- 1Department of Earth Sciences, University of Adelaide, Adelaide, SA, Australia
- 2School of Biological Sciences, University of Adelaide, Adelaide, SA, Australia
- 3Water Studies, School of Chemistry, Monash University, Melbourne, VIC, Australia
- 4CSIRO Agriculture and Food, Glen Osmond, SA, Australia
- 5School of Environment and Science, Griffiths University, Southport, QLD, Australia
- 6Future Industry Institute, University of South Australia, Adelaide, SA, Australia
The Coorong Lagoon is a unique hydrological and depositional system at the terminus of the Murray–Darling Basin, the largest river system in Australia. It exhibits large salinity, nutrient, and organic matter gradients, providing a modern analogue to study and validate the use of δ15N and δ13C as tracers of past and contemporary geochemical cycles in estuarine environments. To this end, water and surface sediment samples were analyzed for particulate organic nitrogen (PON) and carbon (POC) concentrations, and the respective δ15N and δ13C signatures of particulate nitrogen and carbon. PON and POC exhibited positive relationships to chlorophyll-a, indicating the dominance of phytoplankton production upon suspended organic matter. There was also a general trend of increasing δ15N of PON (δ15NPON) values and decreasing δ13C of particulate carbon (δ13CPC) values with increasing salinity and eutrophication in the restricted South Lagoon. In a multiple linear regression for δ15NPON, the best two predictors in combination are PON and C:N molar ratio, highlighting the importance of productivity and the type or source of organic matter. For δ13CPC, the best two predictors are total dissolved phosphorus and latitude, suggesting influences from productivity and proximity to the ocean. Sediment δ15N values across the Coorong Lagoon overlap with the δ15NPON in the water column, suggesting that PON derived from algal material represents the main source of nitrogen to lagoon sediments. We hypothesize that limited N loss via denitrification leads to PON being recycled almost exclusively to ammonium, due to low rates of nitrification and dominance of dissimilatory nitrate reduction to ammonium (DNRA). We propose that preferential volatilization of 14N in ammonia increases the δ15N of ammonium assimilated by phytoplankton, thereby increasing the δ15N within suspended organic matter and surface sediment in the South Lagoon. By contrast, the gradient exhibited in δ13CPC data was countered by a relatively constant sedimentary organic carbon δ13C. Data from the Coorong, therefore, suggest that δ15N values in sediments can be used to infer palaeoproductivity in this hypereutrophic and hypersaline depositional environment, however, the measured δ13CPC may be influenced by δ13CDIC or preferential loss of 13C during sedimentation that alter the sedimentary δ13C record of organic carbon.
Introduction
Nitrogen and carbon are bioessential elements and key constituents of living organisms and accumulated organic matter in both marine and terrestrial environments. The isotopic compositions of these elements can provide source and process-related information for both modern and past depositional environments (Casciotti 2016; DeNiro and Epstein 1978; Mettam and Zerkle 2021; Miyake and Wada 1967; Ohkouchi, et al., 2015; Sigman and Casciotti 2001). Nitrogen stable isotope (δ15N) composition in sedimentary archives are used to infer changes in the biogeochemical nitrogen cycle, water column redox, anoxia/euxinia, and nutrient conditions in past marine and coastal environments (e.g., Sigman and Casciotti, 2001; Wang et al., 2018; Cox et al., 2019; Davis et al., 2019; Isaji et al., 2019; Obrist-Farner et al., 2019; Tuite et al., 2019; Zhu et al., 2020). The stable isotope composition of carbon (δ13C) in marine sedimentary archives, including both carbonates and organic sediments, is also interpreted to reflect aquatic productivity and carbon cycling from local to global scales, as well as to assess the relative contributions of terrestrial and marine organic matter, and marine vs. freshwater mixing in past aquatic environments (e.g., Popp et al., 1997; Meyers and Lallier-Verges 1999; Wilson et al., 2005; Lamb et al., 2006). Sediment δ13C records have also been used to determine relative sea level fluctuations in marine, coastal, and estuarine environments (e.g., Chmura and Aharon et al., 1995; Lamb et al., 2006; Goslin et al., 2017). Carbon and nitrogen isotope analyses are often complimented by the molar ratio of C:N within sediments, which represents an index for primary producers in the system, where higher C:N ratios (>20) are usually found in organic matter with a higher lignin or cellulose content, namely, derived from higher plant matter (Elser et al., 2000). By contrast, C:N ratios <10 are more commonly found in algal, bacterial, and cyanobacterial biomass due to the absence of reinforced cell walls and, thus, the greater relative contribution of amino acids and pigments to the total molecular composition (Meyers and Lallier-Verges 1999; Cadd et al., 2018).
Interpretation of δ15N and δ13C from sedimentary archives, however, requires a detailed understanding of the above processes and their impacts on the isotopic fractionation and cycling of nutrient and carbon species in modern marine and coastal systems (Casciotti 2016; Mettam and Zerkle 2021). This is particularly important in hypersaline and eutrophic environments, which are common in coastal and lacustrine settings, where the state and composition of the biological community is strongly affected by changes in the physiochemical parameters (Brookes, et al., 2009; Isaji, et al., 2019; Tweedley, et al., 2019). Nevertheless, to date, only a limited number of studies have examined variability in the δ15N and δ13C of organic matter within modern depositional systems characterized by extreme hypersaline and hypereutrophic conditions (e.g., Chen et al., 2017; Isaji, et al., 2019; Šajnović, et al., 2020). Rather, studies in hypersaline environments have predominantly focused on using δ13C in diatoms, ostracods, microbial mat deposits, and carbonates, or sedimentary facies and evaporate deposits as proxies of palaeoclimate and palaeosalinity (e.g., Sylvestre et al., 2005; Frantz et al., 2014; Schröder et al., 2018; Buongiorno et al., 2019; Cuna-Rodriguez et al., 2020).
The Coorong Lagoon and Murray Mouth Estuary in South Australia, the terminus of the largest river system in Australia (the Murray–Darling Basin), is a Ramsar-listed wetland of international importance. It comprises a unique coastal aquatic and depositional system that exhibits a strong salinity gradient, ranging from fresh in the northern end, which receives riverine inflows to hypersaline in the south (e.g., 3–160 PSU). There is also a progressive increase in total nutrient and organic matter stocks in the more restricted and hypersaline South Lagoon, which reaches hypereutrophic conditions due to reduced freshwater flushing and nutrient retention (Mosley, et al., 2020). The transition to hypereutrophic conditions has caused deposition of organic rich sulfidic black muds in the modern system (Herczeg et al., 2001; Fitzpatrick et al., 2019; Haynes et al., 2019). The natural salinity and eutrophication gradient, existence of both terrestrial and marine water and nutrient sources, and current depositional environment makes the Coorong Lagoon an interesting analogue to aid interpretations of sedimentary δ15N and δ13C proxy records from ancient coastal lagoon systems and euxinic ocean conditions.
The aim of this study is to investigate the variability in the δ15N and δ13C of organic matter in the waters and surface sediments of the Coorong Lagoon in the context of a broad salinity and nutrient gradient. These findings are intended to support the interpretation of δ15N and δ13C data from sedimentary archives rich in organic matter (e.g., organic-rich shales), with implications for ancient hypersaline and hypereutrophic environments in euxinic ocean and semi-restricted coastal settings.
Study area
The Coorong Lagoon is a shallow (∼1.3 m deep) and narrow (∼2 km wide) lagoon system that runs northwest to southeast along the South Australian coast for approximately 110 km, separated from the ocean by a sand barrier (Figure 1). A narrow constriction (∼100 m wide) in the middle of the Coorong Lagoon, at Parnka Point, separates the lagoon into two: the North Lagoon and South Lagoon (Figure 1; Mosley, 2016; Stone et al., 2016; Gibbs et al., 2019).
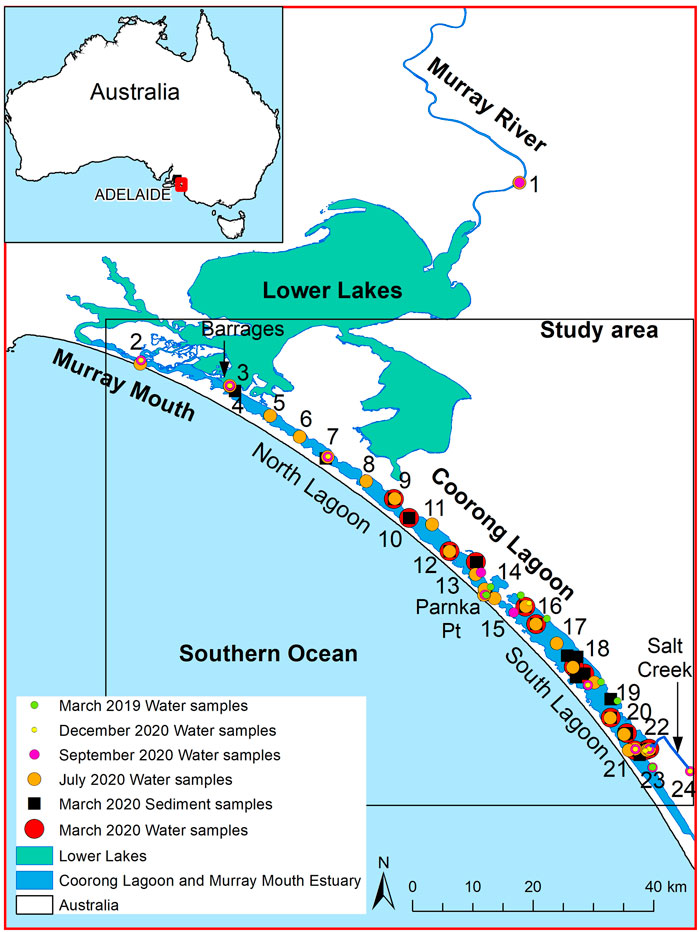
FIGURE 1. Map of the Coorong Lagoon including location of water and sediment sampling sites. Parnka Point is located at site number 14 and Policeman’s point is located at site number 19.
The Murray Mouth, a constricted outlet to the Southern Ocean at the northwestern end of the Coorong Lagoon, allows exchange of seawater into the North Lagoon, influencing water levels in the Coorong Lagoon (Kingsford, et al., 2011). To the east of the Murray Mouth, Murray River waters enter the Coorong Lagoon via the Lower Lakes from regulated barrage structures along the Lower Lakes–Murray Mouth connection, with the Tauwitchere barrage (site 3; Figure 1) being the largest and closest to the Coorong Lagoon (Mosley, 2016). Rising tides and high freshwater flows from the Murray River refill the lagoon and lower salinities throughout the Coorong Lagoon in winter (Brookes et al., 2009; Brookes et al., 2020; Ryan, 2019). Fresh to brackish continental waters also enter the southern end of the endorheic South Lagoon from Salt Creek, however, flows are not sufficient to counter evaporation and to lower salinity in this “terminal” lagoon with no major outflows (Figure 1). Thus, the Coorong operates as a reverse estuary with salinity increasing from the Murray Mouth toward the South Lagoon (Geddes and Butler, 1984; Geddes et al., 2016: Figure 2). While there is a latitudinal gradient, the water column is well mixed vertically with no salinity or other gradients present due to strong wind mixing in the shallow lagoon (Mosley et al., 2019).
Prior to European settlement, there is evidence that the Coorong was less saline, less turbid, and had lower nutrient concentrations compared with the system today (Dick, et al., 2011; Krull, et al., 2009; McKirdy, et al., 2010). Annual sedimentation rates during the Holocene ranged from 1.6 mm in the North Lagoon to <1 mm in the South Lagoon (Haynes et al., 2019). Since European settlement, waters from the Murray–Darling Basin have been diverted, and the rivers within the basin have become highly regulated with streamflow reduced by 60% (Aldridge, et al., 2019; Ryan, 2019).
Currently, the lack of fresh water flushing and evaporation of the lagoon water concentrates salts, nutrients, and other solutes in the water column, especially in the South Lagoon, and has accelerated sedimentation rates. The impact of these reduced flows, land-use changes, and evaporation of the lagoon water has caused increasing trends in salinity, total nutrients, and chlorophyll-a, and a shift to a system dominated by phytoplankton rather than submerged aquatic plants (Herczeg, et al., 2001; Mosley, et al., 2020; Reeves, et al., 2015; Stone, et al., 2016). Consequently, a large proportion of the nutrient inputs from the Murray River and Salt Creek are retained within the lagoon, especially the South Lagoon, and not exported to the ocean (Cook, et al., 2010; Stone, et al., 2016). Thus, the Coorong Lagoon is hypereutrophic with high total nutrient loads and very high algal loads all increasing southward, although dissolved nutrients are low due to rapid uptake by algae (Aldridge, et al., 2019; Ford 2007; Geddes and Butler 1984; Stone, et al., 2016). These impacts have led to a shift in the phytoplankton community from microalgae to cyanobacteria dominated throughout the lagoon, with filamentous green algae blooms becoming an ongoing problem in the South Lagoon (Leterme et al., 2015; Collier et al., 2017; Aldridge et al., 2019). Additionally, sedimentation rates have exponentially increased throughout the Coorong Lagoon due to anoxic preservation of the increased organic matter, and these changes are reflected in the deposition of organic-rich sulfidic black muds since the 1950s (Herczeg et al., 2001; Fitzpatrick et al., 2019; Haynes et al., 2019).
Methods
Sample collection and preservation
Coorong Lagoon water samples were collected in March 2019; and March, July, September, and December 2020, and sediment samples were collected in March 2020. As the water column throughout the Coorong Lagoon is shallow and well mixed, representative water samples were collected from ∼50 cm below the water surface. Onsite measurements of physical and chemical water properties were recorded using a calibrated YSI™ Pro DSS Sonde and handheld meter, including the temperature, dissolved oxygen concentration, salinity, turbidity, and pH. Most samples were collected by boat; however, due to inclement weather and/or boat access issues, some samples were collected by wading from the northern shore. The location of all samples collected in these field trips are shown in Figure 1, and the sampling methods used are specified in Supplementary Table S1.
For isotope analysis of particulate N and C, aliquots of unfiltered water samples were passed separately through PALL type A/E glass fiber filters with a 1-μm pore size. For total dissolved nitrogen (TDN), total dissolved phosphorus (TDP), and NH4+ concentrations, water samples were filtered using 0.2-μm PES syringe filters into acid-cleaned HDPE bottles. All samples including filters containing particulate matter were stored frozen until analysis.
Sediment samples, with a water column of approximately 1 m above, were collected using a “Russian D” auger to a depth of approximately 50 cm. Some shallow shoreline samples were collected with a polycarbonate core tube pushed into the sediment. To investigate the recent transition to hypersaline and eutrophic conditions in the Coorong Lagoon subsamples of the surface (0–2 or 0–5 cm layer), “modern” sediment identified by the organic rich layer, due to rapid mixing in the top up to 5 cm (Krull et al., 2009), were homogenized at the time of sampling for analysis. Samples were immediately placed in sealed vials with no air gap and cooled on ice. Upon return to the laboratory (within 48 h), samples were frozen at −20°C until analysis.
Additionally, Ruppia tuberosa (hereafter Ruppia) and cyanobacteria mat biomass samples were collected from Noonamenna, and Ruppia and filamentous algae samples were collected from Salt Creek sampling sites in March 2020.
Analytical procedure for nutrient concentrations and isotopes in water samples
Analyses for total particulate nitrogen and carbon concentration, 15N/14N ratio and 13C/12C ratio were analyzed from the PALL type A/E glass fibe filters at Monash University analytical and stable isotope facility using a continuous flow isotope ratio mass spectrometer (CF-IRMS; Sercon Ltd., UK; Russell et al., 2018). The C/N molar ratio was estimated based on beam area using internal standards with known amount of carbon and nitrogen along with the samples for each analysis. Previous comparisons between the concentrations of organic carbon and total carbon suspended in Coorong waters indicated that inorganic carbon accounted for a minor (undetectable) fraction of the suspended load. As a consequence, due to the small sample sizes available, and due to the primary focus of this study being the nitrogen isotope system, particulate carbon samples were not acidified prior to isotope analysis. As a consequence, the suspended carbon isotope data reported here are strictly “total particulate carbon;” however, we interpret this signal to primarily reflect the 13C/12C of particulate organic carbon. The isotopic and elemental composition of these samples, as described below, supports this interpretation. Concentrations of NH4+, TDN, and TDP were analyzed at Monash University analytical and stable isotope facility. Nutrient concentrations were quantified spectrophotometrically following the procedures in Standard Methods for Water and Wastewater (APHA 2005), using a Lachat QuikChem 8000 Flow Injection Analyzer (FIA). Samples for TDN and TDP were digested with alkaline persulfate prior to analysis via FIA. Dissolved phosphorus was measured as orthophosphate using method 4500-P G as described in APHA (2005). The accuracy of these analyses was within ±2%. Chlorophyll-a were measured at the Australian Water Quality Centre (AWQC), South Australia, a National Association of Testing Authorities (NATA) accredited laboratory.
Analytical procedure for nutrient concentrations and isotopes in sediment and aquatic plant samples
Total carbon and nitrogen concentrations were measured via high-temperature combustion and infrared detection using a LECO CNS TruMAC Analyzer in the Environmental Analysis Laboratory, Lismore, Australia. Total organic carbon (%Corg) was measured using the same analytical method following pretreatment of the sample with dilute HCl to remove inorganic carbon. The 15N/14N and 13C/12C ratios of sedimentary organic matter were analyzed by EA-IRMS in the Environmental Analysis Laboratory, Lismore, Australia, with HCl pretreatment prior to carbon isotope analysis as above. All carbon and nitrogen isotope data are reported using the standard delta notation, relative to the standards of air for nitrogen and PDB for carbon.
Ruppia, cyanobacteria, and filamentous algae samples were dried at 60°C, pulverized, and weighed into tin cups before being analyzed at Monash University on an ANCA GSL2 elemental analyzer interfaced to a Hydra 20–22 continuous-flow isotope ratio mass spectrometer (Sercon Ltd. UK). Quality control was carried out using four internal standards (ammonium sulfate, sucrose, gelatine, and bream), which were calibrated against internationally recognized reference materials including USGS 40, USGS 41, IAEA N1, USGS 25, USGS 26, and IAEAC-6. The internal standards were used to correct for any variations as a result of peak size linearity and instrumental drift with typical reproducibility of ±0.2‰ for both δ13C and δ15N. Based on these internal standards, the accuracy of our data was calculated to fall within ±0.3‰ for δ15N and ±0.2‰ for δ13C.
Statistical analysis
Processes controlling water column nutrient and POM concentrations were explored through principal component analysis (PCA; Jackson 1992) of the variable latitude, salinity, pH, DO, temperature, turbidity, alkalinity, chlorophyll-a, PON, POC, TOC, DOC, TN, TDN, DON, TKN, TP, TDP, FRP, and C:N molar ratio (Supplemental Analysis and Results; Supplementary Table S1) using the vegan package (Oksanen, et al., 2020) in R. Best subset regression was performed using the regsubsets function in the package leaps3.1 for R (Lumley, 2020) to guide exploratory data analysis to identify the best single and combination of variables that explain variability in δ15NPON and δ13CPC. These proposed relationships were then explored using classical multiple linear regression, with model significance tests conducted using analysis of variance (ANOVA; Chambers and Hastie, 1992), and only models that were significant with variance inflation factors (VIF) close to 1 are kept and presented (Fox and Monette 1992; Fox and Weisberg 2018).
Results
Water column results
There is a general pattern of increasing salinity (Figure 3A), and PON (Figure 3B) and POC concentrations (Figure 3C) from the North Lagoon to the South Lagoon. PON and POC concentrations increase from the North Lagoon (∼0.2 mg L−1 N and ∼1.4 mg L−1 C, respectively) to the South Lagoon (up to 0.9 mg L−1 N and 6.7 mg L−1 C; Figures 3B, C). In the warmer seasons, salinity in the South Lagoon can become concentrated by evaporation to >100 PSU (Figure 3A), however, PON and POC do not appear to increase in concentration to a similar extent (Figure 3D).
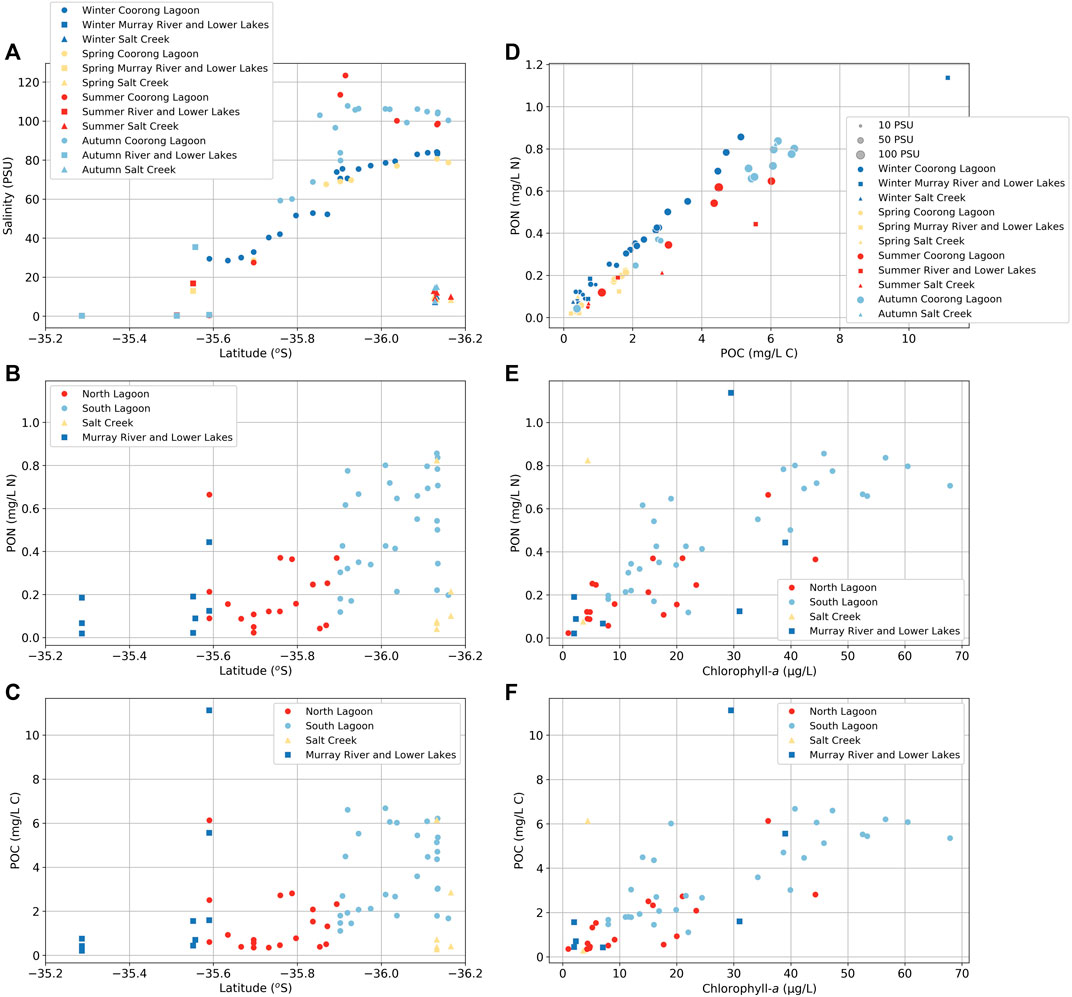
FIGURE 3. (A) Salinity plotted along sample latitude showing sampling season. (B) Particulate organic nitrogen (PON) concentrations and (C) particulate organic carbon (POC) concentrations plotted against sample latitude. (D) PON against POC showing sampling season. (E) PON concentrations and (F) POC concentrations plotted against chlorophyll-a concentrations.
In general, salinity, PON and POC concentrations in the Murray River are low (<1 PSU, <0.2 mg L−1 N, and <0.5 mg L−1 C, respectively), although relatively high PON and POC concentrations occur in the Lower Lakes (upstream of Tauwitchere barrage; up to 1.1 mg L−1 N and 11 mg L−1 C, respectively; Figures 3B, C) and salinities in the Murray Mouth can reach that of seawater (∼35 PSU). Finally, salinity, PON and POC concentrations in Salt Creek are generally low compared with the South Lagoon (<15 PSU, <0.2 mg L−1 N, and <2.8 mg L−1 C, respectively). However, during the March sampling period, PON and POC concentrations were similar to that of the South Lagoon (0.8 mg L−1 N and 6 mg L−1 C, respectively).
C:N molar ratios of POM average 9 ± 4 in the North Lagoon, 9 ± 1 in the South Lagoon, 9 ± 4 in Salt Creek, 8 ± 4 in the Murray River, with larger C:N ratios in the Lower Lakes (14 ± 2) and Murray Mouth (14 ± 8; Supplementary Table S2). These are all higher than the Redfield C:N ratio of 6.6 and represent phytoplankton.
The δ15NPON values average 4.3 ± 2.9‰ (Figure 4A; Supplementary Table S2), and the δ13CPC values average −26.5 ± 1.9‰ in the Coorong (Figure 4B; Supplementary Table S2). The δ15NPON and δ13CPC values in the Coorong show opposite trends with δ15NPON increasing from the North Lagoon to South Lagoon, and δ13CPC values decreasing across the same latitudinal gradient. The average δ15NPON in the South Lagoon (+4.5 ± 1‰) is ∼1‰ higher compared with the North Lagoon (+3.8 ± 2‰; Supplementary Table S2). There is also a 2‰ increase in δ13CPC values from the South Lagoon (−27.4 ± 1.5‰) to the North Lagoon (−25.2 ± 1.8‰; Supplementary Table S2). Lower Lakes δ13CPC values also average −25.1 ± 0.7‰, whereas the Murray River δ13CPC values average −29.3 ± 0.9‰, and the Salt Creek δ13CPC values average −25.3 ± 2‰. The δ15NPON values in Salt Creek and Murray River are (+3.43 ± 1.5‰ and +3.9 ± 3.5‰, respectively). Ruppia from the North Lagoon and Salt Creek regulator had δ13C values of −13.8‰ and −15.3‰, whereas filamentous algae from the Salt Creek regulator had δ13C values of −12.5‰ and a cyanobacterial mat from Noonamenna in the North Lagoon had δ13C values of −18.1‰ (Supplementary Table S2).
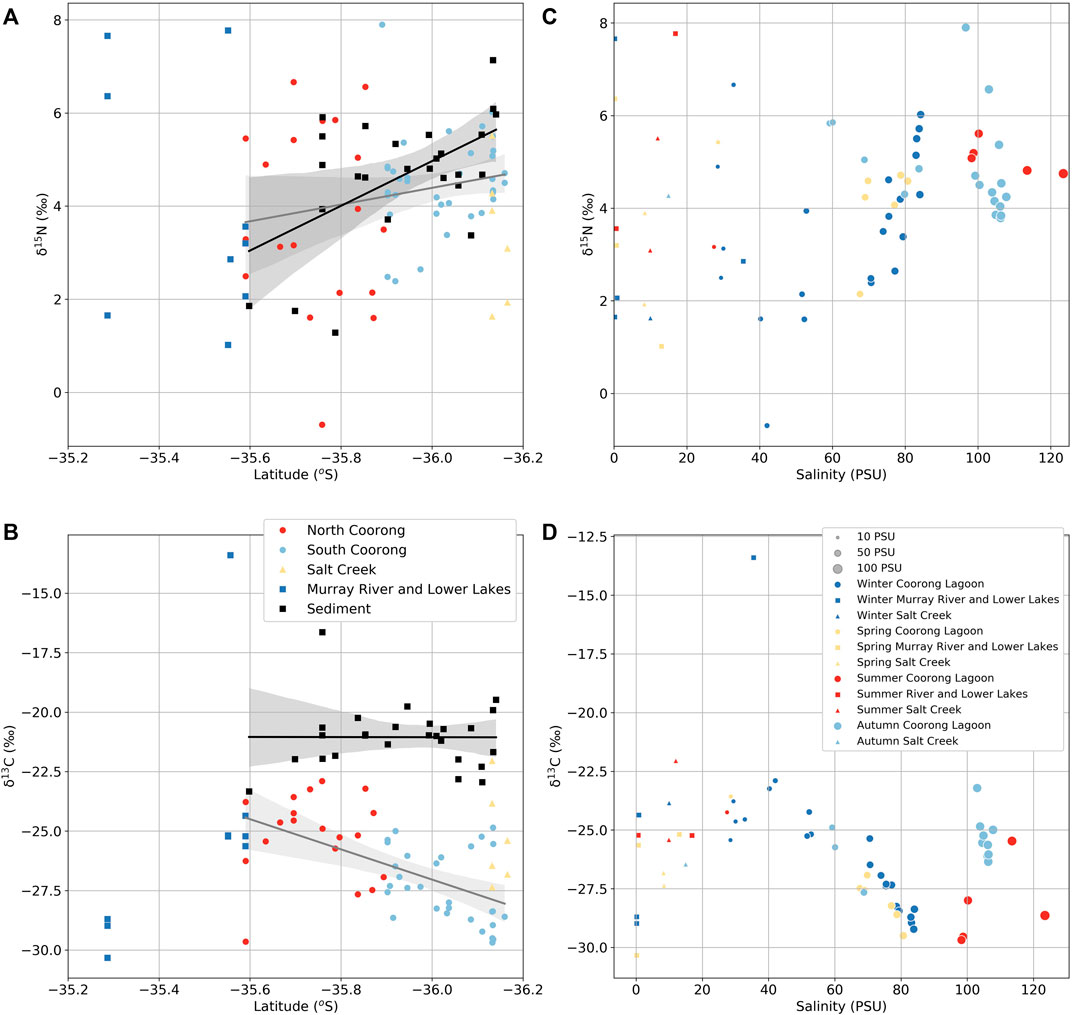
FIGURE 4. (A) PON and bulk sediment δ15N results and (B) particulate carbon (PC) and bulk sediment δ13C results against sample latitude. Legend in (B) applies to (A) as well. (C) δ15NPON results and (D) δ13CPC results against salinity. In both, the gray regression line represents all Coorong Lagoon POM isotope values and black regression line represents the bulk sediment isotope values. Legend in (D) applies to (C) as well.
N and C isotope results for sediments
Total organic carbon and total nitrogen in the South Lagoon sediments (6.6 ± 2.6% and 0.4 ± 0.3%, respectively) are double that of the North Lagoon sediments (1.8 ± 1.8% and 0.2 ± 0.2%, respectively; Supplementary Table S3). The C:N molar ratios of both the North Lagoon and South Lagoon are 9 ± 2 (Supplementary Table S3).
Sedimentary organic matter δ15N values range from +1.3‰ up to +7.1‰ and increase from the North Lagoon (+4.0 ± 1.7‰) to South Lagoon (+5.1 ± 0.9‰; Figure 4A; Supplementary Table S3). By contrast, there is no significant difference between the North Lagoon (−21 ± 1.8‰) and South Lagoon (−21 ± 1.0‰) bulk sediment δ13C values, with the full range from −16.6‰ to −23.3‰ (Figure 4B).
Discussion
Processes controlling water column nutrient and POM concentrations
Salinity increases from north to south along the Coorong Lagoon (Figure 2) with higher concentrations of dissolved ions in summer compared with winter, especially in the South Lagoon (Figure 3A), due to evaporation and the lack of seasonal flushing owing to the Coorong Lagoon being a reverse estuary (Webster 2010). Similar trends to those seen for salinity are also shown for PON, POC (Figures 3B, C), and other nutrient concentrations, such as TN, TOC, and DOC, which also exhibit increasing concentrations from north to south, as previously reported (e.g., Aldridge et al., 2019). Interestingly, the range of variability in PON and POC across the latitudinal gradient is greater than that of salinity, and they do not increase in concentration to a similar extent in summer compared with winter (Figure 3D). Instead, the variability in PON and POC across the lagoon primarily reflects phytoplankton biomass, as reflected by the correlation with chlorophyll-a concentration (Figures 3E, F), whereby biomass is higher in the South Lagoon (Mosley et al., 2019; Mosley et al., 2020). Carbon: chlorophyll-a ratios of ∼50:1 support this interpretation, and that of Ford (2007), who found that most of the POC in the Coorong can be accounted for by phytoplankton production (Reynolds 1997). Additionally, the C:N molar ratios (9 ± 3; Supplementary Table S2) of POM throughout the North and South Lagoons are consistent with predominantly phytoplankton-derived suspended organic matter (cellulose, lipid, and protein, etc.; Cadd et al., 2018; Huang et al., 2020; Meyers and Lallier-Vergès 1999; Tyson 1995).
Principal components analysis (PCA) of the water chemistry data shows that chlorophyll-a, PON, POC, DON, TDN, TKN, TN, TOC, and salinity all covary with the first principal component (Figure 5). This confirms that nutrient concentrations are the primary driver for productivity and, hence, PON and POC concentrations in Coorong waters. Additionally, covariation of salinity with nutrient, chlorophyll-a, and POM concentrations highlights that all are primarily controlled by the lack of flushing. Thus, the overall increase in productivity and nutrient concentrations from north to south in the Coorong appears to primarily be controlled by the lack of flushing due to hydrological restriction of the South Lagoon that acts as an endorheic system, or a “closed basin” with no major outflows.
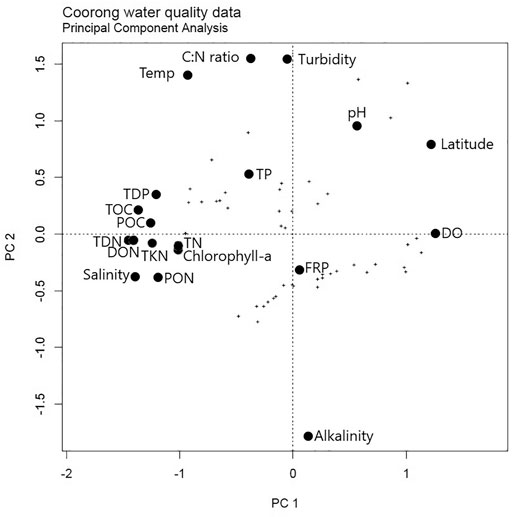
FIGURE 5. Principal component analysis (PCA) results for the Coorong Lagoon water dataset (Supplementary Table S1).
A lack of flushing ensures that the nutrients, suspended load, and sediments are inefficiently removed from the lagoon system into the ocean, further exacerbating their progressive accumulation in the South Lagoon. The reduced amount of flushing over recent years is linked to the shallowing of the South Lagoon due to enhanced evaporation, and physical restrictions caused by the buildup of sediments in the narrower channels of the Coorong (Haynes et al., 2019). The high salinity also inhibits macroinvertebrate survival and bioturbation (Dittmann et al., 2015; Remaili et al., 2018), thus promoting anoxia and sulfate reduction in the surface sediments, especially in the South Lagoon. Thus, we hypothesize that this lack of flushing and restriction of the South Lagoon are one of the fundamental causes of its salinization, the accumulation of nutrients, enhanced productivity, and hence, the buildup of particulate organic matter, all of which contribute to local sediment anoxia, especially in the South Lagoon (Mosley et al., 2020).
Processes controlling POM isotope values
The combined effects of flushing, salinization, and eutrophication also appear to affect the δ15NPON and δ13CPC isotope values throughout the entire Coorong Lagoon (Figure 4). There is a general decrease in δ13CPC from north to south with increasing salinity (Figures 4B, D). However, the δ13CPC values of samples near Salt Creek are higher (∼26‰) compared with the rest of the South Lagoon samples (Figures 4B, D). Likewise, there is a subtle increase in average δ15NPON from north to south with increasing salinity (Figures 4A, C).
Redundancy analysis (RDA) and best subsets regression were used to preliminarily explore which of the environmental variables in the Coorong best predict variability in δ15NPON and δ13CPC. These observations were then formally tested using multiple linear regression, with models validated using model-to-model ANOVA tests and by rejecting models with variance inflation factors >1.5. The best single predictor of δ15NPON was POC (R2 = 0.15, p = 0.004; Table 1), followed closely by PON (R2 = 0.13, p = 0.006; Table 1). The best two predictors for δ15NPON in a multiple linear regression are PON and C:N molar ratio (R2 = 0.26, ANOVA p-value < 0.001; Table 1), beyond which more complex models failed to meet significance test criteria. This suggests that of the measured variables, phytoplankton biomass or productivity, in addition to the composition of the suspended organic matter—either taxonomic make up or input from other sources—are the principal controls over δ15NPON, although with a large amount of variance unexplained. The correlation between δ15NPON and biomass can be potentially explained by the higher productivity and recycling of nitrogen between the sediment and water column in the South Lagoon. Additionally, changes in nitrification/denitrification, as well as the Rayleigh fractionation of nitrogen during assimilation, may also promote an increase in δ15NPON by preferentially removing 14N from the lagoonal water (e.g., Wada 1980; Zanden and Rasmussen 1999; Ohkouchi et al., 2015). Overall, our data from the Coorong Lagoon suggest that variability in suspended PON concentration and δ15NPON are best interpreted as tracers of productivity and associated nitrogen cycling.
The best single predictor of δ13CPC is total Kjeldahl nitrogen (TKN), a measure of the total concentration of organic nitrogen and ammonia (R2 = 0.35, p-value < 0.001; Table 1). In a multiple linear regression, the best two predictors in combination are TDP and latitude (R2 = 0.44, ANOVA p-value = 0.006; Table 1). Broadly speaking, this relationship between δ13CPC, TDP, and latitude is best explained by proximity to marine carbon sources, in combination with a complex array of factors linked to nutrient driven productivity (Krull, et al., 2009; McKirdy, et al., 2010). The influence of marine–freshwater mixing on δ13CPC values is manifested by the higher, marine-like δ13C values near the Murray Mouth (Middelburg and Nieuwenhuize 1998), which decline with both increasing or decreasing salinity (Figure 4D). This marine effect is likely countered by possible kinetic isotopic fractionation during CO2 hydration and hydroxylation during periods of intense productivity in hypersaline waters, which could contribute to observed 13C-depleted DIC values and the trend of the decreasing δ13CPC at salinities between 40 and 100 PSU (Figure 4D) (Clark, et al., 1992; Lazar and Erez 1992). The higher δ13CPC values of the hypersaline (>100 PSU) South Lagoon in summer and autumn sampling periods are more readily interpreted in the context of eutrophication and changes in phytoplankton composition as progression to filamentous algae (δ13CPC = −12.5‰) dominance during high evaporation periods in the South Lagoon (Leterme et al., 2015; Collier et al., 2017) could lead to increased δ13CPC. Additionally, higher δ13CPC values could be due to decreased photosynthetic carbon isotope fractionation at higher phytoplankton growth rates (e.g., Laws et al., 1995; Zanden and Rasmussen 1999; Ohkouchi et al., 2015). Nevertheless, several other potential mechanisms and processes may contribute to the relationship between δ13CPC and nutrient concentration, which require further investigation. For example, in organic rich sediments, higher δ13CPC can be linked to microbial mineralization of detrital organic matter, leading to the release of 13C enriched carbon into the water column. In addition, it is known that the South Lagoon receives a considerable amount of dissolved Sr from Salt Creek and/or via groundwater inputs (Shao, et al., 2018; Shao, et al., 2021), and thus it is also plausible that such alkaline continental water inputs also contain 13C-enriched DIC, which may thus contribute to the higher δ13CPC observed in the southernmost part of the Coorong Lagoon (Figure 4D). Future research into δ13C of DIC is required to verify this hypothesis.
Processes controlling sediment N and C isotope values
Before delving into the sediment δ15N and δ13C results, it is important to note that the South Lagoon sediments have a greater proportion of total organic carbon and total nitrogen than those in the North Lagoon (Supplementary Table S3), reflecting higher net deposition of organic matter in the South Lagoon (Haynes et al., 2019). In fact, the total organic carbon contents in the South Lagoon sediments are approaching those of black shales.
It has been determined above that water column δ15NPON and δ13CPC values, and the total organic carbon and total nitrogen in the sediment, throughout the Coorong Lagoon are affected by nutrient concentrations and associated productivity, as well as proximity to the ocean. However, for sediment-derived δ15N and δ13C proxy signals to be useful tracers of the above processes in palaeolagoon or coastal systems, it is essential to understand the preservation and “transfer” of δ15NPON and δ13CPC signals into a local sediment.
Comparison of δ15NPON and sediment δ15N values (Figure 4A) shows that generally sediment δ15N overlaps with the “long-term” average δ15NPON suggesting autochthonous phytoplankton-derived nitrogen represents the primary source of N to the lagoon sediment. This is consistent with algal deposition from a highly eutrophic water column (Pérez-Ruzafa, et al., 2019) and previous research using nitrogen and carbon isotopic biomarkers (Krull et al., 2009; McKirdy et al., 2010). In fact, McKirdy et al. (2010) found South Lagoon sediments to be continuously richer in total nitrogen reflecting higher productivity and hence net deposition of organic matter and nutrients compared with the North Lagoon during the mid-to-late Holocene. They also found a decrease in δ15N in the upper ∼50 cm due to a greater contribution by halotolerant cyanobacteria, and this is also preserved in our data with an increase in sediment δ15N from 3.9‰ to 5.5‰ from 0–2 to 5–10 cm from a site in the North Lagoon (Supplementary Table S3).
Currently, it is likely that the sediment δ15N values are not significantly altered from the δ15NPON values due to the shallow water column (Tesdal, et al., 2013). Nitrification of ammonium is also likely severely limited in the oxygen-poor, organic matter and sulfide-rich sediments, as nitrifying bacteria have a lower affinity for oxygen than aerobic heterotrophs and other chemoautotrophs and are outcompeted by these under oxygen-limited conditions (Kemp, et al., 1990). This effect would be reinforced by the extremely low macroinvertebrate diversity and abundance in the South Lagoon sediments (Dittmann, et al., 2015; Tweedley, et al., 2019), as the presence of macrofauna typically enhances benthic nitrification by increasing sediment oxygenation and the provision of aerobic niches for nitrifying bacteria in their burrow wall sediments (Welsh, 2003; Stief, 2013). Limited nitrification rates would also intrinsically limit N-losses from the lagoon as gaseous products via denitrification and competition with the alternative nitrate reduction process dissimilatory nitrate reduction to ammonium (DNRA) would further decrease N-loss (An and Gardner 2002; Ford 2007; Valiente et al., 2022). DNRA competes with denitrification and, therefore, limits N-loss as N2 by recycling the nitrate produced from ammonium by nitrification back to ammonium leading to enhanced retention of nitrogen in the system (e.g., Burgin and Hamilton 2007; Giblin et al., 2013; Hardison, et al., 2015; Magri, et al., 2020). The organic matter-rich, highly reduced sediments that are present in the South lagoon would favor DNRA over denitrification as a nitrate reduction process (An and Gardner, 2002; Nizzoli et al., 2006; Molnar et al., 2013). Thus, overall benthic N-cycling is dominated by processes that favor the retention, accumulation, and recycling of N, rather than N-loss processes and thereby supporting ongoing eutrophication of the South Lagoon. This hypothesis is supported by the higher nitrogen content in sediments in the South Lagoon (Supplementary Table S3), as well as high NH4+ concentrations (up to 10 mg L−1: Supplementary Table S1) measured in sediment pore waters in the South Lagoon, which would support high diffusive fluxes of ammonium to the overlying water to fuel phytoplankton production and further PON loads to the sediment. Additionally, in the absence of other quantitatively significant N-loss processes (flushing and denitrification) volatilization of ammonia (NH3) to the atmosphere may be a quantitatively important N-loss process, which would be facilitated by the Coorong water with pH values >8 (Supplementary Table S1). This would also result in isotopic fractionation due to preferential volatilization of light 14NH3 compared with 15NH3 (Li et al., 2012). This would result in the residual dissolved ammonium available for phytoplankton being 15N-enriched and the ammonium regenerated during remineralization of this phytoplankton biomass also being 15N-enriched. Thus, progressively over time repeated cycles of ammonium assimilation and remineralization, isotopic fractionation during ammonia volatilization may have contributed to the heavy δ15N values (5.6‰–6.8‰) in sediment and PON in the South Lagoon, and the overlap in the sediment and PON δ15N values (Figure 4A).
The transfer of δ13CPC variability into the sediment δ13C record appears more complex. The sediment δ13C signatures are consistently higher (5‰–7‰) and less geographically variable compared with δ13CPC (Figure 4B), with the δ13C of sedimentary TOC varying around −21 ± 1‰ (Figure 4B). It is possible that the sediment δ13C values represent a mixture between aquatic plant and algae deposition, since the Ruppia, cyanobacterial mat, and filamentous algae δ13C values range between −12.5‰ and −18.1‰. However, this observation contrasts with the general absence of aquatic plants in the majority of the South Lagoon presently due to hypersalinity and light limitations due to turbidity and phytoplankton blooms (Dick, et al., 2011, Kim, et al., 2013; Aldridge et al., 2019). An alternative interpretation is that terrestrial detritus from areas bordering the lagoons also contributes to the sedimentary pool (Middelburg and Nieuwenhuize 1998). However, isotope signatures for local terrestrial plants (δ13C values between −25‰ and −30‰; Krull et al., 2009) indicate that this mixture cannot entirely explain the observed sediment δ13C values. Also, a previous study at nearby Lake Alexandrina showed that <10% of organic matter preserved in sediments was from terrestrial plants (Herczeg, et al., 2001). Instead, it is also possible that isotopically light carbon could have been lost from the sediment due to methanogenesis or preferential microbial mineralization of 12C. Conversely, if isotopically heavy carbon is delivered to the lagoon sediment as carbonate, whereupon it dissolves in the low pH environment of the sediment, the isotopically heavy CO2 produced could be assimilated by phytoplankton and bacteria. Alternatively, it is possible that the δ13C of sedimentary carbon reflects a contribution by a large mass of recalcitrant carbon (i.e., resistant to decomposition) preserved from the now absent macrophyte communities. However, Krull et al. (2009) found that Ruppia megacarpa (δ13C of −13‰) was the predominant source of organic carbon in the North Lagoon prior to the 1950s. They determined that the upper modern 0–5 cm of sediment organic matter δ13C, which fluctuates around −22 ± 1.2‰ and is identical within error to our analyses of −21 ± 1.3‰ (Supplementary Table S3), is predominantly degraded phytoplankton. In addition, the sediment C:N ratios of 9 ± 2 are consistent with a phytoplankton source (Krull et al., 2009; McKirdy et al., 2010). Thus, it is possible that the δ13CPC measured in this study is influenced by DIC and the sediment organic δ13C carbon is a result of algal deposition similar to the sediment δ15N values. The above interpretations to explain the observed δ13C variability in sedimentary carbon archives across the Coorong Lagoon thus need further process-based investigations to corroborate the validity of these different hypotheses and scenarios.
Implications for palaeo-environmental reconstructions based on δ15N-δ13C proxies
The acquired data from the restricted, hypersaline, and hypereutrophic Coorong Lagoon represent a useful case study to test the sensitivity and fidelity of δ15N and δ13C proxies as tracers of past environmental conditions and their temporal changes in lagoonal and coastal systems. Here we show that the δ15N in both suspended and sedimentary organic matter is sensitive to spatial variability in phytoplankton biomass, which, in turn, is primarily controlled by local nitrogen mineralization or recycling with eutrophication primarily being driven by internal processes not external nutrient inputs. In part, this is linked to the hypersalinity and eutrophic conditions related to lagoon restriction and the associated decrease in flushing of nutrient-rich lagoonal waters, which yielded progressively higher δ15N signatures (up to +8‰) in the South Lagoon. Furthermore, surficial lagoon sediment δ15N values follow the same general pattern as the overlying water column δ15NPON suggesting that processes that determine δ15Ν in the water column are also reflected in the sediments. This is due to a high degree of dominance of internal processes, as N assimilation into PON is being regenerated by breakdown of PON in the sediment. Whereas, if the δ15NPON was driven by external nutrient inputs, then δ15NPON would be intermediate between that of these inputs and the ammonium coming from the sediment. Thus, the correlation between δ15NPON and sediment δ15N values representing modern sediment (Krull et al., 2009) is mostly a function of the unique restricted, hypersaline, and hypereutrophic conditions characteristics of the Coorong Lagoon, and especially for its southern parts. However, there is greater variance in the δ15NPON values compared with the sediment δ15N values suggesting that the sediment is an integration of the relatively long-term signals derived from the water column. Therefore, based on this case study, it would appear that δ15NPON is largely recorded and preserved in the sediment δ15N archives in these types of lagoonal and coastal systems, and that nitrogen isotope analysis of ancient estuarine/lagoon or coastal marine sediments has the potential to be used as a proxy for palaeo-productivity in hypersaline and hypereutrophic depositional systems. These results also have relevance for understanding deep time sedimentary systems, particularly the ancient anoxic (Archean) sediments where organic matter and N may be preserved from the water column sources.
In contrast, the δ13C of the surficial sediments in the Coorong does not directly reflect that of δ13CPC, suggesting the δ13CPC is influenced by variable δ13CDIC. An additional hypothesis is that preferential mineralization of nitrogen over carbon is occurring, where carbon spends relatively more time tied up as sediment organic matter. Additionally, the δ13C of the surficial sediments could be the result of diagenetic alteration on δ13C at sediment–water interface upon the deposition of local organic matter. If the former is the case, it is conceivable that δ13C of sediment organic matter is autochthonous phytoplankton derived carbon. In order to verify these effects more research is needed into the influence of various inputs, sources, and local biogeochemical cycling of both inorganic and organic carbon in the Coorong Lagoon.
Overall, the Coorong Lagoon with its extreme gradient in environmental conditions and processes, and deposition of organic rich sulfidic sediments, can provide potential insights into ancient ocean conditions in relation to nitrogen and carbon isotope patterns under highly sulfidic conditions with no significant bioturbation. Similar conditions are considered to have occurred during ocean anoxic events and the deposition of ancient black shale environments in deep time (Meyer and Kump 2008; Rickard, 2012). Thus, the Coorong Lagoon represents a useful analogue to better understand C and N isotope records and redox conditions during deposition of organic-rich sediments in ancient and redox-stratified basins.
Conclusion
Investigations into contemporary carbon and nitrogen cycles and associated isotope variability are essential for interpreting sedimentary δ15N and δ13C records in the context of past salinity and nutrient conditions in restricted estuarine/lagoonal and coastal systems. To this end, the δ15N and δ13C of suspended particulate matter and underlying sediments were investigated in the Coorong Lagoon, South Australia, to test the effect of eutrophication and increased salinity across a wide natural environmental gradient.
In the highly restricted Coorong Lagoon, the lack of freshwater flushing and high evaporation rates causes hypersalinity and eutrophication of local lagoonal waters, particularly in the South Lagoon. This lack of flushing causes a north–south trend of increasing nutrient load, which is also reflected by increasing POM and chlorophyll-a concentrations due to increased phytoplankton productivity in more restricted and nutrient-rich parts of the lagoon. This nutrient-productivity gradient, in addition to changes in marine–freshwater mixing and differences in organic matter composition, is reflected by both the δ15N and δ13C of suspended particulate matter in the water column.
Importantly, this water column δ15N signal or an isotope gradient of particulate organic matter (δ15NPON) is also effectively transferred and recorded in local sediment archives deposited in the Coorong Lagoon. By contrast, δ13C differs markedly between suspended particulate matter and surficial lagoon sediments, suggesting the influence of either recalcitrant legacy organic matter derived from now absent aquatic macrophytes, or the effects of diagenetic processes and carbon remineralization at the sediment–water interface. Overall, our results suggest that variability in the δ15N of sediment organic matter could provide a robust proxy for palaeo-productivity in the Coorong and/or similar coastal lagoon/estuarine systems elsewhere. It is possible that, similar to the sediment δ15N values, sediment organic δ13C carbon is a result of algal deposition and δ13CPC measured in this study is influenced by variable δ13CDIC. However, if δ13CPC is not influenced by DIC sedimentary organic δ13C signal and archives in this system offers a more complex record of past environmental change, which may be still representative of previous conditions, or instead considerably modified and altered by diagenetic processes.
Data Availability Statement
The original contributions presented in the study are included in the article/Supplementary Material. Further inquiries can be directed to the corresponding author.
Author Contributions
SP, JT, LM, and JF contributed to the conception and design of the study. WW performed the sample analysis. SL, YS, and ZW contributed to the data collection. SP wrote the first draft of the manuscript. All authors contributed to manuscript revision, read, and approved the submitted version.
Funding
This project is funded as part of the South Australian Government’s Healthy Coorong, Healthy Basin Program, which is jointly funded by the Australian and South Australian governments. Initial calibrations and pilot studies on N isotope proxies in Coorong waters and anoxic sediments were also supported via ARC Linkage Grant LP160101353.
Conflict of Interest
The authors declare that the research was conducted in the absence of any commercial or financial relationships that could be construed as a potential conflict of interest.
Publisher’s Note
All claims expressed in this article are solely those of the authors and do not necessarily represent those of their affiliated organizations, or those of the publisher, the editors, and the reviewers. Any product that may be evaluated in this article, or claim that may be made by its manufacturer, is not guaranteed or endorsed by the publisher.
Acknowledgments
The Coorong Lagoon and surrounding lands are the home of the Ngarrindjeri Nations and First Nations of the South East peoples, and we, therefore, pay respect to their elders past and present, and acknowledge the longstanding and continued link between these lands and their traditional owners. The authors would like to also acknowledge the Goyder Institute for Water Research for supporting this project. The Goyder Institute for Water Research is the delivery partner for research components of Healthy Coorong, Healthy Basin, providing independent research to inform future management decisions for the region. The authors would like to thank Dr. Sebastien Lamontagne (CSIRO land and water, Australia) for helpful and productive discussions. Garry Hera-Singh and Glen Hill are kindly thanked for boat driving support and sharing their local knowledge.
Supplementary Material
The Supplementary Material for this article can be found online at: https://www.frontiersin.org/articles/10.3389/feart.2021.727971/full#supplementary-material
References
Aldridge, K., Mosley, L., and Oliver, R. (2019). Water quality in the Coorong Lower Lakes and Murray Mouth. Natural History of the Coorong, Lower Lakes, and Murray Mouth Region (Yarluwar-Ruwe). (Editors) L. Mosley, Q. Ye, S. Sheperd, S. Hemming, and R. Fitzpatrick. Adelaide, South Australia: University of Adelaide Press.
An, S., and Gardner, W. (2002). Dissimilatory Nitrate Reduction to Ammonium (DNRA) as a Nitrogen Link, versus Denitrification as a Sink in a Shallow Estuary (Laguna Madre/Baffin Bay, Texas). Mar. Ecol. Prog. Ser. 237, 41–50. doi:10.3354/meps237041
APHA (2005). Standard Methods for the Examination of Water and Waste Water. Standard Methods for the Examination of Water and Waste Water. Washington DC: American Public Health Association, American Water Works Association, and Water Environment Federation.
Brookes, J., Lamontagne, S., Aldridge, K., Benger, S., Bissett, A., Bucater, L., et al. (2009). An Ecosystem Assessment Framework to Guide Management of the Coorong. Final Report of the CLLAMMecology Research Cluster. An Ecosystem Assessment Framework to Guide Management of the Coorong. Final Report of the CLLAMMecology Research Cluster. Canberra, Australia: CSIRO.
Brookes, J., Aldridge, K., Hipsey, M., Busch, B., Ye, Q., Gibbs, M., et al. (2020). Ecological condition of the Lower Lakes and Coorong. Murray Darling Basin Australia: Its future management. Australia: Elsevier.
Buongiorno, J., Gomez, F. J., Fike, D. A., and Kah, L. C. (2019). Mineralized Microbialites as Archives of Environmental Evolution, Laguna Negra, Catamarca Province, Argentina. Geobiology 17, 199–222. doi:10.1111/gbi.12327
Burgin, A. J., and Hamilton, S. K. (2007). Have We Overemphasized the Role of Denitrification in Aquatic Ecosystems? A Review of Nitrate Removal Pathways. Front. Ecol. Environ. 5, 89–96. doi:10.1890/1540-9295(2007)5[89:hwotro]2.0.co;2
Cadd, H. R., Tibby, J., Barr, C., Tyler, J., Unger, L., Leng, M. J., et al. (2018). Development of a Southern Hemisphere Subtropical Wetland (Welsby Lagoon, South-East Queensland, Australia) through the Last Glacial Cycle. Quat. Sci. Rev. 202, 53–65. doi:10.1016/j.quascirev.2018.09.010
Casciotti, K. L. (2016). Nitrogen and Oxygen Isotopic Studies of the Marine Nitrogen Cycle. Annu. Rev. Mar. Sci. 8, 379–407. doi:10.1146/annurev-marine-010213-135052
Chen, X., McGowan, S., Zeng, L., Xu, L., and Yang, X. (2017). Changes in Carbon and Nitrogen Cycling in a Floodplain lake over Recent Decades Linked to Littoral Expansion, Declining Riverine Influx, and Eutrophication. Hydrological Process. 31, 3110–3121. doi:10.1002/hyp.11254
Chmura, G. L., and Aharon, P. (1995). Stable Carbon Isotope Signatures of Sedimentary Carbon in Coastal Wetlands as Indicators of Salinity Regime. J. Coastal Res. 11, 124–135.
Clark, I. D., Fontes, J.-C., and Fritz, P. (1992). Stable Isotope Disequilibria in Travertine from High pH Waters: Laboratory Investigations and Field Observations from Oman. Geochimica et Cosmochimica Acta 56, 2041–2050. doi:10.1016/0016-7037(92)90328-g
Collier, C., van Dijk, K-J., Erftemeijer, P., Foster, N., Hipsey, M., O'Loughlin, E., et al. (2017). Optimising Coorong Ruppia Habitat: Strategies to Improve Habitat Conditions for Ruppia Tuberosa in the Coorong (South Australia) Based on Literature Review, Manipulative Experiments and Predictive Modelling. Reports to Department of Environment and Natural Resources (DEWNR). (Editor) M. Waycott. Adelaide, South Australia: University of Adelaide, School of Biological Sciences, Adelaide.
Cook, P. L. M., Aldridge, K. T., Lamontagne, S., and Brookes, J. D. (2010). Retention of Nitrogen, Phosphorus and Silicon in a Large Semi-arid Riverine lake System. Biogeochemistry 99, 49–63. doi:10.1007/s10533-009-9389-6
Cox, G. M., Sansjofre, P., Blades, M. L., Farkaš, J., and Collins, A. S. (2019). Dynamic Interaction between basin Redox and the Biogeochemical Nitrogen Cycle in an Unconventional Proterozoic Petroleum System. Sci. Rep. 9, 5200. doi:10.1038/s41598-019-40783-4
Cuña-Rodríguez, C., Piovano, E. L., García-Rodríguez, F., Sylvestre, F., Rostek, F., Bernasconi, S. M., et al. (2020). Paleolimnological Record of the Pampean plains (Argentina) as a Natural Archive of South American Hydroclimatic Variability since the LGM to the Current Warm Period. Quat. Sci. Rev. 250, 106675. doi:10.1016/j.quascirev.2020.106675
Davis, C. V., Ontiveros‐Cuadras, J. F., Benitez‐Nelson, C., Schmittner, A., Tappa, E. J., Osborne, E., et al. (2019). Ongoing Increase in Eastern Tropical North Pacific Denitrification as Interpreted through the Santa Barbara Basin Sedimentary δ 15 N Record. Paleoceanography and Paleoclimatology 34, 1554–1567. doi:10.1029/2019pa003578
DeNiro, M. J., and Epstein, S. (1978). Influence of Diet on the Distribution of Carbon Isotopes in Animals. Geochimica et Cosmochimica Acta 42, 495–506. doi:10.1016/0016-7037(78)90199-0
Dick, J., Haynes, D., Tibby, J., Garcia, A., and Gell, P. (2011). A History of Aquatic Plants in the Coorong, a Ramsar-Listed Coastal Wetland, South Australia. J. Paleolimnol 46, 623–635. doi:10.1007/s10933-011-9510-4
Dittmann, S., Baring, R., Baggalley, S., Cantin, A., Earl, J., Gannon, R., et al. (2015). Drought and Flood Effects on Macrobenthic Communities in the Estuary of Australia's Largest River System. Estuarine, Coastal Shelf Sci. 165, 36–51. doi:10.1016/j.ecss.2015.08.023
Elser, J. J., Fagan, W. F., and Denno, R. F. (2000). Nutritional constraints in terrestrial and freshwater food webs. Nature 408, 578–580.
Fitzpatrick, R. W., Shand, P., and Mosley, L. M. (2019). Soils in the Coorong, Lower Lakes and Murray Mouth Region. Natural History of the Coorong, Lower Lakes, and Murray Mouth Region (Yarluwar-Ruwe). (Editors) L. Mosley, Q. Ye, S. Shepherd, S. Hemming, and R. Fitzpatrick. Adelaide, South Australia: University of Adelaide Press.
Ford, P. W. (2007). Biogeochemistry of the Coorong. Review and Identification of Future Research Requirements. Canberra, Australia: CSIRO.
Fox, J., and Weisberg, S. (20182018). Visualizing Fit and Lack of Fit in Complex Regression Models with Predictor. Effect Plots and Partial Residuals 87, 27. doi:10.18637/jss.v087.i09
Fox, J., and Monette, G. (1992). Generalized Collinearity Diagnostics. J. Am. Stat. Assoc. 87, 178–183. doi:10.1080/01621459.1992.10475190
Frantz, C. M., Petryshyn, V. A., Marenco, P. J., Tripati, A., Berelson, W. M., and Corsetti, F. A. (2014). Dramatic Local Environmental Change during the Early Eocene Climatic Optimum Detected Using High Resolution Chemical Analyses of Green River Formation Stromatolites. Palaeogeogr. Palaeoclimatol. Palaeoecol. 405, 1–15. doi:10.1016/j.palaeo.2014.04.001
Geddes, M., Shiel, R., and Francis, J. (2016). Zooplankton in the Murray Estuary and Coorong during Flow and No-Flow Periods. Trans. R. Soc. South Aust. 140, 1–16. doi:10.1080/03721426.2016.1151497
Geddes, M. C., and Butler, A. J. (1984). Physicochemical and Biological Studies on the Coorong Lagoons, South Australia, and the Effect of Salinity on the Distribution of the Macrobenthos. Trans. R. Soc. South Aust. 108, 51–62.
Gibbs, M., Joehnk, K., Webster, I., and Heneker, T. (2019). Hydrology and Hydrodynamics of the Lower Lakes, Coorong and Murray Mouth. Natural History of the Coorong, Lower Lakes, and Murray Mouth Region (Yarluwar-Ruwe). (Editors) L. Mosley, Q. Ye, S. Shepherd, S. Hemming, and R. Fitzpatrick. Adelaide, South Australia: University of Adelaide Press.
Giblin, A., Tobias, C., Song, B., Weston, N., Banta, G., and Rivera-Monroy, V. (2013). The Importance of Dissimilatory Nitrate Reduction to Ammonium (DNRA) in the Nitrogen Cycle of Coastal Ecosystems. Oceanog 26, 124–131. doi:10.5670/oceanog.2013.54
Goslin, J., Sansjofre, P., Van Vliet-Lanoë, B., and Delacourt, C. (2017). Carbon Stable Isotope (δ13 C) and Elemental (TOC, TN) Geochemistry in Saltmarsh Surface Sediments (Western Brittany, France): a Useful Tool for Reconstructing Holocene Relative Sea-Level. J. Quat. Sci. 32, 989–1007. doi:10.1002/jqs.2971
Hardison, A. K., Algar, C. K., Giblin, A. E., and Rich, J. J. (2015). Influence of Organic Carbon and Nitrate Loading on Partitioning between Dissimilatory Nitrate Reduction to Ammonium (DNRA) and N2 Production. Geochimica et Cosmochimica Acta 164, 146–160. doi:10.1016/j.gca.2015.04.049
Haynes, D., Tibby, J., Fluin, J., and Skinner, R. (2019). Palaeolimnology of the Lower Lakes and Coorong Lagoon. Natural History of the Coorong, Lower Lakes, and Murray Mouth Region (Yarluwar-Ruwe). (Editors) L. Mosley, Q. Ye, S. Shepherd, S. Hemming, and R. Fitzpatrick. Adelaide, South Australia: University of Adelaide Press.
Herczeg, A. L., Dogramaci, S. S., and Leaney, F. W. J. (2001). Origin of Dissolved Salts in a Large, Semi-arid Groundwater System: Murray Basin, Australia. Mar. Freshw. Res. 52, 41–52. doi:10.1071/mf00040
Huang, C., Chen, F., Zhang, S., Chen, C., Meng, Y., Zhu, Q., et al. (2020). Carbon and Nitrogen Isotopic Composition of Particulate Organic Matter in the Pearl River Estuary and the Adjacent Shelf. Estuarine, Coastal Shelf Sci. 246, 107003. doi:10.1016/j.ecss.2020.107003
Isaji, Y., Kawahata, H., Ogawa, N. O., Kuroda, J., Yoshimura, T., Jiménez-Espejo, F. J., et al. (2019). Efficient Recycling of Nutrients in Modern and Past Hypersaline Environments. Sci. Rep. 9, 3718. doi:10.1038/s41598-019-40174-9
Jackson, J. E. (1992). A User’s Guide to Principal Components. A User’s Guide to Principal Components. New York: John Wiley & Sons.
Kemp, W. M., Sampou, P., Caffrey, J., Mayer, M., Henriksen, K., and Boynton, W. R. (1990). Ammonium Recycling versus Denitrification in Chesapeake Bay Sediments. Limnol. Oceanogr. 35, 1545–1563. doi:10.4319/lo.1990.35.7.1545
Kim, D. H., Aldridge, K. T., Brookes, J. D., and Ganf, G. G. (2013). The Effect of Salinity on the Germination of Ruppia Tuberosa and Ruppia Megacarpa and Implications for the Coorong: A Coastal Lagoon of Southern Australia. Aquat. Bot. 111, 81–88. doi:10.1016/j.aquabot.2013.06.008
Kingsford, R. T., Walker, K. F., Lester, R. E., Young, W. J., Fairweather, P. G., Sammut, J., et al. (2011). A Ramsar Wetland in Crisis - the Coorong, Lower Lakes and Murray Mouth, Australia. Mar. Freshw. Res. 62, 255–265. doi:10.1071/mf09315
Krull, E., Haynes, D., Lamontagne, S., Gell, P., McKirdy, D., Hancock, G., et al. (2009). Changes in the Chemistry of Sedimentary Organic Matter within the Coorong over Space and Time. Biogeochemistry 92, 9–25. doi:10.1007/s10533-008-9236-1
Lamb, A. L., Wilson, G. P., and Leng, M. J. (2006). A Review of Coastal Palaeoclimate and Relative Sea-Level Reconstructions Using δ13C and C/N Ratios in Organic Material. Earth-Science Rev. 75, 29–57. doi:10.1016/j.earscirev.2005.10.003
Laws, E. A., Popp, B. N., Bidigare, R. R., Kennicutt, M. C., and Macko, S. A. (1995). Dependence of Phytoplankton Carbon Isotopic Composition on Growth Rate and [CO2)aq: Theoretical Considerations and Experimental Results. Geochimica et Cosmochimica Acta 59, 1131–1138. doi:10.1016/0016-7037(95)00030-4
Lazar, B., and Erez, J. (1992). Carbon Geochemistry of marine-derived Brines: I. 13C Depletions Due to Intense Photosynthesis. Geochimica et Cosmochimica Acta 56, 335–345. doi:10.1016/0016-7037(92)90137-8
Leterme, S. C., Allais, L., Jendyk, J., Hemraj, D. A., Newton, K., Mitchell, J., et al. (2015). Drought Conditions and Recovery in the Coorong Wetland, South Australia in 1997-2013. Estuarine, Coastal Shelf Sci. 163, 175–184. doi:10.1016/j.ecss.2015.06.009
Li, L., Lollar, B. S., Li, H., Wortmann, U. G., and Lacrampe-Couloume, G. (2012). Ammonium Stability and Nitrogen Isotope Fractionations for NH4+-NH3(aq)-NH3(gas) Systems at 20-70°C and pH of 2-13: Applications to Habitability and Nitrogen Cycling in Low-Temperature Hydrothermal Systems. Geochimica et Cosmochimica Acta 84, 280–296. doi:10.1016/j.gca.2012.01.040
Lumley, T. (2020). Package ‘leaps’. Version 3.1. Available at: https://CRAN.R-project.org/package=leaps.
Magri, M., Benelli, S., Bonaglia, S., Zilius, M., Castaldelli, G., and Bartoli, M. (2020). The Effects of Hydrological Extremes on Denitrification, Dissimilatory Nitrate Reduction to Ammonium (DNRA) and Mineralization in a Coastal Lagoon. Sci. Total Environ. 740, 140169. doi:10.1016/j.scitotenv.2020.140169
McKirdy, D. M., Thorpe, C. S., Haynes, D. E., Grice, K., Krull, E. S., Halverson, G. P., et al. (2010). The Biogeochemical Evolution of the Coorong during the Mid- to Late Holocene: An Elemental, Isotopic and Biomarker Perspective. Org. Geochem. 41, 96–110. doi:10.1016/j.orggeochem.2009.07.010
Mettam, C., and Zerkle, A. L. (2021). Nitrogen Isotopes in Deep Time. Cambridge: Cambridge University Press.
Meyer, K. M., and Kump, L. R. (2008). Oceanic Euxinia in Earth History: Causes and Consequences. Annu. Rev. Earth Planet. Sci. 36, 251–288. doi:10.1146/annurev.earth.36.031207.124256
Meyers, P. A., and Lallier-vergés, E. (1999). Lacustrine Sedimentary Organic Matter Records of Late Quaternary Paleoclimates. J. Paleolimnology 21, 345–372. doi:10.1023/a:1008073732192
Middelburg, J. J., and Nieuwenhuize, J. (1998). Carbon and Nitrogen Stable Isotopes in Suspended Matter and Sediments from the Schelde Estuary. Mar. Chem. 60, 217–225. doi:10.1016/s0304-4203(97)00104-7
Miyake, Y., and Wada, E. (1967). The Abundance Ratio of 15N/14N in marine Environments. Rec. oceanographic works Jpn. 9, 176–192.
Molnar, N., Welsh, D. T., Marchand, C., Deborde, J., and Meziane, T. (2013). Impacts of Shrimp Farm Effluent on Water Quality, Benthic Metabolism and N-Dynamics in a Mangrove forest (New Caledonia). Estuarine, Coastal Shelf Sci. 117, 12–21. doi:10.1016/j.ecss.2012.07.012
Mosley, L., Gaget, V., and Furst, D. (2019). Healthy Coorong, Healthy Basin – Water Quality Monitoring Requirements Review. Adelaide. South Australia: Royal Society of South Australia.
Mosley, L., Priestley, S., Brookes, J., Dittmann, S., Farkaš, J., Farrell, M., et al. (2020). Coorong Water Quality Synthesis with a Focus on the Drivers of Eutrophication. Adelaide, South Australia: Goyder Institute for Water Research Technical Report Series No. 20/10.
Mosley, L. (2016). Barrage Release Optimisation Trial August 2015: Assessment of Environmental Outcomes and Achievement of Management Objectives. Adelaide, Australia: University of Adelaide.
Nizzoli, D., Welsh, D., Fano, E., and Viaroli, P. (2006). Impact of Clam and Mussel Farming on Benthic Metabolism and Nitrogen Cycling, with Emphasis on Nitrate Reduction Pathways. Mar. Ecol. Prog. Ser. 315, 151–165. doi:10.3354/meps315151
Obrist-Farner, J., Brenner, M., Curtis, J. H., Kenney, W. F., and Salvinelli, C. (2019). Recent Onset of Eutrophication in Lake Izabal, the Largest Water Body in Guatemala. J. Paleolimnol 62, 359–372. doi:10.1007/s10933-019-00091-3
Ohkouchi, N., Ogawa, N. O., Chikaraishi, Y., Tanaka, H., and Wada, E. (2015). Biochemical and Physiological Bases for the Use of Carbon and Nitrogen Isotopes in Environmental and Ecological Studies. Prog. Earth Planet. Sci. 2, 1. doi:10.1186/s40645-015-0032-y
Oksanen, J., Blanchet, F. G., Friendly, M., Kindt, R., Legendre, P., McGlinn, D., et al. (2020). Vegan Community Ecology Package Version 2.5-7 November 2020.
Pérez-Ruzafa, A., Campillo, S., Fernández-Palacios, J. M., García-Lacunza, A., García-Oliva, M., Ibañez, H., et al. (2019). Long-Term Dynamic in Nutrients, Chlorophyll a, and Water Quality Parameters in a Coastal Lagoon during a Process of Eutrophication for Decades, a Sudden Break and a Relatively Rapid Recovery. Front. Mar. Sci. 6, 26. doi:10.3389/fmars.2019.00026
Popp, B. N., Parekh, P., Tilbrook, B., Bidigare, R. R., and Laws, E. A. (1997). Organic Carbon δ13C Variations in Sedimentary Rocks as Chemostratigraphic and Paleoenvironmental Tools. Palaeogeogr. Palaeoclimatol. Palaeoecol. 132, 119–132. doi:10.1016/s0031-0182(97)00061-8
Reeves, J. M., Haynes, D., García, A., and Gell, P. A. (2015). Hydrological Change in the Coorong Estuary, Australia, Past and Present: Evidence from Fossil Invertebrate and Algal Assemblages. Estuaries and Coasts 38, 2101–2116. doi:10.1007/s12237-014-9920-4
Remaili, T. M., Simpson, S. L., Bennett, W. W., King, J. J., Mosley, L. M., Welsh, D. T., et al. (2018). Assisted Natural Recovery of Hypersaline Sediments: Salinity Thresholds for the Establishment of a Community of Bioturbating Organisms. Environ. Sci. Process. Impacts 20, 1244–1253. doi:10.1039/c8em00092a
Reynolds, C. S. (1997). Vegetation Processes in the Pelagic: a Model for Ecosystem Theory. Xxvii, 371 P. Oldendorf/Luhe, Germany: Ecology Institute, 1997. (Excellence in Ecology No. 9). Price DM 68.00. J. Mar. Biol. Assoc. United Kingdom 77, 919.
Rickard, D. (2012). Chapter 13 - Euxinic Systems. Developments in Sedimentology. (Editor) D. Rickard. Amsterdam, Netherlands: Elsevier.
Russell, D. G., Wong, W. W., and Cook, P. L. M. (2018). Negligible Isotopic Fractionation of Nitrogen within Temperate Zostera Spp. Meadows. Biogeosciences 15, 7225–7234. doi:10.5194/bg-15-7225-2018
Ryan, D. (2019). The Role of Climate in Shaping the Coorong, Lower Lakes and Murray Mouth. Natural History of the Coorong, Lower Lakes, and Murray Mouth Region (Yarluwar-Ruwe). (Editors) L. Mosley, Q. Ye, S. Shepherd, S. Hemming, and R. Fitzpatrick. Adelaide, South Australia: University of Adelaide Press.
Šajnović, A., Grba, N., and Neubauer, F. (2020). Geochemistry of Sediments from the Lopare Basin (Bosnia and Herzegovina): Implications for Paleoclimate, Paleosalinity, Paleoredox and Provenance. Acta Geologica Sinica - English Edition 94, 1591–1618. doi:10.1111/1755-6724.14324
Schröder, T., van 't Hoff, J., Ortiz, J. E., de Torres Pèrez-Hidalgo, T. J., López-Sáez, J. A., Melles, M., et al. (2018). Shallow Hypersaline Lakes as Paleoclimate Archives: A Case Study from the Laguna Salada, Málaga Province, Southern Spain. Quat. Int. 485, 76–88. doi:10.1016/j.quaint.2017.08.006
Shao, Y., Farkaš, J., Holmden, C., Mosley, L., Kell-Duivestein, I., Izzo, C., et al. (2018). Calcium and Strontium Isotope Systematics in the Lagoon-Estuarine Environments of South Australia: Implications for Water Source Mixing, Carbonate Fluxes and Fish Migration. Geochimica et Cosmochimica Acta 239, 90–108. doi:10.1016/j.gca.2018.07.036
Shao, Y., Farkaš, J., Mosley, L., Tyler, J., Wong, H., Chamberlayne, B., et al. (2021). Impact of Salinity and Carbonate Saturation on Stable Sr Isotopes (δ88/86Sr) in a Lagoon-Estuarine System. Geochimica et Cosmochimica Acta 293, 461–476. doi:10.1016/j.gca.2020.11.014
Sigman, D. M., and Casciotti, K. L. (2001). Nitrogen Isotopes in the Ocean. Encyclopedia of Ocean Sciences. (Editor) J. H. Steele. Oxford: Academic Press.
Stief, P. (2013). Stimulation of Microbial Nitrogen Cycling in Aquatic Ecosystems by Benthic Macrofauna: Mechanisms and Environmental Implications. Biogeosciences 10, 7829–7846. doi:10.5194/bg-10-7829-2013
Stone, D., Palmer, D., Hamilton, B., Cooney, C., and Mosley, L. (2016). Coorong, Lower Lakes and Murray Mouth Water Quality Monitoring Program 2009—2016: Summary Report. South Australia: Environment Protection Authority.
Sylvestre, F., Sifeddine, A., Turcq, B., Gil, I. M., Albuquerque, A. L. S., Lallier-Verges, E., et al. (2005). Hydrological Changes Related to the Variability of Tropical South American Climate from the Cabo Frio Lagoonal System (Brazil) during the Last 5000 Years. The Holocene 15, 625–630. doi:10.1191/0959683605hl823rr
Tesdal, J.-E., Galbraith, E. D., and Kienast, M. (2013). Nitrogen Isotopes in Bulk marine Sediment: Linking Seafloor Observations with Subseafloor Records. Biogeosciences 10, 101–118. doi:10.5194/bg-10-101-2013
Tuite, M. L., Williford, K. H., and Macko, S. A. (2019). From Greenhouse to Icehouse: Nitrogen Biogeochemistry of an Epeiric Sea in the Context of the Oxygenation of the Late Devonian Atmosphere/ocean System. Palaeogeogr. Palaeoclimatol. Palaeoecol. 531, 109204. doi:10.1016/j.palaeo.2019.05.026
Tweedley, J. R., Dittmann, S. R., Whitfield, A. K., Withers, K., Hoeksema, S. D., and Potter, I. C. (2019). Chapter 30 - Hypersalinity: Global Distribution, Causes, and Present and Future Effects on the Biota of Estuaries and Lagoons. (Editors) E. Wolanski, J. W. Day, M. Elliott, and R. Ramachandran. Coasts and Estuaries. Amsterdam, Netherlands: Elsevier.
Tyson, R. V. (1995). Sedimentary Organic Matter. Sedimentary Organic Matter. London: Chapman & Hall.
Valiente, N., Jirsa, F., Hein, T., Wanek, W., Prommer, J., Bonin, P., et al. (2022). The Role of Coupled DNRA-Anammox during Nitrate Removal in a Highly saline lake. Sci. Total Environ. 806, 150726. doi:10.1016/j.scitotenv.2021.150726
Wada, E. (1980). Nitrogen Isotope Fractionation and its Significance in Biogeochemical Processes Occurring in marine Environments. Isotope Mar. Chem., 375–398.
Wang, T., Ravelo, A. C., Ren, H., Dang, H., Jin, H., Liu, J., et al. (2018). Nitrogen Isotope Variations in the Northern South China Sea since Marine Isotopic Stage 3: Reconstructed from Foraminifera‐Bound and Bulk Sedimentary Nitrogen. Paleoceanography and Paleoclimatology 33, 594–605. doi:10.1029/2018pa003344
Webster, I. T. (2010). The Hydrodynamics and Salinity Regime of a Coastal Lagoon - the Coorong, Australia - Seasonal to Multi-Decadal Timescales. Estuarine, Coastal Shelf Sci. 90, 264–274. doi:10.1016/j.ecss.2010.09.007
Welsh, D. T. (2003). It's a Dirty Job but Someone Has to Do it: The Role of marine Benthic Macrofauna in Organic Matter Turnover and Nutrient Recycling to the Water Column. Chem. Ecol. 19, 321–342. doi:10.1080/0275754031000155474
Wilson, G. P., Lamb, A. L., Leng, M. J., Gonzalez, S., and Huddart, D. (2005). δ13C and C/N as Potential Coastal Palaeoenvironmental Indicators in the Mersey Estuary, UK. Quat. Sci. Rev. 24, 2015–2029. doi:10.1016/j.quascirev.2004.11.014
Zanden, M. J. V., and Rasmussen, J. B. (1999). Primary consumer δ13C and δ15N and the trophic position of aquatic consumers. Ecology 80, 1395–1404. doi:10.1890/0012-9658(1999)080[1395:pccana]2.0.co;2
Keywords: δ15N, δ13C, particulate organic matter, palaeoenvironment, hypersaline, hypereutrophic, Coorong Lagoon, Australia
Citation: Priestley SC, Tyler J, Liebelt SR, Mosley LM, Wong WW, Shao Y, Woolston Z, Farrell M, Welsh DT, Brookes JD, Collins AS, Keneally C and Farkaš J (2022) N and C Isotope Variations Along an Extreme Eutrophication and Salinity Gradient in the Coorong Lagoon, South Australia. Front. Earth Sci. 9:727971. doi: 10.3389/feart.2021.727971
Received: 20 June 2021; Accepted: 14 December 2021;
Published: 14 February 2022.
Edited by:
Magali Ader, UMR7154 Institut de Physique du Globe de Paris (IPGP), FranceReviewed by:
Naohiko Ohkouchi, Japan Agency for Marine-Earth Science and Technology (JAMSTEC), JapanZhanfei Liu, University of Texas at Austin, United States
Christopher Junium, Syracuse University, United States
Copyright © 2022 Priestley, Tyler, Liebelt, Mosley, Wong, Shao, Woolston, Farrell, Welsh, Brookes, Collins, Keneally and Farkaš. This is an open-access article distributed under the terms of the Creative Commons Attribution License (CC BY). The use, distribution or reproduction in other forums is permitted, provided the original author(s) and the copyright owner(s) are credited and that the original publication in this journal is cited, in accordance with accepted academic practice. No use, distribution or reproduction is permitted which does not comply with these terms.
*Correspondence: Stacey C. Priestley, c3RhY2V5LnByaWVzdGxleUBhZGVsYWlkZS5lZHUuYXU=