong, D. (2012). Shallow Gas and Focused Fluid Flow Systems in the Pearl River Mouth Basin, Northern South China Sea. Mar. Geology. 315-318, 1–14. doi:10.1016/j.margeo.2012.05.003
Sun, Q., Wu, S., Lü, F., and Yuan, S. (2010). Polygonal Faults and Their Implications for Hydrocarbon Reservoirs in the Southern Qiongdongnan Basin, South China Sea. J. Asian Earth Sci. 39, 470–479. doi:10.1016/j.jseaes.2010.04.002
Tewksbury, B. J., Hogan, J. P., Kattenhorn, S. A., Mehrtens, C. J., and Tarabees, E. A. (2014). Polygonal Faults in Chalk: Insights from Extensive Exposures of the Khoman Formation, Western Desert, Egypt. Geology 42, 479–482. doi:10.1130/g35362.1
Turrini, L., Jackson, C. A.-L., and Thompson, P. (2017). Seal rock Deformation by Polygonal Faulting, Offshore Uruguay. Mar. Pet. Geology. 86, 892–907. doi:10.1016/j.marpetgeo.2017.06.038
Velayatham, T., Holford, S. P., Bunch, M. A., and King, R. C. (2021). Fault Controlled Focused Fluid Flow in the Ceduna Sub-Basin, Offshore South Australia; Evidence from 3D Seismic Reflection Data. Mar. Pet. Geology. 127, 104813. doi:10.1016/j.marpetgeo.2020.104813
Victor, P., and Moretti, I. (2006). Polygonal Fault Systems and Channel Boudinage: 3D Analysis of Multidirectional Extension in Analogue Sandbox Experiments. Mar. Pet. Geology. 23, 777–789. doi:10.1016/j.marpetgeo.2006.06.004
Watterson, J., Walsh, J., Nicol, A., Nell, P. A. R., and Bretan, P. G. (2000). Geometry and Origin of a Polygonal Fault System. J. Geol. Soc. 157, 151–162. doi:10.1144/jgs.157.1.151
Wu, S. G., Sun, Q. L., Wu, T. Y., Yuan, S. Q., Ma, Y. B., and Yao, G. H. (2009). Polygonal Fault and Oil-Gas Accumulation in Deep-Water Area of Qiongdongnan Basin. Acta Petrolei. Sinica. 30, 22–26. 32. doi:10.7623/syxb200901005
Keywords: polygonal faults, fluid migration, genesis mechanism, dewatering, seismic data
Citation: Xia Y, Yang J, Chen Y, Lu S, Wang M, Deng S, Yao Z and Lu M (2022) A Review of the Global Polygonal Faults: Are They Playing a Big Role in Fluid Migration?. Front. Earth Sci. 9:786915. doi: 10.3389/feart.2021.786915
Received: 30 September 2021; Accepted: 08 December 2021;
Published: 21 January 2022.
Edited by:
Weiqi Fu, China University of Mining and Technology, ChinaReviewed by:
Serdar Dindar, Izmir Kâtip Çelebi University, TurkeyCopyright © 2022 Xia, Yang, Chen, Lu, Wang, Deng, Yao and Lu. This is an open-access article distributed under the terms of the Creative Commons Attribution License (CC BY). The use, distribution or reproduction in other forums is permitted, provided the original author(s) and the copyright owner(s) are credited and that the original publication in this journal is cited, in accordance with accepted academic practice. No use, distribution or reproduction is permitted which does not comply with these terms.
*Correspondence: Jinxiu Yang, eWFuZ2ppbnhpdUB1cGMuZWR1LmNu
Disclaimer: All claims expressed in this article are solely those of the authors and do not necessarily represent those of their affiliated organizations, or those of the publisher, the editors and the reviewers. Any product that may be evaluated in this article or claim that may be made by its manufacturer is not guaranteed or endorsed by the publisher.
Research integrity at Frontiers
Learn more about the work of our research integrity team to safeguard the quality of each article we publish.