- 1Cerema, Technopôle Brest Iroise, Plouzané, France
- 2Lab’Urba, Université Gustave Eiffel, Champs-sur-Marne, France
- 3Saint-Venant Hydraulics Laboratory and Cerema, Chatou, France
Coastlines are subject to multiple developments related to land use planning and the effects of climate change. These developments generally lead to an increase in the risk of coastal flooding. This article focuses on systems to protect against this hazard, and presents a bibliographical analysis on methods and operational tools to strengthen their resilience. This analysis is carried out by considering that a system of protection is a component of the territory to which it provides protection and that it is therefore necessary to study in depth the relations between this system and the various components of the territory (the natural environment, the built environment and the social and institutional environment). Based on this hypothesis, the concepts of risk and resilience applied to floods are specified and the protection and adaptation strategies commonly used in recent decades are described. This retrospective on concepts and strategies leads us to structure the state-of-the-art analysis on methods and operational tools in relation to two issues: 1) understanding risks; 2) adapting and transforming protection systems. In each case, the use of the concept of resilience implies a clear distinction between systemic and analytical approaches. Finally, this bibliographical analysis reveals the need to revise the protection system concept in order to promote the integration of these systems in the territories. Accordingly, new methodological developments could be considered using ecosystem and socio-economic approaches.
1 Introduction
Coasts are subject to multiple changes: climate change, demographic growth, poor land management, the unsustainable use of natural resources, declining ecosystems (UNISDR, 2015), etc. These changes generate an increase in natural hazards, as well as the emergence of new risks, by combination or cascade effect (IPCC, 2019). The low elevation coastal zone (classified as having land less than 10 m above sea level), where people and infrastructure are most exposed to coastal hazards, is currently home to around 11% of the global population (around 680 million people). By the year 2050, the population in this zone is projected to grow to more than one billion under all socio-economic scenarios (Merkens et al., 2016). In the coming decades, the expected acceleration of sea level rise in response to continuing global warming will exacerbate the vulnerability of many low-lying, densely populated coastal regions of the world, and very likely will become a major threat in the near future for a significant fraction of humanity (Cazenave and Le Cozannet, 2013). Coastal flooding caused by marine flooding or insufficient drainage of continental waters to the sea is generally the main hazard. The multiple changes and the increase of this hazard over a few decades raises the question of how to adapt the levee systems deployed over a millennium (Deboudt and Goeldner-Gianella, 2015; Guében-Venière, 2015; Welch et al., 2017), especially since the techniques used contribute greatly to the degradation of the protection provided by natural features (Syvitski and Saito, 2007; Syvitski, 2008; Syvitski et al., 2009). In order to reduce the risk of disaster, progressive or more radical changes will have to be considered, not in response to extreme events, but as part of a planned approach (Zevenbergen et al., 2008).
Flood protection may involve many components: event forecasting, protection structures, reduction of vulnerability with urban planning constraints, crisis management plans, etc. (FLOODsite, 2009a). Among all these measures, protection systems, including protection works and natural features, generally remain the first defense of a community. However, the proper functioning of the system requires continuous risk assessment and appropriate maintenance or adaptation strategies and actions (CIRIA MEDDE USACE, 2013). Thus, communities are confronted with two principal issues:
• Managing protection systems with limited material and human resources, to maintain performance;
• Adapting protection systems in response to changes in the environment, and if possible in anticipation of longer-term developments.
The fact that we inherit, generation after generation, the management of these systems, may lead to a preference for the first type of activity, which can result in conservative attitudes, resistance to change, and mainly technical approaches. In this case, methodological guides of structure management and adaptation, e.g., (CIRIA MEDDE USACE, 2013), are considered only as a form of support to continue to maintain the existing performance. Yet in the 21st century, with major disturbances in our environment, adaptation should prevail over resistance to change, to limit social and economic damage (Hallegatte et al., 2013; Klijn et al., 2015).
In this article, the aim is to review the methods and operational tools for managing and adapting flood protection systems, to identify any gaps in the state-of-the-art and to provides new insight for research on the theme of flood protection systems adaptation and transformation.
To achieve this objective, the concepts of risk and resilience applied to floods will be specified and the protection strategies commonly used in recent decades will be described. This retrospective on concepts and strategies will lead us to structure the state-of-the-art analysis on operational methods and tools in relation to two issues:
• Understanding risks;
• Adapting and transforming systems.
Finally, the discussion will focus on the shortcomings of the state-of-the-art and the corresponding avenues of research.
2 Retrospective on Concepts and Strategies
In recent decades, flood protection strategies have been governed by two principles: risk management and resilience development (Disse et al., 2020). A retrospective of the evolution of strategies in relation to the concepts of risk and resilience is therefore essential to inform our thinking and to structure a critical analysis of the methods of understanding and reducing risks. This part will address successively:
• The definition of flood risk and flood risk management strategies;
• The concept of resilience and its definitions;
• The implications of the concept of resilience on methods to understand and reduce risk.
Considering these three points, a structure of the analysis of the state-of-the-art will be proposed.
2.1 Definition of Flood Risk and Flood Risk Management Strategies
Before the concept of resilience led to new approaches to risk prevention, many risk-based strategies were deployed. It is necessary to clarify this concept and to assess how it has been used.
2.1.1 Definition of Flood Risk
As a first approach, the risk of flooding can be understood through the “Source-Pathway-Receptor-Consequence (SPRC)” conceptual model (Gouldby and Samuels, 2005). This is a simple conceptual model for representing processes that lead to a particular consequence (FLOODsite, 2009a). For a risk to arise, there must be:
• A hazard that consists of a “source” or initiator event (e.g., in the marine domain, a cyclone or a tsunami, and in the land domain, coastal river flooding, runoff, rising water tables);
• A “receptor” (e.g., people or properties) that has the potential to be affected by the hazard; and
• A pathway between the source and the receptor (i.e., flood routes including through or over defences).
The SPRC model considers risk to be the result of the exposure of a vulnerable stake to a hazard. Therefore, flood risk can be defined in at least two alternative ways, the former being more suited to qualitative approaches and the latter to quantitative approaches (FLOODsite, 2009b).
The following formula reflects the first definition:
1) risk = hazard (flood) * exposure * vulnerability (of the society or the area).
This first definition is often preferred by urban planners, who usually consider the hazard as a given and utilize spatial planning and crisis management as the means to adapt to that given. The Figure 1 illustrates the application of this concept in the case of the impact assessment of coastal flooding on the Sangatte municipality (France). The cartographic representations of the hazard and the vulnerable exposed stakes give, by superposition, a representation of the risk.
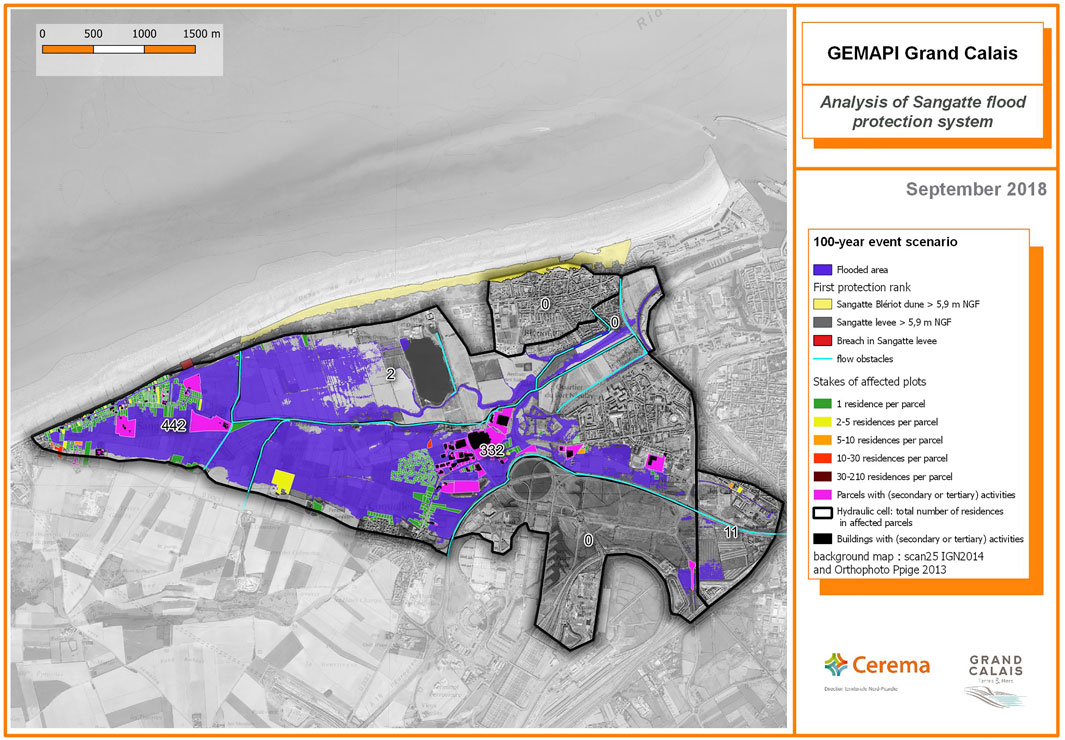
FIGURE 1. Flood risk analysis in the municipality of Sangatte (Cerema, 2020). Credit: Kevin Corsiez, Bruno Kerloc’h, Cerema.
However, in an attempt to quantify risk, and considering that the word « risk » suggests a probability of occurrence, a second definition may be sought. To do this:
• The two terms « exposure » and « vulnerability » are substituted by « consequences », with the consequences being generally more quantifiable (for example, in number of fatalities and economic damage) than the previous two terms;
• The hazard can be represented by its probability distribution.
This yields the second definition:
2) risk = probability (of the flood) * consequences.
The second definition is preferred by engineers, who usually strive for a reduction of the probability of flooding with protection means, and hence need to be able to calculate risk. Thomas et al. (2016) illustrated this approach. These authors examined the Dungeness and Romney Marsh coastal zone (United Kingdom), a region of high value in terms of habitat and energy assets. The objective was to redesign aging coastal defences in order to protect against a 0.5% annual probability event of flooding and to reduce consequences of storm surges to an acceptable level. Considering two parameters—the joint significant wave height (Hs) and water level (WL)—, Thomas et al. (2016) associated a storm impact model (simulating the overwashing discharge at the defence crest) with a flood inundation model (simulating the inundation extent and volume for the Hs and WL combination). Thus, they explored which combinations of wave and water levels generated the greatest threat, as shown by the Figure 2.
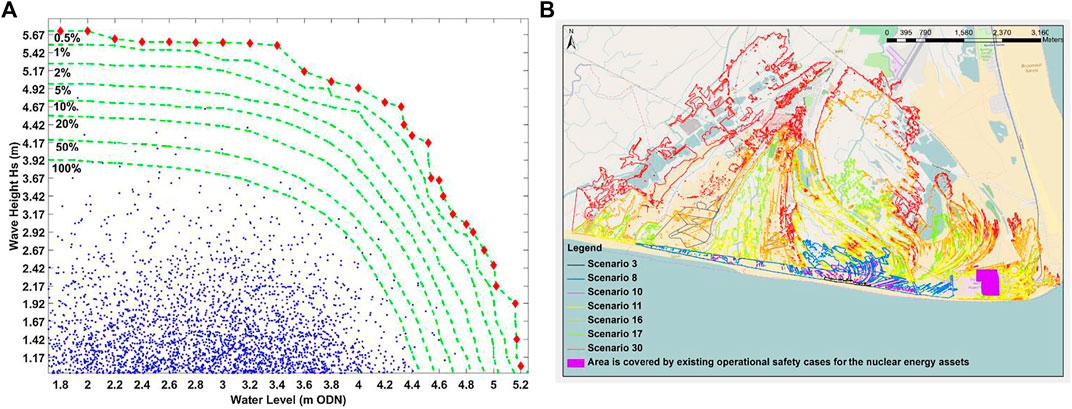
FIGURE 2. (A): joint Hs and WL probability curves of the observed conditions at Dungeness (the blue dots represent wave height and water observations at Dungeness; the green lines are the probability curves (the annual probability of occurrence shown ranges from 100% down to 0.5%); the red diamonds show the 30 selected combinations of wave height and water level that are modelled in the figure on the right). (B): maximum flood extent outlines for seven selected scenarios (Thomas et al., 2016).
Klijn et al. (2015) showed that the two competing definitions can be reconciled and thus the dialogue between levee engineers and planners in charge of town planning can be facilitated.
2.1.2 Flood Risk Management
Flood risk management has changed significantly in recent decades: until the end of the 20th century, the main objective was with strong engineering means, to keep flood off the land. Focus has been on reducing the probability that communities would experience floods, generally through investing in such measures as flood embankments, channel enlargement, pumping schemes, and sea walls. From the 2000s, the main objective became the reduction of risks for people and property. Therefore, measures to reduce the vulnerability of society were considered with the same attention as water management measures (FLOODsite, 2009a).
Figure 3 shows how, following the SPRC model, risk can be reduced by addressing one or more of the three risk factors: vulnerability, exposure, and/or hazard. The reduction of these three risk factors can be achieved through different policy and action choices over time until limits to adaptation might be reached (IPCC, 2019). Diverse measures can be implemented (general land use planning, dedicated protection systems, application of urban planning rules, flood forecasting and warning systems), but a “residual risk” will always remain.
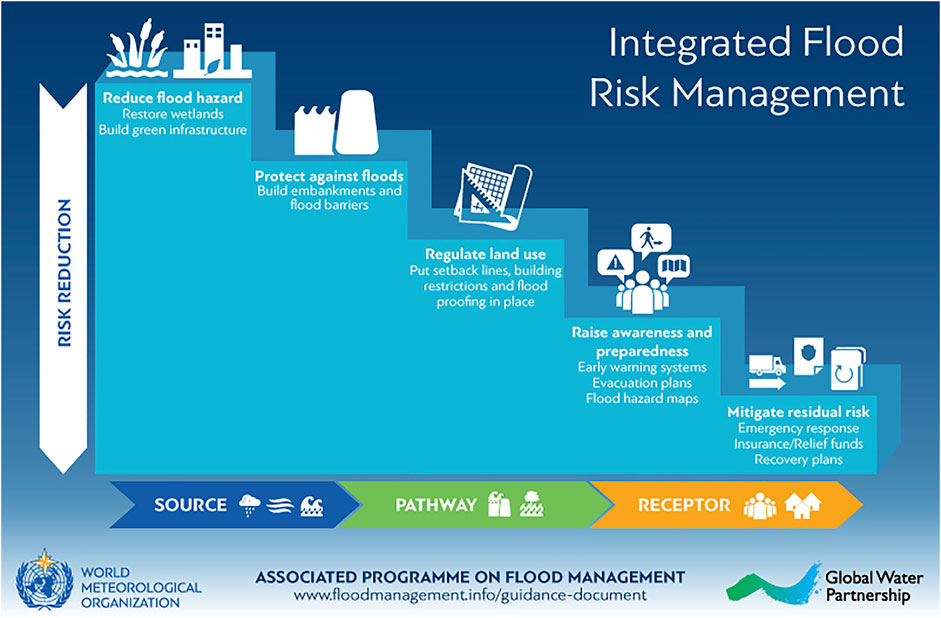
FIGURE 3. Integrated flood risk management (WMO, 2017). Credit: World meteorological organization.
In support of these strategies, the use of numerical models is a well-established technique for the understanding and representation of weather-marine phenomena (Daniel et al., 2007; Tiberi‐Wadier et al., 2021) and tsunamis (Sugawara, 2021), predicting their impacts on coastal zones and warn. In the fluvial field, studies using hydrodynamic models can also be utilised for flood management in general (Mehta et al.,2021), and in particular to make decision relating to the operation of dams, the relocation of people living in floodplain areas (Yadav and Mangukiya, 2021) and the developments of water courses and wetlands (Patel et al., 2018; Mehta and Yadav, 2020; Kumar and Mehta, 2021).
Flood risk management has proven successful at reducing the threat of some flooding hazards. It is a useful approach for assessing risks and guiding decisions on implementing protection measures (Disse et al., 2020). Nevertheless, in the changing context of the 21st century, marked by the increasing artificialization of soils and the multiplication of uses, the degradation of ecosystems and the services they provide (Millenn. Ecosyst. Assess., 2003; Millenn. Ecosyst. Assess., 2005; Campbell et al., 2009), flood risk management strategies based on the SPRC model or other simple models may be insufficient. To halt the ongoing processes in many territories, the scope of disaster risk reduction strategies needs to be broadened to address both natural and man-made hazards and all associated environmental, technological and biological risks (UNISDR, 2015).
To define these new strategies, the conceptual framework had to evolve. The concept of resilience corresponds to a new paradigm in the field of risk management and land use planning.
2.2 The Concept of Resilience and Its Definitions
Resilience enables people and ecosystems to cope with the shocks and stresses associated with changes, and to adapt and even transform themselves as needed. Indeed some changes are so substantial (and, often, abrupt) that they fundamentally alter the functioning of the system (Arctic Council, 2016). Therefore, the concept of resilience is essential to address the adaptation and transformation of the systems.
Resilience has a variety of definitions, although these all share a commonality in referring to the ability of a system to recover from disturbance. Definitions vary regarding what defines a « system » and as to what recovery means (Gersonius., 2016). In this section, we will therefore explore the various definitions that may have been given depending on the types of systems (Rodina, 2018): engineering systems, ecosystems, human societies, or systems encompassing all of these components (social-ecological systems).
According to Holling’s pioneering article (1973), it is possible to distinguish, without opposing them, two types of resilience, later renamed “technical resilience” and “ecological resilience” (Holling, 1996) which apply to engineering systems and ecosystems respectively:
• Engineering resilience is the ability of a system to return to an equilibrium state after a temporary disturbance. The more rapidly it returns, and with the least fluctuation, the more stable it is. The emphasis is on return time: for a « fail-safe » engineering design, efficiency, constancy, and predictability are all sought-after qualities.
• Ecological resilience determines the persistence of relationships within a system and is a measure of the ability of these systems to absorb changes of state variables, driving variables, and parameters, and still persist. Ecological resilience is not based on the equilibrium paradigm but considers multiple equilibria possible (Gunderson, 2000). Hence, resilience is not just about being persistent but also about developing new trajectories and evolving in a new system (Folke, 2006).
With these definitions in mind, a system can be very resilient and still fluctuate greatly, i.e., have low stability.
In addition, for systems such as human communities, social resilience is used to describe the ability of groups or communities to cope with external stresses and disturbances as a result of social, political, and environmental change (Adger, 2000).
Lastly, to address the issues arising in the context of global changes such as climate change and other major anthropogenic disturbances, the concept of resilience has been developed to cover social and ecological aspects more broadly. Socio-ecological resilience is defined as the capacity of interconnected social, economic, and ecological systems to cope with a hazardous event, trend, or disturbance, responding or reorganising in ways that maintain their essential function, identity, and structure. Resilience is a positive attribute when it maintains capacity for adaptation, learning, and/or transformation (Walker et al., 2004).
2.3 Implications of the Concept of Resilience on Methods to Understand and Reduce Risk
Risk management decision-making requires first a proper understanding of risks in order to be able to subsequently consider reducing them. In relation to these two objectives, the methods should therefore contribute to:
• Characterizing the resilience of systems. The objective is not only to assess their capabilities, but also to recognize the limitations of these capabilities and therefore to understand the risks, by identifying events, trends, and disruptions that systems would not be able to cope with.
• The adaptability of systems, that is, the ability of actors to influence resilience (Walker et al., 2004), knowing that their actions in relation to resilience development may be intentional or unintentional (Levin, 1998).
The concept of resilience has a major implication on the methods intended to understand and reduce risks: whatever the definition adopted, resilience refers to a system and consequently leads to a preference for systemic approaches, whereas methods based on simple models (such as the SPRC model) correspond mainly to analytical approaches. The fields of application and potential of these two approaches must be clearly identified in order to be able to use them in a relevant way.
Analytical approaches apply preferentially to stable systems, consisting of elements with so-called linear interactions, that is to say which can be described by fixed rules or by continuous mathematical laws. The guiding principle of these approaches is to proceed in the manner of Descartes (1637), whose principle was to “divide each of the difficulties examined into as many parcels as possible that would be required to best resolve them”. Analytical approaches were developed since the late 1930s and implemented in the industrial field, and then gradually extended to other sectors, including flood protection. Despite the undeniably positive results, the persistence of unanticipated disasters shows that this approach has limits. The emergence of non-detectable or uncontrollable risks through analytical approaches is due to the existence of complex systems, which include:
• The dynamics of interactions, especially the existence of feedback between the elements which makes it difficult to distinguish between causes and effects;
• The simultaneous presence of order and disorder that makes it difficult to predict changes;
• properties and « global » behaviour of the system that are not directly deduced from the properties and behaviour of each of its elements (IMdR, 2018).
In summary, Lissack (1999) defined emergence as an overall system behaviour that comes out of the interaction of many participants-behaviour that cannot be predicted or even envisioned from knowledge of what each component of a system does in isolation.
Contrary to what analytical approaches imply, in a process of aggregating a set of locally controlled situations, risks can emerge at a higher level. The examination of linear processes and cause-effect pairs is insufficient to understand the dynamic behaviour of the whole system (Figure 4). To understand the dynamics of these systems, it is necessary—but unfortunately not always sufficient—to represent the whole system as a « web » (Cassel and Hinsberger, 2017).
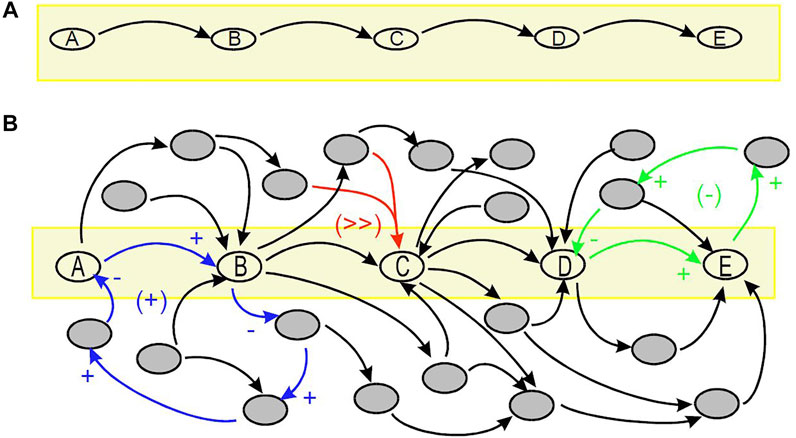
FIGURE 4. (A) A simplified notion of cause–effect chains: A causes B causes C, etc.; (B) a more realistic description of complex cause—effect webs with synergistic effects (>>), positive (+) and negative (−) feedback mechanisms (Cassel-Gintz, 2001).
These two approaches are fundamentally different, to the point that they correspond to two distinct paradigms, of which Krob (2008), in his paper on complex industrial systems, maked a comparison, which stated:
• A systemic approach aims for a comprehensive understanding, applies to heterogeneous systems, is based on views expressed in a collaborative framework, and produces relative conclusions (taking into account external constraints to the system) by utilising logic and systems representation;
• An analytical approach aims for a comprehensive understanding, applies to homogeneous systems, is based on detailed representation modes, utilises experts, and concludes with certainty, using scientific disciplines.
The territories and their protection systems correspond to environments where everything evolves and can be constantly questioned (over a period of a few hours, particularly during a meteo-oceanic event, or over a period of a few decades e.g., due to poorly controlled urbanisation). The dynamic nature and intrinsic complexity of these systems make their comprehension, design, and supervision extremely difficult and analytical approaches inoperative on a global scale. In this context, systemic analysis is intended to define « the architecture of a system », that is to say, its invariant part, which can reasonably be considered as fixed over time (Krob, 2008).
Despite this formal opposition, systemic and analytical approaches are in fact complementary in practice: once the processes of risk emergence have been identified by systemic approaches, analytical approaches are needed to accurately describe the mechanisms at work and quantitatively characterise the hazard. Similarly, since protection principles have been established by a comprehensive systemic approach, the relay should be taken by the engineering analyst to ensure the proper sizing of the system. Therefore, it is the overall scheduling of investigations and analyses that deserves the most attention. Considering that it is preferable to have a global vision of a system before considering more targeted studies, a systemic analysis should generally be undertaken upstream, within the framework of an integrated approach (considering the multiple components of the territory) and should be inclusive (involving multiple stakeholders). This systemic analysis may then include recommendations in its conclusions as to any necessary analytical extensions.
Furthermore, if the complexity of a system depends on the intrinsic parameters, it also depends on extrinsic parameters (ImdR, 2018), including:
• The observer: complexity generally increases with its degree of expertise and requirement;
• The time horizon: complexity is greater on distant horizons with more uncertainty;
• The operating conditions and the available data: when the operating conditions are met and the available data are sufficient, a problem can be relatively simple, and when this is not the case, it can become complex (hence the importance of observation and monitoring).
Ultimately, the concept of resilience leads to the adoption of systemic approaches and in the implementation of these approaches, to being attentive to the nature of the system under consideration, to the context of the analysis, to the point of view adopted and to the time horizon to which it can be conducted. On this last point, it should be stressed that adaptation is an ongoing process of adjusting to changes, with no end point. This means that defining successful adaptation is more about the sustainability of processes and the principles of fairness and equity than it is about measuring outcomes at any given point in time (Stafford-Smith et al., 2011; Hurlimann et al., 2014; Barnett et al., 2015). Successful adaptation is therefore a matter of “socially and environmentally sustainable development pathways, including both social justice and environmental integrity” (Eriksen et al., 2011).
2.4 State-of-The-Art Analysis Principles
The objective of analysing operational methods and tools for strengthening the resilience of protection systems requires specifying the characteristics of the systems studied, the environment in which these systems operate, as well as the points of view according to which these systems should be examined.
2.4.1 System Caracteristics
According to the International Levee Handbook (CIRIA MEDDE USACE, 2013), a levee system is defined as “a set of structures or protective elements, presenting an overall hydraulic coherence to ensure the effective protection of a previously identified group of stakes”.
However, this definition does not explicitly include natural formations, the essential role of which must be stressed in protecting against coastal flooding (IPCC, 2019). Moreover, the term “levee system” refers only to one of the two essential functions of protection systems; that of containing water by obstructing its passage. But a protection system must perform a second function; that of draining the water that accumulates inside the system by facilitating its flow outwards. Therefore, a broader and more explicit definition will be adopted for coastal flood protection systems, namely:
A set of anthropogenic structures and natural formations, presenting a global coherence from the hydraulic point of view to ensure the effective protection of a previously identified group of stakes against coastal flooding of marine, fluvial, pluvial, or phreatic origin.
Figure 5 shows schematically how these elements can be positioned in a coastal zone.
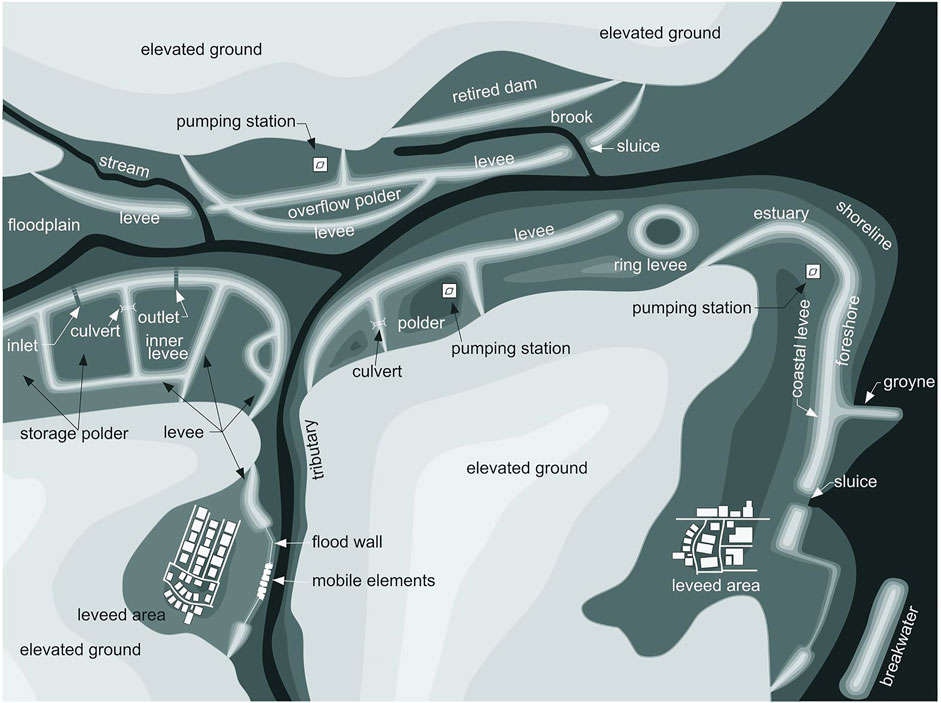
FIGURE 5. Schematic representation of a coastal flood protection system (CIRIA MEDDE USACE, 2013). Credit: Reinhard Pohl, Technische Universität Dresden.
Anthropogenic or natural structures with a recognized protective role generally perform this function only in relation to other structures (e.g., salt marshes, mangroves and other wetlands). Mangroves, salt marshes, and coral reefs occur along about 40–50% of the world’s coastlines (Burke et al., 2011; Giri et al., 2011; McOwen et al., 2017; IPCC, 2019). These ecosystems provide important ecosystemic services including coastal protection by wave attenuation and shoreline stabilisation. A global meta-analysis of 69 studies demonstrated that, on average, these ecosystems together reduced wave heights between 35 and 71% at the delimited locations considered (Narayan et al., 2016), with coral reefs, salt marshes, mangroves, and seagrass/kelp beds reducing wave heights by 54–81%, 62–79%, 25–37% and 25–45% respectively. Moreover, sandy beaches and dunes occur at all latitudes covering approximately 34% of the world’s coastlines (Hardisty, 1994). The protection offered by these natural formations can be considered close to 100% for a robust dune belt in the absence of a major disturbance disrupting the hydrosedimentary cell functioning.
Protection systems are therefore an integrated set of technical and natural elements, which do not provide protection separately but in combination. Moreover, the regular action of managers of natural or anthropogenic structures (as well as other actors involved for example in regulation or financing) is a necessary condition for the proper functioning of these systems. Protection systems can therefore be considered as socio-ecological systems (SES). Such a system is defined by the IPCC (2019) as:
An integrated system that includes human societies and ecosystems, in which humans are part of nature. The functions of such a system arise from the interactions and interdependence of the social and ecological subsystems. The system’s structure is characterised by reciprocal feedbacks, emphasising that humans must be seen as a part of, not apart from, nature (Berkes and Folke, 1998; Arctic Council, 2016).
According to (Millenn. Ecosyst. Assess., 2005), SESs produce a « bundle » of ecosystem services (ES), categorized as provisioning (e.g., freshwater, crops, and meat), regulating (e.g., flood and climate regulation), and cultural services (e.g., recreation and spiritual values).
SESs should be considered as Complex Adaptive Systems (CAS): a system of interconnected components characterised by emergent behavior, self-organisation, adaptation, and substantial uncertainties about system behavior (Biggs et al., 2012).
Building on this critical idea, Bigg et al. (2012) defines resilience as the capacity of an SES to sustain a desired set of ecosystem services in the face of disturbance and ongoing evolution and change.
2.4.2 System Environment
Studying coastal flood protection systems requires reframing them within the context of more comprehensive systems, considering not only the events against which they provide protection, but also changes and disturbances, slow or rapid, localized or diffuse, to which they are exposed, and more generally, all the constraints arising from the natural, the built and the social environment, at the level of the territory or at higher levels (such as the region or the Earth). In summary, these elements can be described as follows.
In the current day and age, the coast is a space which is both particularly exposed to natural phenomena and yet remains attractive to people. It is also subject to the spread of urbanisation (Lichter et al., 2011; Jonkman et al., 2013; Güneralp et al., 2015; Neumann et al., 2015; Muis et al., 2016) in the context of increasing international trade and globalisation. These changes may lead to situations of local concern in terms of fitness for habitation and exposure to natural disasters (Hallegatte et al., 2013; Pycroft et al., 2016; Tiggeloven et al., 2020), in particular engendered by marine submersions.
Marine submersions are caused by storms or hurricanes (Harris, 1963; Graumann, 2006; Heurtefeux et al., 2007; Dietrich et al., 2010; Bertin et al., 2012; Mazas and Kergadallan, 2014; Emanuel, 2017; Emerton et al., 2020) or by earthquakes or other underwater terrain movements causing tsunamis (Margaritondo, 2005; Intergovernmental Oceanographic Commission, 2012; Archana and Twinkle, 2021; Schreurs, 2021). These events have been documented in the history of humanity as the origin of many major disasters, with arguably the deadliest events having been caused by phenomena of geophysical origin (Flather, 2001).
The phenomena at the origin of marine submersions are complex in both their mode of generation (weather-marine phenomena or tsunamis) and in their propagation to the coast, including their last phase of interaction with the coasts. The Figure 6 summarises the hydraulic processes in relation to the morphological evolutions that take place on the time scale of an event or on longer time scales.
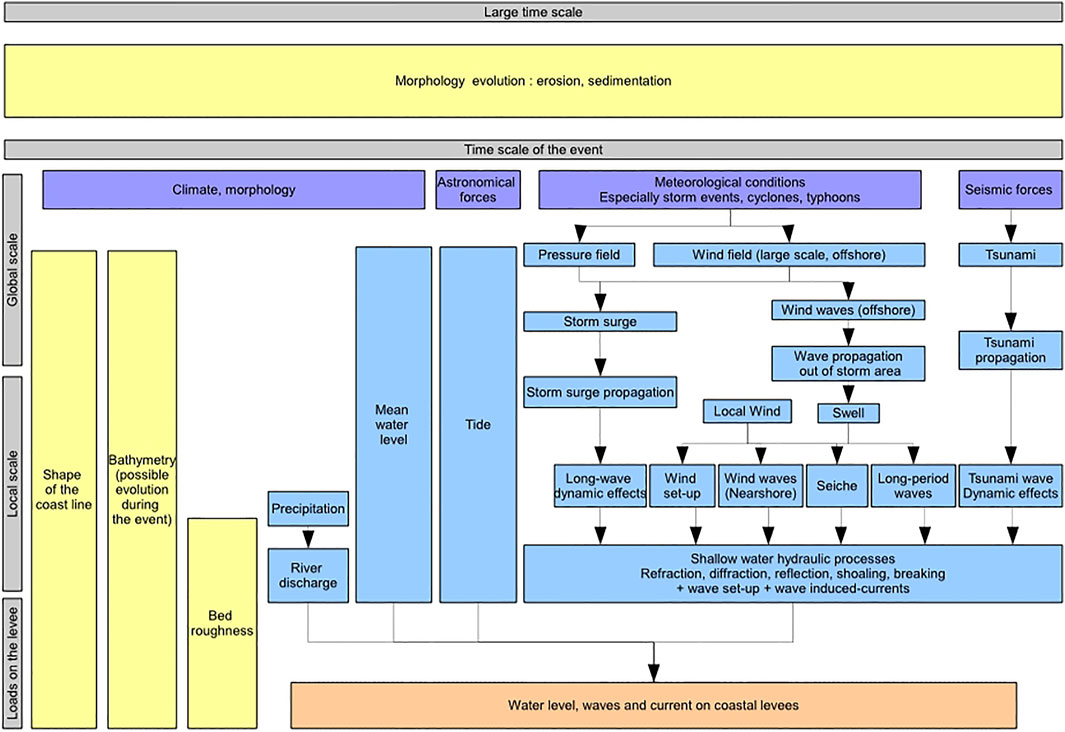
FIGURE 6. The process of hydraulic load generation (CIRIA MEDDE USACE, 2013)—Credit: Marc Igigabel.
The risk of coastal flooding is also generated by other phenomena that occur separately or jointly, such as the overflow of rivers, the accumulation of rainwater, and a rising water table. This situation requires special arrangements to be made in the design of protection systems: drainage of water in vulnerable areas must complement the defences against sea and river waters.
Risks on most coastlines will be greatly aggravated by sea level variations. In particular, rising sea levels, by increasing water depths, promote the propagation of waves to the coast and contribute greatly to the increase in the frequency of extreme events as well as to the weakening of coastlines, especially by erosion. Sea level variations occur for the following reasons, summarized by the IPCC (2019):
• Changes in ocean volume as a result of a change in the mass of water in the ocean (e.g., due to melting of glaciers and ice sheets);
• Changes in ocean volume as a result of changes in ocean water density (e.g., water volume expansion under warmer conditions);
• Changes in the shape of the ocean basins and changes in the Earth’s gravitational and rotational fields; and
• Local subsidence or uplift of the land.
These processes are represented schematically in the Figure 7.
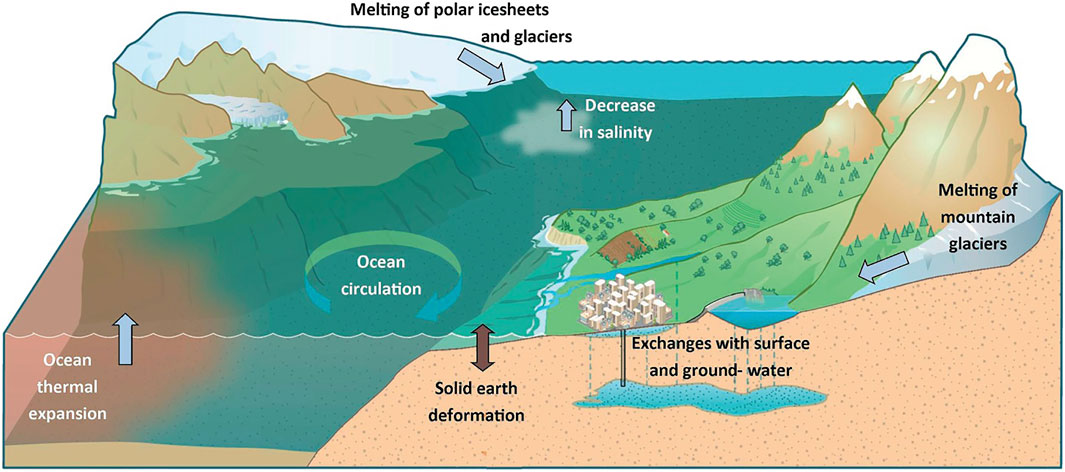
FIGURE 7. Sketch showing the main factors causing sea level changes (Cazenave and Le Cozannet, 2013).
Among the causes of sea level change identified by the IPCC (2019), it is possible to distinguish between changes in the masses or volume of the oceans, and changes of the land with respect to the sea surface. In the first case, a sea level change is defined as « eustatic »; otherwise, it is defined as « relative » (Rovere et al., 2016).
This leads to two essential definitions (IPCC, 2019):
• The Global Mean Sea Level (GMSL) can be defined as an average of the eustatic sea level at a global scale. The following definition can therefore be adopted:
Mean distance between the sea surface and the center of the Earth.
• The Relative Sea Level (RSL) can be defined as the following:
Sea level measured by a tide gauge with respect to the land upon which it is situated.
Climate change effects (described in the IPCC 2007; IPCC, 2012; IPCC, 2014; IPCC, 2019; IPCC, 2021), and in particular the RSL rise, is on most coastlines causing a worsening of coastal risks (not only does this include the risk of coastal flooding, but also the risks of erosion, shoreline retreat, and dune migration). However, at present, attributing impacts to sea level rise remains difficult in most regions (IPCC, 2019), since impacts were exacerbated by human-induced non-climatic drivers, such as land use change, land subsidence caused by groundwater and/or hydrocarbon extraction (Dixon et al., 2006; Nicholls et al., 2008; Erkens et al., 2015; Sarah and Soebowo, 2018; Tessler et al., 2018), disturbance of sedimentary movement in rivers and along coasts (Syvitski and Saito, 2007; Syvitski, 2008; Vinh et al., 2014; Tessler et al., 2015; Welch et al., 2017; Dunn et al., 2018; Ouillon, 2018; Tessler et al., 2018; Thi Ha et al., 2018), coastal erosion (Doody 2013; Pontee, 2013; Luijendijk et al., 2018; Ndour et al., 2018) and coastal habitat degradation (Dahl and Stedman, 2013; Mediterranean Wetlands Observatory, 2014; Murray et al., 2014; Gardner et al., 2015; Kummu et al., 2016; Ramsar, 2016; Li et al., 2018; Sippo et al., 2018).
In the coming decades, the frequency of extreme sea levels will vary significantly across coastlines (Buchanan et al., 2016). In particular, many coastal areas in the lower latitudes may expect amplification factors of 100 or larger by mid-century, regardless of the scenario. By the end of the century and in particular under RCP8.5, such amplification factors are widespread along the global coastlines (Vousdoukas et al., 2018).
Depending on the coastline, the risk of coastal flooding can vary. On a global scale, there are three cases of particular concern (IPCC, 2019):
• Deltas: the long-term sustainability of populated deltas is often more affected by large-scale engineering projects than by sea-level rise. For deltas, accelerated soil compaction associated with petroleum and groundwater mining can exceed natural subsidence rates by an order of magnitude. Consequences include shoreline erosion, threatened mangrove swamps and wetlands, increased salinisation of cultivated land, and hundreds of millions of humans that are put at risk;
• Low-lying islands can be extremely exposed when they experience low variability in their water level (for statistical reasons, they then experience the effects of RSL rise more frequently) and this situation is compounded when the coral reefs or mangroves that protect the coasts suffer from the effects of climate change and local anthropogenic actions;
• Arctic regions whose coastlines are eroding as a result of thawing permafrost and reduced frozen sea surfaces, which promotes the formation and propagation of higher waves to land. These phenomena also naturally lead to an increase in the risk of marine flooding.
2.4.3 Analysis Points of View
In order to favour systemic approaches, it is important to consider that a protection system belongs to a larger system. For this reason, the system of protection will be considered as a component of the territory to which it provides protection, making it possible to pay particular attention to the methods dealing with the relations between this system and other elements of the territory. The bibliographical analysis will therefore cross-examine the points of view of specialists in protection structures and specialists in land use planning.
With regard to land planning, account will be taken of the fact that urban systems contain assets of high value and complex and interdependent infrastructure networks (e.g., power supplies, communications, water, transport, etc.). The infrastructure networks are critical for the continuity of economic activities as well as for the population’s basic living needs. The availability of these resources is also required for fast and effective recovery after flood disasters. Damage to critical infrastructure assets during flooding can result in significant secondary consequences which may be more serious than the direct damage caused by the flood. For example, the destructive power and widespread disruption to infrastructure caused by Sandy in 2012 to the east coast of North America and the Caribbean has led this hurricane to be ranked as one of the costliest storm events for insurers (FloodProBE, 2013).
3 Results
Risk management decision-making requires methods to:
• Understand the risks through a global approach;
• Adapt the governance of protection systems;
• Define strategies for adapting protection systems.
The methods relating to these three objectives will be explored successively in this section, based on the conceptual framework and the principles established above. The emphasis will be more on systemic approaches, with the related analytical approaches being more relevant to the quantification of risk and the implementation of the technical and economic sizing of protection systems. Thus, for adaptation strategies, simplified approaches based mainly on the rise of RSL will be distinguished from global approaches.
To take better account of protection systems in their environment, the bibliographic analysis will focus on the following SESs:
• The territory which is an eminently complex system in terms of the number and diversity of the elements which constitute it (physical, biological, and anthropogenic constituents), and by the interconnection of these elements and their relations with the external environment;
• The coastal flood protection system, which may be regarded as a subsystem of the territory in which it is situated, which certainly has fewer and less diverse elements. However, a high degree of complexity is maintained when ecological and social dimensions are taken into account.
The latter will be considered as a subsystem of the former.
3.1 Methods of Risk Understanding
The study of the state-of-the-art will progress initially by following three axes that can be considered essential in the preparation of a systemic analysis:
• Multiple risk assessment on a coastal territory;
• Defining spatial-temporal analysis frameworks;
• Observing complex systems.
On this basis, investigations will then be carried out using the methods applicable at the territorial level, and then at the level of the protection system. Considering the nature of SESs, guidelines will be sought not only in the physical and technical fields, but also in the social, economic and ecological fields.
3.1.1 Multiple Risk Assessment
At the shoreline, marine floodings are generated by tsunamis (the effects of the earthquake may also be felt at the submersion site) or occurs during the passage of atmospheric depressions, which are often associated with high winds and waves breaking inland of the intertidal zone. If interest is first on the coastal hydraulic phenomena, the hazard may also occur, not only by overflowing and overtopping from the sea, but also through coastal river flooding, runoff, rising water tables … However, the flooding can generate other phenomena: humid ground or powerful currents that may cause mudslides, tree falls, cliff failures, cavity collapses, or erosion impacting building foundations or local infrastructure … The adoption of a systemic approach to risk in the context of observed and projected global changes is required to comprehend, as openly as possible, the conditions for the emergence of risk. In this type of approach, the hazard should no longer be considered in a restricted manner resulting only from hydro-meteorological phenomena, but rather as emerging from a combination of phenomena, potentially of various natures which develop across a range of timescales. The definition of « hazard » in the Hyōgo Framework for Action 2005–2015 (UNISDR, 2015) is therefore more appropriate:
« A potentially damaging physical event, phenomenon or human activity that may cause the loss of life or injury, property damage, social and economic disruption, or environmental degradation. Hazards can include latent conditions that may represent future threats and can have different origins: natural (geological, hydrometeorological and biological) or induced by human processes (environmental degradation and technological hazards) ».
To study the hazard implies first analyzing the different physical components, their possible combinations and the chain of events. In a global approach, Gill and Malamud (2014) present a broad overview of the interaction relationships between 21 natural hazards. The Figure 8 represents a selection of 16 of them, drawn from six hazard groups: geophysical, hydrological, shallow Earth, atmospheric, biophysical, and space hazards. As a necessary complement, Liu et al. (2016) provide a systematic hazard interaction classification based on the geophysical environment that allows for the consideration of all possible interactions (independent, mutual exclusion, parallel and series) between different hazards, and for the calculation of the probability and magnitude of multiple interacting natural hazards occurring together.
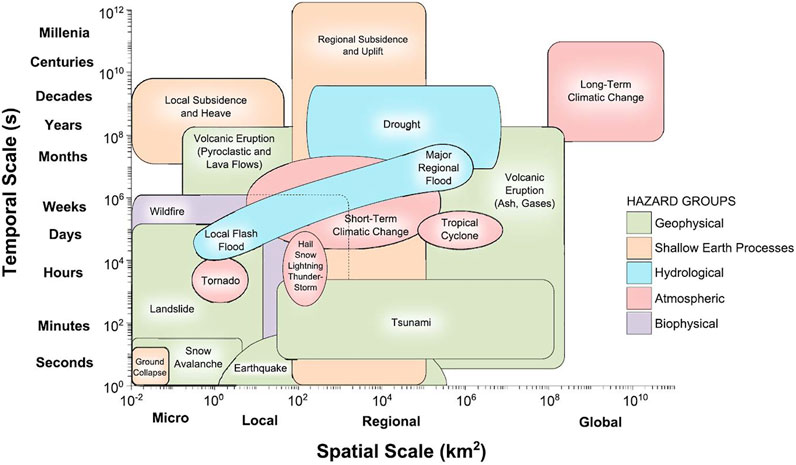
FIGURE 8. Spatial and temporal scales of 16 selected natural hazards. Shown on logarithmic axes are the spatial and temporal scales over which the 16 natural hazards act (Gill and Malamud, 2014).
Increasingly, multi-hazard risk assessments are undertaken at the coast (IPCC, 2019). For example, Kunte et al. (2014) characterised the vulnerabiliy of coastal lands in India to erosion, submersion, and inundation, and demonstrated that the inclusion of socio-economic parameters should be considered in the overall assessment of vulnerability. Van Hattum et al. (2020) also stressed the importance of socio-economic parameters and noted that poverty in particular magnifies the impact of floods as poor people are often highly exposed to floods, and are also more vulnerable and less resilient.
To comprehensively understand risk, Hagenlocher et al. (2018) have come to the conclusion that the definition of adaptation strategies and measures (including ecosystem-based options) requires spatially explicit information on the exposure, vulnerabilities, and risks associated with different combinations of hazards in an integrative manner, not only focusing on societal aspects or ecosystems alone, but also taking into account interactions with other systems, such as neighbouring territories. In particular, ecological dimension should be considered more systematically in risk assessments. Addressing this gap, the authors presented an innovative assessment concept and indicator-based methodology that is sensitive to the specific (multi-)hazard setting in a given territory (Figure 9). These modular sets of vulnerability indicators adapt flexibly to the hazard situation.
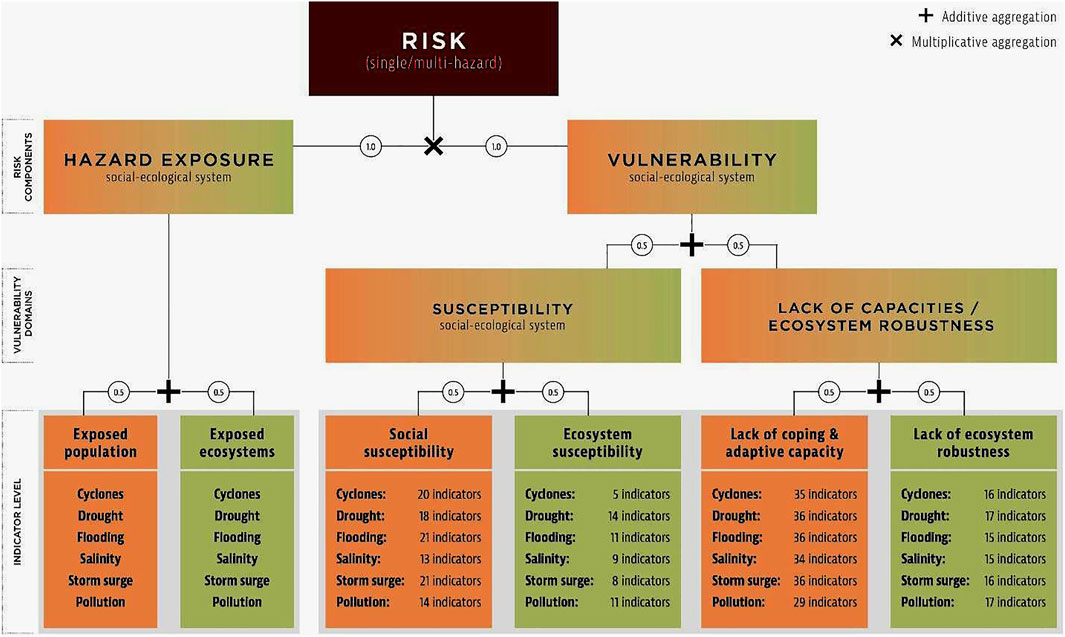
FIGURE 9. The Global Risk Index: concept and structure. Credit: Hagenlocher et al. (2018).
It should be noted, however, that this methodology is based on quantitative indicators and cannot report cascading effects and feedbacks. In addition, it ignores the hazards of biological origin, for example, the likelihood that floods degrade health conditions, in particular, by the deterioration of drinking water and sanitation systems. Analyses which widen the spectrum of investigations to include the emergence of risks should therefore be considered, but these analyses are conditional on their application in a smaller geographical area, allowing better consideration of local specificities. Thus, Cerema (2019) established, for the city of Semarang (Indonesia), a more open model of systemic functioning, with emphasis on the importance of domino effects and feedbacks.
Finally, it should be stressed that the multi-risk approach should not only apply at the level of the territories, but should also apply directly to protective structures since they are sensitive to a wide range of factors: meteorological, hydraulic, and morphological actions, as well as biological and anthropogenic actions. For example, the effects of prolonged periods of drought should be carefully considered, particularly in the context of climate change: levees are vulnerable to drought if the structure or its subsoil contains peat or highly organic clay in a zone/level below which the groundwater table may fall during drought. Dehydration of peat in levees causes shrinkage and a further loss of weight causes reduced stability. This may ultimately result in breaching due to horizontal sliding (CIRIA MEDDE USACE, 2013). An example of failure due to sliding occurred in Wilnis (Supplementary Figure S1), the Netherlands, in 2003. Bottema et al. (2020) described in detail the drought effects and drought management practices for Dutch and English levees.
3.1.2 Definition of Spatial-Temporal Analysis Frameworks
Whether one considers several phenomena in a risk analysis, or after preliminary investigations, one chooses to focus on one of them, it is always necessary:
• Spatially, to limit the territory in which the study is being carried out and within that territory, if necessary, carry out new spatial subdivisions (in particular if analytical approaches are to be considered);
• Temporally, to assess the risks in relation to changes in the situation at various times.
This framing can progress only by iteration with investigations about the context and the phenomena likely to contribute to the manifestation of risk.
Regarding the risk of urban flooding, considering that this hazard cannot be managed in isolation at the city scale, and responses to potential flood impacts are complicated by interlinked political, socio-economic, and environmental changes, Zevenbergen et al. (2008) suggested to develop a framework in which spatio-temporal relations are further defined and investigated, in order to « provide clarity regarding both the feedback loops that cause vulnerability, as well as those that build resilience, and how they interact across differing spatial scales ».
To achieve this objective, cities can be considered as an intermediate-level SES between a higher-level SES (in this example, the watershed) and lower-level SESs, (buildings, infrastructure, and networks). The Figure 10 is a schematic depiction of the propagation of a flood wave through a territory in the case of a failure or overtopping of the protection system. In addition to its particular role in flood risk management, a coastal flood protection system could be considered in this scheme in the same category as buildings and other networks. A protection structure or a natural formation could therefore appear on the figure in place of a building.
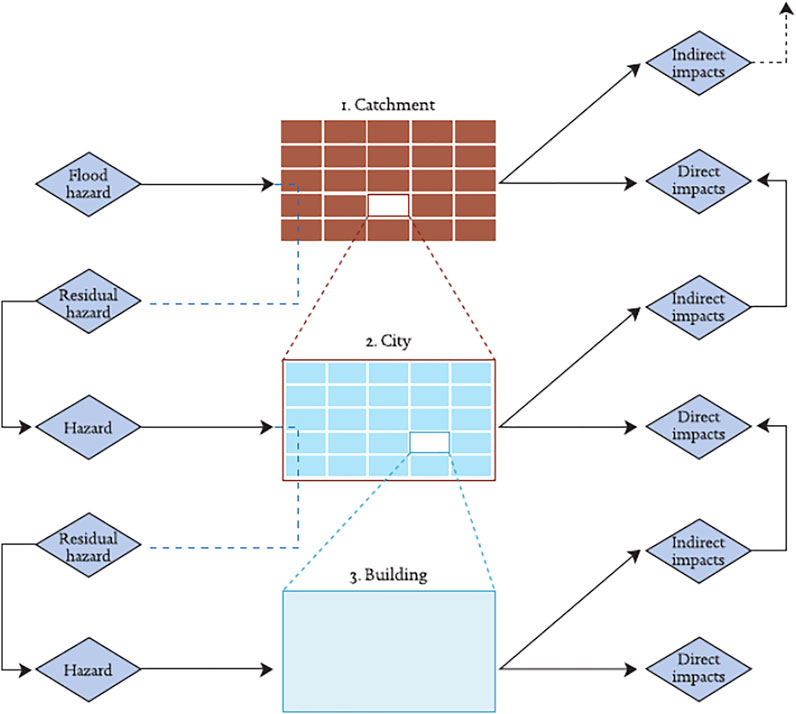
FIGURE 10. Flood resilience: travelling across spatial levels. This picture depicts the propagation of a flood wave through the catchment system in case of a failure or overtopping of the primary flood protection system. (Zevenbergen et al., 2008).
For a « system of protection » SES, additional guidance is provided by research on:
• The assessment of levee performance and the development of geographic information systems for their maintenance (Serre et al., 2009; Michel, 2018);
• The development of a methodology for the analysis of the response of a protection system subject to a marine submersion event (Igigabel, 2016; Igigabel et al., 2022).
3.1.3 Complex Systems Observation
Understanding how phenomena interact with one another, and their significance for people and ecosystems, requires a holistic approach that looks at human and natural dynamics together. The concepts of resilience and SES contribute to provide this holistic view (Arctic Council, 2016).
The key starting point of this process is the integration of different kinds of knowledge and a variety of experiences in which scientists, the public, and decision-makers in policy and practice collaborate to generate not only scientifically reliable, but also context-appropriate, socially robust and actionable knowledge (Weichselgartner and Kasperson, 2010), while always keeping in mind that knowledge integration is necessary but not sufficient: cooperation between actors must continue with resilience-building programmes (Weichselgartner and Kelman, 2014).
Increased interest in resilience management has driven the development of standardized tools for:
• Assessments that focus on deepening understanding of system dynamics; and
• Quantitative measures that aim to capture and quantify resilience in a rigorous and repeatable way. Resilience, however, as a property of CASs, is not easily measured.
Measuring and monitoring a narrow set of indicators or reducing resilience to a single unit of measurement may block the deeper understanding of system dynamics needed to apply resilience thinking and inform management actions (Quinlan et al., 2016). This observation only reflects the aforementioned problem: analytical approaches may prove inappropriate to apprehend complex dynamic systems. Observation of these systems should necessarily involve systemic approaches. To manage the systemic approach of a theme (« risks to a coastal area » is a possible theme, for example) or a territory, an observatory may be a pertinent tool for providing information, but also for disseminating this information. An observatory can be defined as an observation system set up by one or more organisations to monitor the evolution of a phenomenon, an area or a portion of a territory in time and space; most observatories take the form of computer applications in which data are aggregated and presented in the form of tables, maps, or statistical indicators. The objectives, the users, and the temporalities considered by the observatory should be precisely defined (Bourlier et al., 2020).
3.1.4 Risk Assessment at the Level of a Territory
Risk assessment methods at the level of a territory focus on urban environments, where flood protection stakes are most prevalent. The scale of the urban district is especially important because it is at this scale that cities carry out their adaptation, transformation and/or development operations (Serre et al., 2018). These approaches are selected for their overall operational character, as well as for the links established with flood protection systems. However, there are other models or approaches (Barroca et al., 2013; The Rockefeller Foundation, 2015) aimed at the analysis or assessment of urban resilience from a more general point of view.
The methods used to study an urban system consist of a double characterisation of its structural and functional aspects. The structural aspect corresponds to the spatial organisation of the system whereas through functional analysis, it is essential to characterise the temporal phenomena, such as flows, exchanges, and feedback. The understanding of risk at the scale of a territory can therefore be engaged by successively defining the limits of the system, its environment, its components, and finally by associating functions (Serre et al., 2018). In an urban system, this method shows the interdependence of the networks and critical infrastructures of the studied area, and hence, the domino effects and impact chains (Bourlier et al., 2020).
The Figure 11 shows the application of a functional analysis at the neighbourhood level. It indicates contact and normal operational flow relationships between a neighbourhood’s individual components and its external environment factors.
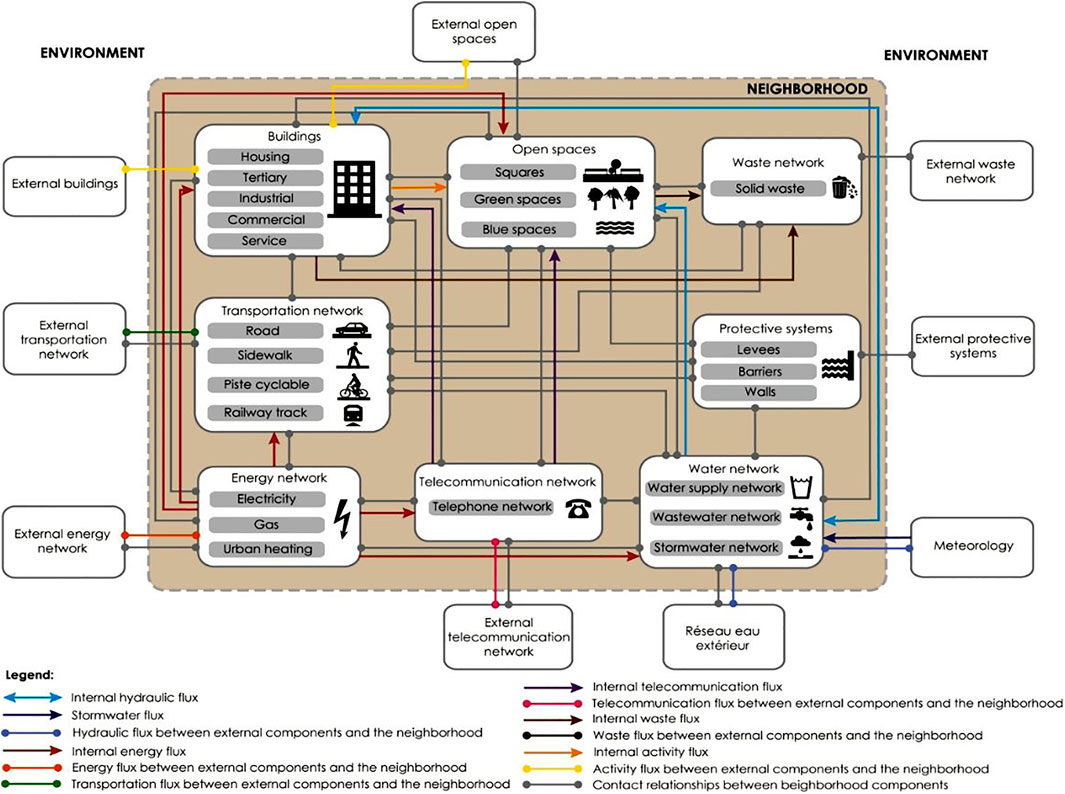
FIGURE 11. Functional diagram of the neighbourhood system (Serre et al., 2018).
The predominance of the systems approach is marked by the focus of the study, which is mainly on the relationships between the elements, and avoids analysing the resilience of a territory through a sectoral approach based on specializations (e.g., flood protection, energy and transport). It should be noted that analytical approaches can be efficient only if the limitations of the system—determining what is internal and what is external—have been well delineated and the internal organisation of the system has been well described. Risk analysis can be continued by studying failure mechanisms for each function (Balsells et al., 2012). In applying these methods, the relations within the territory in question (the neighbourhood) and with the external environment of that territory should be well identified, particularly in regards to the structures performing a protection function. The interdependence plays the role of a risk diffusion factor. And as Lhomme et al. (2013) stated, networks then act as vectors of risk propagation. These analysis principles have been implemented by several authors, for example, by studying the effects of Hurricane Katrina (Heinzlef et al., 2019) and New Orleans’ recovery (Balsells et al., 2012) after this event.
Although for Serre et al. (2018), a neighbourhood is considered a social, economic, and physical/technical system, the above-mentioned authors generally placed more emphasis on the physical/technical dimension. In addition, other authors address:
• The human aspect of the management of the urban system. For example, Zevenbergen et al. (2015) presented a pragmatic screening procedure, referred to as a « Quick Scan methodology ». Its purpose is « to provide guidance for network operators and decision makers on identifying and rating those critical infrastructure networks and hot spot buildings that may be at risk from flooding, and assessing where intervention will be most feasible and cost beneficial—the so-called quick wins »;
• Social and urban aspects in addition to technical aspects. For example, Heinzlef et al. (2019) defined a resilient hierarchical index (Tate, 2012) constructed on the basis of three indicators of urban, social, and technical resilience. Within this framework, urban resilience is defined by the proactive capacities that the urban system must develop in order to (re)act in the face of the disaster, thereby developing learning and anticipation skills.
3.1.5 Risk Assessment at the Level of a Protection Sytem
The multiple natural and anthropogenic actions to which the levees are exposed, as well as the heterogeneity of the structures and their very long length, requires the consideration of significant margins of uncertainty of the structure’s behaviour. The protection provided by levee systems is therefore always limited, and must be considered as such: when natural phenomena exceed a certain intensity, the structures allow water to pass through, either by overflowing or wave overtopping, or through a breach in the event of an accidental rupture. In either case, the risk may increase, in particular by an acceleration of hydraulic phenomena. Since the positive effect of reducing flood frequency is more immediately apparent than its potential negative effects, levee systems often produce a false sense of security (CIRIA MEDDE USACE, 2013).
This has led to an extensive literature on:
• Modelling and assessing the risk of levee failure (e.g., Stanczak and Oumeraci, 2012; Wu and Li, 2017; Zhang et al., 2017). The effect of transitions, whether in the geometric design of the structure or in materials used, should not be overlooked in assessments as shown for example by van Bergeijk et al. (2019) and Simm et al. (2019);
• Estimating flows across embankments exposed to swell under either emerged (e.g., Suzuki et al., 2017) or submerged (e.g., Formentin and Zanuttigh, 2018) conditions. Note that in the latter case, the calculation of the flow through a breach can also be considered.
Chapter 8 of the International Levee Handbook (CIRIA MEDDE USACE, 2013) reviews good engineering practices for structural dimensioning by combining hydraulic and geotechnical approaches. It can also be used to analyse the risk of failure.
Nevertheless, the problem of performance limits and the increase in risk in the case of an event exceeding the nominal operating conditions should be tackled within a broader framework than that of a single structure with only the protection function. On this topic, Marijnissen et al. (2021) assessed the safety of a double-levee system (Supplementary Figure S2).
In the more general case of a system with a front and a rear defense (e.g., a storm surge barrier and levees), the front defense can improve the reliability of the rear defense by reducing the load on this rear defense (Dupuits et al., 2017). Other studies also show the effectiveness of hybrid solutions combining land-based structure (for example a dike) with the effect of a natural formation such as coastal wetlands and mangroves (Vuik et al., 2018; Vuik et al., 2019). Similarly, the construction of brushwood dams is often a relevant solution: at exposed coasts, strong waves and currents may impede the settling of fine sediments and the establishment of salt marsh vegetation or mangroves (Winterwerp et al., 2013). Construction of a system with brushwood dams (Figure 12) creates shelter, facilitates sedimentation, and prevents erosion. Combining these dams with drainage ditches improves consolidation of the settled sediment. This method has successfully been applied for centuries, and has led to artificial salt marshes along 450 km of the Dutch, German, and Danish Wadden Sea coastline (Bakker et al., 2002; Hofstede, 2003).
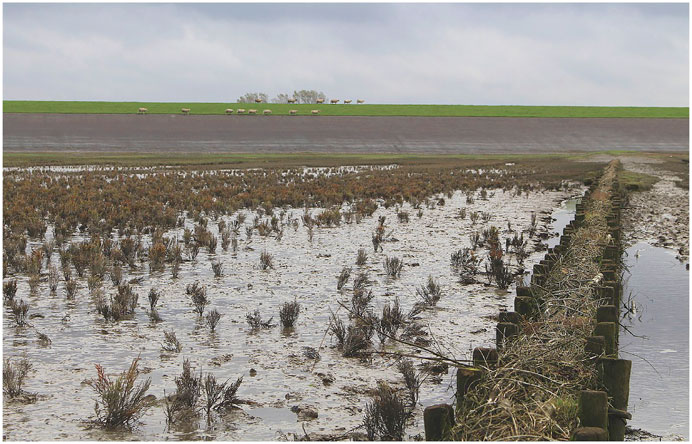
FIGURE 12. Brushwood dams in the Dutch Wadden Sea, which create artificial shelter for salt marsh vegetation from waves and currents. The levee is visible in the background (Photo: V. Vuik).
Risk assessment approaches for protection systems are hybrid in nature. The diversity of natural structures and formations, as well as the diversity of the environments in which these components are placed, require the adoption of systemic approaches. However, the objectives of performance and rationalization of the resources employed, also force the adoption of analytical approaches. In particular, this is done in order to evaluate their reaction to various demands, or to determine quantitatively the human, financial, and material requirements for the management and adaptation of the protection system. Framing analytical approaches through systemic approaches is an ongoing challenge for managers of protection systems.
The structure performance assessment is crucial since this process provides input for the evaluation of the residual risk in a leveed area. In addition, the results of the evaluation of the structures will determine, along with the results of the risk attribution at the scale of the system, the priorities for action: if the risk attributed to a structure is high, then the requirements on its structural state will also be high, which will give it a better ranking in the intervention priorities of the levee manager. The main difficulty therefore lies in evaluating the performance of each component of the system. To meet this challenge, the concept of the fragility curve was explored. In the context of flood risk assessment, a fragility curve represents the probability of breach of the defence given a set of loading conditions and therefore represents (along with the probability of overflowing and wave overtopping) part of the likelihood of water flowing from source to receptor in the SPRC model. This analytical approach can be either deterministic or probabilistic, as shown in the Supplementary Figure S3. In the deterministic design, the assumption is made that the probability of failure is zero until the design load event is reached, at which point the probability switches to 1. In reality, as the load rises above the design condition, the probability of failure rises and only after considerable extra load has been applied does it actually approach 1. Similarly, there is a small but significant probability of failure at conditions below the design loading. This is what probabilistic approaches represent (Simm et al., 2008).
While these modes of representation are interesting for understanding the complexity of levee behaviour, the associated methods are difficult to apply due to the geotechnical heterogeneity, uncertainties about hydraulic loads, —even more so on coastlines exposed to waves—, and the multiple failure modes. In fact, since levees commonly extend over several tens or even hundreds of kilometres, their management can benefit from computer assistance in terms of data organisation, management, and representation (spatial representation and evolution over time), but will always require expertise for their diagnosis. For example, the system designed by Serre et al. (2009) has two components:
• A geographic information system (GIS) application that allows collecting data necessary for managing a levee portfolio;
• A Spatial Decision Support System (SDSS) which uses performance indicators to assist levee managers in prioritizing maintenance activities.
This type of operating mode led Hathout et al. (2019) to question the validity of human judgment. These authors presented approaches of elicitation, calibration and aggregation of expert opinions to evaluate the reliability of river levees. Applying the proposed weighting and aggregation procedure reduces variability in the probability of failure estimate and provides a better probabilistic distribution of aggregated expert opinion than that of individual expert opinions. The results allowed for the identification of a trend of aggregated expert opinions indicating over or lack of confidence.
This review of the state-of-the-art shows that it is dangerous to seek « automation » of risk assessment at the level of a structure and, a fortiori, at the level of a protection system. The methods of evaluation which combine human expertise and the advantages of data management by computer means should indeed be sought. Since each structure is unique, monitoring its behaviour over time is essential, especially during major events through feedback. However, it should be considered in the application of this principle that in a future affected by multiple changes, decisions based solely on past events will no longer necessarily be relevant and that more anticipation will be required in the application of risk analysis methods and also in the definition of adaptation strategies.
3.2 Methods for Defining Adaptation Strategy and Changing System Governance
As a follow-up to the study of methods for understanding risk, this section deals with methods for defining adaptation strategies for territories and their protection systems. Since changes in governance patterns are generally necessary for the effective implementation of adaptation strategies, this issue will also be addressed.
Drafting an adaptation policy requires thorough insight into the precise type and magnitude of future problems, the possibilities to counteract or reduce these in terms of strategic alternatives composed of physical measures and policy instruments. These strategic alternatives must be evaluated on performance, cost and side effects and opportunity criteria (Klijn et al., 2015). As such, scenario planning and the recent concept of Climate Resilient Development Pathways (CRDP) deserve to be highlighted, because they represent methods of governance and management of SESs essential to operationalize the concepts of adaptability and transformability. This section is devoted to the study of these methods and their gouvernance implications.
3.2.1 Scenario Planning and Pathways for Building Resilience to Climate Change
The definition of strategies for the adaptation and a fortiori the transformation of SESs can be based on the establishment of scenarios. Scenario planning is a systemic method for thinking creatively about possible complex and uncertain futures. The central idea of scenario planning is to consider a variety of possible futures that include many of the important uncertainties in the system rather than to focus on the accurate prediction of a single outcome (Peterson et al., 2003). Scenarios can help overcome fundamental problems about transformation by organising thinking in a coherent way. With so many changes happening simultaneously, the very complexity of the situation can become a barrier to understanding and action. The scenarios can organise information about transformation in a comprehensible way that facilitates discussion and action (Walker et al., 2004).
The definition of adaptation strategies largely depends on the dynamic nature of the SESs, which requires considering several time horizons and assessing uncertainties. The study of several scenarios helps to achieve these two objectives. To this end, it is possible to use general data, although transposition across territories is often difficult:
• For climate change scenarios, the use of climate model projections (Moss, 2008; Moss et al., 2010; van Vuuren et al., 2011) is recommended. However, the further down the chain of effects, the larger the uncertainties about the degree of an effect (Haasnoot, 2013), and sometimes even about the direction. Moreover, when climate changes beyond the range historically observed, unforeseen shifts may occur which complicate the downscaling of global climate scenarios to the national or regional level (Klijn et al., 2015);
• For socio-economic development scenarios (O’Neill et al., 2017), Klijn et al. (2015) stated that the autonomous developments in the socio-economic subsystem are even more uncertain than climate change scenarios because of the global scale of economic developments and their dependency on very dynamic geo-political changes. Nevertheless, for demographic, economic, and land-use developments, a scenario approach may be applied.
The use of scenarios may also involve analytical techniques, including:
• The policy tipping points (Kwadijk et al., 2010). A tipping point is reached if the magnitude of change is such that the current management strategy can no longer meet its objectives. Beyond the tipping point, an alternative adaptive strategy is needed. By applying this approach, the following basic questions of decision makers are answered: what are the first issues that we will face as a result of climate change and when can we expect this. In the west of the Netherlands, the results show, for instance, that climate change and the RSL rise are more likely to cause a threat to the fresh water supply than flooding; and
• The adaptation pathways (Haasnoot et al., 2013). This method relates to an analytical approach for exploring and sequencing a set of possible actions based on alternative external developments over time. The authors tested this concept by producing an adaptive plan for long-term water management of the Rhine Delta in the Netherlands that considers the deep uncertainties about the future arising from social, political, technological, economic, and climate changes. The Supplementary Figure S4 provides a representation of this approach.
Addressing the major challenge of climate change adaptation and in order to take into account the Sustainable Development and Poverty Eradication Goals, it is also possible to use the concept of Climate-resilient development pathway (CRDP), represented by the Supplementary Figure S5 (Kainuma, 2018; Roy et al., 2018).
CRDPs are increasingly being explored as an approach for combining scientific assessments, stakeholder participation, and forward-looking development planning, acknowledging that pursuing CRDP is not only a technical challenge of risk management but also a social and political process (Roy et al., 2018). This implies an organisation of the process by the institutions in charge of the governance of the territories. CRDPs aim to establish narratives of hope and opportunity that can extend beyond risk reduction and coping—in particular integrating the fight against climate change (Amundsen, 2018)—and are critical in driving motivation, creative thinking and behavioural changes in response to climate change (Myers et al., 2012; Prescott and Logan, 2018). The definition of the scenarios should be based on general strategies for enhancing social-ecological resilience. These principles, on which CRDPs are based, were the subject of an integrative presentation by Bigg et al. (2012) on the basis of a review of the scientific literature (Supplementary Figure S6).
According to Bigg et al. (2012), the principles of resilience management fall into two levels:
• Principles that relate to generic SES properties to be managed; and
• Principles that relate to key attributes of SESs governance.
The implications for coastal flood protection systems of these principles are presented below. Issues related to the adaptation of governance are presented in this section. Issues related to adaptation strategies will be addressed in the next section.
3.2.2 Adaptation of Gouvernance
A territory subject to coastal flooding should be considered a CAS and the issue of protection against this risk can, as such, be dealt with in conjunction with other issues including freshwater management. In the context of adaptation to the effects of climate change, this is particularly relevant when one of the main threats to coastal territories is the salinisation of freshwater bodies and soils. « Understanding an SES as a CAS » involves a paradigm shift. This paradigm shift in particular implies a change in the mode of governance. The Supplementary Figure S7 shows how the evolution of mental perceptions and representations on this subject of fresh water, through the transition from an analytical to a systemic way of thinking, may result in a change in governance and improved flood resilience. The standard perception of freshwater management leads to inflexible, and often ineffective, command-and-control approaches, whereas accounting for ecological complexity opens avenues to adaptability, which builds better resilience to events such as flooding (Moberg and Galaz, 2005). One such pathway may be to assess the role of wetlands in flood mitigation and to anticipate across several scales (including those of the region and of the territory) increases in capacity that may be required in response to climate change (e.g., changes in precipitation patterns and changes in thermal regulation by glaciers) and land-use changes (e.g., increases in runoff caused by deforestation and urbanization).
The framework established by Bigg et al. (2012) calls for governance that intends to bridge two approaches:
• Adaptive management, which focuses on “learning by doing” as a strategy to deal with uncertainty in CASs; and
• Co-management, which builds on the idea of participatory resource management and community–government linkages.
These two approaches are often grouped under the same term « adaptive co-management » since the research of Ruitenbeek and Cartier (2001). Adaptive co-management combines « the iterative learning dimension of adaptive management and the linkage dimension of collaborative management in which rights and responsibilities are jointly shared » (Armitage et al., 2007).
The participatory nature of adaptive co-management enables sharing and reflecting on experiences, ideas, and values with others, which builds trust and relationships and facilitates social learning as well as collective action (Olsson et al., 2004). Participatory learning processes can help actors learn about each other’s mental models, which builds social capital, in turn supporting institutional change and conflict resolution (Biggs et al., 2011).
On « coastal territory » SESs, the utilisation of an expanded circle of actors can be sought in different ways, for example:
• In the definition of town planning rules, a consultation phase may involve all stakeholders in the decision-making process. Perherin (2017) described in detail how the debate between the actors can be organised around the production of hazard maps carried out as part of the elaboration of coastal risk prevention plans;
• Citizen participation in data collection and analysis can reduce the vulnerability of urban areas to rainfall flooding, as shown by the European FloodCitiSense research programme (McCrory and Veeckman, 2017), which had study sites at Birmingham, Brussels, and Rotterdam;
• In urban flood management strategies, the use of flood risk as a design parameter for spatial planning increases flood risk awareness and improves the development of practical strategies to cope with and communicate the residual risk (Zevenbergen et al., 2008). Following this principle, an applied scientific research project was executed by a learning network nested across multiple scale levels, from the national level to the local planning and street/building level in the city of Dordrecht (Netherlands).
The evolution of modes of governance is a necessary condition for change when a profound transformation is envisaged. This is supported by three studies realised in China (Jiang et al., 2018), in Australia (Hurlimann et al., 2014) and in Italia (Vitale et al., 2020). In the three cases, it appears that the change in governance should also be accompanied by institutional changes, and administrative reorganisation. The governance implications of the adaptive co-management principles should therefore be well understood methodologically. Olsson et al. (2004) showed that the self-organising process of adaptive co-management development, facilitated by rules and incentives of higher levels, has the potential to expand desirable stability domains of a region and make SESs more resistant to change. The European Flood Risk Directive (Directive 2007/60/EC) is in line with this principle by encouraging the participation of local stakeholders in the development of flood risk management plans. Its application has indeed led to an institutional and administrative restructuring of governance, by developing more integrated visions of land use planning by combining the issues of risk prevention and management of aquatic environments. The refocusing of institutions on the geographical perimeters of emerging risk (e.g., river basins) is another notable advance (Cerema, 2020).
While a review of the modes of governance can be initiated by a top-down process as is the case for the implementation of the European directive, which can lead to real progress, this observation should not, however, obscure the fact that all regions of the world do not benefit from a national or supra-national organisation that encourages changes in the modes of governance. To initiate a change in governance, Termeer et al. (2017) proposed a methodological framework to clarify how governance can be improved through bottom-up initiatives and how this change can be initiated. In complement, Cundill and Fabricius (2010) identified system attributes and key variables that could form the basis for monitoring the governance dimension of adaptive co-management.
3.3 Adaptation Methods (Simplified Approach Based on RSL Rise)
The increase in RSL triggers multiple adverse effects (Supplementary Figure S8) and often appears, rightly or wrongly, as the most important factor in the appearance of risk. Moreover, the monitoring of a single parameter has a practical advantage for the establishment and implementation of a strategy. For these two reasons, the adaptation options in response to this factor alone have a high visibility in the scientific literature.
The various types of response are addressed below, both technically and economically.
3.3.1 Different Types of Responses
Building on previous research (Wong, 2014), the IPCC report (2019) describes, in addition to the option of no response, five main modes of adaptation to mean and extreme sea level rise: advance, protect, retreat, accommodate, and ecosystem-based adaptation. These options are shown in the Supplementary Figure S9.
The IPCC report (2019) in Chapter 4 provides a comprehensive analysis for each of these options based on six criteria: observed responses, projected responses, cost of responses, effectiveness of responses, co-benefits and drawbacks of responses, governance challenges (or barriers), and economic efficiency.
Nevertheless, the typology of adaptation options with reference to sea level rise is based on strong implicit assumptions that are not justified in many cases. Thus, the options described are only applicable if the following assumptions are, either completely or partially, verified:
• The coastal strip must have a significant slope, and therefore the possibility of reducing the exposure of the stakes by providing a zone for retreat at a short distance from the coast. The reality of territories is often quite different: the deltas are very flat areas (Syvitski and Saito, 2007) and many small islands do not have an area allowing for retreat;
• The territory must have a topography maintained at an altimeter level sufficiently high over time. In many territories (such as deltas, small islands, and arctic territories), the observed phenomena and long-term projections indicate that this topography is likely to vary under the effects of subsidence or erosion of coastal features;
• The territory must have sufficient environmental stability. Most territories experience major disturbances in their natural environment [e.g., acidification, rising ocean temperatures (Gattuso et al., 2018), and the degradation of wetlands] and the built environment (particularly as a result of population growth). The simplified approaches consider the issues separately and propose adaptation solutions for each of them. However, these issues belong to a territory, i.e., an SES that should be considered as a CAS, and solutions should be defined at the level of the system in order to take into account the interrelationships between its components.
The main interest of the approach focused on RSL rise is to provide spatio-temporal benchmarks in the definition of protection strategies. This parameter is also used in global studies to identify, under several scenarios, the exposed territories, the associated demographics, and economic issues in order to determine the benefits that could be expected from effective protection. Nevertheless, the results of these studies appear to be fragile when it comes to assessing the real possibilities for adaptation of territories and their protection systems. This fragility naturally increases over longer-term projections (Hallegatte et al., 2013; Tiggeloven et al., 2020).
3.3.2 Economic Evaluation of the Different Types of Responses
As for the technical aspects, it is important to avoid approaching coastal flood protection from an economic perspective with overly simplified approaches. As coastal flood protection systems consist of a set of structures such as levees (sometimes layered in several rows), breakwaters, seawalls, which are possibly supplemented by storm barriers and natural features, the economic assessment should be based on:
• The length of the structures and not the length of the coastline. Where possible, consideration should also be given to the height of the structures as proposed by Lenk et al. (2017) on coastal dikes;
• The search for protection structure combinations, with the aim of optimising costs. In particular, coupling natural features with artificial structures, which in turn strengthen the natural features, is a good way to limit long-term spending. Ideally the economic study should cover an even wider spectrum, encompassing the natural environment, the protection system, and the built environment. This was proposed by Aerts (2018).
The studies of Lenk et al. (2017) and Aerts (2018) provided interesting theoretical contributions to cost assessment. However, the results obtained remain fragmented, and the uncertainties on unit costs are far too high for a construction manager to utilise for cost estimates for a particular project (a rapid estimate on the basis of the unit costs appearing in the current public work contracts would be more accurate). The main reason is that the initial data used for these studies comes from bibliographic reviews, with data of multiple international origins. In reality, estimates can only be made on the basis of costs observed currently in the given context (which therefore presupposes the availability of recent national or regional studies), because the availability of resources and the general economic context are decisive.
The international state-of-the-art on this issue should therefore progress, not by seeking cost estimates, but by supplementing and refining the methodology for observing costs on a territory.
3.3.3 Conclusion on Simplified Approaches
In conclusion, decisions on a territory cannot be made by considering the various issues separately and by seeking solutions of only modifying the coastline, as might be suggested by the simplified schemes presenting adaptation options. On the contrary, the issues should be considered globally and should take into account the physical, human, and biological dynamics of the territory envisaged in its three spatial dimensions. The use of methods that make it possible to understand these developments is therefore necessary to give meaning to adaptation decision-making. Ultimately, if simplified approaches are not devoid of interest, they are analytical approaches that should be supplemented with a comprehensive systemic approach.
3.4 Adaptation Methods (Comprehensive Approach)
To avoid maladaptation risks, adaptation strategies should be integrated into a comprehensive scheme, as represented on the Supplementary Figure S10. This scheme comprises:
• Mitigation, which refers to human actions to limit climate change by reducing emissions and enhancing the sinks of greenhouse gases;
• Adaptation, which refers to processes of adjustment by natural or human systems to actual or expected climate and its effects, intended to moderate harm or exploit beneficial opportunities.
Synergies can be developed between climate action, risk prevention, and sustainability (Hackmann et al., 2014). It is therefore important to identify these synergies when they relate to coastal flood protection systems.
In general, the principles of traditional development using civil engineering for the construction of levees and drainage systems lead inevitably to a degradation of the ESs of protection, and therefore to an increase in risk following processes that correspond to:
• Increased dependence on technologies such as levees (Wong, 2014; Welch et al., 2017), pumping and drainage systems (Bloetscher et al., 2011; Aerts, 2018), and storm barriers in estuaries (Burdick and Roman, 2012). In the future, it will be necessary to develop ecosystem-based solutions, including in urban context where the term for these solutions is « green infrastructure » (Culwick and Bobbins, 2016);
• Unsustainable resource management. Fresh water, sand, armourstone, and wood are multiple-use resources. Clarification is needed on the status of these resources (Renaud et al., 2013; Day, 2016; Brakenridge et al., 2017) and the uses (Peduzzi, 2014; Torres et al., 2017) to which they should be allocated. It would seem more relevant in the context of climate change and RSL rise to direct these resources preferentially towards the protection of threatened territories than towards the construction of new buildings on exposed sectors.
Ultimately, as the protection system is nested in the territory, proactive actions are required at the level of the community. These actions fall into three categories:
• The preservation or restoration of ecosystems that play a protective role and their support in the face of the effects of climate change. However, in the long term, such adaptation does assume that the climate can be stabilised. Under changing climatic conditions, there are limits to the effectiveness of ecosystem-based adaptation, and these limits are currently difficult to determine (IPCC, 2019);
• The reduction of hazard exposure by controlling urbanization: water management primarily involves the management of space and in particular, the liberation of low-lying areas where water could be oriented without negative consequences (Angel et al., 2005; Zevenbergen et al., 2008);
• The reduction of the vulnerability of infrastructure (Zhu et al., 2010; Mikami et al., 2015; Hatzikyriakou and Lin, 2018; Duy et al., 2019; Singh et al., 2021), buildings (Zhu et al., 2010; Scussolini et al., 2017), and other assets: on this point, it is more of a question of coordinating actions over time.
The search for nature-based solutions should also be encouraged in flood protection (Culwick and Bobbins, 2016; Alves et al., 2020; United States Environmental Protection Agency US EPA, 2020). The other ESs to which these solutions are likely to contribute to (in particular water purification, climate regulation, and reduction of air pollution) can thus be integrated into the review (Gersonius, 2016; van der Nat et al., 2016; McPhillips et al., 2020).
Other accommodation measures exist, including protection structures. Nevertheless, these measures should be considered as temporary measures, given the increase in hazard: economic challenges to hard protection increase with higher sea levels and will make adaptation unaffordable before technical limits are reached (IPCC, 2019). Therefore, investing in levee systems not knowing the extent to which such systems can protect against future events is only acceptable if it does not impede upon the implementation of other actions contributing to risk protection with more long-term assurance. In this sense, short-term benefits should be sought, only if the cost of the transitional measures is low enough, so that it does not penalize investment in longer-term strategies.
Adaptation strategies can be defined at the level of a country, city and neighbourhood, as shown by the following examples:
• In the Netherlands, on the deltas of the Rhine, the Meuse and the Scheldt, an integrated strategy for multiple issues (including flood protection, water resource management, and sanitation), defined at the national level, is implemented locally (Klijn et al., 2015; Bloemen al., 2018);
• In Dublin, Ireland, the development of the city’s resilience to floods is undertaken on the basis of a diagnosis considering the interrelationships of technical networks and the impact of their malfunctions on vital services (Lhomme et al., 2021);
• In a neighbourhood in Beira, Mozambique, an action programme integrates civil engineering operations, nature-based solutions, and warning and evacuation measures (Van Berchum et al., 2020).
Where the increase in risk is significant, restructuring and urban transformation operations may be necessary. In this case, it is not a question of adapting the existing system, but of rebuilding a new system. As the strategies can be very different in rural and urban contexts, it is necessary to give at least two examples:
• New Orleans, U.S.A. This example shows the significant transformation challenge for coastal megacities facing subsidence, disturbance of sedimentary fluxes, and the effects of climate change (Constanza et al., 2006; Fischetti, 2015);
• The Mekong Delta, Vietnam. This territory represents an example of transformation in the rural context which is characterised by an evolution of economic activities. The smaller spatial constraints in the rural environment allow significant processing capacities (Ha et al., 2012; Renaud et al., 2015; Kruse et al., 2020).
Unfortunately, in practice, the process of transformation is generally undertaken under the constraint of difficulties which may arise on a daily basis (for example, due to a large subsidence or the rise of the RSL leading to a marine transgression), or during a major meteo-oceanic event. In response to the latter case, the Build Back Better initiative (Clinton, 2006) has been promoted in post-disaster recovery to encourage communities and stakeholders to rebuild beyond the pre-disaster state for safer, more sustainable and resilient communities.
4 Discussion
The literature review leads to general conclusions on the concept of a coastal flood protection system as well as specific conclusions on approaches to risk understanding and adaptation strategies. In each case, certain shortcomings have appeared in the state-of-the-art, which makes it possible to identify new avenues of research.
4.1 Conclusions on the Protection System Concept
The bibliographical analysis was carried out by considering a system of protection against coastal flooding as a socio-ecological system, a complex adaptive system, integrating components of the natural environment, of the built environment, and the social and institutional environment. If this hypothesis has made it possible to define the scope of the analysis and to define its structure, it is clear that the methods identified address the various aspects (ecological, technical, social and economic) of the various components of the environment (natural, constructed, social and institutional), but without attaching them to this central concept of socio-ecological system. In order to seek unity and coherence, the concept of a system of protection as an SES should therefore be described precisely, and in this way the relations between these systems and the territories in which they are situated should be formally deciphered.
As a follow-up to this conceptual work, methods for defining and characterizing existing protection systems should be further developed. Work has already been completed as part of marine submersion experience feedbacks (Igigabel, 2016; Igigabel et al., 2022). This work should be continued to consider multiple hazards, including several types of events. These methodologies should in particular specify the links between the protection structures and the other structural elements of the territory (both the stakes to be protected and the infrastructure or natural), while offering operational tools for the development of supply chains for strategic material resources [e.g., sand and armourstone (Le Turdu et al., 2016)].
In a more general way, the methodologies, together with governance and expertise, should foster the integration of strategies for adapting protection systems into land use planning, resource management, and environmental conservation policies. Improving the overall efficiency and effectiveness of the system could be achieved through the coordinated development of information management systems, methodologies, and operational tools.
In relation to these general objectives, two types of approaches should be the subject of new methodological developments: ecosystem approaches and socio-economic approaches.
4.2 Development of Ecosystem Approaches
Ecosystem-based analysis should be conducted on SESs consistent with the multiple themes that an adaptation project must address:
• The watershed is a territory relevant for the consideration of global and local changes, especially when they affect the management of water, sediments, and other materials that may be utilised for protection against coastal flooding (for example, armourstone, and wood);
• The territory in which the protection system is established makes it possible to consider the changes in the natural environment and the built environment and to plan for adaptations of the protection systems in a coordinated manner with these changes.
Concerning the coastal flood protection ES, it should be noted that the rate of soil sealing, the RSL, sedimentary transit, and other processes influencing erosion phenomena (sea states, including wave climate and marine currents) are slow variables whose monitoring is necessary to understand the system evolution and the associated risks. Thus, one important response for preparing for RSL rise is to improve observational systems (including tide gauges, wave buoys, and remote sensing techniques), because in many places around the world, current frequencies and intensities of ESL events are not well understood due to a lack of observational data (IPCC, 2019).
4.3 Development of Socio-Economic Approaches
The bibliographic review shows that:
• In urban contexts, adaptation strategies will certainly have greater financial resources, but they will also be subject to greater constraints, the rigidity imposed by the urban fabric, and the conflicts of interest which can arise in response to a change in the levels of protection or the appropriation of the areas necessary for the adaptation of the system. Stable institutions with strong prerogatives will be necessary to carry out these projects with sufficient anticipation and involvment of the community according to the principles of adaptive co-management. Given the scale of the challenge, additional methodological developments should certainly be sought on this subject;
• Relevant forms of adaptation can only be achieved through adequate knowledge of the system in place including all of its anthropogenic and natural components. The comparison of several options for adaptation at various time horizons can be carried out by economic evaluations. However, the cost of adapting and maintaining the structures can only be estimated in the regional economic context. Therefore, it is more important to look for methodological frameworks that can be applied in different contexts on the basis of local cost observation, than to seek unit costs at the international level, which cannot be applied universally (Igigabel and Yates, 2018).
5 Conclusion
The emergence of the concept of resilience, by opening up a range of additional modes of analysis provides an opportunity to redefine a more relevant methodological framework in a period marked by the effects of climate change and other environmental disturbances.
While definitions of this concept may vary depending on the types of systems, resilience development in all cases involves adopting systemic approaches that contrast with analytical approaches that founded most protection strategies in the 20th century. From the study of the methods, it appears in particular that the observation and analysis of SESs should be carried out first as part of a systemic approach considering the possibility of multiple risks. After this global analysis, which integrates the multiple issues of the territory and is inclusive with regard to its actors, analytical approaches can be led, dedicated to specific points relating to quantitative evaluations and dimensioning.
Understanding the risks in a territory provides a basis on which adaptation scenarios can be built. Analytical techniques exist to decide over time between the different options offered by these scenarios. Furthermore, the idea that improving resilience increases the chances of sustainable development in a changing and uncertain environment is reflected in the relatively recent concept of the CRDP. With this perspective, risk management should not be seen as a constraint only, but as an opportunity for sustainable development. Regardless of the projection technique chosen, the SESs should be considered as CASs which should lead to the emphasis on the substantial uncertainties surrounding these systems, and therefore, the need to continually learn and experiment, and adaptively manage uncertainty, disturbance, and surprise rather than attempt to eliminate them. This principle, coupled with that of participatory management of resources and links between communities and governments, forms the basis of a recommended mode of governance, which includes engaging transformational forms of adaptation: adaptive co-management.
Using these analytical techniques, adaptation or transformation strategies can be considered using two joint approaches:
• Considering territories and their coastal flood protection systems as CASs, in relation to the observed and projected responses of the natural and built environment;
• Considering the RSL rise as a major parameter in the evolution of coastal hazards, including coastal flooding or salinisation of inland waters and soils.
In practice, these two approaches should be coordinated. In all cases, assessing the real possibilities of adaptation at various times requires considering all the components of protection (the natural environment, the built environment, and the levee system), and seeking the optimization of performance coupled to the reduction of expenditures over the long term. The definition of these strategies should be integrated into a territorial project in order to avoid forms of maladaptation which invariably result in environmental degradation and higher risks due to processes of increasing dependence on technologies and inappropriate resource management. Only long-term planning, consisting of a redevelopment of territories to preserve or restore the protection ESs, and limiting exposure to hazards will make it possible to gradually reduce dependence on containment technologies and to progress in implementing the principles of adaptive co-management. Whatever the SES considered, the existence of stable institutions capable of carrying out and financing projects over their entire duration is an essential condition for the effective implementation of the strategies.
Data Availability Statement
The original contributions presented in the study are included in the article/Supplementary Material, further inquiries can be directed to the corresponding author.
Author Contributions
This article is a synthesis of a bibliographic analysis by MI, under the direction of YD and MY. All authors listed have made a substantial, direct, and intellectual contribution to the work and approved it for publication.
Conflict of Interest
The authors declare that the research was conducted in the absence of any commercial or financial relationships that could be construed as a potential conflict of interest.
Publisher’s Note
All claims expressed in this article are solely those of the authors and do not necessarily represent those of their affiliated organizations, or those of the publisher, the editors and the reviewers. Any product that may be evaluated in this article, or claim that may be made by its manufacturer, is not guaranteed or endorsed by the publisher.
Supplementary Material
The Supplementary Material for this article can be found online at: https://www.frontiersin.org/articles/10.3389/feart.2021.756936/full#supplementary-material
Supplementary Figure S1 | A breach in the Wilnis levee due to drought. Credit: STOWA.
Supplementary Figure S2 | Double dike system (Marijnissen et al., 2021)
Supplementary Figure S3 | The true fragility curve compared with the deterministic design (Sayers and Meadowcroft, 2005).
Supplementary Figure S4 | An example of an adaptation pathways map (left) and a scorecard presenting the costs and benefits of the nine possible pathways presented in the map (right). The colors in the scorecard refer to the actions A (red), B (orange), C (green), and D (blue) (Haasnoot et al., 2013).
Supplementary Figure S5 | | Climate-resilient development pathway. From Hock, R., G. Rasul, C. Adler, B. Cáceres, S. Gruber, Y. Hirabayashi, M. Jackson, A. Kääb, S. Kang, S. Kutuzov, Al. Milner, U. Molau, S. Morin, B. Orlove, and H. Steltzer. In: IPCC, 2019, Figure CB2.2.
Supplementary Figure S6 | The seven principles for managing the resilience of an SES (Bigg et al., 2012).
Supplementary Figure S7 | A comparison of analytical thinking and systemic thinking (Moberg and Galaz, 2005). Illustration by Henrik Ernstson.
Supplementary Figure S8 | Overview of the main cascading effects of sea level rise. From Oppenheimer, M., B. C. Glavovic, J. Hinkel, R. van de Wal, A. K. Magnan, A. Abd-Elgawad, R. Cai, M. Cifuentes-Jara, R. M. DeConto, T. Ghosh, J. Hay, F. Isla, B. Marzeion, B. Meyssignac, and Z. Sebesvari. In: IPCC, 2019: Sea Level Rise and Implications for Low-Lying Islands, Coasts and Communities, Figure 4.13.
Supplementary Figure S9 | Different types of responses to coastal risks and sea level rise. From Oppenheimer, M., B. C. Glavovic, J. Hinkel, R. van de Wal, A.K. Magnan, A. Abd-Elgawad, R. Cai, M. Cifuentes-Jara, R.M. DeConto, T. Ghosh, J. Hay, F. Isla, B. Marzeion, B. Meyssignac, and Z. Sebesvari. In: IPCC, 2019: Sea Level Rise and Implications for Low-Lying Islands, Coasts and Communities, Box 4.3, Figure 1.
Supplementary Figure S10 | Overview of the main mitigation and adaptation measures (from Abram, N., J.-P. Gattuso, A. Prakash, L. Cheng, M.P. Chidichimo, S. Crate, H. Enomoto, M. Garschagen, N. Gruber, S. Harper, E. Holland, R.M. Kudela, J. Rice, K. Steffen, and K. von Schuckmann. In: IPCC, 2019, Framing and Context of the Report, Figure 1.2).
References
Aerts, J. (2018). A Review of Cost Estimates for Flood Adaptation. Water 10 (11), 1646. doi:10.3390/w10111646
Alves, A., Vojinovic, Z., Kapelan, Z., Sanchez, A., and Gersonius, B. (2020). Exploring Trade-Offs Among the Multiple Benefits of green-blue-grey Infrastructure for Urban Flood Mitigation. Sci. Total Environ. 703, 134980. doi:10.1016/j.scitotenv.2019.134980
Amundsen, H., Hovelsrud, G. K., Aall, C., Karlsson, M., and Westskog, H. (2018). Local Governments as Drivers for Societal Transformation: towards the 1.5 °C Ambition. Curr. Opin. Environ. Sustainability 31, 23–29. doi:10.1016/j.cosust.2017.12.004
Angel, S., Sheppard, S. C., and Civco, D. L. (2005). The Dynamics of Urban Expansion. Washington, DC: Transport and Urban development departmentThe World Bank.
Archana, C. V., and Twinkle, R. S. (2021). Mathematical Modeling of Tsunami Wave Propagation at Mid Ocean and its Amplification and Run-Up on Shore. J. Ocean Eng. Sci. doi:10.1016/j.joes.2021.03.003
Arctic Council, (2016). Arctic Resilience Report. Editors M. Carson, and G. Peterson (Stockholm: Stockholm Environment Institute and Stockholm Resilience Centre. Available at: www.arctic-council.org/arr (Accessed 0604 2021).
Bakker, J. P., Esselink, P., Dijkema, K. S., Van Duin, W. E., and De Jong, D. J. (2002). Restoration of Salt Marshes in The Netherlands. Hydrobiologia 478, 29–51. doi:10.1023/a:1021066311728
Balsells, M., Becue, V., Barroca, B., Diab, Y., and Serre, D. (2012). “Flood Resilience Assessment of New Orleans Neighborhood over Time,” in Resilience and Urban Risk Management. Editor D. Serre (London: Taylor & Francis Group), 143–150.
Barnett, J., Evans, L. S., Gross, C., Kiem, A. S., Kingsford, R. T., Palutikof, J. P., et al. (2015). From Barriers to Limits to Climate Change Adaptation: Path Dependency and the Speed of Change. E&S 20 (3), 5. doi:10.5751/ES-07698-200305
Barroca, B., DiNardo, M., and Mboumoua, I. (2013). De la vulnérabilité à la résilience : mutation ou bouleversement. echogeo (24), 0–18. doi:10.4000/echogeo.13439
Berkes, F., and Folke, C. (1998). Linking Sociological and Ecological Systems: Management Practices and Social Mechanisms for Building Resilience. New York, USA: Cambridge University Press. Available at: https://searchworks.stanford.edu/view/3834100.
Bertin, X., Bruneau, N., Breilh, J.-F., Fortunato, A. B., and Karpytchev, M. (2012). Importance of Wave Age and Resonance in Storm Surges: The Case Xynthia, Bay of Biscay. Ocean Model. 4 16–30, doi:10.1016/j.ocemod.2011.11.001
Biggs, D., Abel, N., Knight, A. T., Leitch, A., Langston, A., and Ban, N. C. (2011). The Implementation Crisis in Conservation Planning: Could "mental Models" Help. Conservation Lett. 4 (3), 169–183. doi:10.1111/j.1755-263X.2011.00170.x
Biggs, R., Schlüter, M., Biggs, D., Bohensky, E. L., BurnSilver, S., Cundill, G., et al. (2012). Toward Principles for Enhancing the Resilience of Ecosystem Services. Annu. Rev. Environ. Resour. 37 (1), 421–448. doi:10.1146/annurev-environ-051211-123836
Bloemen, P., Reeder, T., Zevenbergen, C., Rijke, J., and Kingsborough, A. (2018). Lessons Learned from Applying Adaptation Pathways in Flood Risk Management and Challenges for the Further Development of This Approach. Mitig Adapt Strateg. Glob. Change 23 (7), 1083–1108. doi:10.1007/s11027-017-9773-9
Bloetscher, F., Heimlich, B., and Meeroff, D. E. (2011). Development of an Adaptation Toolbox to Protect Southeast Florida Water Supplies from Climate Change. Environ. Rev. 19, 397–417. doi:10.1139/a11-011
Bottema, M., Gunn, N., Britt van Haastrecht, , Vonk, Bart., and Henk van Hemert, (2020). “Managing Drought Effects on Levees in The Netherlands and England,” in Proceedings of FloodRisk2020 conference.
Bourlier, B., Taillandier, F., Heinzlef, C., Curt, C., Davies, N., and Serre, D. (2020). “A Flood Resilience Observatory in French Polynesia: Results and Research Perspectives From the ILOTS Project,” in In Proceedings of the FLOODrisk 2020‐4th European Conference on Flood Risk Management, Online, June, 22–24 2021.. Proc. FloodRisk2020 Conf.
Brakenridge, G. R., Syvitski, J. P. M., Niebuhr, E., Overeem, I., Higgins, S. A., Kettner, A. J., et al. (2017). Design with Nature: Causation and Avoidance of Catastrophic Flooding, Myanmar. Earth-Science Rev. 165, 81–109. doi:10.1016/j.earscirev.2016.12.009
Buchanan, M. K., Kopp, R. E., Oppenheimer, M., and Tebaldi, C. (2016). Allowances for Evolving Coastal Flood Risk under Uncertain Local Sea-Level Rise. Climatic Change 137 (3–4), 347–362. doi:10.1007/s10584-016-1664-7
Burke, L., Reytar, K., Spalding, M., and Perry, A. (2011). Reefs at Risk Revisited. Washington, DC: World Resources Institute, 130.
Campbell, A., Kapos, V., Scharlemann, J. P. W., Bubb, P., Chenery, A., Coad, L., et al. (2009). Review of the Literature on the Links between Biodiversity and Climate Change: Impacts, Adaptation and Mitigation. Secretariat of the Convention on Biological Diversity CBD, Montreal. 42, 124
Cassel, M. a., and Hinsberger, M. (2017). Flood Partnerships: a Participatory Approach to Develop and Implement the Flood Risk Management Plans. J. Flood Risk Manage. 10 (2), 164–172. doi:10.1111/jfr3.12086
Cassel-Gintz, M. (2001). GIS-gestützte analyse globaler muster anthropogener Waldschädigung: eine sektorale anwendung des Syndromkonzepts. PIK-Report no. 71; Potsdam Institute for Climate Impact Research. Available at: http://www.pik-potsdam.de/research/publications/pikreports/summary-report-no-71 (accessed June 28, 2021).
Cazenave, A., and Cozannet, G. L. (2014). Sea Level Rise and its Coastal Impacts. Earth's Future 2, 15–34. doi:10.1002/2013EF000188
Cerema, (2020). Synthèse et principales leçons de l’appel à partenaires GEMAPI. Collection : Connaissances.
Cerema, (2019). The Resilience of the Indonesian Coastline to Natural Hazards. Semarang and Subsidence. Collection : connaissances.
Clinton, W. J. (2006). Key Propositions for Building Back Better: Lessons Learned from Tsunami Recovery (Office of the Secretary-General’s Special Envoy for Tsunami Recovery: 2006. New York: United Nations.
Constanza, R., Mitsch, W. J., and Day, J. W. (2006). A New Vision for New Orleans and the Mississippi Delta: Applying Ecological Economics and Ecological Engineering. Front. Ecol. Environ. 4, 465–472.
Culwick, C., and Bobbins, K. (2016). A Framework for a Green Infrastructure Planning Approach in the Gauteng City–RegionGCRO Research Report No. 04, Gauteng City–Region Observatory (GRCO. Johannesburg, South Africa, 127.
Cundill, G., and Fabricius, C. (2010). Monitoring the Governance Dimension of Natural Resource Co-management. Ecol. Soc. 15 (1), 15. doi:10.5751/es-03346-150115
D. Armitage, F. Berkes, and N. Doubleday (Editors) (2007). Adaptive Co-management: Collaboration, Learning, and Multi-Level Governance (Vancouver, Can: Univ. B.C. Press).
Dahl, T. E., and Stedman, S. M. (2013). Status and Trends of Wetlands in the Coastal Watersheds of the Conterminous United States 2004 to 2009Fish and Wildlife Service & National Oceanic and Atmospheric Administration, Na Tional Marine Fisheries Service. U.S. Department of the Interior. Retrieved from: https://www.fws.gov/wetlands/Documents/Status-and-Trends-of-Wetlands-In-the-Coastal-Watersheds-of-the-Conterminous-US-2004-to-2009.pdf (Accessed on January 2, 2022).
Daniel, P., Haie, B., and Aubail, X. (2007). Operational Forecasting of Tropical Cyclones Storm Surges at Météo-France », JCOMM Scientific and Technical Symposium on Storm Surges, Séoul, Corée. Available at: http://www.meteorologie.eu.org/mothy/surcotes/pierre_daniel_jcomm_seoul_2007.pdf.
Day, J. W., Agboola, J., Chen, Z., D’Elia, C., Forbes, D. L., Giosan, L., et al. (2016). Approaches to Defining Deltaic Sustainability in the 21st century. Estuarine, Coastal Shelf Sci. 183, 275–291. doi:10.1016/j.ecss.2016.06.018
Descartes, (1637). Discours de la méthode. Pour bien conduire sa raison, et chercher la vérité dans les sciences.
Dietrich, J. C., Bunya, S., Westerink, J. J., Ebersole, B. A., Smith, J. M., Atkinson, J. H., et al. (2010). A High-Resolution Coupled Riverine Flow, Tide, Wind, Wind Wave, and Storm Surge Model for Southern Louisiana and Mississippi. Part II: Synoptic Description and Analysis of Hurricanes Katrina and Rita. Monthly Weather Rev. 138, 378–404. doi:10.1175/2009MWR2907.1
Disse, M., Johnson, T. G., Leandro, J., and Hartmann, T. (2020). Exploring the Relation between Flood Risk Management and Flood Resilience. Water Security 9, 100059. doi:10.1016/j.wasec.2020.100059
Dixon, T. H., Amelung, F., Ferretti, A., Novali, F., Rocca, F., Dokka, R., et al. (2006). Subsidence and Flooding in New Orleans. Nature 441, 587–588. doi:10.1038/441587a
D. M. Burdick, and C. T. Roman (Editors) (2012). “Salt Marsh Responses to Tidal Restriction and Restoration,” Tidal Marsh Restoration (Springer), 373–382.
Doody, J. P. (2013). Coastal Squeeze and Managed Realignment in Southeast England, Does it Tell Us Anything about the Future. Ocean Coastal Manag. 79, 34–41. doi:10.1016/j.ocecoaman.2012.05.008
Dunn, F. E., Nicholls, R. J., Darby, S. E., Cohen, S., Zarfl, C., and Fekete, B. M. (2018). Projections of Historical and 21st century Fluvial Sediment Delivery to the Ganges-Brahmaputra-Meghna, Mahanadi, and Volta Deltas. Sci. Total Environ. 642, 105–116. doi:10.1016/j.scitotenv.2018.06.006
Dupuits, E. J. C., Schweckendiek, T., and Kok, M. (2017). Economic Optimization of Coastal Flood Defense Systems. Reliability Eng. Syst. Saf. 159, 143–152. doi:10.1016/j.ress.2016.10.027
Duy, P. N., Chapman, L., and Tight, M. (2019). Resilient Transport Systems to Reduce Urban Vulnerability to Floods in Emerging-Coastal Cities: A Case Study of Ho Chi Minh City, Vietnam. Trav. Behav. Soc. 15, 28–43. doi:10.1016/j.tbs.2018.11.001
Emanuel, K. (2017). Assessing the Present and Future Probability of Hurricane Harvey's Rainfall. Proc. Natl. Acad. Sci. USA 114 (48), 12681–12684. doi:10.1073/pnas.1716222114
Emerton, R., Cloke, H., Ficchi, A., Hawker, L., de Wit, S., Speight, L., et al. (2020). Emergency Flood Bulletins for Cyclones Idai and Kenneth: A Critical Evaluation of the Use of Global Flood Forecasts for International Humanitarian Preparedness and Response. Int. J. Disaster Risk Reduction 50, 101811. doi:10.1016/j.ijdrr.2020.101811
Eriksen, S., Aldunce, P., Bahinipati, C. S., Martins, R. D. A., Molefe, J. I., Nhemachena, C., et al. (2011). When Not Every Response to Climate Change Is a Good One: Identifying Principles for Sustainable Adaptation. Clim. Develop. 3, 7–20. doi:10.3763/cdev.2010.0060
Erkens, G., Bucx, T., Dam, R., De Lange, G., and Lambert, J. (2015). Sinking Coastal Cities. Proc. IAHS 372, 189–198. doi:10.5194/piahs-372-189-2015
Flather, R. A. (2001). “Storm Surges,” in Encyclopaedia of Ocean Sciences. Editors J. Steele, S. Thorpe, and K. Turekian (San Diego, California: Academic), 2882–2892. doi:10.1006/rwos.2001.0124
FloodProBE, (2013). Technologies for Flood Protection of the Built Environment. Technologies for the Cost-effective Flood Protection of the Built Environment.
FLOODsite, (2009a). Flood Risk Assessment and Flood Risk Management. An Introduction and Guidance Based on Experiences and Findings of FLOODsite (An EU-Funded Integrated Project). Delft: Deltares | Delft Hydraulics, 140. 978 90 814067 1 0.
FLOODsite, (2009b). Language of Risk. Project Definitions. Report T34-02-01. Second edition, 55. e-publication on www.floodsite.net.
Folke, C. (2006). Resilience: The Emergence of a Perspective for Social-Ecological Systems Analyses. Glob. Environ. Change 16 (3), 253–267. doi:10.1016/j.gloenvcha.2006.04.002
Fischetti, M. (2015). Is New Orleans Safer Today Than When Katrina Hit 10 Years Ago?. Sci. American. Available at: www.scientificamerican.com/article/is-new-orleans-safer-today-than-when-katrina-hit-10-years-ago/ (Accessed August 13, 2021)
Formentin, S. M., and Zanuttigh, B. (2018). A New Method to Estimate the Overtopping and Overflow Discharge at Over-washed and Breached Dikes. Coastal Eng. 140, 240–256. doi:10.1016/j.coastaleng.2018.08.002
Gardner, R. C., Barchiesi, S., Beltrame, C., Finlayson, C. M., Galewski, T., Harrison, I., et al. (2015). State of the World’s Wetlands and Their Services to People : A Compilation of Recent Analyses. Ramsar Briefing Note No. 7. Gland, Switzerland: Ramsar Convention Secretariat.
Gattuso, J.-P., Magnan, A. K., Bopp, L., Cheung, W. W. L., Duarte, C. M., Hinkel, J., et al. (2018). Ocean Solutions to Address Climate Change and its Effects on marine Ecosystems. Front. Mar. Sci. 5 (337), doi:10.3389/fmars.2018.00337
Gersonius, (2016). Flood Resilience in Water Sensitive Cities: Guidance for Enhancing Flood Resilience in the Context of an Australian Water Sensitive City Melbourne, Australia. Clayton, VA: Cooperative Research Centre for Water Sensitive Cities.
Gill, J. C., and Malamud, B. D. (2014). Reviewing and Visualizing the Interactions of Natural Hazards. Rev. Geophys. 52, 680–722. doi:10.1002/2013RG000445
Giri, C., Ochieng, E., Tieszen, L. L., Zhu, Z., Singh, A., Loveland, T., et al. (2011). Status and Distribution of Mangrove Forests of the World Using Earth Observation Satellite Data. Glob. Ecol. Biogeogr. 20, 154–159. doi:10.1111/j.1466-8238.2010.00584.x
Gouldby, B., and Samuels, P. (2005). Language of Risk - Project Definitions. FLOODsite Report T32-04-01Available at: http://www.floodsite.net.
Graumann, A. (2006). Hurricane Katrina, A Climatological Perspective. Updated August 2006. NOAA’s National Climatic Data CenterAvailable at:https://www1.ncdc.noaa.gov/pub/data/techrpts/tr200501/tech-report-200501z.pdf.
Guében-Venière, S. (2015). De l’équipement à la gestion du littoral, ou comment vivre avec les aléas météo-marins aux Pays-Bas ? », Géoconfluences. mis en ligne le 14 décembre 2015. URL Available at: http://geoconfluences.ens-lyon.fr/informations-scientifiques/dossiers-thematiques/risques-et-societes/articles-scientifiques/littoral-pays-bas.
Gunderson, L. H. (2000). Ecological Resilience-In Theory and Application. Annu. Rev. Ecol. Syst. 31, 425–439. doi:10.1146/annurev.ecolsys.31.1.425
Güneralp, B., Güneralp, İ., and Liu, Y. (2015). Changing Global Patterns of Urban Exposure to Flood and Drought Hazards. Glob. Environ. Change 31, 217–225. doi:10.1016/J.GLOENVCHA.2015.01.002
Ha, T. T. T., van Dijk, H., and Bush, S. R. (2012). Mangrove Conservation or Shrimp Farmer's Livelihood? the Devolution of forest Management and Benefit Sharing in the Mekong Delta, Vietnam. Ocean Coastal Manag. 69, 185–193. doi:10.1016/j.ocecoaman.2012.07.034
Haasnoot, M. (2013). Anticipating Change. Sustainable Water Policy Pathways for an Uncertain Future. PhD thesis. UT Twente. 978-90-365-3559-5. https://ris.utwente.nl/ws/portalfiles/portal/6060551/thesis_M_Haasnoot.pdf.
Haasnoot, M., Kwakkel, J. H., Walker, W. E., and ter Maat, J. (2013). Dynamic Adaptive Policy Pathways: A Method for Crafting Robust Decisions for a Deeply Uncertain World. Glob. Environ. Change 23 (2), 485–498. doi:10.1016/j.gloenvcha.2012.12.006
Hackmann, H., Moser, S. C., and St. Clair, A. L. (2014). The Social Heart of Global Environmental Change. Nat. Clim Change 4 (8), 653–655. doi:10.1038/nclimate2320
Hagenlocher, M., Renaud, F. G., Haas, S., and Sebesvari, Z. (2018). Vulnerability and Risk of Deltaic Social-Ecological Systems Exposed to Multiple Hazards. Sci. Total Environ. 631-632, 71–80. doi:10.1016/j.scitotenv.2018.03.013
Hallegatte, S., Green, C., Nicholls, R. J., and Corfee-Morlot, J. (2013). Future Flood Losses in Major Coastal Cities. Nat. Clim Change 3, 802–806. doi:10.1038/nclimate1979
Hardisty, J. (1994). “Beach and Nearshore Sediment Transport,” in K Pye. Sediment Transport and Depositional Processes (London, UK: Blackwell), 216–255.
Harris, D. L. (1963). « Characteristics of the Hurricane Storm Surge ». Technical Paper, 48. Washington, D.C.: United States Weather Bureau, 139.
Hathout, M., Vuillet, M., Carvajal, C., Peyras, L., and Diab, Y. (2019). Expert Judgments Calibration and Combination for Assessment of River Levee Failure Probability. Reliability Eng. Syst. Saf. 188, 377–392. doi:10.1016/j.ress.2019.03.019
Hatzikyriakou, A., and Lin, N. (2018). Assessing the Vulnerability of Structures and Residential Communities to Storm Surge: An Analysis of Flood Impact during Hurricane Sandy. Front. Built Environ. 4. doi:10.3389/fbuil.2018.00004
Heinzlef, C., Becue, V., and Serre, D. (2019). Operationalizing Urban Resilience to Floods in Embanked Territories - Application in Avignon, Provence Alpes Côte D'azur regionProvence Alpes Côte D’azur Region. Saf. Sci. 118 (February), 181–193. doi:10.1016/j.ssci.2019.05.003
Heurtefeux, H., Grosset, S., and Valantin, P.-Y., (2007). Une approche alternative de la gestion des risques côtiers, l'exemple de la petite Camargue, tem, 3, 13, mis en ligne le 15 février 2012, consulté le 17 septembre 2021. URL Available at: http://journals.openedition.org/tem/491.doi:10.4000/tem.491
Hofstede, J. L. A. (2003). Integrated Management of Artificially Created Salt Marshes in the Wadden Sea of Schleswig-Holstein, Germany. Wetl. Ecol. Manag. 11, 183–194. doi:10.1023/a:1024248127037
Hurlimann, A., Barnett, J., Fincher, R., Osbaldiston, N., Mortreux, C., and Graham, S. (2014). Urban Planning and Sustainable Adaptation to Sea-Level Rise. Landscape Urban Plann. 126, 84–93. doi:10.1016/j.landurbplan.2013.12.013
Igigabel, M. (2016). Coastal Flood protection Systems. Xynthia Feedback Experience. Case Studies Conclusion. Revue Paralia 9, 4. doi:10.5150/revue-paralia.2016.s04
Igigabel, M., Nédélec, Y., Bérenger, N., Flouest, N., Bernard, A., Chassé, P., et al. (2022). Guidelines for Analysing Coastal Flood Protection Systems After a Submersion. Water 14, 15. doi:10.3390/w14010015
Igigabel, M., and Yates, M. (2018). Cost Study of Coastal protection. Int. Conf. Coastal. Eng. 1 (36), 87. doi:10.9753/icce.v36.papers.87
ImdR (2018). 21e congrès de maîtrise des risques et de sûreté de fonctionnement. Available at: https://Marchiennes-ouvertes.fr/hal-02065309/document (Accessed December 20, 2021).
Intergovernmental Oceanographic Commission (2012). Tsunami, the Great Waves. in Illus. IOC Brochure 2012-4. Second Revised Edition (Paris: UNESCO), 16.
IPCC (2007). in Climate Change 2007: The Physical Science Basis. Contribution of Working Group I to the Fourth Assessment Report of the Intergovernmental Panel on Climate Change. Editors S. Solomon, D. Qin, M. Manning, Z. Chen, M. Marquis, K. B. Averytet al. (Cambridge, United Kingdom and New York, NY, USA: Cambridge University Press), 996.
IPCC (2014). Climate Change 2014: Synthesis Report. Contribution of Working Groups I, II and III to the Fifth Assessment Report of the Intergovernmental Panel on Climate Change [Core Writing Team. Editors R. K. Pachauri, and L. A. Meyer (Geneva, Switzerland: IPCC), 151.
IPCC (2021). Climate Change 2021: The Physical Science Basis. Contribution of Working Group I to the Sixth Assessment Report of the Intergovernmental Panel on Climate Change [Masson-Delmotte. Editors, P. Zhai, A. Pirani, S. L. Connors, C. Péan, S. Bergeret al. (Cambridge University Press).
IPCC (2019). in IPCC Special Report on the Ocean and Cryosphere in a Changing Climate. Editors H.- O. Pörtner, D. C. Roberts, V. Masson-Delmotte, P. Zhai, M. Tignor, E. Poloczanskaet al.
IPCC (2012). Managing the Risks of Extreme Events and Disasters to Advance Climate Change AdaptationSpecial Report of the Intergovernmental Panel on Climate Change. Cambridge, England: Cambridge University Press.
Jiang, Y., Zevenbergen, C., and Ma, Y. (2018). Urban Pluvial Flooding and Stormwater Management: A Contemporary Review of China's Challenges and "sponge Cities" Strategy. Environ. Sci. Pol. 80, 132–143. doi:10.1016/j.envsci.2017.11.016
Jonkman, S. N., Hillen, M. M., Nicholls, R. J., Kanning, W., and van Ledden, M. (2013). Costs of Adapting Coastal Defences to Sea-Level Rise- New Estimates and Their Implications. J. Coastal Res. 290, 1212–1226. doi:10.2112/jcoastres-d-12-00230.1
Kainuma, M. (2018). “Cross-Chapter Box 1: Scenarios and Pathways,” in Global Warming of 1.5ºC. An IPCC Special Report on the Impacts of Global Warming of 1.5ºC above Pre-industrial Levels and Related Global Greenhouse Gas Emission Pathways, in the Context of Strengthening the Global Response to the Threat of Climate Change, Sustainable Development, and Efforts to Eradicate Poverty. Editors V. Masson-Delmotte, P. Zhai, H. O. Pörtner, D. Roberts, J. Skea, P. R. Shuklaet al. (Geneva, Switzerland: World Meteorological Organization), 62–64.
Klijn, F., Kreibich, H., de Moel, H., and Penning-Rowsell, E. (2015). Adaptive Flood Risk Management Planning Based on a Comprehensive Flood Risk Conceptualisation. Mitig Adapt Strateg. Glob. Change 20 (6), 845–864. doi:10.1007/s11027-015-9638-z
Krob, D. (2008). Éléments d’architecture des systèmes complexes. Available at: http://www.afscet.asso.fr/msc/textes‐2009/Krob‐elements‐archisys.pdf (Accessed December 20, 2021)).
Kruse, J., Koch, M., Khoi, C. M., Braun, G., Sebesvari, Z., and Amelung, W. (2020). Land Use Change from Permanent rice to Alternating rice-shrimp or Permanent Shrimp in the Coastal Mekong Delta, Vietnam: Changes in the Nutrient Status and Binding Forms. Sci. Total Environ. 703, 134758. doi:10.1016/j.scitotenv.2019.134758
Kumar, Y., and Mehta, D. (2021). Water Productivity Enhancement through Controlling the Flood Inundation of the Surrounding Region of Navsari Purna River, 1. India. doi:10.10.22034/WPJ.2021.264752.1024
Kummu, M., de Moel, H., Salvucci, G., Viviroli, D., Ward, P. J., and Varis, O. (2016). Over the hills and Further Away from Coast: Global Geospatial Patterns of Human and Environment over the 20th-21st Centuries. Environ. Res. Lett. 11 (3), 034010. doi:10.1088/1748-9326/11/3/034010
Kunte, P. D., Jauhari, N., Mehrotra, U., Kotha, M., Hursthouse, A. S., and Gagnon, A. S. (2014). Multi-hazards Coastal Vulnerability Assessment of Goa, India, Using Geospatial Techniques. Ocean Coastal Manag. 95, 264–281. doi:10.1016/j.ocecoaman.2014.04.024
Kwadijk, J. C. J., Haasnoot, M., Mulder, J. P. M., Hoogvliet, M. M. C., Jeuken, A. B. M., Van der Krogt, R. A. A., et al. (2010). Using Adaptation Tipping Points to Prepare for Climate Change and Sea Level Rise: a Case Study in the Netherlands. Wires Clim. Change 1 (5), 729–740. doi:10.1002/wcc.64
Le Turdu, V., Igigabel, M., Barlier, S., Masset, J.-P., Bodet, R., Dumas, M., et al. (2016). Inventory of Armourstone. E3s Web Conf. 7, 12006. doi:10.1051/e3sconf/20160712006
Lenk, S., Rybski, D., Heidrich, O., Dawson, R. J., and Kropp, J. P. (2017). Costs of Sea Dikes - Regressions and Uncertainty Estimates. Nat. Hazards Earth Syst. Sci. 17 (5), 765–779. doi:10.5194/nhess-17-765-2017
Levin, S. A. (1998). Ecosystems and the Biosphere as Complex Adaptive Systems. Ecosystems 1, 431–436. doi:10.1007/s100219900037
Lhomme, S., Laganier, R., Diab, Y., and Serre, D. (2021). The Resilience of the City of Dublin to Flooding: from Theory to Practice. cybergeo. mis en ligne le 18 novembre 2019, consulté le 31 octobre 2021. URLAvailable at: http://journals.openedition.org/cybergeo/33480. doi:10.4000/cybergeo.33480
Lhomme, S., Serre, D., Diab, Y., and Laganier, R. (2013). Analyzing Resilience of Urban Networks: A Preliminary Step towards More Flood Resilient Cities. Nat. Hazards Earth Syst. Sci. 13, 221–230. doi:10.5194/nhess-13-221-2013
Li, X., Bellerby, R., Craft, C., and Widney, S. E. (2018). Coastal Wetland Loss, Consequences, and Challenges for Restoration. Anthropocene Coasts 1 (0), 1–15. doi:10.1139/anc-2017-0001
Lichter, M., Vafeidis, A. T., Nicholls, R. J., and Kaiser, G. (2010). Exploring Data-Related Uncertainties in Analyses of Land Area and Population in the "Low-Elevation Coastal Zone" (LECZ). J. Coastal Res. 27 (4), 757–768. doi:10.2112/jcoastres-d-10-00072.1
Lissack, M. R. (1999). Complexity: the Science, its Vocabulary, and its Relation to Organizations. Emergence 1 (1), 110–126. doi:10.1207/s15327000em0101_7
Liu, B., Siu, Y. L., and Mitchell, G. (2016). Hazard Interaction Analysis for Multi-hazard Risk Assessment: a Systematic Classification Based on hazard-forming Environment. Nat. Hazards Earth Syst. Sci. 16, 629–642. doi:10.5194/nhess-16-629-2016
Luijendijk, A., Hagenaars, G., Ranasinghe, R., Baart, F., Donchyts, G., and Aarninkhof, S. (2018). The State of the World's Beaches. Sci. Rep. 8, 1–11. doi:10.1038/s41598-018-24630-6
Margaritondo, G. (2005). Explaining the Physics of Tsunamis to Undergraduate and Non-physics Students. Eur. J. Phys. 26, 401–407. doi:10.1088/0143-0807/26/3/007
Marijnissen, R. J. C., Kok, M., Kroeze, C., van Loon-Steensma, J. M., and van Loon-Steensma, (2021). Flood Risk Reduction by Parallel Flood Defences - Case-Study of a Coastal Multifunctional Flood protection Zone. Coastal Eng. 167, 103903. ISSN 0378-3839. doi:10.1016/j.coastaleng.2021.103903
Mazas, F., Kergadallan, X., Garat, P., and Hamm, L. (2014). Applying POT Methods to the Revised Joint Probability Method for Determining Extreme Sea Levels. Coastal Eng. 91, 140–150. doi:10.1016/j.coastaleng.2014.05.006
McCrory, G., and Veeckman, C. (2017). D1.1 FloodCitiSense Conceptual and Methodological Framework Available at: http://dx.doi.org/10.13140/RG.2.2.22323.32801 (Accessed December 20, 2021). doi:10.13140/RG.2.2.22323.32801
McOwen, C., Weatherdon, L., Bochove, J.-W., Sullivan, E., Blyth, S., Zockler, C., et al. (2017). A Global Map of Saltmarshes. Bdj 5 (e11764), e11764–13. doi:10.3897/BDJ.5.e11764
McPhillips, L. E., Matsler, M., Rosenzweig, B. R., and Kim, Y. (2020). What Is the Role of green Stormwater Infrastructure in Managing Extreme Precipitation Events. Sustainable Resilient Infrastructure 6, 133–142. doi:10.1080/23789689.2020.1754625
Mediterranean Wetlands Observatory, (2014). Biodiversity: Status and Trends of Species in Mediterranean Wetlands
Mehta, D. J., Eslamian, S., and Prajapati, K. (2021). Flood Modelling for a Data-Scare Semi-arid Region Using 1-D Hydrodynamic Model: a Case Study of Navsari Region. Model. Earth Syst. Environ. doi:10.1007/s40808-021-01259-5
Mehta, D. J., and Yadav, S. M. (2020). Hydrodynamic Simulation of River Ambica for Riverbed Assessment: A Case Study of Navsari Region, 127, 140 doi:10.1007/978-981-13-8181-2_10
Merkens, J.-L., Reimann, L., Hinkel, J., and Vafeidis, A. T. (2016). Gridded Population Projections for the Coastal Zone under the Shared Socioeconomic Pathways. Glob. Planet. Change 145, 57–66. doi:10.1016/j.gloplacha.2016.08.009
Michel, H. (2018). Évaluation probabiliste de la fiabilité structurelle des digues fluviales à partir du jugement d’expert. Architecture, aménagement de l’espace. Français: Université Paris-Est.
Mikami, T., Esteban, M., and Shibayama, T. (2015). “Storm Surge in New York City Caused by Hurricane Sandy in 2012,” in Handbook of Coastal Disaster Mitigation for Engineers and Planners. Editors M. Esteban, H. Takagi, and T. Shibayama (Butterworth-Heinemann), 115–131. doi:10.1016/B978-0-12-801060-0.00007-1
Millenn Ecosyst Assess, (2005). Ecosystems and Human Well-Being: Biodiversity Synthesis. Washington, DC: World Resour. Inst.
Millenn Ecosyst. Assess, (2003). Ecosystems and Human Well-Being: A Framework for Assessment. Washington, DC: Island Press.
Moberg, F., and Galaz, V. (2005). Resilience: Going from Conventional to Adaptive Freshwater Management for Human and Ecosystem Compatibility, 3. Stockholm: Swedish Water House Policy BriefStockholm International Water Institute.
Moss, R. H., Edmonds, J. A., Hibbard, K. A., Manning, M. R., Rose, S. K., van Vuuren, D. P., et al. (2010). The Next Generation of Scenarios for Climate Change Research and Assessment. Nature 463, 747–756. doi:10.1038/nature08823
Moss, R. (2008). “Towards New Scenarios For Analysis of Emissions, Climate Change, Impacts and Response Strategies,” in IPCC Expert Meeting Report, Noordwijkerhout, The Netherlands, September 19–21, 2007, 25 Available at: https://www.ipcc.ch/site/assets/uploads/2018/05/expert-meeting-ts-scenarios-1-1.pdf (Accessed December 20, 2021.
Muis, S., Verlaan, M., Winsemius, H. C., Aerts, J. C. J. H., and Ward, P. J. (2016). A Global Reanalysis of Storm Surges and Extreme Sea Levels. Nat. Commun. 7, 20169. doi:10.1038/ncomms11969
Murray, N. J., Clemens, R. S., Phinn, S. R., Possingham, H. P., and Fuller, R. A. (2014). Tracking the Rapid Loss of Tidal Wetlands in the Yellow Sea. Front. Ecol. Environ. 12, 267–272. doi:10.1890/130260
Myers, T. A., Nisbet, M. C., Maibach, E. W., and Leiserowitz, A. A. (2012). A Public Health Frame Arouses Hopeful Emotions about Climate Change. Climatic Change 113 (3–4), 1105–1112. doi:10.1007/s10584-012-0513-6
Narayan, S., Beck, M. W., Reguero, B. G., Losada, I. J., van Wesenbeeck, B., Pontee, N., et al. (2016). The Effectiveness, Costs and Coastal protection Benefits of Natural and Nature-Based Defences. PLoS One 11 (5), e0154735. doi:10.1371/journal.pone.0154735
Ndour, A., Laïbi, R. A., Sadio, M., Degbe, C. G. E., Diaw, A. T., Oyédé, L. M., et al. (2018). Management Strategies for Coastal Erosion Problems in West Africa: Analysis, Issues, and Constraints Drawn from the Examples of Senegal and Benin. Ocean Coastal Manag. 156, 92–106. doi:10.1016/j.ocecoaman.2017.09.001
Neumann, B., Vafeidis, A. T., Zimmermann, J., and Nicholls, R. J. (2015). Future Coastal Population Growth and Exposure to Sea-Level Rise and Coastal Flooding - A Global Assessment. PLoS One 10, e0118571. doi:10.1371/journal.pone.0118571
Nicholls, R. J., Hanson, S., Herweijer, C., and Patmore, N. (2008). Ranking Port Cities with High Exposure and Vulnerability to Climate Extremes. available at: https://www.oecd-ilibrary.org/content/workingpaper/011766488208.
Olsson, P., Folke, C., and Berkes, F. (2004). Adaptive Comanagement for Building Resilience in Social?Ecological Systems. Environ. Manage. 34, 75–90. doi:10.1007/s00267-003-0101-7
O’Neill, B. C., Kriegler, E., Ebi, K. L., Kemp-Benedict, E., Riahi, K., Rothman, D. S., et al. (2017). The Roads Ahead: Narratives for Shared Socioeconomic Pathways Describing World Futures in the 21st century. Glob. Environ. Chang. 42, 169–180.
Ouillon, S. (2018). Why and How Do We Study Sediment Transport? Focus on Coastal Zones and Ongoing Methods. Water 10 (4), 390–434. doi:10.3390/w10040390
Patel, S. B., Mehta, D. J., and Yadav, S. M. (2018). One Dimensional Hydrodynamic Flood Modeling for Ambica River, South Gujarat. Journal of Emerging Technologies and Innovative Research.
Perherin, C. (2017). La concertation lors de la cartographie des aléas littoraux dans les Plans de Prévention des Risques : enjeu majeur de prévention. Géographie. Université de Bretagne occidentale – BrestFrançais.
Deboudt, P., and Goeldner-Gianella, L., Dépoldériser en Europe occidentale. Pour une géographie et une gestion intégrées du littoral », Territoire en mouvement Revue de géographie et aménagement [En ligne], 27–28, (2015). mis en ligne le 18 mars 2015, consulté le 18 mai 2021.Available at: http://journals.openedition.org/tem/2687 doi:10.4000/tem.2687
Peduzzi, P. (2014). Sand, Rarer Than One Thinks. Environ. Develop. 11, 208–218. doi:10.1016/j.envdev.2014.04.001
Peterson, G. D., Cumming, G. S., and Carpenter, S. R. (2003). Scenario Planning: a Tool for Conservation in an Uncertain World. Conservation Biol. 17, 358–366. doi:10.1046/j.1523-1739.2003.01491.x
Pontee, N. (2013). Defining Coastal Squeeze: A Discussion. Ocean Coastal Manag. 84, 204–207. doi:10.1016/j.ocecoaman.2013.07.010
Prescott, S., and Logan, A. (2018). Larger Than Life: Injecting hope into the Planetary Health Paradigm. Challenges 9 (13), 13–27. doi:10.3390/challe9010013
Pycroft, J., Abrell, J., and Ciscar, J.-C. (2016). The Global Impacts of Extreme Sea-Level Rise: A Comprehensive Economic Assessment. Environ. Resource Econ. 64, 225–253. doi:10.1007/s10640-014-9866-9
Quinlan, A. E., Berbés-Blázquez, M., Haider, L. J., and Peterson, G. D. (2016). Measuring and Assessing Resilience: Broadening Understanding through Multiple Disciplinary Perspectives. J. Appl. Ecol. 53 (3), 677–687. doi:10.1111/1365-2664.12550
Ramsar (2016). Les zones humides : une protection naturelle contre les catastrophes. Available at: https://www.ramsar.org/sites/default/files/fs_9_drr_fr_30j_2.pdf.
Renaud, F. G., Le, T. T. H., Lindener, C., Guong, V. T., and Sebesvari, Z. (2015). Resilience and Shifts in Agro-Ecosystems Facing Increasing Sea-Level Rise and Salinity Intrusion in Ben Tre Province, Mekong Delta. Climatic Change 133 (1), 69–84. doi:10.1007/s10584-014-1113-4
Renaud, F. G., Syvitski, J. P., Sebesvari, Z., Werners, S. E., Kremer, H., Kuenzer, C., et al. (2013). Tipping from the Holocene to the Anthropocene: How Threatened Are Major World Deltas. Curr. Opin. Environ. Sustainability 5 (6), 644–654. doi:10.1016/j.cosust.2013.11.007
Rodina, L. (2018). Defining "water Resilience": Debates, Concepts, Approaches, and Gaps. WIREs Water 6, 2. doi:10.1002/wat2.1334
Rovere, A., Stocchi, P., and Vacchi, M. (2016). Eustatic and Relative Sea Level Changes. Curr. Clim. Change Rep. 2, 221–231. doi:10.1007/s40641-016-0045-7
Roy, J., Tschakert, P., Waisman, H., and Halim, S. A. (2018). “Sustainable Development, Poverty Eradication and Reducing Inequalities. In: Global Warming of 1.5°C. An IPCC Special Report on the Impacts of Global Warming of 1.5°C Above Pre-Industrial Levels and Related Global Greenhouse Gas Emission Pathways,,” in The Context of Strengthening the Global Response to the Threat of Climate Change, Sustainable Development, and Efforts to Eradicate Poverty Editors V. Masson-Delmotte, P. Zhai, H. O. Pörtner, D. Roberts, J. Skea, P. R. Shuklaet al. (Geneva, Switzerland: World Meteorological Organization). https://www.ipcc.ch/sr15/.
Ruitenbeek, J., and Cartier, C. (2001). The Invisible Wand: Adaptive Co-management as an Emergent Strategy in Complex Bio-Economic Systems. Occasional Paper Number 34. CIFOR,Available at: https://core.ac.uk/display/132638335.
Sarah, D., and Soebowo, E. (2018). Land Subsidence Threats and its Management in the North Coast of Java. IOP Conf. Ser. Earth Environ. Sci. 118, 012042. doi:10.1088/1755-1315/118/1/012042
Sayers, P., and Meadowcroft, I. (2005). “RASP-A Hierarchy of Risk-Based Methods and Their Application,” in Defra Flood and Coastal Management Conference, 2005, York, United Kingdom, July 5–7, 2005.
Schreurs, M. A. (2021). Reconstruction and Revitalization in Fukushima a Decade after the “Triple Disaster” Struck: Striving for Sustainability and a New Future Vision. Int. J. Disaster Risk Reduction 53, 102006. doi:10.1016/j.ijdrr.2020.102006
Scussolini, P., Tran, T. V. T., Koks, E., Diaz‐Loaiza, A., Ho, P. L., and Lasage, R. (2017). Adaptation to Sea Level Rise: A Multidisciplinary Analysis for Ho Chi Minh City, Vietnam. Water Resour. Res. 53, 10841–10857. doi:10.1002/2017WR021344
Serre, D., Barroca, B., Balsells, M., and Becue, V. (2018). Contributing to Urban Resilience to Floods with Neighbourhood Design: the Case of Am Sandtorkai/Dalmannkai in Hamburg. J. Flood Risk Manage. 11, S69–S83. doi:10.1111/jfr3.12253
Serre, D., Peyras, L., Maurel, P., Tourment, R., and Diab, Y. (2009). A Spatial Decision Support System Aiding Levee Managers in Their Repair and Maintenance Planning. J. Decis. Syst. 18, 347–373. doi:10.3166/jds.18.347-373
Simm, J., Collell, M. R., Flikweert, J., Tourment, R., Neutz, C., and van Steeg, P. (2019). “Sustainable and Safe Dams Around the World,” in Proceedings of the ICOLD 2019 Symposium, (ICOLD 2019), Ottawa, ON, CRP Press, June 9–14, 2019,, 2298–2308
Simm, J., Gouldby, B. P., Sayers, P. B., Flikweert, J. J., Wersching, S., and Bramley, M. E. (2008). “Representing Fragility of Flood and Coastal Defences: Getting into the Detail,” in Flood Risk Management: Research and Practice. Editors Samuels, P., Huntington, S., Allsop, W., and Harrop, J. ( CRC Press).
Singh, P., Amekudzi-Kennedy, A., Woodall, B., and Joshi, S. (2021). Lessons from Case Studies of Flood Resilience: Institutions and Built Systems. Transportation Res. Interdiscip. Perspect. 9, 100297. doi:10.1016/j.trip.2021.100297
Sippo, J. Z., Lovelock, C. E., Santos, I. R., Sanders, C. J., and Maher, D. T. (2018). Mangrove Mortality in a Changing Climate: An Overview. Estuarine, Coastal Shelf Sci. 215, 241–249. doi:10.1016/j.ecss.2018.10.011
Smith, M. S., Horrocks, L., Harvey, A., and Hamilton, C. (2011). Rethinking Adaptation for a 4 ° C World. Phil. Trans. R. Soc. A. 369, 196–216. doi:10.1098/rsta.2010.0277
Stanczak, G., and Oumeraci, H. (2012). Modeling Sea dike Breaching Induced by Breaking Wave Impact-Laboratory Experiments and Computational Model. Coastal Eng. 59 (Issue 1), 28–37. doi:10.1016/j.coastaleng.2011.07.001
Sugawara, D. (2021). Numerical Modeling of Tsunami: Advances and Future Challenges after the 2011 Tohoku Earthquake and Tsunami. Earth-Science Rev. 214, 103498. doi:10.1016/j.earscirev.2020.103498
Suzuki, T., Altomare, C., Veale, W., Verwaest, T., Trouw, K., Troch, P., et al. (2017). Efficient and Robust Wave Overtopping Estimation for Impermeable Coastal Structures in Shallow Foreshores Using SWASH. Coastal Eng. 122, 108–123. doi:10.1016/j.coastaleng.2017.01.009
Syvitski, J. P. M., Kettner, A. J., Overeem, I., Hutton, E. W. H., Hannon, M. T., Brakenridge, G. R., et al. (2009). Sinking Deltas Due to Human Activities. Nat. Geosci 2, 681–686. doi:10.1038/ngeo629
Syvitski, J. P. M., and Saito, Y. (2007). Morphodynamics of Deltas under the Influence of Humans. Glob. Planet. Change 57, 261–282. doi:10.1016/j.gloplacha.2006.12.001
Tate, E. (2012). Social Vulnerability Indices: a Comparative Assessment Using Uncertainty and Sensitivity Analysis. Nat. Hazards 63, 325–347. doi:10.1007/s11069-012-0152-2
Termeer, C. J. A. M., Dewulf, A., and Biesbroek, G. R. (2017). Transformational Change: Governance Interventions for Climate Change Adaptation from a Continuous Change Perspective. J. Environ. Plann. Manag. 60 (4), 558–576. doi:10.1080/09640568.2016.1168288
Tessler, Z. D., Vörösmarty, C. J., Grossberg, M., Gladkova, I., Aizenman, H., Syvitski, J. P. M., et al. (2015). Profiling Risk and Sustainability in Coastal Deltas of the World. Science 349 (6248), 638–643. doi:10.1126/science.aab3574
Tessler, Z. D., Vörösmarty, C. J., Overeem, I., and Syvitski, J. P. M. (2018). A Model of Water and Sediment Balance as Determinants of Relative Sea Level Rise in Contemporary and Future Deltas. Geomorphology 305, 209–220. doi:10.1016/j.geomorph.2017.09.040
The Rockefeller Foundation (2015). City Resilience Index. Available at: https://www.rockefellerfoundation.org/wp‐content/uploads/CRI‐Revised‐Booklet1.pdf (Accessed December 20, 2021.
Thi Ha, D., Ouillon, S., and Van Vinh, G. (2018). Water and Suspended Sediment Budgets in the Lower Mekong from High-Frequency Measurements (2009-2016). Water 10 (7), 846. doi:10.3390/w10070846
Thomas, P., Brown, J. M., and Plater, A. J. (2016). Flood Inundation Uncertainty: The Case of a 0.5% Annual Probability Flood Event. Environ. Sci. Pol. 59, 1. doi:10.1016/j.envsci.2016.01.018
Tiberi‐Wadier, A. L., Goutal, N., Ricci, S., Sergent, P., Taillardat, M., Bouttier, F., et al. (2021). Strategies for Hydrologic Ensemble Generation and Calibration: On The Merits of Using Model‐Based Predictors. J. Hydrol. 599, 126233. doi:10.1016/j.jhydrol.2021.126233
Tiggeloven, T., de Moel, H., Winsemius, H. C., Eilander, D., Erkens, G., Gebremedhin, E., et al. (2020). Global-scale Benefit-Cost Analysis of Coastal Flood Adaptation to Different Flood Risk Drivers Using Structural Measures. Nat. Hazards Earth Syst. Sci. 20, 1025–1044. doi:10.5194/nhess-20-1025-2020
Torres, A., Brandt, J., Lear, K., and Liu, J. (2017). A Looming Tragedy of the Sand Commons. Science 357 (6355), 970–971. doi:10.1126/science.aao0503
United States Environmental Protection Agency [US EPA] (2020). What is Green Infrastructure? [Overviews and Factsheets]. US EPA. Available at: https://www.epa.gov/greeninfrastructure/what-green-infrastructure (Accessed on December 20, 2021).
van Berchum, E. C., van Ledden, M., Timmermans, J. S., Kwakkel, J. H., and Jonkman, S. N. (2020). Rapid Flood Risk Screening Model for Compound Flood Events in Beira, Mozambique. Nat. Hazards Earth Syst. Sci. 20, 2633–2646. doi:10.5194/nhess-20-2633-2020
van Bergeijk, V. M., Warmink, J. J., Frankena, M., and Hulscher, S. J. M. H. (2019). “Modelling Dike Cover Erosion by Overtopping Waves: The Effects of Transitions,” in Coastal Structures 2019. Bundesanstalt für Wasserbau, Coastal Structures Conference 2019, Hannover, Lower Saxony, Germany, September 30, 2019. Editors N. Goseberg, and T. Schlurmanndoi:10.18451/978-3-939230-64-9_110
van der Nat, A., Vellinga, P., Leemans, R., and van Slobbe, E. (2016). Ranking Coastal Flood protection Designs from Engineered to Nature-Based. Ecol. Eng. 87, 80–90. doi:10.1016/j.ecoleng.2015.11.007
van Hattum, K. C., Lars, T. D. R., Matthijs, F. M., Bos, J. K., and Hans, H. D. M. (2020). “Shifting Away from Asset Damage and towards Well-Being Loss within Flood Risk Management,” in Proceedings of the FLOODrisk 2020‐4th European Conference on Flood Risk Management, Online, June 22–24, 2021.
van Vuuren, D. P., Edmonds, J., Kainuma, M., Riahi, K., Thomson, A., Hibbard, K., et al. (2011). The Representative Concentration Pathways: An Overview. Climatic Change 109 (1–2), 5–31. doi:10.1007/s10584-011-0148-z
Vinh, V. D., Ouillon, S., Thanh, T. D., and Chu, L. V. (2014). Impact of the Hoa Binh Dam (Vietnam) on Water and Sediment Budgets in the Red River basin and delta. Hydrol. Earth Syst. Sci. 18 (10), 3987–4005. doi:10.5194/hess-18-3987-2014
Vitale, C., Meijerink, S., Moccia, F. D., and Ache, P. (2020). Urban Flood Resilience, a Discursive-Institutional Analysis of Planning Practices in the Metropolitan City of Milan. Land Use Policy 95, 104575. doi:10.1016/j.landusepol.2020.104575
Vousdoukas, M. I., Bouziotas, D., Giardino, A., Bouwer, L. M., Mentaschi, L., Voukouvalas, E., et al. (2018). Understanding Epistemic Uncertainty in Large-Scale Coastal Flood Risk Assessment for Present and Future Climates. Nat. Hazards Earth Syst. Sci. 18 (8), 2127–2142. doi:10.5194/nhess-18-2127-2018
Vuik, V., Borsje, B. W., WillemsenWillemsen, P. W. J. M., and Jonkman, S. N. (2019). Salt Marshes for Flood Risk Reduction: Quantifying Long-Term Effectiveness and Life-Cycle Costs. Ocean Coastal Manag. 171, 96–110. doi:10.1016/j.ocecoaman.2019.01.010
Vuik, V., van Vuren, S., Borsje, B. W., van Wesenbeeck, B. K., Jonkman, S. N., and Jonkman, S. N. (2018). Assessing Safety of Nature-Based Flood Defenses: Dealing with Extremes and Uncertainties. Coastal Eng. 139, 47–64. doi:10.1016/j.coastaleng.2018.05.002
Walker, B., Holling, C. S., Carpenter, S. R., and Kinzig, A. (2004). Resilience, Adaptability and Transformability in Social–Ecological Systems. Ecol. Soc. 9 (2), 5. doi:10.5751/es-00650-090205
Weichselgartner, J., and Kasperson, R. (2010). Barriers in the Science-Policy-Practice Interface: Toward a Knowledge-Action-System in Global Environmental Change Research. Glob. Environ. Change 20 (2), 266–277. doi:10.1016/j.gloenvcha.2009.11.006
Weichselgartner, J., and Kelman, I. (2014). Geographies of Resilience. Prog. Hum. Geogr. 39 (3), 249–267. doi:10.1177/0309132513518834
Welch, A. C., Nicholls, R. J., and Lázár, A. N. (2017). Evolving Deltas: Coevolution with Engineered Interventions. Elem. Sci. Anth 5 (49), 1–18. doi:10.1525/elementa.128
Winterwerp, J. C., Erftemeijer, P. L. A., Suryadiputra, N., Van Eijk, P., and Zhang, L. (2013). Defining Eco-Morphodynamic Requirements for Rehabilitating Eroding Mangrove-Mud Coasts. Wetlands 33 (3), 515–526. doi:10.1007/s13157-013-0409-x
WMO (2017). Selecting Measures and Designing Strategies for Integrated Flood Management – A Guidance Document. Available at: https://www.floodmanagement.info/publications/guidance%20-%20selecting%20measures%20and%20designing%20strategies_e_web.pdf (Accessed December 20, 2021.
Wong, P. P. (2014). “Coastal Systems and Low-Lying Areas,” in Climate Change 2014: Impacts, Adaptation, and Vulnerability. Part A: Global and Sectoral Aspects. Contribution of Working Group II to the Fifth Assessment Report of the Intergovernmental Panel of Climate Change [Barros. Editors C. B. Field, D. J. Dokken, M. D. Mastrandrea, K. J. Mach, T. E. Biliret al. (Cambridge, United Kingdom and New York, NY, USA: Cambridge University Press), 361–409.
Wu, W., and Li, H. (2017). A Simplified Physically-Based Model for Coastal dike and Barrier Breaching by Overtopping Flow and Waves. Coastal Eng. 130, 1–13. doi:10.1016/j.coastaleng.2017.09.007
Yadav, S. M., and Mangukiya, N. K. (2021). “Semi-Arid River Basin Flood: Causes, Damages, and Measures,” in Proceedings of the Fifth International Conference in Ocean Engineering (ICOE2019). Editor Sundar, V., Sannasiraj, S. A., Sriram, V., and Nowbuth, M. D.(Springer, Singapore: Lecture Notes in Civil Engineering,) 106. doi:10.1007/978-981-15-8506-7_16
Zevenbergen, C., van Herk, S., Escarameia, M., Gersonius, B., Serre, D., Walliman, N., et al. (2015). Assessing Quick Wins to Protect Critical Urban Infrastructure from Floods: a Case Study in Bangkok, Thailand. J. Flood Risk Manage. 11, S17–S27. doi:10.1111/jfr3.12173
Zevenbergen, C., Veerbeek, W., Gersonius, B., and Van Herk, S. (2008). Challenges in Urban Flood Management: Travelling across Spatial and Temporal Scales. J. Flood Risk Manag. 1 (2), 81–88. doi:10.1111/j.1753-318X.2008.00010.x
Zhang, Y., Chen, G., Hu, J., Chen, X., Yang, W., Tao, A., et al. (2017). Experimental Study on Mechanism of Sea-dike Failure Due to Wave Overtopping. Appl. Ocean Res. 68, 171–181. ISSN 0141-1187. doi:10.1016/j.apor.2017.08.009
Zhu, X., Linham, M. M., and Nicholls, R. J. (2010). Technologies for Climate Change Adaptation: Coastal Erosion and Flooding TNA Guidebook Series, Roskilde: Danmarks Tekniske Universitet. Risø Nationallaboratoriet for Bæredygtig Energi [Available at: http://orbit.dtu.dk/files/5699563/Technologies%20for%20Climate%20Change%20Adaptation-Coastal%20Erosion%20and%20Flooding.pdf .
Keywords: resilience, flood protection systems, climate change adaptation, methods, system analysis
Citation: Igigabel M, Diab Y and Yates M (2022) Exploring Methodological Approaches for Strengthening the Resilience of Coastal Flood Protection System. Front. Earth Sci. 9:756936. doi: 10.3389/feart.2021.756936
Received: 11 August 2021; Accepted: 07 December 2021;
Published: 08 February 2022.
Edited by:
Jorge Eduardo Teixeira Leandro, University of Siegen, GermanyReviewed by:
Darshan Mehta, Dr. S. & S. S. Ghandhy College of Engineering & Technology, IndiaChristoph Mudersbach, Bochum University of Applied Sciences, Germany
Copyright © 2022 Igigabel, Diab and Yates. This is an open-access article distributed under the terms of the Creative Commons Attribution License (CC BY). The use, distribution or reproduction in other forums is permitted, provided the original author(s) and the copyright owner(s) are credited and that the original publication in this journal is cited, in accordance with accepted academic practice. No use, distribution or reproduction is permitted which does not comply with these terms.
*Correspondence: Marc Igigabel, bWFyYy5pZ2lnYWJlbEBjZXJlbWEuZnI=