- 1Institute of Global Environmental Change, Xi’an Jiao Tong University, Xi’an, China
- 2College of Urban and Environmental Sciences, Northwest University, Xi’an, China
- 3Research Center for Earth System Science, Yunnan University, Kunming, China
- 4State Key Lab of Loess and Quaternary Geology, Institute of Earth Environment, Chinese Academy of Sciences, Xi’an, China
- 5Department of Earth and Environmental Sciences, University of Minnesota, Minneapolis, MN, United States
The dissolved uranium (U) content in the water column of saline lakes varies little between ice-free seasons throughout the whole water column. Such uniformity allows for the potential absolute dating and/or paleohydrologic interpretations of lake sediments and biogenic shell materials using U isotopes. Before using these methods in cold regions, however, it is necessary to evaluate the effects that ice freeze-thaw processes have on the distribution of U isotopes in saline lake waters, and to determine the amount of variation in U isotopic values when such processes occur. In this paper, we collected ice and dissolved water samples from six lakes with variable salinity in February 2021. Five groundwater and three water samples from rivers into Qinghai Lake were sampled in November 2020. The sampled water was analyzed for dissolved concentrations of 238U and the activity ratio of 234U/238U ([234U/238U]AR). The results show that the 238U concentration of ice samples was less than that of the underlying water. The [234U/238U]AR of ice in the five saline lakes was similar to that of the underlying water with less than a 10‰ variation, suggesting no observable fractionation between ice and dissolved water. Thus, the ice freeze-thaw processes have almost no effect on the uranium content and [234U/238U]AR of the sampled saline lakes, which were characterized by a limited recharge volume from surface runoff, groundwater, and ice volume, namely the close saline lake in arid alpine background. The results from the indoor freeze-thaw experiments also showed that the U isotopic composition of Qinghai Lake waters and ice were similar with the 238U concentration of the ice was about 40% of that of the dissolved lake water, supporting the data obtained from natural saline lakes. The above results provide important insights into whether it is feasible to use U isotopes for absolute dating and/or paleohydrologic analysis of lake sediments or biogenic shell materials. In addition, the results are important for evaluating the [234U/238U]AR and uranium concentrations in seawater when there exists a process of melting polar ice, and for determining the initial delta 234U variations needed for dating of coral and other fossil materials.
Introduction
Studies of the distribution of dissolved uranium (U) isotopes in water have mainly focused on problems of glacial sedimentation at the two poles and oceanic circulation (Sarin and Church, 1994; Moran et al., 1997). Existing research results show that the U concentration of seawater is relatively consistent, and exhibits a linear relationship with salinity (Rengarajan et al., 2003; Schmidt, 2006). Changes observed in the atmosphere, hydrosphere, cryosphere, and biosphere indicate that the Earth has warmed (Desbruyères et al., 2016; Francis et al., 2017; Lavergne et al., 2019). During the preceding decades, key indicators of the climate system are changing at unprecedented rates during at least the last two centuries, which are at levels that have not been observed in hundreds to thousands of years (Hansen et al., 2010; Tierney et al., 2020; Trewin et al., 2020). During the past 10 years (2011–2020), temperature exceeded the warmest centennial-scale range that was reconstructed for the Holocene during about the past 6,500 years [0.2–1°C relative to 1850–1900] (Gulev et al., 2021). The total loss of land ice (including both glaciers and ice sheets) was the largest contributor to global mean sea level rise, which was estimated to occur at a rate of about 3.7 [3.2–4.2] mm/year between 2006 and 2018 (Cazenave et al., 2018; Velicogna et al., 2020). The above processes will result in a change in U content and the activity ratio of 234U/238U ([234U/238U]AR) of sea water. This change may provide insights into the nature of the change in sea level as well as various oceanographic circulation patterns and processes. Changes in U isotopic abundances are also needed to establish the initial delta 234U variations in corals, which are often used for dating. Thus, it is necessary and of significance to understand the effects of ice freeze-thaw processes on the U isotope distribution of sea water including the 238U concentration and the [234U/238U]AR. Further, limited hydrological studies on saline lake systems have been conducted to date, and research methods required for their hydrological analysis have yet to be systematically developed. In fact, very few studies have explored the 238U concentration and [234U/238U]AR of saline lakes, or the effects of ice freeze-thaw processes on U isotope distribution in lake waters.
Hydrologically closed saline lakes located in the Qinghai region and the Qaidam Basin of China provide the potential to precisely evaluate the effects of ice freeze-thaw processes on U isotope distribution in both saline and fresh lake waters of variable salinity. These lakes are also easily accessible allowing for efficient sample collection. In this study, we selected several lakes with different salinities in the Qinghai-Tibet Plateau and sampled ice and the underlying water for a complete U isotope analysis to gain insights into the effects of ice freeze-thaw processes on the U isotope distribution of the lake waters. We also conducted inhouse (laboratory) freezing experiments on three batches of lake water samples collected between 2018 and 2021 from Qinghai Lake to evaluate the effects of ice freeze-thaw processes on the U isotope distribution of lake water. By combining the U isotope distribution results from both the inhouse freezing experiments and natural lake water samples, we obtained a better understanding of the changes in U distribution in lake waters that occur during the ice freeze-thaw process, which helps in exploring the potential for using U isotopes as environmental indicators and/or the dating of corals and other fossil materials.
Materials and Methods
Sampling Locations
There are many lakes in the Qinghai region and Qaidam Basin. Most of these lakes are classified as saline lakes, in that they possess a salinity with the variation from 8.86 to 72.36‰. The water samples used in this study were collected from six lakes with different salinities. Four of the sampled lakes were located in the northeastern part of the Qaidam Basin, including Gahai Lake (GH), Keluke Lake (KLK), Tuosu Lake (TS), and Xiligou Lake (XLG) (Figure 1). These four lakes can be divided into three categories based on the basis of salinity: freshwater lakes (including KLK), saline lakes (TS, XLG) and salt lakes (GH). The study area is characterized by a typical alpine continental climate in which large temperature differences occur between day and night. Precipitation in the region is low, while evaporation throughout the year is relatively high. The main vegetation types include alpine meadows and alpine step communities. The overall elevation of the area is about 3,000 m masl. Han et al. (2019) determined, using data from the National Meteorological Station of Delhi City, that the mean annual precipitation over the period of 1961–2017 was 185.1 mm, and the mean annual evaporation ranged between 2,242.8 and 2,439.4 mm. Precipitation is unevenly distributed during the year, showing a unimodal distribution. Maximum precipitation occurs in June to July, while the minimum precipitation occurs in December. The total area of GH is about 37 km2; it possesses a mean water depth of 8 m and a maximum water depth of 15 m. The lake basin has no perennial surface rivers. Recharge relies on atmospheric precipitation and subsurface runoff. TS has a total area of about 180 km2 and a maximum water depth of 25.7 m. The main source of the water in TS originates from drainage, atmospheric precipitation, and groundwater (Zheng and Zhang, 2002; Huang and Han, 2007).
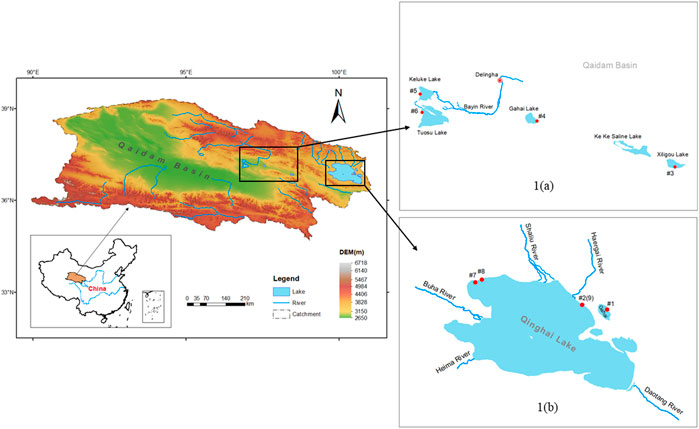
FIGURE 1. Map showing the locations of the sampled lakes in the northeast part of the Qaidam Basin and Qinghai region.
In addition to the four lakes sampled in the Qaidam Basin, water and ice samples were collected from two lakes with varied salinities in the Qinghai region: Qinghai Lake (QHH) and Qinghai Gahai (QHGH) (Figure 1). The surface area of Lake Qinghai (36°15′–38°20′N, 97°50′–101°20′E) is approximately 4,400 km2. The area of the lake is approximately 29,660 km2. The geological and geographical environment and current climate of this area have been examined in detail by Colman et al. (2007) and Jin et al. (2010).
Lake Qinghai is the largest inland close lake in China by surface area. The lake is developed within a basin surrounded by three mountain ranges including the Datong Mountains to the north, the Riyue Mountains to the east, and the Qinghai Nanshan Mountains to the south (Bian et al., 2000). The lake is divided into two nearly equal sub-basins by a NNW-trending horst, from which an island (Mt Haixin) emerges. Several minor fault scarps also can be found in the lake basin. The lake bed is generally flat and the lake is hydrologically closed and evaporative (Zhang et al., 1994). The six largest rivers that form the Lake Qinghai catchment are, in order of their discharge, the Buha, Shaliu, Hargai, Quanji, Daotang and Heima rivers. Hydrologically, these rivers are greatly impacted by the area’s monsoonal climate, and they supply over 87% of the water, dissolved load, and sediment discharge to the lake. Over half of these rivers come from the Buha River (Jin et al., 2009). Meltwater from surrounding mountain glaciers accounts for only 0.3% of the total runoff (Colman et al., 2007).
Qinghai Gahai Lake (36°57′–37°3′N, 100°31′–100°36′E) is located along the northeast bank of Qinghai Lake at an altitude of 3,196.6 m. As one of the three sub-lakes of Qinghai Lake, Qinghai Gahai is now hydrologically closed without river inputs. The lake is 12 km long from northwest to southeast, and 6 km wide from southwest to northeast. It possesses a surface area of 47.5 km2. Qinghai Gahai Lake is generally 8–9.5 m deep and slightly alkaline with a pH of 9.25. The salt content of the lake water is about 31.73 g/L. The lake is recharged by shallow groundwater. The center of the lake is located about 400 m from the coast, and there is deep groundwater recharge at the bottom of the lake.
Sampling Design and Methods
All ice and water samples were collected in February 2021 (see Table 1 for details). To determine the effect of collecting samples in different years on U content in lake water, four samples were collected in Qinghai Lake from 2017 to 2019. In addition, river water and groundwater samples were collected from the rivers recharging Qinghai Lake to verify whether surface runoff would affect lake U concentrations and [234U/238U]AR. Previous studies showed that the U concentration and [234U/238U]AR of waters from the same lake in the Qaidam Basin varied little aerially or vertically within the water column or seasonally through time (Zhang et al., 2019; Zhao et al., 2020). Thus, we believe that the samples from one location of each lake are sufficient to represent the U content and the [234U/238U]AR distribution characteristics of these sampled lakes, and that the U data of surface water can represent the entire water column of the lake. Sampling periods were selected that possessed large quantities of lake ice in the northeastern part of the Qaidam Basin and the Qinghai region; during the other seasons, the lakes are nearly ice free. Thus, the sampled ice and lake waters should show the typical U distribution within the ice and the underlying water. By comparing the U composition of the sampled lake water in summer with the maximum water input published in Zhao et al. (2020), we expected to gain a deeper understanding of effects of ice freeze-thaw processes on U isotope distribution in saline lake waters and the potential environmental implications on the observed U data.
To further evaluate the effects of ice freeze-thaw processes on the U isotope distribution of lake water, three water samples were collected from QHH in September 2018, July 2019 and February 2021 (Table 2). We preformed inhouse freezing experiments on these three QHH water samples in the laboratory to further verify the results of the data obtained from the “natural” ice and water samples collected in the field.
Samples of surface ice and the underlying water were collected dozens of meters offshore in high density polyethylene (HDPE) plastic bottles (Nalgene 2002-0032) by hand wearing Derma Free Vinyl Gloves. After collection, about 1,000 ml of each water sample was filtered through a mixed cellulose filter membrane (0.8-µm pore size, Millipore AAWP04700) with a manual vacuum filter system (Nalgene, 300-4100; Nalgene, 6133-0010). The filtrates were stored for later analysis.
After collection, ice samples were placed at room temperature to melt. The melted ice water was also filtered through a mixed cellulose filter membrane (0.8-μm pore size, Millipore AAWP04700) using a manual vacuum filter system (Nalgene, 300-4100; Nalgene, 6133-0010), and stored for later analysis. The research of Ku et al. (1977) showed that there is no evident difference on the [234U/238U]AR on the filtered Atlantic seawater and the unfiltered seawater, but has little effect on U concentration. Therefore, we believe that U concentration filtered by 0.8 μm filters could represent the content of dissolved U in saline lake water to a certain extent.
QHH water samples were collected in three batches over various sampling periods for in-house freezing experiments. The experiment mimicked natural sample freezing conditions in the field, during which the three previously filtered QHH water samples were frozen at −18°C in a freezer for 5–6 h to form half water and half ice. Then the ice and water were separated, and stored for later analysis.
Analytical Methods
The samples were analyzed for selected water chemistry parameters (e.g., anions, cations, salinity, total dissolved solids (TDS), and pH at the Key Laboratory of Surface System and Environmental Carrying Capacity of Shaanxi Province, Northwest University. Anion and cation concentrations of the samples were determined using two Dionex AQUION ICs (Chen et al., 2007; Scientific, 2016). Conductivity (ms/cm), TDS (g/L), salinity (‰), and pH were determined using a Mettler-Toledo Five Easy Plus pH meter. Uranium isotopes in the samples were measured at the Xi’an Jiaotong University Isotope Laboratory. The analysis of U isotope abundances included a two-step process, including chemical separation and instrumental analysis. Chemical separation involved sample digestion, centrifugal coprecipitation, ion exchange resin separation, uranium isotope collection, and purification. More specifically, to analyze the U isotopic compositions of the water, the acidified aliquots of each sample (∼25 ml) were spiked with 233U–236U–229Th, preconcentrated and digested with HNO3 and HClO4, and then co-precipitated with Fe oxyhydroxide. The Fe precipitate was moved to a centrifuge tube for centrifugation and rinsed with deionized H2O (>18 MΩ) to eliminate water-soluble seawater cations. The precipitate was then dissolved in 14 N HNO3 (1 N = 1 M) and moved to a Teflon beaker, before being dried and dissolved in 7 N HNO3 for anion-exchange chromatography with an AG1-X8, 100–200 mesh size resin and a polyethylene frit. Separation was performed in Teflon columns with a 0.25 ml column volume (CV). The first separation was performed in Teflon columns with 0.5 ml 7 N HNO3, 0.25 ml of 8 N HCl (to get rid of Fe and Th fraction), and 0.5 ml of deionized H2O (to gather the U fraction). The U fractions were then dried with two drops of HClO4 and brought to volume with 7 N HNO3. The final U fractions were then dried with two drops of HClO4 and dissolved in weak nitric acid for analysis on the mass spectrometer. The concentrations of 234U, 235U, and 238U were calculated by isotope dilution using the nuclide ratios determined on a Thermo-Finnigan Neptune mass spectrometer. All measurements were performed using a peak-jumping routine in ion counting mode on the discreet dynode multiplier behind the retarding potential quadrupole. Each sample measurement was bracketed by the measurement of an aliquot of the run solution, which was utilized to adjust for the instrument background count rates on the measured masses. For U, the measurement uncertainties involved propagated errors from the ICP-MS isotope ratio measurements, spike concentrations, and blank corrections. The procedural blanks for chemical and mass spectrometric analyses at the Laboratory of Isotope Geochemistry in Xi’an Jiao Tong University are approximately 50 ag (1.3 × e5 atoms) for 234U; 3 fg (7.7 × e6 atoms) for 235U; and 150 fg (3.8 × e8 atoms) for 238U.
The experimental analysis methods can be divided into two parts: chemical separation and MC-ICP-MS mass spectrometry. For chemical separation, we used duplicate samples in each batch to ensure the reliability of the data. The experimental results showed that the repeatability of the duplicate samples in the chemical analysis process was excellent (Table 3), and the yield of all samples was about 93%. For instrument analysis process, NBL-112A (New Brunswick Laboratory) U isotope standard solution was used for external calibration during the analysis by MC-ICP-MS mass spectrometer. The experimental results showed that the average of NBL-112A standard solution was −38.23 ± 1.21‰(2σ), and the measurement precision of dissolved 238U content and [234U/238U]AR in lake water samples was 0.89–1.35‰ (2σ). The methods used herein have been fully described by Cheng et al. (2000, 2013) and Shen et al. (2002, 2012).
Results
Distribution of Uranium Isotopes in Lake Ice and Waters in the Qinghai Region and the Northeastern Part of Qaidam Basin
The water chemistry results of each sampled lake are presented in Table 4, including the aqueous 238U concentration and the [234U/238U]AR. Aqueous 238U concentrations of the freshwater lakes in the northeastern part of the Qaidam Basin were generally low, which is consistent with the results of previous analyses of lake water samples collected in June, July and the beginning of December without ice coverage (Zhao et al., 2020). The 238U concentration of ice from the five sampled lakes were less than that of water under the ice, except for samples from QHH. The 238U concentrations of the ice from the five lakes with varying salinity ranged from approximately three to forty percent of the U content of the underlying water. The lower the salinity of lake water, the lower U content in the forming overlying ice. The 238U concentration of ice in QHH was slightly less than that of water under the ice. The reason for this is not clear at this time and will be the topic of future studies.
The [234U/238U]AR values were similar between the ice and water from the six lakes of varying salinity; the [234U/238U]AR exhibited very limited variation. In fact, the variation in [234U/238U]AR ratios between the ice and water in the five saline lakes and the salt lake were all within 2%. The variation in [234U/238U]AR between ice and water in the freshwater lake reached up to 23%.
In conclusion, during the icing process, the U content was significantly different between the ice and water; it was lower in ice than in water. In contrast, the [234U/238U]AR did not change significantly between the saline lakes; differences in the values were within 2% and slightly lower in ice than the underlying water.
Distribution of Uranium Isotopes in Ice and Water From QHH as Determined by Inhouse Freezing Experiments
Laboratory studies were carried out to 1) further determine the fidelity of the isotopic changes observed during the freezing and transformation of “natural” lake water to ice, and 2) assess the anomalies in the results of ice and water in Qinghai Lake during the ice-on (covered) season. The indoor freeze-thaw experiments were conducted on three batches of QHH water samples collected in different seasons, including periods when the lake was and was not covered in ice. The samples from QHH were obtained in September 2018, July 2019 and February 2021.
The results of the inhouse freezing experiments were similar to that observed for the natural lake samples (Table 5). The 238U concentration of the ice was slightly less than that of the water in all three batches of samples. The 238U concentration of the ice was about 40% of that in the water. In addition, we noticed that the 238U concentrations of the water collected in February were lower than those of the water obtained in July and September. The [234U/238U]AR resulting from the inhouse freezing experiments also supports those of the nature samples. The activity ratios did not change significantly during the phase transition from water to ice in the three batches of collected samples; differences between the samples were within 0.5%.

TABLE 5. Results of inhouse freezing experiments on three batches of Qinghai Lake water samples collected during different seasons.
Distribution of Uranium Isotope in Recharge Rivers and Groundwater Recharging in Qinghai Lake
In November 2020, five groundwater samples in recharge rivers and three water samples from recharging rivers into Qinghai Lake. The water chemistry results of each sampled river are presented in Table 6, including the aqueous 238U concentration and the [234U/238U]AR. Aqueous 238U concentrations of the recharge rivers of Qinghai Lake is 2.1–2.6 μg/L, and [234U/238U]AR is 2.010–2.325, with a limited variation. The 238U concentrations and [234U/238U]AR of the groundwater in Qinghai Lake region ranged from 3.8 to 14.4 μg/L and 1.442 to 2.562 respectively. The U concentration of recharge rivers and groundwater is lower than that of Qinghai Lake, while [234U/238U]AR is higher than that of Qinghai Lake.
Discussion
U Isotopes Variations and the Controlling Factors in the Ocean and Saline Lake System
U Isotopes Variations and the Controlling Factors in the Ocean
Seawater [234U/238U]AR is higher than secular equilibrium (Chen et al., 1986). This reflects the process of α-recoil during decay of 238U which leads to high [234U/238U]AR in river waters (Chabaux et al., 2008) and to a flux of 234U into the oceans from marine sediment pore-waters (Klinkhammer and Palmer, 1991). These inputs keep the seawater [234U/238U]AR high and counteract the decay of excess 234U in the oceans, which constantly attempts to return it to a secular equilibrium value of 1. Uranium concentrations and [234U/238U]AR have been determined in 63 seawater samples from the Atlantic, the Pacific, the Arctic, and the Antarctic oceans. These analyses show that: 1) the uranium concentration of seawater with a salinity of 35‰ was 3.35 ± 0.2 µgl−1; and 2) the [234U/238U]AR was 1.14 ± 0.03. Current estimates of the average world river uranium concentration (0.3–0.6 µgl−1) and [234U/238U]AR (1.2–1.3) and of the diffusional 234U influx from sediments (0.3 dpm cm−2 10−3 yr−1) are essentially consistent with a model that depicts a steady state distribution of uranium in the ocean. However, the 0.3–0.6 µgl−1 values for river uranium may be an upper limit estimate. The ratios reported for global rivers ([234U/238U]AR = 1.171; Chabaux et al., 2008), and the ratios measured for four rivers discharging into Lake Qinghai located in an arid alpine climate.
[234U/238U]AR varied between 1.984 and 2.506 (Zhang et al., 2019). New Zealand rivers located in an Alpine high latitude area exhibited a wide range of [234U/238U]AR, varying from 1.09 to 4.61 (Robinson et al., 2004). This range spans that previously measured in rivers from elsewhere.
Uranium is removed from the oceans by diffusion across the sediment-water interface of organic-rich sediments. This pathway is the largest single sink in the global uranium budget of this element (Klinkhammer and Palmer, 1991). Dissolved uranium is drawn into suboxic sediments along a concentration gradient established by the precipitation of an insoluble phase which forms when U(VI) is reduced to U(IV) (Ku et al., 1977). This transformation occurs relatively late in the diagenetic sequence, after the microbially mediated dissolution of manganese and iron oxides, and may be induced by the onset of sulfate reduction. Metallo-organics play an important role in the diagenetic behavior of this element as some uranium is released into solution when labile organics are consumed at the sediment-water interface (Francke et al., 2020). In contrast, the diagenesis of authigenic iron and manganese oxides exerts a negligible influence on the uranium diagenetic cycle (Klinkhammer and Palmer, 1991). Variations in the uranium concentration of sediment with time are controlled directly by the uranium content of the source material settling from the water column, and indirectly, by the organic content of this material and the sedimentation rate. Since diffusion from seawater dramatically influences the short-term burial rate of uranium, down-core distributions of dissolved and solid uranium can provide an estimate of recent sedimentation rates in rapidly accumulating sediments. The research from Cowart (1980) determined the concentration of dissolved uranium and [234U/238U]AR were determined in water samples from 23 locations in the Edwards carbonate aquifer of south central Texas. The [234U/238U]AR was about 1.20 in an oxidized aquifer and above 2.0 in a reduced aquifer.
U Isotope Variations and the Controlling Factors in the Saline Lake System
Limited studies on hydrological systems in saline lakes have been conducted and research methods have been systematically developed. The research dates back to Osmond et al. (1974) who established a two-end mixing model based on the relationship between [234U/238U]AR, 1/U, and U/TDS (total dissolved solids) in the water of Sambhar lake. They found that one end of the lake had a low uranium content and low salinity, while the other end had the opposite. The U content measured by Zhao et al. (2020) in several saline lakes and our unpublished salt lakes shows the 238U concentration varied with the range of 12.6–200 μg/L. Other studies have also shown that the U content of lakes water varies directly with TDS (salinity) and pH, the latter of which is partially controlled by evaporation and/or other secondary concentration pathways (Osmond et al., 1974; Borole et al., 1982; Yadav and Sarin, 2009; Zhang et al., 2019; Chen et al., 2020; Zhao et al., 2020). Moreover, the U concentration indicate that the length of these processes, which are controlled by the duration of water-rock interaction, is probably an important factor to consider when accounting for the variation in lake salinities within a similar geographical area. In addition, research has shown that biogeochemical process have an impact on the mobility of uranium species. These biogeochmical processes include the behavior of colloids suspended phase and colloids, the sorption and complexation processes on inorganic soil constituents such as clay minerals, oxides, Mn (III/IV) oxides, and organic matter, biological fixation and transformation processes, and competition with a certain number of OM and/or cations (such as Ca2+ and Mg2+) (Andersson et al., 2001; Koch-Steindl and PröHl, 2001; Fredrickson et al., 2002; Dong and Brooks, 2006; Belli et al., 2015; Strakhovenko and Gas’kovaet, 2018; Chen et al., 2020; Francke et al., 2020). The [234U/238U]AR in saline lakes is closely related to the input of water to the lake, evaporation or other secondary concentration pathways, water–rock interactions involving sediments, the influx of atmosphere dust, and the system’s organic matter, content, among other factors as well as the evolution, degree of metamorphous, and hydrochemistry and biogeochemistry of the saline lakes (Koch-Steindl and PröHl, 2001; Strakhovenko and Gas’kovaet, 2018; Zhang et al., 2019; Chen et al., 2020; Francke et al., 2020; Zhao et al., 2020).
Applications to Paleoceanography
Global greenhouse gas warming controlled erosion processes are predicted to affect 61% of the ice-free landmasses during the 21st century, a number that increases to 80% when land use is considered (Ostberg et al., 2018). These processes have the potential to induce large variability in the weathering of the continents and the composition of chemical fluxes to the ocean. Seawater [234U/238U]AR provide global-scale information about continental weathering and are vital for marine uranium-series geochronology. Existing research suggests that the Atlantic [234U/238U]AR started to increase before major sea-level rise and overshot the modern value by 3‰ during early deglaciation. In addition [234U/238U]AR during deglacial times in the Pacific Ocean converged with that in the Atlantic after the abrupt resumption of Atlantic meridional overturning circulation. This led Chen et al. (2016) to suggest that ocean mixing and early deglaciation released excess 234U through the enhanced subglacial melting of the Northern Hemisphere ice sheets, and the change in 234U has driven the observed evolution in [234U/238U]AR. To assess the reliability of this hypothesis, it is necessary to evaluate the migration and transformation of 238U concentration and the [234U/238U]AR during the transformation between ice and seawater of a given salinity and set of hydrochemical conditions. Therefore, we conducted this study to explore whether the freezing and thawing process of ice affects the U concentration and [234U/238U]AR in lake waters, which were easier to sample than waters in polar regions.
As shown in Figure 2, the 238U concentration of the ice from the five lakes with varying salinity was approximately three to forty percent of the U content of the overlying water. Moreover, the lower the salinity, the lower the ratio of U in ice to the underlying water. The [234U/238U]AR between ice and water in the six lakes with varying salinity were similar and varied within a narrow range of about 2%. All exhibited lower [234U/238U]AR in ice than in the underlying water. In addition, the [234U/238U]AR observed during the inhouse freezing experiments of water from QHH supported the observations of the nature lake samples; differences in the ratios between ice and water in the three batches of samples did not change significantly as they were within the measurement error (Figure 3). In conclusion, the 238U concentration and the [234U/238U]AR resulting from the indoor freeze-thaw experiments support the results of the field observations of the natural saline lake samples. The concentration of 238U of seawater consistently varies by about 3.2 ppb between the Pacific and Atlantic Oceans (Chen et al., 1986). The 238U concentration of ice possessed a lower concentration than that of water, and thus did not result in an observable change in the 238U concentration of seawater.
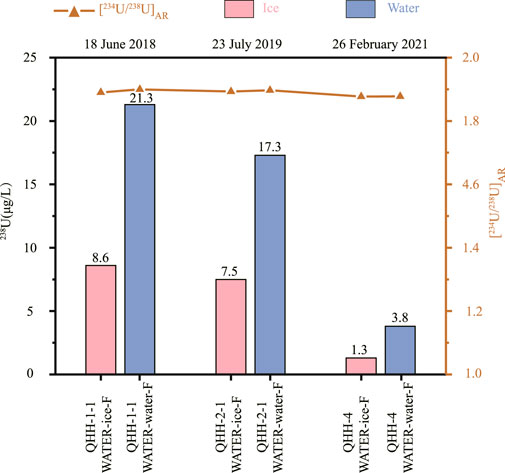
FIGURE 3. Differences in 238U concentration and [234U/238U]AR between ice and water obtained during the inhouse freezing experiments conducted on three batches of Qinghai Lake water samples.
These data show that the freezing and thawing process of ice does not cause [234U/238U]AR to change in the remaining water. Chen et al. (2016) did mention that other factors other than ice melting could be responsible for the increase [234U/238U]AR. However, their main point was that the early deglacial release of excess 234U drove the observed evolution in [234U/238U]AR. If the increase of [234U/238U]AR was dependent on factors other than ice melting (e.g. the leaching of continental matter into the water), then the Pacific and Atlantic oceans at lower latitudes would have warmed faster, melted faster, and responded faster to the [234U/238U]AR change, which is not that the case.
To try to understand the cause of the above phenomenon, we first explored whether the freeze-thaw process of ice leads to an increase in the activity ratio of the water column using natural samples and inhouse freeze-thaw experiments. The preliminary results showed that the freezing and thawing process of ice does not cause [234U/238U]AR increase of the remaining water. Second, we explored whether the input of the river could increase the [234U/238U]AR of the remaining water. Current estimations of the average world river uranium concentration (0.3–0.6 µgl−1) and [234U/238U]AR (1.2–1.3), combined with the diffusional 234U influx from sediments (0.3 dpm cm−2 10−3yr−1), are essentially consistent with a model that depicts a steady state distribution of uranium in the ocean. However, the 0.3–0.6 µgl−1 values for river uranium may be an upper limit estimate (Ku et al., 1977). The ratios reported for global rivers ([234U/238U]AR = 1.171) (Chabaux et al., 2008) and the ratios of four rivers discharging into the Lake Qinghai catchment located in an arid alpine climate possessed [234U/238U]AR varying from 1.984 to 2.506 (Zhang et al., 2019). New Zealand rivers located in alpine high latitude regions exhibit a very wide range of [234U/238U]AR, varying between 1.09 and 4.61 (Robinson et al., 2004). This range spans that previously measured in rivers from other locations. Based on the above [234U/238U]AR in river waters from low latitudes, and the ratios reported for global rivers ([234U/238U]AR = 1.171) (Chabaux et al., 2008), it would be difficult for low latitude riverine inputs to result in a 3 per mil increase in the [234U/238U]AR of sea water in the Pacific and Atlantic because of a higher [234U/238U]AR input of river. Thus, we speculate that the North Atlantic Ocean, which is also located at low latitudes, responds faster than the Pacific Ocean, most likely because the Arctic Ocean, located at high latitudes, carries inputs from five recharging rivers characterized by sandy and clay particle debris, then when the early deglaciation occurs. The inputs from rivers with high [234U/238U]AR leads to an increase in the [234U/238U]AR in the Arctic Ocean, which in turn affects the North Atlantic waters at low latitudes first and then affects the Pacific Ocean by way of the oceanic conveyor belt. Third, uranium is removed from the oceans by diffusion across the sediment-water interface of organic-rich sediments. This pathway is the largest single sink in the global budget of this element (Klinkhammer and Palmer, 1991). Dissolved uranium is drawn into suboxic sediments along a concentration gradient established by the precipitation of an insoluble phase which forms when U(VI) is reduced to U(IV) (Ku et al., 1977). Cowart (1980) measured the concentration of dissolved uranium and [234U/238U]AR were determined in water samples from 23 locations in the Edwards carbonate aquifer of south central Texas, and found that the [234U/238U]AR was about 1.20 in an oxidized aquifer, and above 2.0 in reduced aquifer. Thus, during deglaciation, there exists one possibility, the reduced seawater below the ice is mixed into the overlying seawater.
In summary, we suggest when early deglaciation occurs, the mixing of reduced seawater and/or the inputs from rivers with high [234U/238U]AR leads to an increase in the [234U/238U]AR in the Arctic Ocean, which in turn affects the North Atlantic waters at low latitudes first before influencing the Pacific Ocean by way of the oceanic conveyor belt. The specific reasons for this phenomenon need to be explored in future studies.
Based on the results of the in-house freezing experiments, there were differences in [234U/238U]AR in the ice and water sampled in February (winter) and July/September (summer); lower [234U/238U]AR values were measured in winter than in summer. However, there were no differences in [234U/238U]AR between the ice and water samples obtained in February (winter) (Table 5; Figure 3). Similar results were observed for the natural lake samples collected in winter and summer (Table 4, Table 5).
These results, combined with the limited ice volume in the Arctic, provide insights into the observed change in [234U/238U]AR in the Atlantic, which started to increase before major sea-level rise and overshot the modern value by 3‰ during early deglaciation. We speculate that the phenomenon may be due to a change in the redox conditions to a more oxidized state at the bottom of the water column during the early stages of deglaciation and/or from the inputs of river waters from high altitude regions that usually possess a higher activity ratio. Because at pH values and CO2 concentrations typical of oxic seawater, the predominant uranium species, U(VI), is highly soluble and occurs as a stable carbonate ion complex, Ca/Mg-UO2−(CO3) complexes (Djogić et al., 1986; Dong and Brooks, 2006; Chen et al., 2020). In contrast, in oxygen-depleted waters, rapid removal of U by the sediment depends on reduction of U(VI) to U(IV) (Tribovillard et al., 2006; Algco and Tribovillard, 2009). In addition, a large quantity of uranium in depositional archives is mostly bound to organic matter as demonstrated by weak to strong positive correlations between naturally occurring uranium and OM contents (Min et al., 2000; Regenspurg et al., 2010; Francke et al., 2020). Uranium can be mineralized in the presence of OM forming strong UO2-OM bonds (Lenhart et al., 2000; Li et al., 2014). If the uranium bound to residual OM controls [234U/238U]AR, then non-detrital uranium is usually characterized by values above 1. Thus, when polar ice melting is combined with the oxidation of the sea floor, the minerogenic U-OM bounds and/or U-carboxyl groups should be preferentially oxidized and released with higher [234U/238U]AR. This hypothesis needs to be tested by further work.
The accurate evaluation of excess 234U is important for marine uranium-series geochronology; thus, it is also necessary to investigate the causes of the variation in the [234U/238U]AR of seawater. For example, if the 234U concentration of coral during glacial periods is about 3.2 ppm, and the initial [234U/238U]AR differs by 3‰, then the determined age will differ by thousands of years (Esat and Yokoyama, 2006).
Applications to Ancient Lakes
Previous studies of 238U concentration and the [234U/238U]AR showed that there was no significant change between sampling sites in the same saline lake based on our published paper (Zhang et al., 2019; Zhao et al., 2020). There is also no evident difference between samples that were collected from a specific lake on different dates during the summer without ice coverage. Lake water samples collected during periods of maximum and minimum discharge have also been used to evaluate the seasonal variations in aqueous 238U. We found that 238U concentration did not change with the seasons in these saline lakes. If the 238U concentration and the [234U/238U]AR remain consistent through time, then sediment ages and/or sedimentation rates can be determined from lake sediment and/or biogenic carbonate in the future, thus allowing for the accurate reconstruction of paleoclimatic and paleoenvironmental conditions.
Since the saline lakes in the Qaidam Basin and Qinghai region have a freezing period of about 3–4 months per year, it is necessary to determine whether the 238U concentration and the [234U/238U]AR remain consistent. The results obtained herein show that differences in 238U concentration existed between the ice and the underlying water in all sampled saline lakes and in fresh water. Significant differences in 238U concentration occurred between winter ice and summer lake waters (without ice) that are significantly recharged by exogenous rivers, including TS, KLK, and QHH. Conversely, there was no significant differences in 238U concentration between winter ice and summer waters (without ice) in saline lakes receiving less exogenous riverine inputs, including QHGH, XLG and GH [lake water data in summer were published in Zhang et al. (2019) and Zhao et al. (2020)]. Therefore, in the arid Qaidam Basin and Qinghai region, the freeze-thaw process of ice does not appear to affect the concentration of 238U in saline lakes, but the proportion of exogenously recharged water may affect its concentration. In addition, when ice exists with limited oxygen exchange with atmospheric, with the increase of water column, changes in the redox environment at the sediment-water interface may also affect the concentration of 238U in the water column. Based on our results, the 238U concentration in lacustrine sedimentary secondary carbonate minerals and the interannual resolution of biogenic carbonate shells are expected to provide useful insights into lake paleohydrological changes. The 238U concentration of biogenic carbonate shells may possess a seasonal resolution that could reveal the redox environment at the water-sediment interface in QHH.
There were no significant differences in the [234U/238U]AR of the five saline lakes between ice and the underlying water when both were collected at the same time. The differences in the [234U/238U]AR were all within 2%, with lower [234U/238U]AR in ice. Except for QHGH, there were no significant differences (i.e., exceeding measurement error) in the [234U/238U]AR between winter ice and summer waters on various sampling dates. By contrast, there were significant differences in the [234U/238U]AR of lake waters between winter ice and summer waters in QHH, XLG, GH, TS and KLK (see Zhang et al. 2019; Zhao et al., 2020). The differences in U concentration and [234U/238U]AR in lake water between freezing and non-freezing seasons may be due to the influences of several factors such as surface runoff, groundwater and ice volume.
The study area of this paper is in an arid area of high altitude. Most of the lakes studied are closed saline lakes, and the area of surface runoff and underground recharge of these lakes are very limited. Taking Qinghai Lake as an example (which has the largest proportion of replenishment in the study area), the average water level is 3,193.2 m, the lake surface area is 4,260 km2, and the lake water reserve is 71.593 billion m3 (Liu, 2004). Using changes in calculated water volume from 1959 to 2000, the average annual surface water inflow to Qinghai Lake was 1.526 billion m3. Precipitation on the lake surface contributed about 1.561 billion m3 to the lake, and groundwater replenished 603 million m3 of water to the lake (Liu, 2004). The ratio of precipitation over the lake surface to recharge for Qinghai Lake is the highest of the lakes studied. Zhu and Xie (2018) summarized the annual and seasonal precipitation of Qinghai Lake in different years during the past 50 years. The annual precipitation of Qinghai Lake was concentrated in summer and autumn, and the precipitation was the lowest in winter (Zhu and Xie, 2018). In the previous study, we selected samples collected in Qinghai Lake in different years with different precipitation patterns, and the experimental results showed that precipitation and surface runoff in Qinghai Lake had almost no effect on U content and [234U/238U]AR of the lake (Table 7). In 2020, we collected water from a river that recharged Qinghai Lake and groundwater near the recharge river and measured its U content and [234U/238U]AR (Table 6). The results showed that the effects of atmospheric precipitation, surface runoff and groundwater on U concentration and [234U/238U]AR of Qinghai Lake water were negligible. In addition, the samples studied in this paper were collected at the end of February, when the water in Qinghai Lake exhibited its maximum degree of ice cover. The average depth of Qinghai Lake is about 21m, and the average thickness of the ice sheet is about 29.4 cm. Only ∼2% (by volume) of the surface water was frozen. Consequently, the limited volume of ice will not affect U concentration and [234U/238U]AR of the sampled saline lakes.
The ice freeze-thaw processes have almost no effect on the uranium content and [234U/238U]AR of the sampled saline lakes, which were characterized by a limited recharge volume from surface runoff, groundwater, and ice volume, namely the close saline lake in arid alpine background. An exception was U content in Qinghai Lake. We speculate that the low U concentration in the water column of Qinghai Lake during winter freezing is due to the 30 cm thick ice layer that prevents the infiltration of atmospheric oxygen, with the increase of depth of water column (∼21 m). The ice-water interface resembles a redox interface, resulting in a low U content of the water column under the ice.
In summary, the freeze-thaw processes of the lake will not result in significant changes in [234U/238U]AR in excess of 2% in the water column of saline lakes. The [234U/238U]AR in these saline and freshwater lakes are closely related to the characteristics of the input water and the associated water–rock interactions involving sediments, atmospheric dust and organic material, among other factors during the evolution stage, metamorphous degree, and hydrochemistry of the saline lakes. Based on these results, we suggest that there is a potential to maintain relatively consistent levels of [234U/238U]AR in saline lakes characterized by a closed lake basin and which have a limited input of exogenous recharge water and limited organic matter. Usually, the [234U/238U]AR of dissolved water will increase when organic matter is present (Francke et al., 2020). The [234U/238U]AR of dissolved water from exogenously recharged water is usually above 1, increasing with an increase in latitude and altitude (Chabaux et al., 2008,2011; Zhang et al., 2019; Zhao et al., 2020). In such lake environments, lacustrine sedimentary secondary carbonate minerals and interannual biogenic carbonate shells are expected to provide data valuable for reconstructing lake chronologies.
Conclusion
Samples of ice and the underlying water from six lakes of differing salinity were collected for U composition analysis. These data were combined with the U composition determined for ice and water during inhouse freezing experiments conducted on three batches of water samples from QHH. The results show that there were no significant differences in the [234U/238U]AR of the five saline lakes between ice and the overlying water. The observed variations in [234U/238U]AR were all within 2%, with lower [234U/238U]AR in ice. The inhouse freezing experiments conducted on the three batches of QHH samples support the [234U/238U]AR results obtained from the natural samples. These preliminary data do not support the hypothesis presented in earlier publications that the deglacial release of excess 234U from enhanced subglacial melting of the Northern Hemisphere ice sheets caused the observed increase in 234U/238U values by 3‰. We speculate that the phenomenon may be due to changes in redox water mixing during the onset of deglaciation and/or to the input of recharge waters from rivers located at high altitudes that usually possess a higher activity ratio. By comparing the data obtained herein with published [234U/238U]AR data from saline lakes sampled during the summer without ice coverage, we can speculate that there is a potential to maintain relatively consistent levels of [234U/238U]AR in saline lakes within closed lake basins, particularly those that possess limited organic material, and that are characterized by a limited influx of exogenous recharge water (e.g., GH). In such lake environments, lacustrine sediments containing secondary carbonate minerals and interannual biogenic carbonate shells are expected to be useful for reconstructing a lake chronology.
In addition, there was no significant difference in 238U concentrations between winter ice and summer water in saline lakes with less exogenous river recharge and limited groundwater, including QHGH, XLG and GH. Thus, the 238U concentration of lacustrine secondary carbonate minerals and interannual biogenic carbonate shells are expected to reveal lake paleohydrological changes in such parameters as the redox environment at the water-sediment interface.
Data Availability Statement
The original contributions presented in the study are included in the article/supplementary material, further inquiries can be directed to the corresponding authors.
Author Contributions
The research was supervised by PZ and XL with guidance provided by RE. Samples were collected by PZ, XP, CC, and XL. Experiment method was instructed by PZ, CC. The paper written by PZ with revised provided by LT, XL, YN, and RE. All authors have read and agreed to the published version of the manuscript.
Funding
This study was supported by the National Natural Science Foundation of China (No. 41873013, No. 41888101) and U.S. NSF (No. 1702816).
Conflict of Interest
The authors declare that the research was conducted in the absence of any commercial or financial relationships that could be construed as a potential conflict of interest.
Publisher’s Note
All claims expressed in this article are solely those of the authors and do not necessarily represent those of their affiliated organizations, or those of the publisher, the editors, and the reviewers. Any product that may be evaluated in this article, or claim that may be made by its manufacturer, is not guaranteed or endorsed by the publisher.
References
Algeo, T. J., and Tribovillard, N. (2009). Environmental Analysis of Paleoceanographic Systems Based on Molybdenum–Uranium Covariation. Chem. Geology. 268 (3), 211–225. doi:10.1016/j.chemgeo.2009.09.001
Andersson, P. S., Porcelli, D., Gustafsson, Ö., Ingri, J., and Wasserburg, G. J. (2001). The Importance of Colloids for the Behavior of Uranium Isotopes in the Low-Salinity Zone of a Stable Estuary. Geochimica Et Cosmochimica Acta 65 (1), 13–25. doi:10.1016/s0016-7037(00)00514-7
Belli, K. M., DiChristina, T. J., Van Cappellen, P., and Taillefert, M. (2015). Effects of Aqueous Uranyl Speciation on the Kinetics of Microbial Uranium Reduction. Geochimica et Cosmochimica Acta 157 (15), 109–124. doi:10.1016/j.gca.2015.02.006
Bian, Q., Liu, J., Luo, X., Xiao, J., and Levshin, A. (2000). Geotectonic Setting, Formation and Evolution of the Qinghai Lake. Seismology Geology. 22 (1), 20–26.
Borole, D. V., Krishnaswami, S., and Somayajulu, B. L. K. (1982). Uranium Isotopes in Rivers, Estuaries and Adjacent Coastal Sediments of Western India: Their Weathering, Transport and Oceanic Budget. Geochimica Et Cosmochimica Acta 46 (2), 125–137. doi:10.1016/0016-7037(82)90240-x
Cazenave, A., Meyssignac, B., Ablain, M., Balmaseda, M., Bamber, J., Barletta, V., et al. (2018). Global Sea-Level Budget 1993-present. Earth Syst. Sci. Data 10 (3), 1551–1590. doi:10.5194/ESSD-10-1551-2018
Chabaux, F., Bourdon, B., and Riotte, J. (2008). Chapter 3 U-Series Geochemistry in Weathering Profiles, River Waters and Lakes. J. Environ. radioactivity 13, 49–104. doi:10.1016/S1569-4860(07)00003-4
Chabaux, F., Granet, M., Larqué, P., Riotte, J., Skliarov, E. V., Skliarova, O., et al. (2011). Geochemical and Isotopic (Sr, U) Variations of lake Waters in the Ol'khon Region, Siberia, Russia: Origin and Paleoenvironmental Implications. Comptes Rendus Geosci. 343, 462–470. doi:10.1016/j.crte.2011.07.004
Chen, J. H., Edwards, R. L., and Wasserburg, G. J. (1986). 238U, 234U and 232Th in Seawater. Earth Planet. Sci. Lett. 80 (3-4), 241–251. doi:10.1016/0012-821x(86)90108-1
Chen, T., Robinson, L. F., Beasley, M. P., Claxton, L. M., Andersen, M. B., Gregoire, L. J., et al. (2016). Ocean Mixing and Ice-Sheet Control of Seawater 234U/238U during the Last Deglaciation. Science 354 (6312), 626–629. doi:10.1126/science.aag1015
Chen, X., Zheng, W., and Anbar, A. D. (2020). Uranium Isotope Fractionation (238U/235U) during U(VI) Uptake by Freshwater Plankton. Environ. Sci. Technol. 54, 2744–2752. doi:10.1021/acs.est.9b06421
Chen, Z. L., Mallavarapu, M., and Naidu, R. (2007). Determination of Bromate and Bromide in Seawater by Ion Chromatography, with an Ammonium Salt Solution as mobile Phase, and Inductively Coupled Plasma Mass Spectrometry. Chromatographia 65 (1-2), 115–118. doi:10.1365/s10337-006-0128-z
Cheng, H., Edwards, R. L., Hoff, J., Gallup, C. D., Richards, D. A., and Asmerom, Y. (2000). The Half-Lives of Uranium-234 and Thorium-230. Chem. Geology. 169 (1-2), 17–33. doi:10.1016/s0009-2541(99)00157-6
Cheng, H., Lawrence Edwards, R., Shen, C.-C., Polyak, V. J., Asmerom, Y., Woodhead, J., et al. (20132013). Improvements in 230Th Dating, 230Th and 234U Half-Life Values, and U-Th Isotopic Measurements by Multi-Collector Inductively Coupled Plasma Mass Spectrometry. Earth Planet. Sci. Lett. 371-372, 82–91. doi:10.1016/j.epsl.2013.04.006
Colman, S. M., Yu, S. Y., An, Z., Shen, J., and Henderson, A. C. G. (2007). Late Cenozoic Climate Changes in China’s Western interior: A Review of Research on Lake Qinghai and Comparison with Other Records. Quat. Sci. Rev. 26 (2007), 2281–2300. doi:10.1016/j.quascirev.2007.05.002
Cowart, J. B. (1980). The Relationship of Uranium Isotopes to Oxidation/reduction in the Edwards Carbonate Aquifer of Texas. Earth Planet. Sci. Lett. 48, 277–283. doi:10.1016/0012-821x(80)90191-0
Desbruyères, D. G., Purkey, S. G., Mcdonagh, E. L., Johnson, G. C., and King, B. A. (2016). Deep and Abyssal Ocean Warming from 35years of Repeat Hydrography. Geophys. Res. Lett. 43 (19), 10,356–10,365. doi:10.1002/2016gl070413
Djogić, R., Sipos, L., and Branica, M. (1986). Characterization of Uranium(VI) in Seawater1. Limnol. Oceanogr. 31 (5), 1122–1131. doi:10.4319/lo.1986.31.5.1122
Dong, W., and Brooks, S. C. (2006). Determination of the Formation Constants of Ternary Complexes of Uranyl and Carbonate with Alkaline Earth Metals (Mg2+, Ca2+, Sr2+, and Ba2+) Using Anion Exchange Method. Environ. Sci. Technol. 40 (15), 4689–4695. doi:10.1021/es0606327
Esat, T. M., and Yokoyama, Y. (2006). Variability in the Uranium Isotopic Composition of the Oceans over Glacial-Interglacial Timescales. Geochimica et Cosmochimica Acta 70 (16), 4140–4150. doi:10.1016/j.gca.2006.06.013
Francis, J. A., Vavrus, S. J., and Cohen, J. (2017). Amplified Arctic Warming and Mid-latitude Weather: New Perspectives on Emerging Connections. Wiley Interdiscip. Rev. Clim. Change 8 (5), e474. doi:10.1002/wcc.474
Francke, A., Dosseto, A., Just, J., Wagner, B., and Jones, B. G. (2020). Assessment of the Controls on (234U/238U) Activity Ratios Recorded in Detrital Lacustrine Sediments. Chem. Geology. 550, 119698. doi:10.1016/j.chemgeo.2020.119698
Fredrickson, J. K., Zachara, J. M., Kennedy, D. W., Liu, C., Duff, M. C., Hunter, D. B., et al. (2002). Influence of Mn Oxides on the Reduction of Uranium(VI) by the Metal-Reducing Bacterium Shewanella Putrefaciens. Geochimica et Cosmochimica Acta 66 (18), 3247–3262. doi:10.1016/s0016-7037(02)00928-6
Gulev, S., Thorne, P., Ahn, J., Dentener, F., Domingues, C., Gerland, S., et al. (2021). Climate Change 2021: The Physical Science Basis. Contribution of Working Group I to the Sixth Assessment Report of the Intergovernmental Panel on Climate Change. UK: Cambridge University Press.
Han, T. F., Qi, D. L., Chen, H. S., Xiang, S. G., Shi, X. Y., Liu, C. F., et al. (2019). Temporal and Spatial Distribution Characteristics of Precipitation in Qaidam Basin. Desert and Oasis Meteorology 013 (002), 69–75. (in Chinese).
Hansen, J., Ruedy, R., Sato, M., and Lo, K. (2010). Global Surface Temperature Change. Rev. Geophys. 48 (4), RG4004. doi:10.1029/2010RG000345
Huang, Q., and Han, F. Q. (2007). Salt Lake Evolution and Paleoclimate Fluctuations in Qaidam Basin. Beijing: Science Press. (in Chinese).
Jin, Z., You, C.-F., Yu, T.-L., and Wang, B.-S. (2010). Sources and Flux of Trace Elements in River Water Collected from the Lake Qinghai Catchment, NE Tibetan Plateau. Appl. Geochem. 25 (10), 1536–1546. doi:10.1016/j.apgeochem.2010.08.004
Jin, Z., Yu, J., Wang, S., Zhang, F., Shi, Y., and You, C.-F. (2009). Constraints on Water Chemistry by Chemical Weathering in the Lake Qinghai Catchment, Northeastern Tibetan Plateau (China): Clues from Sr and its Isotopic Geochemistry. Hydrogeol J. 17 (8), 2037–2048. doi:10.1007/s10040-009-0480-9
Klinkhammer, G. P., and Palmer, M. R. (1991). Uranium in the Oceans: Where it Goes and Why. Geochimica et Cosmochimica Acta 55, 1799–1806. doi:10.1016/0016-7037(91)90024-y
Koch-Steindl, H., and Pröhl, G. (2001). Considerations on the Behaviour of Long-Lived Radionuclides in the Soil. Radiat. Environ. Biophys. 40 (2), 93–104. doi:10.1007/s004110100098
Ku, T.-L., Mathieu, G. G., and Knauss, K. G. (1977). Uranium in Open Ocean: Concentration and Isotopic Composition. Deep Sea Res. 24 (11), 1005–1017. doi:10.1016/0146-6291(77)90571-9
Lavergne, T., Sørensen, A. M., Kern, S., Tonboe, R., Notz, D., Aaboe, S., et al. (2019). Version 2 of the EUMETSAT OSI SAF and ESA CCI Sea-Ice Concentration Climate Data Records. The Cryosphere 13 (1), 49–78. doi:10.5194/tc-13-49-2019
Lenhart, J. J., Cabaniss, S. E., MacCarthy, P., and Honeyman, B. D. (2000). Uranium(VI) Complexation with Citric, Humic and Fulvic Acids. Radiochimica Acta 88 (6), 345–354. doi:10.1524/ract.2000.88.6.345
Li, P., Xu, Z. Q., Wang, Y., and Wang, P. C. (2014). The Relationships between Uranium Mineralization and Organic Matter in 373 Uranium deposit. Amr 962-965, 203–207. doi:10.4028/www.scientific.net/amr.962-965.203
Liu, X. Y. (2004). Hydrological Characteristics of Qinghai Lake Basin. J. China Hydrol. 24 (002), 60–61. (in Chinese).
Min, M.-Z., Meng, Z.-W., Sheng, G.-Y., Min, Y.-S., and Liu, X. (2000). Organic Geochemistry of Paleokarst-Hosted Uranium Deposits, South China. J. Geochemical Exploration 68 (3), 211–229. doi:10.1016/s0375-6742(99)00085-0
Moran, S. B., Ellis, K. M., and Smith, J. N. (1997). 234Th/238U Disequilibrium in the central Arctic Ocean: Implications for Particulate Organic Carbon export. Deep Sea Res. Part Topical Stud. Oceanography 44 (8), 1593–1606. doi:10.1016/s0967-0645(97)00049-0
Osmond, J. K., Kaufman, M. I., and Cowart, J. B. (1974). Mixing Volume Calculations, Sources and Aging Trends of Floridan Aquifer Water by Uranium Isotopic Methods. Geochimica Et Cosmochimica Acta 38 (7), 1083–1100. doi:10.1016/0016-7037(74)90006-4
Ostberg, S., Boysen, L., Schaphoff, S., Lucht, W., and Gerten, D. (2018). The Biosphere under Potential Paris Outcomes. Earth's Future 6 (4), 23–39. doi:10.1002/2017ef000628
Regenspurg, S., Margot-Roquier, C., Harfouche, M., Froidevaux, P., Steinmann, P., Junier, P., et al. (2010). Speciation of Naturally-Accumulated Uranium in an Organic-Rich Soil of an alpine Region (Switzerland). Geochimica et Cosmochimica Acta 74 (7), 2082–2098. doi:10.1016/j.gca.2010.01.007
Rengarajan, R., Sarin, M. M., and Krishnaswami, S. (2003). Uranium in the Arabian Sea: Role of Denitrification in Controlling its Distribution. Oceanologica Acta 26 (5-6), 687–693. doi:10.1016/j.oceact.2003.05.001
Robinson, L. F., Henderson, G. M., Hall, L., and Matthews, I. (2004). Climatic Control of Riverine and Seawater Uranium-Isotope Ratios. Science 305 (5685), 851–854. doi:10.1126/science.1099673
Sarin, M. M., and Church, T. M. (1994). Behaviour of Uranium during Mixing in the Delaware and Chesapeake Estuaries. Estuarine, Coastal Shelf Sci. 39 (6), 619–631. doi:10.1016/s0272-7714(06)80013-2
Schmidt, S. (2006). Impact of the Mediterranean Outflow Water on Particle Dynamics in Intermediate Waters of the Northeast Atlantic, as Revealed by 234Th and 228Th. Mar. Chem. 100 (4), 289–298. doi:10.1016/j.marchem.2005.10.017
Scientific, T. (2016). Dionex Aquion Ion Chromatography System Installation Instructions. Waltham, MA, USA: Thermo Scientific Press.
Shen, C.-C., Wu, C.-C., Cheng, H., Lawrence Edwards, R., Hsieh, Y.-T., Gallet, S., et al. (2012). High-precision and High-Resolution Carbonate 230Th Dating by MC-ICP-MS with SEM Protocols. Geochimica et Cosmochimica Acta 99, 71–86. doi:10.1016/j.gca.2012.09.018
Shen, C. C., Edwards, R. L., Cheng, H., Dorale, J. A., Thomas, R. B., Moran, S. B., et al. (2002). Uranium and Thorium Isotopic and Concentration Measurements by Magnetic Sector Inductively Coupled Plasma Mass Spectrometry. Chem. Geology. 185 (3), 165–178. doi:10.1016/s0009-2541(01)00404-1
Strakhovenko, V. D., and Gas’kova, O. L. (2018). Thermodynamic Model of Formation of Carbonates and Uranium mineral Phases in Lakes Namshi-Nur and Tsagan-Tyrm (Cisbaikalia). Russ. Geology. Geophys. 59, 374–385. doi:10.1016/j.rgg.2017.05.002
Tierney, J. E., Poulsen, C. J., Montañez, I. P., Bhattacharya, T., Feng, R., Ford, H. L., et al. (2020). Past Climates Inform Our Future. Science 370 (6517). doi:10.1126/science.aay3701
Trewin, B., Cazenave, A., Howell, S. E. L., Huss, M., Isensee, K., Palmer, M. D., et al. (2020). Headline Indicators for Global Climate Monitoring. Bull. Am. Meteorol. Soc. preprint 102 (1), E20–E37. doi:10.1175/bams-d-19-0196.1
Tribovillard, N., Algeo, T. J., Lyons, T., and Riboulleau, A. (2006). Trace Metals as Paleoredox and Paleoproductivity Proxies: An Update. Chem. Geology. 232 (1-2), 12–32. doi:10.1016/j.chemgeo.2006.02.012
Velicogna, I., Mohajerani, Y., Geruo, A., Landerer, F., Mouginot, J., Noel, B., et al. (2020). Continuity of Ice Sheet Mass Loss in Greenland and Antarctica from the GRACE and GRACE Follow-On Missions. Geophys. Res. Lett. 47 (8), e2020GL087291. doi:10.1029/2020gl087291
Yadav, D. N., and Sarin, M. M. (2009). Geo-chemical Behavior of Uranium in the Sambhar Salt Lake, Rajasthan (India): Implications to "Source" of Salt and Uranium "Sink". Aquat. Geochem. 15 (4), 529–545. doi:10.1007/s10498-009-9066-3
Zhang, P., Cheng, H., Liu, W. G., Mo, L. T., Li, X. Z., Ning, Y. F., et al. (2019). Geochemical and Isotopic (U, Th) Variations in lake Waters in the Qinghai Lake Basin, Northeast Qinghai-Tibet Plateau, China: Origin and Paleoenvironmental Implications. Arabian J. Geosciences 12 (3). doi:10.1007/s12517-019-4255-x
Zhang, P. X., Zhang, B. Z., Qian, G. M., Li, H. J., and Xu, L. M. (1994). The Study of Paleoclimatic Parameter of Qinghai Lake since Holocene. Quat. Sci. 14 (3), 225–238.
Zhao, C., Zhang, P., Li, X., Ning, Y., Tan, L., Edwards, R. L., et al. (2020). Distribution Characteristics and Influencing Factors of Uranium Isotopes in saline Lake Waters in the Northeast of Qaidam Basin. Minerals 10 (1), 74. doi:10.3390/min10010074
Keywords: U isotopes, saline lakes, freeze-thaw processes, Qinghai region, Qaidam basin
Citation: Zhang P, Cao C, Li X, Pei X, Chen C, Liang L, Ning Y, Tan L and Edwards RL (2021) Effects of Ice Freeze-Thaw Processes on U Isotope Compositions in Saline Lakes and Their Potential Environmental Implications. Front. Earth Sci. 9:779954. doi: 10.3389/feart.2021.779954
Received: 20 September 2021; Accepted: 08 November 2021;
Published: 01 December 2021.
Edited by:
Heng Chen, Columbia University, United StatesReviewed by:
Xinming Chen, Florida State University, United StatesAiguo Dong, China University of Geosciences, China
Copyright © 2021 Zhang, Cao, Li, Pei, Chen, Liang, Ning, Tan and Edwards. This is an open-access article distributed under the terms of the Creative Commons Attribution License (CC BY). The use, distribution or reproduction in other forums is permitted, provided the original author(s) and the copyright owner(s) are credited and that the original publication in this journal is cited, in accordance with accepted academic practice. No use, distribution or reproduction is permitted which does not comply with these terms.
*Correspondence: Pu Zhang, emhhbmdwdTM1N0B4anR1LmVkdS5jbg==; Xiangzhong Li, eHpobGkwNEAxNjMuY29t