- 1Department of Chemical and Physical Sciences, University of Toronto Mississauga, Mississauga, ON, Canada
- 2Department of Earth & Environmental Sciences, Vanderbilt University, Nashville, TN, United States
- 3Senckenberg Museum of Natural History, Frankfurt, Germany
Ernietta plateauensis is a semi-infaunal macroscopic eukaryote of unknown affinities common in latest Ediacaran (∼548–539 Ma) shallow marine settings in Namibia. The discovery of in-situ assemblages of Ernietta has demonstrated that these organisms lived in aggregated populations, while studies employing computational fluid dynamics (CFD) modeling have supported the hypothesis that these organisms were likely behaving as gregarious suspension feeders, analogous to many extant invertebrate phyla in present-day marine environments. Careful census and measurement of individuals within these in-situ populations offers an opportunity to examine how their size and location within a larger population affect nutrient delivery dynamics. In this study, we build on previous work by simulating fluid flow over aggregations of Ernietta comprising individuals of disparate sizes, and additionally reconstruct a population of Ernietta preserved in-situ from Farm Hansburg, Namibia. We use a combination of stationary and time-dependent CFD to reconstruct nutrient carrying flow paths, and compare the efficiency with which nutrients are partitioned between individuals of different shapes and sizes. Our results demonstrate that smaller Ernietta experience limited recirculation within their cavities compared to larger individuals. Furthermore, in spatially-accurate distributions, reduced recirculation is limited to isolated individuals of any size, while smaller individuals found downstream of larger ones receive enhanced cavity mixing. These reconstructed flow patterns illustrate that the disadvantage associated with small size is apparently mediated by location within the overall aggregation, suggesting a complex interplay of controls on feeding efficiency. This in turn suggests that aggregations of adult Ernietta would likely have performed a ‘nursery’ function, creating localized conditions ideal for the settlement and growth of younger individuals.
Introduction
Ediacaran fossil assemblages preserve the earliest evidence of macroscopic communities in the fossil record (Narbonne, 2005; Knoll et al., 2006). These fossils have historically been divided into three distinct assemblages referred to as the “Avalon”, “White Sea”, and “Nama” (Waggoner, 2003; Boag et al., 2016), which reflect a combination of distinct preservational (Gehling, 1999; Narbonne, 2005; Droser et al., 2017), paleoenvironmental (Grazhdankin, 2004; Grazhadankin, 2014; Gehling and Droser, 2013; Liu et al., 2015), and evolutionary (Grazhadankin, 2014; Droser et al., 2017) stages. The older Avalon assemblages (e.g., see Liu et al., 2015) are characterized primarily by arboreomorphs, rangeomorphs, and triradialomorphs that persist into the younger White Sea and Nama assemblages (Laflamme et al., 2013). The middle White Sea Assemblage has the greatest morphological and species diversity (Shen et al., 2008), while the youngest Nama Assemblage preserves communities dominated by erniettomorphs, and which are characterized by relatively low species richness and community structures suggestive of limited levels of competition and/or ecological stress (Muscente et al., 2019; Darroch et al., 2015; 2018a,b; although see Vaziri et al., 2021). Previous analyses that have investigated the role of size in in-situ Ediacaran populations have typically focused on Avalon-aged (∼571–558 Ma) rangeomorph communities from Mistaken Point, Newfoundland (Clapham and Narbonne, 2002; Clapham et al., 2003; Darroch et al., 2013; Mitchell et al., 2015; Mitchell and Kenchington, 2018; Boddy et al., 2021). Building off these, more recent numerical studies have focused on how the spatial arrangement of individual organisms within communities may reflect paleoecological and -biological aspects of individual taxa, including potential reproductive modes, dispersal distances, and interspecific interactions (Hall et al., 2015; Mitchell et al., 2015, 2018; Mitchell and Butterfield, 2018; Mitchell and Kenchington, 2018). However, to date no studies have applied these approaches to the latest Ediacaran Nama-aged (∼548–539 Ma) assemblages, which in southern Namibia can be found preserved as large monospecific accumulations in life position (Gibson et al., 2019). In this study, we investigate the size and spatial distributions of individuals within in-situ populations of Ernietta plateauensis from Farm Hansburg, Namibia. We test hypotheses surrounding how these dense populations reflect aspects of life history, and/or adaptations to feeding. Given that communities of erniettomorph Ediacara biota thrived immediately prior to the Cambrian boundary (e.g., Elliott et al., 2016; Meyer et al., 2014; Darroch et al., 2018a; Darroch et al., 2021), understanding the structure and function of their communities will shed valuable light on ecological dynamics during the Ediacaran-Cambrian transition (Darroch et al., 2018a).
Ernietta plateauensis is an iconic Ediacaran taxon that is found in a range of paleoenvironments up until the Ediacaran–Cambrian boundary (Bouougri et al., 2011; Laflamme et al., 2013; Darroch et al., 2015; Smith et al., 2017; Maloney et al., 2020). It is a sack-like organism constructed from modular, tubular elements in a double-walled palisade structure that are alternatingly stitched along a basal medial seam (Jenkins et al., 1981; Elliott et al., 2016; Ivantsov et al., 2016). Individuals lived semi-infaunally with the basal portion buried within the seafloor, and with an upper portion extended above the sediment-water interface (Seilacher, 1992; Seilacher et al., 2003; Bouougri et al., 2011; Seilacher and Gishlick, 2014; Elliott et al., 2016; Ivantsov et al., 2016). Based on their presence in ripple-laminated fine-grained sandstones preserving evidence of microbial mats, Ernietta is interpreted to have lived in protected, high-to medium-energy shallow marine settings with periodic clastic sediment supply (Bouougri et al., 2011; Ivantsov et al., 2016; Maloney et al., 2020). These inner-to outer-ramp environments are characterized by unstable marine redox conditions indicating that Ernietta was likely capable of living in environments characterized by low or fluctuating oxygen levels (Hall et al., 2013; Wood et al., 2015; Bowyer et al., 2017; 2020). Similar to trends observed in other macroscopic eukaryotes (Pteridinium, Windermeria) observed in the Blueflower Formation of Northwestern Canada (Sperling et al., 2016), Ernietta may also have been able to opportunistically colonize environments that experienced transient pulses of oxygenation (Hall et al., 2013; Bowyer et al., 2020). Furthermore, Ernietta fossils are often found in large accumulations and have been interpreted to be in life position based on their vertical orientation (Bouougri et al., 2011; Maloney et al., 2020), lacking apparent biostratinomic compaction (Elliott et al., 2016; Ivantsov et al., 2016), and detailed sedimentological analyses suggesting continued vertical growth (Jenkins et al., 1981; Jenkins, 1992). Previous work employing computational fluid dynamics (CFD) modeling has suggested both that Ernietta likely functioned as a sessile suspension feeder based on the consistent presence of recirculation within its cavity, and that living in aggregated patches led to increased mixing above populations, replenishing nutrients and helping to diffuse any potential waste (i.e., “commensalism” though technically ecological facilitation–see Gibson et al., 2019).
Previous studies (Ivantsov et al., 2016; Gibson et al., 2019) noted that populations of Ernietta in southern Namibia tend to comprise individuals with a wide range of sizes, and non-random spatial organizations. While Gibson et al. (2019) demonstrated certain advantages of gregarious living using simplified, idealized spatial arrangements of uniformly sized Ernietta, these are not entirely representative of the original system, and potentially mask subtitle interactions that result from these more complex community structures. By analogue with modern marine benthic organisms that live gregariously, this raises the question: how might individual size and position within aggregations affect the success of individuals within the broader population? Individual success in modern marine organisms is influenced by a combination of external (environmental) and intrinsic (conspecific) controls. External controls are typically density-independent and may include: local water temperature (Pepin, 1991); local sedimentation rates, volumes, and type (Gilmour, 1999); and the area of available substrate suitable for settlement/recruitment (Harrington et al., 2004). In contrast, intrinsic controls, such as population growth rates, are more likely to be density-dependent (Caley et al., 1996). Interactions between these external and intrinsic controls can regulate the magnitude and direction of density-dependent effects (Hixon et al., 2002; White, 2007; Vermeij and Sandin, 2008; Doropoulos et al., 2017). Examples include prey mortality severity (Raymundo and Maypa, 2004; White, 2007), larval settlement and survivability (Gaines and Roughgarden, 1985; Raimondi, 1990; Doropoulos et al., 2017), and food delivery (Bertness and Grosholz, 1985; Bertness et al., 1991; Leonard et al., 1998). Furthermore, individual survivability at low settlement densities can be density-independent, but there is increasing evidence to suggest that gregarious settlement has the potential to increase survivability depending on environment (Bertness and Grosholz, 1985; Raymundo and Maypa, 2004; Linden and Rinkevich, 2017). This is particularly important in the absence of predation [such as during the Ediacaran (Xiao and Laflamme, 2009)]—as predation is the most frequent cause of post-settlement, density-dependent mortality (Gaines and Roughgarden, 1985). As an example, population assessments of the extant ribbed mussel (Geukensia) residing along the seaward margins of salt marshes have shown that during colder seasons with high tide, low density aggregations have higher mortality rates when compared to denser aggregations (Bertness and Grosholz, 1985).
While these patterns are possible to observe in modern ecosystems, detecting similar interactions within Ediacaran communities is more challenging and requires strategies for indirect assessment. In this study, we explore individual size- and location-specific controls on individual success (measured as inferred feeding efficiency) within Ernietta populations, using the strength of recirculation within the cavities of individuals as a proxy for feeding efficiency. Previous work on Ernietta feeding has suggested that cavity recirculation is linked to suspension feeding implying a positive correlation between recirculation and nutrient acquisition, such that individuals within a population that experience greater cavity recirculation are interpreted to have greater feeding success (Gibson et al., 2019; 2021). To test the effect of size and location on feeding efficiency within populations, we compare patterns of recirculation within idealized arrays of Ernietta, alongside those within a reconstructed population recorded during fieldwork. The results of these analyses reveal whether there are advantages associated with specific locations within populations, and thus whether monospecific communities of Ernietta may have been characterized by competition for space.
Materials and Methods
All simulation parameters and models from this study were based on latest Ediacaran material from Namibia that have been published elsewhere. For a detailed description of the geologic background, see Maloney et al. (2020) and Bouougri et al. (2011). For all simulations, we used a previously published 3D Ernietta model (Gibson et al., 2019) scaled to dimensions reported by Ivantsov et al. (2016). We conducted three groups of simulations: 1) single individual simulations, where a “large” individual was scaled identical to Gibson et al. (2019) simulations, and the “small” individual was isotropically half that size. 2) Idealized populations where we created square arrays comprised of four individuals. And, 3) a spatially-accurate reconstructed population of Ernietta. Within the idealized population arrays, we varied the size of individuals iteratively, producing arrays with smaller individuals upstream (translated by 0.025 m) and downstream (translated by 0.15 m). Again, smaller individuals were isotropically scaled to half of the larger individuals, which fell well within the range reported by previous studies (Ivantsov et al., 2016; Elliott et al., 2016; Gibson et al., 2019). In order to recreate real in-situ populations, we used as a reference a slab from the Kliphoek Member of the Dabis Formation from Farm Hansburg, Namibia, that preserves an in-situ Ernietta population (Figure 1; Supplementary Figure S1; Bouougri et al., 2011; Gibson et al., 2019; Maloney et al., 2020). This slab was collected from the ripple laminated, fine grained sandstone Facies 3 of Maloney et al. (2020), and was solely examined and photographed in the field due to its large size. We digitally reconstructed the Hansburg slab by placing and anisotropically scaling individuals to visually match the exposed surface. Individual dimensions were taken by measuring the long axis length and the length of the axis normal to the long axis. While such techniques did not perfectly replicate the population due to differential burial depths (thus affecting size reconstructions), they did allow us to more accurately replicate Ediacaran populations for CFD analyses than what had been presented elsewhere (Ghisalberti et al., 2014; Gibson et al., 2019). In both sets of analyses, all sizes fell within the published ranges of Ernietta sizes (Gibson et al., 2019).
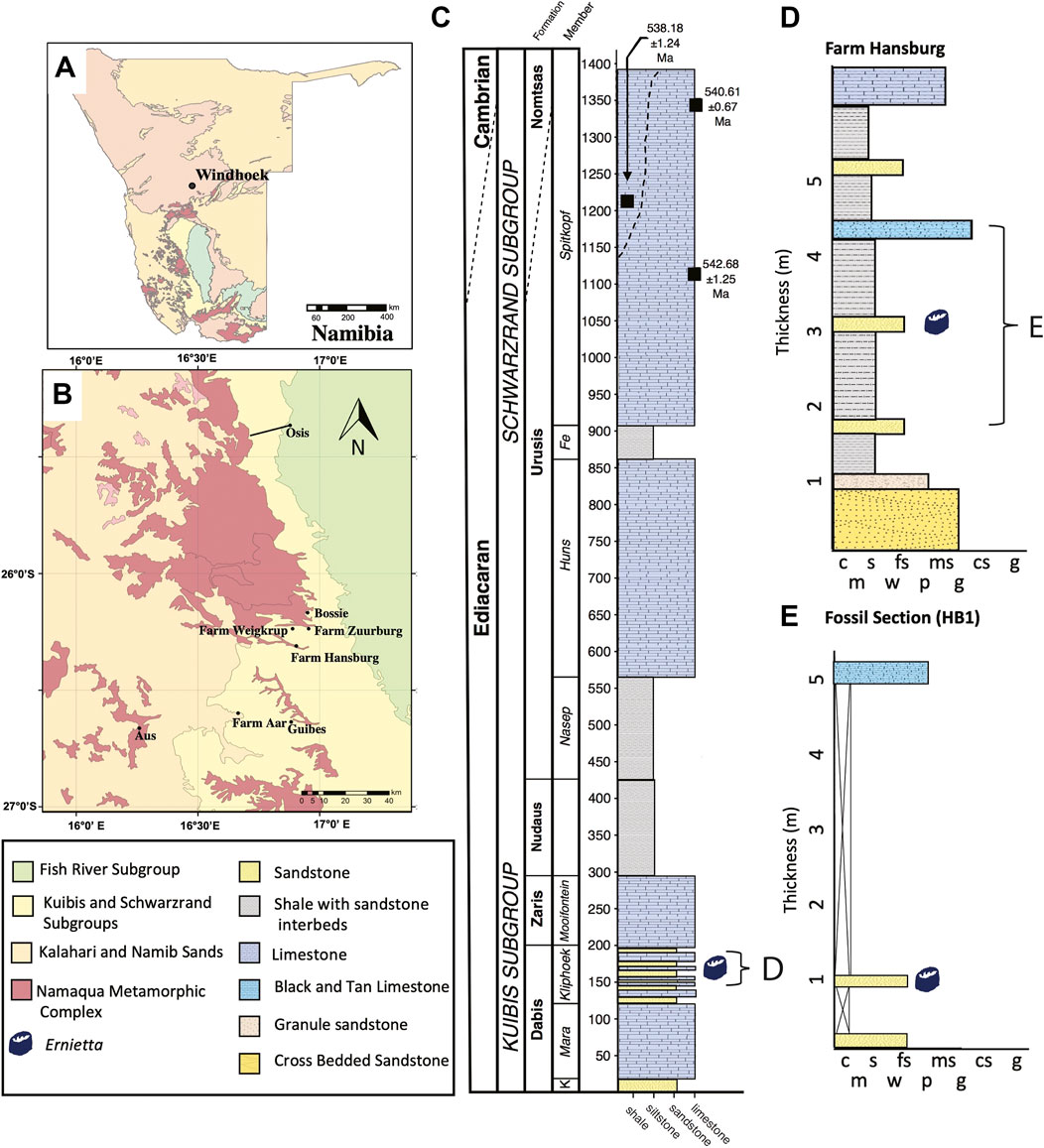
FIGURE 1. Geologic Setting. (A) Map of Namibia showing the distribution of subgroups within the Nama Group. (B) Geologic map showing the study site, Farm Hansburg and other known Ediacaran fossil localities in southern Namibia. (C) Stratigraphy of the Kuibis and Schwarzrand Subgroups in the southern Witputs Subbasin. K-Kaines Member, Fe-Feldschuhhorn (D) Representative log of the stratigraphy at Farm Hansburg. (E) Stratigraphic log of the fossil section at Farm Hansburg where Ernietta slabs were recovered. (Adapted from Maloney et al., 2020).
Because CFD is a computationally intensive technique, we optimized our simulation setups and methods employed based on the question of interest. For all idealized population simulations, we used stationary (time-averaged) Reynolds-Averaged Navier-Stokes (RANS) simulations with the Spalart-Allmaras one-equation eddy viscosity turbulence model (Bardina et al., 1997; Blazek, 2001) as described in Idealized Populations CFD Protocol (Reynolds-Averaged Navier-Stokes). For our Hansburg population analysis, we employed both Spalart-Allmaras RANS and Large Eddy Simulation (LES) turbulence closures using the methods described in Hansburg Population CFD Protocol (Reynolds-Averaged Navier-Stokes and Large Eddy Simulation). Because LES is more accurate and time-dependent, it allowed for more precisely resolved fluid flow patterns in the more complex population setup; however, the time required to solve LES simulations limited its use in idealized populations. For a more in-depth discussion the benefits of LES over RANS, see Gibson et al. (2021). For a more in-depth discussion of turbulence models in general, see Blazek (2001). All CFD simulations were performed in COMSOL Multiphysics 5.6 (COMSOL Multiphysics, RRID:SCR_014,767).
Idealized Populations CFD Protocol (Reynolds-Averaged Navier-Stokes)
CFD simulations using our idealized arrays were set up using a hexahedron flow domain (1.75 m × 0.71 m × 0.25 m; W x D x H) that was sufficiently large to reconstruct flow patterns without influence from boundaries. Following previous setups (e.g., Rahman, 2017), our seafloor and Ernietta individuals were assigned no-slip conditions (e.g., fixing the velocity at 0 m/s), the upper face and flow-parallel faces were assigned slip conditions, and the inlet and outlet conditions were assigned on the upstream and downstream faces, respectively. For the inlet boundary condition, a fully-developed, depth-averaged velocity was prescribed (Gibson et al., 2021), and a zero-pressure condition was assigned to the outlet. We chose U = 0.1, 0.2, 0.5 m/s following previous Ernietta CFD analyses (Gibson et al., 2019). Liquid water material properties [density
Hansburg Population CFD Protocol (Reynolds-Averaged Navier-Stokes and Large Eddy Simulation)
Recent analyses have demonstrated that LES can produce fluid flow results that are more accurate than the widely-employed RANS approach (Gibson et al., 2021). This improvement stems from solving the Navier-Stokes equations without implementing a Reynolds stress tensor (Blazek, 2001). For this reason, we used COMSOL’s LES residual-based variational multiscale (RBVM) turbulence closure on the Hansburg population CFD simulation. For computational ease, we initiated our LES simulations with a previously computed stationary RANS solution following the protocol presented in Idealized Populations CFD Protocol (Reynolds-Averaged Navier-Stokes). We used a hexahedron flow domain (150 cm × 80 cm × 15 cm; W × D × H) that was sufficiently large to reconstruct flow patterns with minimal influence on the population from boundaries in the initial stationary simulation. Following Idealized Populations CFD Protocol (Reynolds-Averaged Navier-Stokes), we applied the same boundary conditions, fluid material properties, and performed a mesh sensitivity analysis (Supplementary 2). Following the idealized population methods, we used the simpler Spalart-Allmaras turbulence closure to solve the flow fields that were used to speed the convergence of the LES simulation. Using the mesh-independent solution (“Normal”; Supplementary Figure S2), we integrated pressure (p) on the upstream and downstream faces to calculate the pressure differential (
Using the same flow domain and mesh procedures described in Idealized Populations CFD Protocol (Reynolds-Averaged Navier-Stokes), we modified our design for the LES analysis by changing the flow-parallel hexahedron slip faces that are orthogonal to the no slip seafloor to be periodic boundaries with a pressure differential
Results
Individual Results
As shown in Figure 2, both the small and large Ernietta individual flow patterns show patterns consistent with CFD descriptions elsewhere (Gibson et al., 2019; 2021). In the small individual simulations, flow enters the cavity from above, is directed to the downstream interior face, and is then forced upstream toward the interior and upstream face, where it is finally directed out of the cavity by mixing with the current above the cavity. A wake of slower, more turbulent water is formed directly downstream of the individual. These patterns are stronger or weaker with faster and slower far field velocities, respectively. The larger individual developed the same patterns of recirculation within the cavity and downstream with a similar relationship between recirculation speed and far field velocity, but the velocities and mixing were greater than those experienced by the smaller individual.
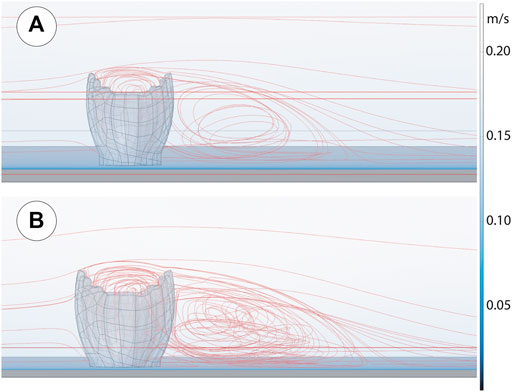
FIGURE 2. Ernietta fluid flow solution for 0.2 m/s inlet velocity. (A) Small Ernietta that is scaled to 0.5x of Figure 1B. (B) Large Ernietta CFD.
Idealized Populations Results
Figure 3 show the results for each of the idealized population CFD studies. In all simulations, there is recirculation in both large and small individuals in the population simulation, though absolute velocities vary depending on size, location, and proximity to neighbouring individuals. Cavity velocities are always slower than the far field velocities, and there is a higher-pressure region upstream of the population where fluid is directed around and over the aggregate. A wake is also formed downstream of the population in all simulations.
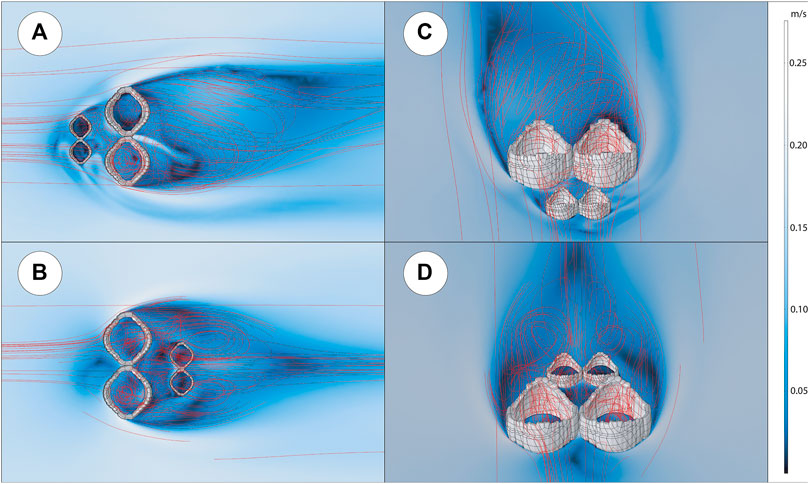
FIGURE 3. Idealized Ernietta population computational fluid dynamics results for inlet velocity of 0.2 m/s. Small Ernietta scaled to 0.5x of large Ernietta. (A) XY view of population with small Ernietta upstream. (B) XY view of population with large Ernietta upstream. (C) Upstream, angled view of population with small Ernietta upstream. (D) Upstream, angled view of population with small Ernietta downstream.
Smaller Upstream
Slower velocities are observed in cavities of the smaller individuals found upstream (Figures 3A,C), while faster velocities are observed in the larger, downstream ones. At the slowest far field velocity (0.1 m/s), small cavities are mostly stagnant upstream. This stagnation decreases when the prescribed flow is increased (0.2 m/s and further at 0.5 m/s). In the larger downstream individuals, flow velocities are faster with minimal stagnation in the lower region of the cavity, regardless of far field velocity. In between the upstream and downstream individuals, there are areas of recirculation that increase in velocity as the far field velocity increases. Vortex shedding occurs on the downstream exterior surfaces, where eddies form and travel downstream in an alternating von Kármán vortex street fashion. As these are stationary solutions, this asymmetry likely results from individual placement as well as asymmetry within the individual Ernietta model.
Smaller Downstream
When smaller Ernietta are placed downstream of larger individuals (Figures 3B,D), consistent cavity recirculation is still present in both upstream and downstream individuals. On average, the larger, upstream individuals experience faster cavity velocities than downstream smaller individuals with a low velocity core within the larger individuals’ cavities. Downstream individuals experience this same pattern at reduced velocities. The viscous sublayer and turbulent boundary layer are disrupted by the large, upstream individuals, where flow is directed between and around these individuals. Vortices are shed off of the downstream face of the larger upstream individuals, where flow then reattaches to the no-slip seafloor downstream of the smaller individuals. This leads to a recirculation of water upstream towards the smaller individuals as evidenced by the streamlines. Lastly, there is less asymmetry in the downstream wake compared to the simulations where the smaller Ernietta are translated upstream of the larger individuals.
In-Situ Hansburg Population Results
Figure 4 and Supplementary Figure S4 show that flow patterns within the reconstructed Hansburg slab broadly follow those described for idealized arrays, with some key distinctions. All individuals receive recirculation within their cavities, and these cavity velocities are reduced compared to the far field velocity. While there is consistent mixing within cavities, most fluid is preferentially directed around and above individuals. Slower velocities are observed in upstream individuals, while faster velocities are observed within the aggregations and within the downstream individuals’ cavities. Smaller Ernietta within the aggregate that are located directly downstream of the largest individual consistently receive fast recirculation–faster than even larger individuals located closer to the upstream face of the largest individual (Figure 4; Supplementary Figure S4). Narrow individuals have slower cavity velocities. Eddies are shed off of all individuals, but orientation to flow, individual shape, and individual location dictate how far downstream eddies persist. Narrow individuals that are oriented broad-side into flow typically exhibit rapid mixing, including upstream recirculation directly behind the individual, while the wakes of those individuals that form part of aggregates are typically disrupted by wakes of neighbouring individuals, leading to additional turbulence. Isolated individuals typically develop small vortex streets that are disrupted by individuals further downstream.
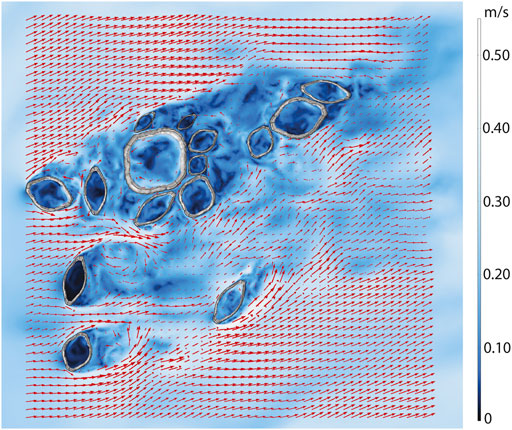
FIGURE 4. Top view of instantaneous (t = 30 s) large eddy simulation computational fluid dynamics flow field results for Farm Hansburg Ernietta population. Arrow sizes are proportional to U magnitude.
Discussion
The effect of individual size on flow patterns varied with the quantity and arrangement of individuals. In single Ernietta simulations, smaller sized individuals developed lower amounts of recirculation. This observation is mirrored in idealized populations, suggesting a potential size control on intra-specific competition, whereby larger individuals should consistently receive greater volumes of fluid required for feeding. However, on the reconstructed Hansburg slab (Figure 4, Supplementary Figure S4) reconstructed flow patterns illustrate that the disadvantage associated with small size is apparently mediated by location within the overall aggregation, suggesting a complex interplay of controls on feeding efficiency, and thus individual success. Below we discuss how the flow pattern results presented here compare with previous CFD studies, both for individuals and idealized populations. We then compare the results of the idealized populations with those of the Hansburg slab to detail ecological signals that emerge from both. Finally, we use these results to address previously suggested hypotheses regarding Ernietta paleobiological reconstructions.
Individuals and Idealized Populations vs Previous Work
Previous CFD analyses on Ernietta individuals and idealized populations investigated how burial depth and individual spacing may have controlled feeding efficiency (Gibson et al., 2019). This work demonstrated that turbulence increased above downstream individuals in addition to generating faster recirculation within the cavities of the same individuals (see Gibson et al., 2019 Figure 3), suggesting that the faciliatory lifestyle exhibited by Ernietta may have imparted a selective advantage for feeding and waste dispersion. While the new CFD results targeting individuals of mixed sizes presented here broadly support the previous results regarding cavity recirculation and suspension feeding, these new data provide additional insights that were not available in the previous analyses. Recirculation is faster within the cavity of larger individuals than in smaller ones, regardless of far field velocity. This indicates that a greater volume of fluid should cycle through larger individuals, potentially delivering larger quantities of food. Also, these new idealized population simulation results partially agree with previous work suggesting that mixing is increased above and within the cavities of downstream individuals. This agreement is strongest for simulations where the larger individuals are downstream of the smaller ones. When smaller individuals were upstream of larger individuals, they received slower velocities than those that were placed downstream (Figure 3). Interestingly, our results demonstrate that the locations of smaller individuals in idealized populations of Ernietta directly affect flow patterns, and thus impact our interpretation of their feeding efficiency. In idealized populations constructed with smaller individuals downstream of larger individuals (Figure 3), smaller individuals experience slower cavity recirculation than the larger individuals (contra Gibson et al., 2019). Based on these comparisons, this would signal that, while vertical mixing is enhanced above aggregations of similarly sized Ernietta, those individuals that are smaller and downstream of larger ones might be flow starved compared to the larger individuals. These idealized populations would then suggest that it might be optimal for Ernietta to form populations of similarly-sized individuals, (i.e., as single cohorts that result from reproduction with spat-fall, however, see below).
Idealized Populations Compared to Hansburg Population
Previous studies have noted that individual Ernietta fossils can vary in shape, with authors attributing these shape variations to a combination of individual growth response to the local environment and/or taphonomy (Elliott et al., 2016; Ivantsov et al., 2016; Smith et al., 2017). In our idealized population models, individuals were identical in shape but were binarily scaled. While cavity velocity magnitudes differed depending on location, the overall recirculation patterns were still comparable between simulations. In contrast, our Hansburg individuals were asymmetrically scaled to better approximate the spatial reconstruction of the Farm Hansburg in-situ population (Supplementary Figure S1). Those results demonstrate cavity and downstream flow patterns are strongly affected by overall individual shape (e.g., slender vs round). Slender individuals receive slower and more asymmetric recirculation compared to rotund individuals. These patterns were present within aggregated and isolated individuals, indicating that overall shape exerts a strong control over internal cavity flow. There may, therefore, have been strong selective pressure for individuals to grow with a more uniform cavity diameter, as evident in many Ernietta deposits (see figures and descriptions from Jenkins et al., 1981; Ivantsov et al., 2016; Elliott et al., 2016; Smith et al., 2017), while cavity filling by deposited sediment would serve to retain the overall oval to circular shape of the cavity.
Our results also show that an individual’s location (or proximity) to neighbors is an equally important control on the generation of flow patterns thought to be important for feeding. Isolated individuals within our Hansburg population experience slower cavity recirculation even when having a more uniform cavity diameter (Figure 4; Supplementary Figure S4), while aggregated individuals experience comparatively faster cavity recirculation. Flow patterns around our individual Ernietta and idealized arrays (Individuals and Idealized Populations vs Previous Work) suggest that smaller individuals experience slower cavity velocities when downstream of larger individuals. However, our Hansburg population results demonstrate that even when smaller individuals were placed immediately downstream of larger individuals, the smaller individuals were constantly exposed to faster velocity flow when compared to isolated forms. At first impression this appears contradictory, but it may highlight the importance of modeling natural systems as opposed to overly simplified (i.e., idealized) ones. Furthermore, our reconstructed population simulations agree with previous results that demonstrated increased mixing above and within downstream cavities (Gibson et al., 2019). This suggests that smaller individuals within aggregates are not hampered when behind a larger individual, but in fact are likely to receive more and better-mixed fluid flow. Thus, while it is less advantageous for an individual to be near the edge of the aggregation, smaller individuals would likely gain access to additional resources that they might not otherwise receive should they be on the edge of the population, or entirely isolated. While little is known about the ontogenetic history of Ernietta, it is reasonable to assume that younger individuals would be smaller; our results suggest that there would thus have been an advantage to settling, or living near, larger individuals within the greater population. In this fashion, aggregations of adult Ernietta would likely have performed a “nursery” function, creating localized conditions favoring the settlement and growth of younger individuals.
This complex interplay of controls on individual feeding success has some parallels in extant benthic marine ecosystems. Studies focused on intraspecific competition arising from spatial density within populations of sessile suspension feeders demonstrate that, in multi-sized aggregations, intraspecific competition can affect both individual size and spatial distribution (Werner and Gilliam, 1984). When different sizes preferentially exploit disparate resources, the competition between adults and juveniles is reduced (Werner and Gilliam, 1984). However, in such cases where exploited resources overlap as is plausible with Ernietta–and size does determine the outcome of competition, younger individuals are often less successful due to metabolic constraints (Peters, 1983). Importantly, the magnitude of this general relationship is greatly influenced by resource availability. While a paucity of resources does typically lead to increased competition, when resources are abundant this can decrease levels of exploitative competition (Hazlett et al., 1975; Werner and Gilliam, 1984). This distinction is important when applying these models to Ediacaran-aged communities in Namibia; recent geochemical work on the Nama Group suggests large scale unstable redox conditions accompanied by decreasing nutrient delivery to inner ramp habitats where Ernietta are typically found (Bowyer et al., 2017; 2020). In combination with the evidence presented here for relatively low-levels of intraspecific competition, this would indicate that populations of Ernietta on Farm Hansburg either: 1) locally received short-lived, adequate nutrient levels to offset multi-sized intraspecific competition; or, 2) Ernietta was an ecological generalist not endemic to oxygenated, nutrient-saturated environments (Darroch et al., 2018b).
Broader Speculation on the Paleobiology of Ernietta
While our simulations were designed as an extension of recent work (Gibson et al., 2019, 2021) with the goal of moving towards reconstructing fluid flow over spatially-accurate 3D populations of Ediacaran taxa, they do allow us to evaluate other hypotheses regarding the paleobiology and life habit of Ernietta. For example, Bouougri et al. (2011) suggested that living Ernietta may have been covered in a slime or mucus layer, based on the orientation of sediment detrital mica flakes associated with fossil molds. While our results cannot support or refute this suggestion, our simulations do at least show that recirculation in the wakes of individual Ernietta would provide one possible mechanism for fine-grained sediment (presumably together with suspended food particles) delivery to downstream surfaces. Given that many modern suspension feeding organisms make use of mucus nets and other structures as a means for capturing food particles (see discussion of aerosol capture mechanisms in Rubenstein and Koehl, 1977; and LaBarbera, 1984), we suggest that an external slime- or mucus-covered surface could have been used to capture suspended particulate material, and which could then have been sorted and/or transported to other feeding organs (see, for example, LaBarbera, 1978). However, without additional evidence for mucus membranes, or indeed any other evidence revealing how food particles are ingested and processed, this model remains highly speculative.
In a similar vein, Ivantsov et al. (2016) suggested that the “apical fan” of Ernietta (i.e., the portion of the organism raised into the water column) may have been used for respiration. While no previous CFD studies have interpreted this anatomy in the context of respiration, many of the flow patterns modelled around Ernietta could have been useful for bringing oxygen-rich water closer to respiratory organs. The increased turbulence modelled in these areas could plausibly have served to replenish dissolved gases, as well as diffuse any exhalents. Although we cannot directly address hypotheses surrounding respiration without substantially adjusting the methods employed here, future coupling of fluid dynamics simulations with oxygen diffusion/uptake models (see, for example, Ghisalberti et al., 2014) may offer an elegant means to test this idea. Future work using this combination of methods could plausibly be used to test the hypothesis that Ernietta was opportunistic, and able to colonize shallow marine environments that were only transiently oxygenated (see Hall et al., 2013; Bowyer et al., 2020).
Summary
Previous paleoecological work focused on the latest Ediacaran Nama interval has suggested that communities of soft-bodied Ediacara biota were relatively depauperate, possessing lower species richness than older Ediacaran communities, as well as having relatively “simple” structures consistent with either competition for a limited number of ecological resources and/or ecological stress (Darroch et al., 2015; 2018a,b). This study adds nuance to this picture, illustrating that, while species poor and relatively simple, the structure of Nama-aged erniettomorph communities was likely influenced by a wide-range of intraspecific ecological factors, including size, location, and (potentially) developmental stage. In this study, we show that individual size and location within a population can have large effects on flow within and around the body cavities, affecting their access to nutrients and well-mixed water. Given the lack of any evidence for asexual reproductive modes such as budding in Ernietta, it is reasonable to hypothesize that these factors might have been crucial during the recruitment and settlement of new individuals. In addition, while not directly assessed here, our flow patterns showing increased fluid delivery downstream of larger individuals also suggests a potential ‘nursery’ effect, with competition for space adjacent to larger Ernietta. Many of these density-dependent factors continue to influence the structure of communities in today’s oceans (Caley et al., 1996; Gilmour, 1999; Harrington et al., 2004), and so reinforce the point that, while we still have little idea where taxa like Ernietta fit (if at all) in the metazoan tree of life, in many respects they experienced similar ecological pressures–and may have utilized similar ecological strategies–as modern benthic marine organisms.
Data Availability Statement
The raw data supporting the conclusion of this article will be made available by the authors, without undue reservation.
Author Contributions
All authors collected field measurements and contributed in writing to this work. BMG and ML generated the digital reconstruction, and BMG conducted the fluid simulations.
Funding
BG received funding from the University of Toronto Mississauga Postdoctoral Fellowship Award and Vanderbilt University’s Alberstadt, Reeseman, and Sterns grant. SD acknowledges NSF-NERC EAR 2007928, National Geographic 9968–16, and a Paleontological Society Arthur J. Boucot Award. SD also acknowledges generous support from the Alexander von Humboldt Foundation, which is sponsored by the Federal Ministry for Education and Research in Germany. KM was supported by the Natural Sciences and Engineering Research Council of Canada (NSERC) postgraduate scholarship and the Chemical and Physical Sciences Research Visit Program (University of Toronto Mississauga). ML was funded by the NSERC Discovery Grant (RGPIN 435402) and National Geographic 9241–14.
Conflict of Interest
The authors declare that the research was conducted in the absence of any commercial or financial relationships that could be construed as a potential conflict of interest.
The handling Editor declared a past co-authorship with the authors KM, ML.
Publisher’s Note
All claims expressed in this article are solely those of the authors and do not necessarily represent those of their affiliated organizations, or those of the publisher, the editors, and the reviewers. Any product that may be evaluated in this article, or claim that may be made by its manufacturer, is not guaranteed or endorsed by the publisher.
Acknowledgments
We thank B. Viljoen and L. Viljoen for their hospitality and access to field sites; D. Furbish, M. Schmeeckle, and I. Rahman for fluid mechanics discussions; G. Gualda for access to computational workstations.
Supplementary Material
The Supplementary Material for this article can be found online at: https://www.frontiersin.org/articles/10.3389/feart.2021.749150/full#supplementary-material
References
Bardina, J. E., Huang, P. G., and Coakley, T. J. (1997). Turbulence Modeling Validation, Testing, and Development. NASA Tech. Memorandum 110446, 1–88. doi:10.2514/6.1997-2121
Bertness, M. D., Gaines, S., Bermudez, D., and Sanford, E. (1991). Extreme Spatial Variation in the Growth and Reproductive Output of the Acorn Barnacle Semibalanus Balanoides. Mar. Ecol. Prog. Ser. 75, 91–100. doi:10.3354/meps075091
Bertness, M. D., and Grosholz, E. (1985). Population Dynamics of the Ribbed Mussel, Geukensia Demissa: the Costs and Benefits of an Aggregated Distribution. Oecologia 67, 192–204. doi:10.1007/bf00384283
Boag, T. H., Darroch, S. A. F., and Laflamme, M. (2016). Ediacaran Distributions in Space and Time: Testing Assemblage Concepts of Earliest Macroscopic Body Fossils. Paleobiology 42, 574–594. doi:10.1017/pab.2016.20
Boddy, C. E., Mitchell, E. G., Merdith, A., and Liu, A. G. (2021). Palaeolatitudinal Distribution of the Ediacaran Macrobiota. J. Geol. Soc., jgs2021–030. doi:10.1144/jgs2021-030
Bouougri, E. H., Porada, H., Weber, K., and Reitner, J. (2011). “Sedimentology and Palaeoecology of Ernietta-Bearing Ediacaran Deposits in Southern Namibia: Implications for Infaunal Vendobiont Communities,” in Advances in Stromatolite Geobiology. Editors J. Reitner, N.V. Quéric, and A. Gernot (Berlin, Heidelberg): Springer, 473–506. doi:10.1007/978-3-642-10415-2_29
Bowyer, F. T., Shore, A. J., Wood, R. A., Alcott, L. J., Thomas, A. L., Butler, I. B., et al. (2020). Regional Nutrient Decrease Drove Redox Stabilisation and Metazoan Diversification in the Late Ediacaran Nama Group, Namibia. Sci. Rep. 10, 1–11. doi:10.1038/s41598-020-59335-2
Bowyer, F., Wood, R. A., and Poulton, S. W. (2017). Controls on the Evolution of Ediacaran Metazoan Ecosystems: A Redox Perspective. Geobiology 15, 516–551. doi:10.1111/gbi.12232
Caley, M. J., Carr, M. H., Hixon, M. A., Hughes, T. P., Jones, G. P., and Menge, B. A. (1996). Recruitment and the Local Dynamics of Open marine Populations. Annu. Rev. Ecol. Syst. 27, 477–500. doi:10.1146/annurev.ecolsys.27.1.477
Clapham, M. E., and Narbonne, G. M. (2002). Ediacaran Epifaunal Tiering. Geol 30, 627–630. doi:10.1130/0091-7613(2002)030<0627:eet>2.0.co;2
Clapham, M. E., Narbonne, G. M., and Gehling, J. G. (2003). Paleoecology of the Oldest Known Animal Communities: Ediacaran Assemblages at Mistaken Point, Newfoundland. Paleobiology 29, 527–544. doi:10.1666/0094-8373(2003)029<0527:potoka>2.0.co;2
Darroch, S. A. F., Cribb, A. T., Buatois, L. A., Germs, G. J. B., Kenchington, C. G., Smith, E. F., et al. (2021). The Trace Fossil Record of the Nama Group, Namibia: Exploring the Terminal Ediacaran Roots of the Cambrian Explosion. Earth-Science Rev. 212, 103435. doi:10.1016/j.earscirev.2020.103435
Darroch, S. A. F., Laflamme, M., and Clapham, M. E. (2013). Population Structure of the Oldest Known Macroscopic Communities from Mistaken Point, Newfoundland. Paleobiology 39, 591–608. doi:10.1666/12051
Darroch, S. A. F., Laflamme, M., and Wagner, P. J. (2018a). High Ecological Complexity in Benthic Ediacaran Communities. Nat. Ecol. Evol. 2, 1541–1547. doi:10.1038/s41559-018-0663-7
Darroch, S. A. F., Smith, E. F., Laflamme, M., and Erwin, D. H. (2018b). Ediacaran Extinction and Cambrian Explosion. Trends Ecol. Evol. 33, 653–663. doi:10.1016/j.tree.2018.06.003
Darroch, S. A. F., Sperling, E. A., Boag, T. H., Racicot, R. A., Mason, S. J., Morgan, A. S., et al. (2015). Biotic Replacement and Mass Extinction of the Ediacara Biota. Proc. R. Soc. B. 282, 20151003. doi:10.1098/rspb.2015.1003
Doropoulos, C., Evensen, N. R., Gómez-Lemos, L. A., and Babcock, R. C. (2017). Density-dependent Coral Recruitment Displays Divergent Responses during Distinct Early Life-History Stages. R. Soc. Open Sci. 4, 170082. doi:10.1098/rsos.170082
Droser, M. L., Tarhan, L. G., and Gehling, J. G. (2017). The Rise of Animals in a Changing Environment: Global Ecological Innovation in the Late Ediacaran. Annu. Rev. Earth Planet. Sci. 45, 593–617. doi:10.1146/annurev-earth-063016-015645
Elliott, D. A., Trusler, P. W., Narbonne, G. M., Vickers-Rich, P., Morton, N., Hall, M., et al. (2016). Ernietta from the Late Edicaran Nama Group, Namibia. J. Paleontol. 90, 1017–1026. doi:10.1017/jpa.2016.94
Gaines, S., and Roughgarden, J. (1985). Larval Settlement Rate: a Leading Determinant of Structure in an Ecological Community of the marine Intertidal Zone. Proc. Natl. Acad. Sci. 82, 3707–3711. doi:10.1073/pnas.82.11.370710.1073/pnas.82.11.3707
Gehling, J. G., and Droser, M. L. (2013). How Well Do Fossil Assemblages of the Ediacara Biota Tell Time? Geology 41, 447–450. doi:10.1130/g33881.1
Gehling, J. G. (1999). Microbial Mats in Terminal Proterozoic Siliciclastics: Ediacaran Death Masks. Palaios 14, 40–57. doi:10.2307/3515360
Ghisalberti, M., Gold, D. A., Laflamme, M., Clapham, M. E., Narbonne, G. M., Summons, R. E., et al. (2014). Canopy Flow Analysis Reveals the Advantage of Size in the Oldest Communities of Multicellular Eukaryotes. Curr. Biol. 24, 305–309. doi:10.1016/j.cub.2013.12.017
Gibson, B. M., Furbish, D. J., Rahman, I. A., Schmeeckle, M. W., Laflamme, M., and Darroch, S. A. F. (2021). Ancient Life and Moving Fluids. Biol. Rev. 96, 129–152. doi:10.1111/brv.12649
Gibson, B. M., Rahman, I. A., Maloney, K. M., Racicot, R. A., Mocke, H., Laflamme, M., et al. (2019). Gregarious Suspension Feeding in a Modular Ediacaran Organism. Sci. Adv. 5, eaaw0260. doi:10.1126/sciadv.aaw0260
Gilmour, J. (1999). Experimental Investigation into the Effects of Suspended Sediment on Fertilisation, Larval Survival and Settlement in a Scleractinian Coral. Mar. Biol. 135, 451–462. doi:10.1007/s002270050645
Grazhadankin, D. (2014). Patterns of Evolution of the Ediacaran Soft-Bodied Biota. J. Paleontol. 88, 269–283. doi:10.1666/13-072
Grazhdankin, D. (2004). Patterns of Distribution in the Ediacaran Biotas: Facies versus Biogeography and Evolution. Paleobiology 30, 203–221. doi:10.1666/0094-8373(2004)030<0203:podite>2.0.co;2
Hall, C. M. S., Droser, M. L., Gehling, J. G., and Dzaugis, M. E. (2015). Paleoecology of the Enigmatic Tribrachidium: New Data from the Ediacaran of South Australia. Precambrian Res. 269, 183–194. doi:10.1016/j.precamres.2015.08.009
Hall, M., Kaufman, A. J., Vickers-Rich, P., Ivantsov, A., Trusler, P., Linnemann, U., et al. (2013). Stratigraphy, Palaeontology and Geochemistry of the Late Neoproterozoic Aar Member, Southwest Namibia: Reflecting Environmental Controls on Ediacara Fossil Preservation during the Terminal Proterozoic in African Gondwana. Precambrian Res. 238, 214–232. doi:10.1016/j.precamres.2013.09.009
Harrington, L., Fabricius, K., De'ath, G., and Negri, A. (2004). Recognition and Selection of Settlement Substrata Determine post-settlement Survival in Corals. Ecology 85, 3428–3437. doi:10.1890/04-0298
Hazlett, B. A., Rubenstein, D., and Rittschof, D. (1975). Starvation, Energy Reserves, and Aggression in the Crayfish Orconectes Virlis (Hagen, 1870) (Decapoda, Cambaridae). Crustaceana 28, 11–16.
Hixon, M. A., Pacala, S. W., and Sandin, S. A. (2002). Population Regulation: Historical Context and Contemporary Challenges of Open vs. Closed Systems. Ecology 83, 1490–1508. doi:10.2307/307196910.1890/0012-9658(2002)083[1490:prhcac]2.0.co;2
Ivantsov, A. Y., Narbonne, G. M., Trusler, P. W., Greentree, C., and Vickers-Rich, P. (2016). Elucidating Ernietta : New Insights from Exceptional Specimens in the Ediacaran of Namibia. Lethaia 49, 540–554. doi:10.1111/let.12164
Jenkins, R. J. F. (1992). “Functional and Ecological Aspects of Ediacaran Assemblages,” in Origin and Early Evolution of the Metazoa. Editors J.H. Lipps, and P.W. Signor (New York: Plenum Press), 131–176. doi:10.1007/978-1-4899-2427-8_5
Jenkins, R. J. F., Plummer, P. S., and Moriarty, K. C. (1981). Late Precambrian Pseudofossils from the Flinders Ranges, South Australia. Trans. R. Soc. South Aust. 105, 67–83.
Knoll, A., Walter, M., Narbonne, G., and Christie-Blick, N. (2006). The Ediacaran Period: a New Addition to the Geologic Time Scale. Lethaia 39, 13–30. doi:10.1080/00241160500409223
LaBarbera, M. (1984). Feeding Currents and Particle Capture Mechanisms in Suspension Feeding Animals. Am. Zool 24, 71–84. doi:10.1093/icb/24.1.71
LaBarbera, M. (1978). Particle Capture by a Pacific Brittle star: Experimental Test of the Aerosol Suspension Feeding Model. Science 201, 1147–1149. doi:10.1126/science.201.4361.1147
Laflamme, M., Darroch, S. A. F., Tweedt, S. M., Peterson, K. J., and Erwin, D. H. (2013). The End of the Ediacara Biota: Extinction, Biotic Replacement, or Cheshire Cat? Gondwana Res. 23, 558–573. doi:10.1016/j.gr.2012.11.004
Leonard, G. H., Levine, J. M., Schmidt, P. R., and Bertness, M. D. (1998). Flow-driven Variation in Intertidal Community Structure in a Maine Estuary. Ecology 79, 1395–1411. doi:10.1890/0012-9658(1998)079[1395:fdviic]2.0.co;2
Linden, B., and Rinkevich, B. (2017). Elaborating an Eco-Engineering Approach for Stock Enhanced Sexually Derived Coral Colonies. J. Exp. Mar. Biol. Ecol. 486, 314–321. doi:10.1016/j.jembe.2016.10.014
Liu, A. G., Kenchington, C. G., and Mitchell, E. G. (2015). Remarkable Insights into the Paleoecology of the Avalonian Ediacaran Macrobiota. Gondwana Res. 27, 1355–1380. doi:10.1016/j.gr.2014.11.002
Maloney, K. M., Boag, T. H., Facciol, A. J., Gibson, B. M., Cribb, A., Koester, B. E., et al. (2020). Paleoenvironmental Analysis of Ernietta-Bearing Ediacaran Deposits in Southern Namibia. Palaeogeogr. Palaeoclimatol. Palaeoecol. 556, 109884. doi:10.1016/j.palaeo.2020.109884
Meyer, M., Elliott, D., Schiffbauer, J. D., Hall, M., Hoffman, K. H., Schneider, G., et al. (2014). Taphonomy of the Ediacaran Fossil Pteridinium Simplex Preserved Three-Dimensionally in Mass Flow Deposits, Nama Group, Namibia. J. Paleontol. 88, 240–252. doi:10.1666/13-047
Mitchell, E. G., and Butterfield, N. J. (2018). Spatial Analyses of Ediacaran Communities at Mistaken Point. Paleobiology 44, 40–57. doi:10.1017/pab.2017.35
Mitchell, E. G., Kenchington, C. G., Harris, S., and Wilby, P. R. (2018). Revealing Rangeomorph Species Characters Using Spatial Analyses. Can. J. Earth Sci. 55, 1262–1270. doi:10.1139/cjes-2018-0034
Mitchell, E. G., Kenchington, C. G., Liu, A. G., Matthews, J. J., and Butterfield, N. J. (2015). Reconstructing the Reproductive Mode of an Ediacaran Macro-Organism. Nature 524, 343–346. doi:10.1038/nature14646
Mitchell, E. G., and Kenchington, C. G. (2018). The Utility of Height for the Ediacaran Organisms of Mistaken Point. Nat. Ecol. Evol. 2, 1218–1222. doi:10.1038/s41559-018-0591-6
Muscente, A. D., Bykova, N., Boag, T. H., Buatois, L. A., Mángano, M. G., Eleish, A., et al. (2019). Ediacaran Biozones Identified with Network Analysis Provide Evidence for Pulsed Extinctions of Early Complex Life. Nat. Commun. 10, 911. doi:10.1038/s41467-019-08837-3
Narbonne, G. M. (2005). The Ediacara Biota: Neoproterozoic Origin of Animals and Their Ecosystems. Annu. Rev. Earth Planet. Sci. 33, 421–442. doi:10.1146/annurev.earth.33.092203.122519
Pepin, P. (1991). Effect of Temperature and Size on Development, Mortality, and Survival Rates of the Pelagic Early Life History Stages of marine Fish. Can. J. Fish. Aquat. Sci. 48, 503–518. doi:10.1139/f91-065
Peters, R. H. (1983). The Ecological Implications of Body Size. Cambridge: Cambridge University Press.
Prandtl, L. (1905). “über Flüssigkeitsbewegung bei sehr kleiner Reibung,” in Verhandlungen des III Internationalen Mathematiker Kongresses (HeidelbergLeipzig, 1904, 484–491.
Rahman, I. A. (2017). Computational Fluid Dynamics as a Tool for Testing Functional and Ecological Hypotheses in Fossil Taxa. Palaeontology 60, 451–459. doi:10.1111/pala.12295
Raimondi, P. T. (1990). Patterns, Mechanisms, Consequences of Variability in Settlement and Recruitment of an Intertidal Barnacle. Ecol. Monogr. 60, 283–309. doi:10.2307/1943059
Raymundo, L. J., and Maypa, A. P. (2004). Getting Bigger Faster: Mediation of Size-specific Mortality via Fusion in Juvenile Coral Transplants. Ecol. Appl. 14, 281–295. doi:10.1890/02-5373
Rubenstein, D. I., and Koehl, M. A. R. (1977). The Mechanisms of Filter Feeding: Some Theoretical Considerations. The Am. Naturalist 111, 981–994. doi:10.1086/283227
Seilacher, A., and Gishlick, A. D. (2014). “Vendobionts: Lost Life Forms of Ediacaran Times,” in Morphodynamics. Editors A. Seilacher, and A.D. Gishlick (Boca Raton: CRC Press, Taylor & Francis Group), 133–148. doi:10.1201/b17557-16
Seilacher, A., Grazhdankin, D., and Legouta, A. (2003). Ediacaran Biota: The Dawn of Animal Life in the Shadow of Giant Protists. Paleontological Res. 7, 43–54. doi:10.2517/prpsj.7.43
Seilacher, A. (1992). Vendobionta and Psammocorallia: Lost Constructions of Precambrian Evolution. J. Geol. Soc. 149, 607–613. doi:10.1144/gsjgs.149.4.0607
Shen, B., Dong, L., Xiao, S., and Kowalewski, M. (2008). The Avalon Explosion: Evolution of Ediacara Morphospace. Science 319, 81–84. doi:10.1126/science.1150279
Smith, E. F., Nelson, L. L., Tweedt, S. M., Zeng, H., and Workman, J. B. (2017). A Cosmopolitan Late Ediacaran Biotic Assemblage: New Fossils from Nevada and Namibia Support a Global Biostratigraphic Link. Proc. R. Soc. B. 284, 20170934. doi:10.1098/rspb.2017.0934
Sperling, E. A., Carbone, C., Strauss, J. V., Johnston, D. T., Narbonne, G. M., and Macdonald, F. A. (2016). Oxygen, Facies, and Secular Controls on the Appearance of Cryogenian and Ediacaran Body and Trace Fossils in the Mackenzie Mountains of Northwestern Canada. Geol. Soc. America Bull. 128, 558–575. doi:10.1130/b31329.1
Vaziri, S. H., Majidifard, M. R., Darroch, S. A. F., and Laflamme, M. (2021). Ediacaran Diversity and Paleoecology from central Iran. J. Paleontol. 95, 236–251. doi:10.1017/jpa.2020.88
Vermeij, M. J. A., and Sandin, S. A. (2008). Density-dependent Settlement and Mortality Structure the Earliest Life Phases of a Coral Population. Ecology 89, 1994–2004. doi:10.1890/07-1296.1
Waggoner, B. (2003). The Ediacaran Biotas in Space and Time. Integr. Comp. Biol. 43, 104–113. doi:10.1093/icb/43.1.104
Werner, E. E., and Gilliam, J. F. (1984). The Ontogenetic Niche and Species Interactions in Size-Structured Populations. Annu. Rev. Ecol. Syst. 15, 393–425. doi:10.1146/annurev.es.15.110184.002141
White, J. W. (2007). Spatially Correlated Recruitment of a marine Predator and its Prey Shapes the Large-Scale Pattern of Density-dependent Prey Mortality. Ecol. Lett. 10, 1054–1065. doi:10.1111/j.1461-0248.2007.01098.x
Wood, R. A., Poulton, S. W., Prave, A. R., Hoffmann, K.-H., Clarkson, M. O., Guilbaud, R., Lyne, J. W., Tostevin, R., Bowyer, F., Penny, A. M., and Curtis, A., and (2015). Dynamic Redox Conditions Control Late Ediacaran Metazoan Ecosystems in the Nama Group, Namibia. Precambrian Res. 261, 252–271. doi:10.1016/j.precamres.2015.02.004
Keywords: Ediacara biota, computational fluid dynamics (CFD), large eddy simulation (LES), Spalart-Allmaras, Nama assemblage
Citation: Gibson BM, Darroch SAF, Maloney KM and Laflamme M (2021) The Importance of Size and Location Within Gregarious Populations of Ernietta plateauensis. Front. Earth Sci. 9:749150. doi: 10.3389/feart.2021.749150
Received: 29 July 2021; Accepted: 04 October 2021;
Published: 18 October 2021.
Edited by:
Shuhai Xiao, Virginia Tech, United StatesReviewed by:
Alex Liu, University of Cambridge, United KingdomLinda Satour, University of Oran 2, Algeria
Copyright © 2021 Gibson, Darroch, Maloney and Laflamme. This is an open-access article distributed under the terms of the Creative Commons Attribution License (CC BY). The use, distribution or reproduction in other forums is permitted, provided the original author(s) and the copyright owner(s) are credited and that the original publication in this journal is cited, in accordance with accepted academic practice. No use, distribution or reproduction is permitted which does not comply with these terms.
*Correspondence: Brandt M. Gibson, YnJhbmR0Lm0uZ2lic29uQHZhbmRlcmJpbHQuZWR1; Simon A.F. Darroch, c2ltb24uYS5kYXJyb2NoQHZhbmRlcmJpbHQuZWR1