- 1Oceanography Department, United States Naval Academy, Annapolis, MD, United States
- 2Air Force Office of Scientific Research, Arlington, VA, United States
- 3Department of Geography, University of Georgia, Athens, GA, United States
- 4Institute of Earth, Ocean, and Atmospheric Sciences, Rutgers, the State University of New Jersey, Newark, NJ, United States
Arctic Amplification is a fundamental feature of past, present, and modelled future climate. However, the causes of this “amplification” within Earth’s climate system are not fully understood. To date, warming in the Arctic has been most pronounced in autumn and winter seasons, with this trend predicted to continue based on model projections of future climate. Nevertheless, the mechanisms by which this will take place are numerous, interconnected. and complex. Will future Arctic Amplification be primarily driven by local, within-Arctic processes, or will external forces play a greater role in contributing to changing climate in this region? Motivated by this uncertainty in future Arctic climate, this review seeks to evaluate several of the key atmospheric circulation processes important to the ongoing discussion of Arctic amplification, focusing primarily on processes in the troposphere. Both local and remote drivers of Arctic amplification are considered, with specific focus given to high-latitude atmospheric blocking, poleward moisture transport, and tropical-high latitude subseasonal teleconnections. Impacts of circulation variability and moisture transport on sea ice, ice sheet surface mass balance, snow cover, and other surface cryospheric variables are reviewed and discussed. The future evolution of Arctic amplification is discussed in terms of projected future trends in atmospheric blocking and moisture transport and their coupling with the cryosphere. As high-latitude atmospheric circulation is strongly influenced by lower-latitude processes, the future state of tropical-to-Arctic teleconnections is also considered.
Introduction: Arctic Amplification–Local and Remote Causes
Arctic-amplified near-surface atmospheric warming is a fundamental feature of past climate warming periods, present-day warming trends, and modeled future changes to the Earth’s climate system. However, the causes of this “Arctic amplification” are not fully understood (Serreze and Barry, 2011). Amplified Arctic warming has been most pronounced during autumn and winter, and model simulations project this will remain the case in future warming scenarios (Serreze and Francis, 2006; Lu and Cai, 2009; Serreze et al., 2009; Screen and Simmonds, 2010; Bintanja and van der Linden, 2013; Cohen et al., 2014; Dai et al., 2019). A number of interconnected physical processes, occurring within and external to the Arctic and with distinct seasonal variability, have been identified as contributing to Arctic amplification (Taylor et al., 2013; Yoshimori et al., 2014a; Kim and Kim, 2017; Lang et al., 2017; Goosse et al., 2018; Park et al., 2018; Stuecker et al., 2018; Ding et al., 2019).
The earliest-identified cause of enhanced warming is the ice-albedo (or surface-albedo) feedback, whereby some initial warming reduces polar ice and snow cover and causes a greater fraction of incoming solar radiation to be absorbed, which further accelerates warming and albedo reduction (Budyko, 1969; Cess et al., 1991; Serreze et al., 2009). Other local feedback processes that may contribute to enhanced warming in the absence of extra-Arctic influence include the lapse rate and Planck feedbacks (Manabe and Wetherald, 1975; Crook et al., 2011; Pithan and Mauritsen, 2014; Hahn et al., 2020; Previdi et al., 2020), as well as cloud and water vapor feedbacks (Graversen and Wang, 2009; Cox et al., 2015; Kay et al., 2016; Södergren et al., 2018; Huang et al., 2019; Feldl et al., 2020; Middlemas et al., 2020) in an atmosphere moistened by sea ice decline (Boisvert et al., 2015; Jun et al., 2016; Taylor et al., 2018; Rinke et al., 2019b). Extra-Arctic processes, in particular changes to large-scale atmospheric and oceanic circulation and related changes in poleward atmospheric moist and dry static energy transport (e.g., Yang et al., 2010; Alexeev and Jackson, 2013; Zhang et al., 2013; Graversen and Burtu, 2016; Gong et al., 2017; Yoshimori et al., 2017), also contribute to Arctic amplification and are entangled with intra-Arctic feedbacks in complex ways. For example, cyclones facilitating poleward warm, moist air from lower latitudes may induce an initial retreat of sea ice, which strengthens the local ice-albedo feedback. This in turn amplifies the lapse rate feedback, which can be understood as a manifestation of accentuated lower-tropospheric warming dictated by the surface temperature change (Boeke et al., 2021). Feldl et al. (2020) and Boeke et al. (2021) argue that Arctic amplification should be attributed to the physical processes that establish the nonuniform vertical warming profile producing the lapse rate feedback, rather than the lapse rate feedback per se.
Given this debate, in this review, we focus on several of the key processes important to the ongoing discussion on Arctic amplification, focusing primarily on processes in the troposphere associated with blocking, moisture transport, and subseasonal influences. Our focus in the next sections is as follows: first, we review atmospheric blocking, including its definition, current challenges to identify present and future blocking, its relationship with moisture transport in the Arctic, and the impacts from Arctic blocking and moisture intrusions. We then review tropical-to-high latitude teleconnections that exert critical control on Arctic processes on the subseasonal time scale, both in the atmosphere and cryosphere. We conclude the review with a forward-looking assessment of how these interrelated topics are expected to evolve in future climate.
Arctic Circulation Features: Blocking, Moisture Transport, and Coupling With the Cryosphere
Blocking Definition, Identification, and Northern Hemisphere Climatology
Blocking is broadly defined as a quasi-stationary anomalous anticyclonic circulation pattern which disrupts the eastward propagation of cyclones and other systems (AMS American Meteorological Society, 2020). The formation of a blocking flow pattern results from disruption of the background flow interactions with Rossby wave dynamics (Woollings et al., 2018 and references therein). Mechanisms contributing to the building and maintenance of blocked systems can include moist-thermodynamic processes during cyclogenesis and transient eddies (e.g., Pfahl et al., 2015; Steinfeld and Pfahl, 2019). More recently, blocking has been described by the column budget of Local Wave Activity, using an analogy to traffic congestion (Nakamura and Huang 2018). Direct meteorological impacts of long-lived blocks vary seasonally, primarily resulting in extreme heat and cold airmasses (e.g., Brunner et al., 2018; Schaller et al., 2018), as well as increased advection of heat and moisture along the periphery of the anticyclones (e.g., Barrett et al., 2020; Mattingly et al., 2018) (Figure 1). In the high latitudes, environmental consequences can include significant loss of sea ice and ablation of land ice, as discussed in detail in Tropical-High Latitude Subseasonal Teleconnections (e.g., McLeod and Mote, 2016; Tedesco et al., 2016; Wernli and Papritz, 2018).
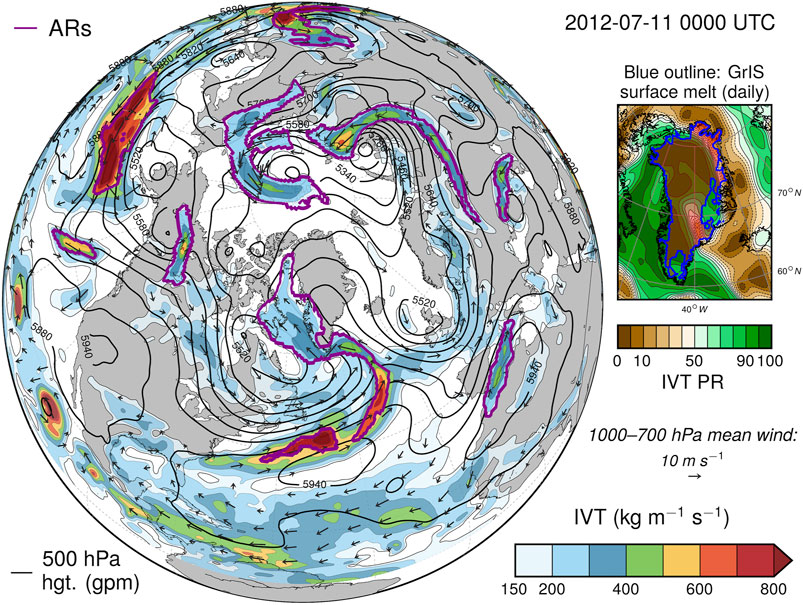
FIGURE 1. Example of atmospheric rivers detected using MERRA-2 data at July 11, 2012 0000 UTC. Purple outlines identify features classified as atmospheric rivers based on the criteria outlined in Mattingly et al. (2018); Table 1.
The broad definition of blocking has led to a variety of different methodological considerations for quantifying blocking and its impacts in the mid- and high-latitudes, particularly regarding spatial and temporal constraints and the dynamic considerations, as explained further in Woollings et al., 2018. The definition of atmospheric blocking has changed over time, resulting in a variety of different blocking identification methods used today. The earliest blocking detection studies, such as Rex (1950), defined blocking as a split jet over a 45° longitudinal span and persisting for 10 or more days. This definition was later revised to include shorter minimum durations, inclusion of latitudinal ranges, geopotential height anomaly thresholds, or a combination of these (e.g., Geb 1966; Charney et al., 1981; Treidl et al., 1981; Lejenas and Øakland, 1983). This has resulted in several papers highlighting advantages and disadvantages of differing methods (Barnes et al., 2012; Woollings et al., 2018; Wachowicz et al., 2021). While we do not intend to detail each separate metric available nor provide an exhaustive list, we briefly discuss some metrics below.
Geopotential Height-Based Indices
Geopotential height-based blocking identification methods are most common, given that geopotential height data is almost universally available in atmospheric reanalysis products and climate model intercomparisons. Consequently, metrics measuring the reversal of the 500-hPa height gradient (e.g., Lejenas and Øakland, 1983; Tibaldi and Molteni, 1990) are most common (Figure 2A). These methods use a constant reference latitude (e.g., 60° N) about which the gradient is computed (Tibaldi and Molteni, 1990), but these approaches may mischaracterize blocking in some regions (Pelly and Hoskins, 2003; Dunn-Sigouin and Son, 2013).
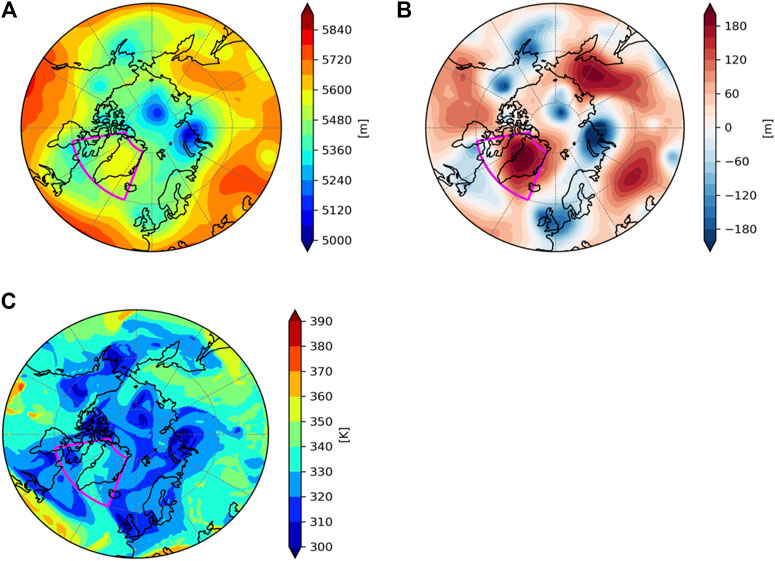
FIGURE 2. A Greenland blocking event on July 11, 2012 seen in (A) 500 hPa height, (B) 500 hPa height anomalies compared with the 1981–2010 JJA climatology, and (C) potential temperature at 2PVU. The box corresponds to the spatial extent of the GBI boundary, from Wachowicz et al. (2021).
Other height-based metrics calculate regional average geopotential height, such as the Greenland Blocking Index (GBI) (Figure 2B) (e.g., Fang 2004; Hanna et al., 2014; Hanna et al., 2016) and Alaska Blocking Index (McLeod et al., 2018), where positive regional anomalies typically indicate blocking conditions. While computationally straightforward, this anomaly type of method may be sensitive to long-term increases in geopotential heights due to climate change (Christidis and Stott, 2015), and may require detrending of the height field prior to calculation (Hanna et al., 2018a; Wachowicz et al., 2021). Similarly, the method described in Dunn-Sigouin and Son, 2013–based on methods from Barriopedro et al. (2010) and Dole and Gordon (1983)–calculates and tracks geopotential height anomalies, which reduces errors in blocking classification that occur when using a constant, reference latitude. Both height reversal and anomaly methods have various spatial, temporal, and amplitude constraints that vary by study, particularly regarding what constitutes instantaneous, regional, and sector blocking events and episodes (e.g., Tibaldi and Molteni, 1990; Dunn-Sigouin and Son, 2013).
PV-Θ -Based Indices
Methods employing a potential vorticity-potential temperature (PV-Θ) approach, which generally follow from Pelly and Hoskins (2003), identify instances of a reversal in the meridional potential temperature (Θ) gradient on a constant PV surface (Figure 2C). An advantage to these methods stems from theory in that Θ is conserved in the absence of diabatic processes (Hoskins et al., 1985; Hoskins 1997). These methods (e.g., Pelly and Hoskins, 2003; Tyrlis and Hoskins, 2008) consider the climatology of annual transient eddy kinetic energy (TEKE) in establishing a reference latitude, which varies with longitude. Moreover, the use of seasonal TEKE may better identify blocking in some regions (Tyrlis and Hoskins, 2008; Masato, et al., 2012; Wachowicz et al., 2021). This method has been adapted to examine Rossby wave breaking characteristics from blocking (e.g., Masato et al., 2012; Masato et al., 2013a; Woollings et al., 2018).
Despite their theoretical advantages, shortcomings of these methodologies include primarily the lack of isentropic variables, primarily in global climate model (GCM) output. As a consequence, others have been able to successfully apply somewhat similar techniques to 500-hPa data to examine blocking in these datasets (e.g., Barnes et al., 2012; Barnes, et al., 2014; Masato et al., 2013b; Simpson et al., 2020). Barnes et al. (2012) report that there is systematically little difference in blocking representation in climate models when comparing the methods, including Tibaldi and Molteni (1990) and Pelly and Hoskins (2003).
While the geographical distribution and frequency of blocking may vary depending on the blocking metric used, some common themes do emerge. Broadly, blocking activity is greatest along the climatological storm tracks—e.g., over the North Atlantic and Pacific Basins or over Asia (Lejenas and Øakland, 1983; Tibaldi and Molteni, 1990; Pelly and Hoskins, 2003). Depending on the method used, blocking occurs over preferred regions including Greenland, Europe, the Central Arctic, Ural-Siberia, and the North Pacific (e.g., Barnes et al., 2014; Horton et al., 2015; Woollings et al., 2018). Furthermore, application of these metrics typically shows blocking to be most common in winter and early spring and least common in summer due to climatological cyclone frequency (Woollings et al., 2018; Lupo 2020).
Stationary and transient eddies within Rossby waves increase poleward moisture and heat transport, and act to enhance upper-level ridges (Franzke et al., 2011; Baggett et al., 2016; Zhang and Wang, 2018; Steinfeld and Pfahl, 2019), leading to blocking conditions over a region (Kim and Kim, 2017; Yang and Magnusdottir, 2017). Rossby wave breaking and diabatic processes, such as latent heat release from moisture transport, can both play an effective role in generating and maintaining blocks (e.g., Masato et al., 2013a; Woollings et al., 2018; Luo et al., 2019; Steinfeld and Pfahl, 2019; Steinfeld et al., 2020). Though the average measured duration of blocking events is dependent on identification methodology, identification efforts indicate that individual blocking events typically last 4 to 9 or more days (Pelly and Hoskins, 2003; Tyrlis and Hoskins, 2008; Lupo et al., 2019). We direct the reader to Lupo (2020) for a more detailed description of specific climatological characteristics of Northern Hemisphere blocks. However, understanding high-latitude moisture transport pathways in more detail is required to fully quantify the impact of blocking within the Arctic climate system and these connections to Arctic amplification more broadly.
Arctic Moisture Transport Pathways and Climatology
The geographical setting of the Northern Hemisphere high latitudes—with oceanic “gateways” in the Atlantic and Pacific sectors opening to the partially confined Arctic Ocean basin, at the downstream terminus of major extratropical storm tracks—facilitates the coupling of Arctic climate with lower-latitude processes. One of the primary ways lower-latitude processes affect the Arctic is through direct poleward heat and moisture fluxes in the atmosphere, which act to partially offset the global radiative imbalance between the tropics and poles (Trenberth and Solomon, 1994; Overland et al., 1996). The major pathways for meridional sensible and latent energy transport into the Arctic are through the North Atlantic (including the Fram Strait and Barents-Kara seas to the east of Greenland and the Labrador Sea/Baffin Bay pathway to the west of Greenland), North Pacific, and Siberian sectors (Sorteberg and Walsh, 2008; Woods et al., 2013; Woods et al., 2017; Dufour et al., 2016; Vázquez et al., 2016; Xu et al., 2020; Mewes and Jacobi, 2019; Mewes and Jacobi, 2020). Most prior studies have focused on meridional moisture rather than sensible heat transport, and have found that moisture transport into the Arctic is greatest during summer and minimized during winter (Dufour et al., 2016). During winter (DJF), poleward moisture transport is mainly confined to the Barents-Kara seas and, to a lesser extent, the Labrador/Baffin Bay and North Pacific transport pathways (Naakka et al., 2019; Nygård et al., 2020). During summer (JJA), mean moisture transport is directed poleward through the North Pacific, Siberian, and Labrador/Baffin Bay pathways, with moisture transport in the North Atlantic sector tending to be diverted zonally over Eurasia and onward into the Siberian pathway (Naakka et al., 2019 - see their Figure 1). Spring (MAM) and autumn (SON) exhibit a mixture of winter and summer characteristics, likely because these averaging periods capture transitions between the maximally frozen and thawed Arctic seasons (Tilinina et al., 2014; Dufour et al., 2016).
Moisture transport into the Arctic does not occur in a temporally uniform manner, but rather is primarily accomplished through intense, short-lived events that are typically linked to cyclones (Sorteberg and Walsh, 2008; Dufour et al., 2016; Rinke et al., 2017; Villamil-Otero et al., 2018; Fearon et al., 2020) and Rossby wave breaking (Liu and Barnes, 2015). These events are often termed “moisture intrusions” (e.g., Doyle et al., 2011; Woods et al., 2013; Woods et al., 2017; Johansson et al., 2017; Yamanouchi, 2019; Ali and Pithan, 2020) and may resemble the narrow “atmospheric rivers” found in the mid-latitudes (Hegyi and Taylor, 2018; Komatsu et al., 2018; Nash et al., 2018; Vázquez et al., 2018).
Greenland Blocking: Impacts on Ice Sheet Surface Mass Balance and Relationships With Moisture Transport
Research efforts during the era of Arctic amplification have placed specific attention on atmospheric blocking and associated moisture transport over Greenland due to its impact on the surface mass balance (SMB) of the Greenland Ice Sheet (GrIS). Greenland blocking events encourage surface melt through a variety of mechanisms that will be discussed below and, as such, are strongly associated with increased GrIS surface melt and runoff over synoptic to decadal timescales (Hanna et al., 2013; Häkkinen et al., 2014; Hermann et al., 2020). This amplified runoff from the ice sheet can impact the climate system in several ways, including through contributions to global sea level rise. GrIS mass loss has contributed approximately 14 mm of sea level rise since the 1970s (Mouginot et al., 2019)–exceeding the upper end of estimates from GCM projections (Slater et al., 2020)–while continued runoff is expected to contribute from 5 to over 30 cm by the end of the century, and complete melt of the ice sheet would raise sea level by over 7 m (Aschwanden et al., 2019).
From a synoptic perspective, Greenland blocking events encourage melt by transporting moist, potentially warm air from unusually low latitudes poleward within the amplified ridge of the blocking anticyclone (Oltmanns et al., 2019; Steinfeld and Pfahl, 2019; Hermann et al., 2020), causing widespread, positive surface temperature anomalies across the ice sheet (Hanna et al., 2014; McLeod and Mote, 2016) (Figure 2). Advection from lower latitudes is greatest along the upstream side of the ridge. A common pattern involves southerly winds tracking the western coast of Greenland and curving anticyclonically to the north of the high-pressure center, transporting warm, moist air poleward through the Labrador Sea/Baffin Bay pathway (Baggett and Lee, 2019; Akers et al., 2020) described in Arctic Moisture Transport Pathways and Climatology (see Figure 1). This results in onshore flow over the ice sheet—a synoptic setting conducive to meltwater production (Mote, 1998b; Fettweis et al., 2011; Fettweis et al., 2013; McLeod and Mote, 2016). This transport of warm, moist air from lower latitudes along with the associated cloud cover anomalies produce widespread surface melt via a spatially varying surface energy balance (SEB) response (Cullather and Nowicki, 2018). However, this exact blocking and moisture transport pattern is not observed during all major GrIS melt events, and the most recent such event in July 2019 involved an unusual blocking pattern transiting westward from Europe to Greenland (Cullather et al., 2020).
The strong advection of heat and moisture during Greenland blocking plays a crucial role in augmenting melt over the steep margins of the ice sheet by mixing warm, moist air to the surface from above the stable boundary layer that typifies the near surface atmosphere, thus enhancing sensible and latent heat flux and increasing the net SEB (Duynkerke and van den Broeke, 1994; van den Broeke and Gallée, 1996; Mattingly et al., 2020). Turbulent heat flux associated with Greenland blocking has been shown to dominate the SEB response during intense melt across much of the ablation zone (Fausto et al., 2016a; Fausto et al., 2016b).
The cloud-radiative effects associated with Greenland blocking are reflective of the interaction between the large-scale synoptic setting and the topography of the ice sheet. As the flow is directed inland to the north of the high-pressure center, anomalously warm and moist air ascends the windward side of the ice sheet, cooling adiabatically (Hahn et al., 2020). This generates an increase in cloud cover and attendant downward longwave radiation while reducing incoming shortwave radiation over northern Greenland and higher elevations of the ice sheet (Hahn et al., 2020; Ward et al., 2020). Conversely, the southern part of the ice sheet is more frequently embedded within the ridge and, consequently, experiences cloud-suppressing subsidence that causes a reduction in downwelling longwave radiation and an increase in incoming shortwave radiation (Hofer et al., 2017; Noël et al., 2019; Hahn et al., 2020; Ward et al., 2020).
The importance of these cloud-radiative effects to the SEB varies geographically as dictated by surface albedo. In locations of high surface albedo, such as northern Greenland and the high-elevation accumulation zone, the SEB is resilient to changes in incoming shortwave radiation and, rather, longwave cloud-radiative effects dominate (Wang B. et al., 2018, Wang et al., 2019; Lenaerts et al., 2019 and references therein). In locations of lower surface albedo, such as southern Greenland and the low-elevation ablation zone, there is a greater sensitivity to changes in incoming shortwave radiation and, consequently, shortwave cloud-radiative effects dominate (Wang W. et al., 2018, Wang et al., 2019; Lenaerts et al., 2019 and references therein; Izeboud et al., 2020). This physical framework explains the seemingly disparate observational results showing that suppressed cloud cover over southern Greenland during frequent blocking events drives GrIS mass loss (Hofer et al., 2017) while also pointing to the critical role of moisture transport and the presence of low-level clouds in generating surface melt at high elevations during extensive melt events (Nghiem et al., 2012; Bennartz et al., 2013; Neff et al., 2014; Van Tricht et al., 2016; Gallagher et al., 2018; Tedesco and Fettweis, 2020).
The impact of each radiative term on the SEB is further complicated by the fact that the surface albedo of the ice sheet is not static with time. In what is referred to as the ice-albedo feedback, surface melt lowers albedo of ice sheet, first by accelerating snow metamorphism, then, in locations of seasonal snowpack, by uncovering lower-albedo glacial ice (Tedesco et al., 2011; Box et al., 2012; Lenaerts et al., 2019 and references therein). Consequently, the influence of incoming shortwave radiation on the SEB increases following Greenland blocking onset (Tedesco et al., 2011; Tedesco et al., 2013; Tedesco et al., 2016) and the efficacy of shortwave cloud radiative effects is maximized at the heart of the melt season (Wang et al., 2019).
A related mechanism whereby Greenland blocking impacts the GrIS is by inhibiting snowfall that would otherwise act to increase both accumulation and surface albedo, thus driving below-normal SMB across portions of the ice sheet that are located within the ridge (Tedesco et al., 2011; Box et al., 2012; Tedesco and Fettweis, 2020). However, the anomalous moisture transport that accompanies Greenland blocking (e.g., Barrett et al., 2020; see also Figure 1) can also cause significant positive SMB anomalies in some situations. Mattingly et al. (2018) show that while strong moisture transport to western Greenland during the melt season is associated with increased melt in the ablation zone, these losses are partially compensated by snowfall in the accumulation zone, while anomalous moisture transport during non-summer months typically produces anomalous snowfall throughout the GrIS, especially in southeast Greenland. This demonstrates that the orientation of blocking anticyclones relative to the topography of the GrIS and their seasonal timing exert critical controls on SMB response.
Arctic Moisture Intrusions: Impacts on Sea Ice and Relationships With Blocking
Numerous studies have found that Arctic moisture intrusions cause sea ice melt or impeded growth. During the cold season, moisture intrusions trigger transitions from the “radiatively clear” to “opaquely cloudy” atmospheric states observed in Arctic field campaigns (Stramler et al., 2011; Woods and Caballero, 2016; Graham et al., 2017b; Cohen et al., 2017; Kayser et al., 2017; Ali and Pithan, 2020). Downwelling longwave radiation is enhanced by anomalous cloud cover and elevated water vapor amounts during subsequent days, which, along with the insulating effect of enhanced snow accumulation during the storms, reduces sea ice growth and may cause the sea ice edge to retreat (Park et al., 2015b; Merkouriadi et al., 2017; Merkouriadi et al., 2020; Persson et al., 2017; Graham et al., 2019). During summer, cyclones are less intense and the warming effect of moisture intrusions may be tempered by their associated negative shortwave cloud radiative effect, but episodes of warm, moist air advection have nevertheless been shown to force sea ice melt (Graversen et al., 2011; Rinke et al., 2019a), with case studies demonstrating the formation of a moist inversion and fog layer over sea ice that enhances downwelling longwave radiation and turbulent heat flux (Tjernström et al., 2015; Tjernström et al., 2019; You et al., 2020). Synoptic storms and moisture intrusions have been found to coincide with the initial melt of sea ice in early summer (Persson, 2012; Else et al., 2014; Hegyi and Deng, 2017) and the period of rapid warming during the Arctic springtime transition (Long and Robinson, 2017). Several studies have found correlations between enhanced winter and spring poleward moisture transport, increased longwave radiation from cloud cover, earlier sea ice melt onset, and decreased sea ice cover during the subsequent September annual minimum (Kapsch et al., 2013; Kapsch et al., 2014; Kapsch et al., 2016; Kapsch et al., 2019; Park et al., 2015a; Cox et al., 2016; Mortin et al., 2016; Cao et al., 2017), although Choi et al. (2014) and Sedlar (2018) find that absorbed shortwave radiation absorption likely plays an important preconditioning role in spring and early summer as well. Winter and spring moisture intrusions are most frequent through the Atlantic sector of the Arctic (Yang and Magnusdottir, 2017; Messori et al., 2018; Hao et al., 2019; Hong et al., 2020; Papritz and Dunn-Sigouin, 2020).
The frequent recent occurrence of atmospheric blocking patterns alongside moist intrusions and warm extremes in the Atlantic and Pacific sectors shows that these circulation features are often closely related, and that blocking also influences sea ice evolution. Anticyclonic flow patterns over the Arctic have been linked to anomalously low summer minimum sea ice extent (Ogi and Wallace, 2012; Bellefamme et al., 2015; Ding et al., 2017; Wernli and Papritz, 2018; Rinke et al., 2019a), to extreme early sea ice melt in the Baffin Bay–Davis Strait–Labrador Sea sector in spring 2013 (Ballinger et al., 2018), and to negative sea ice extent anomalies around Alaska in all seasons (McLeod et al., 2018).
Several recent studies have detailed the complex circulation features and thermodynamic processes that produce episodes of extreme Arctic warmth, including interactions between cyclones, blocking, and moisture transport as well as the coupling between transport from lower latitudes and intra-Arctic thermodynamic processes. Papritz and Dunn-Sigouin (2020) analyzed a climatology of all extreme moisture transport events during winter, finding that planetary-scale anticyclones and synoptic-scale cyclones interact to produce the most extreme events, as blocks deflect the tracks of cyclones poleward and establish a strong poleward transport in the interaction zone between the block and upstream cyclones. During summer, Wernli and Papritz (2018) found that episodic upper-level Arctic anticyclones enhance sea ice reduction, and that extratropical cyclones injecting extratropical air masses with low potential vorticity into the Arctic are responsible for the formation of these anticyclones (in agreement with the extratropical-to-polar transport along moist isentropes described by Laliberté and Kushner (2014)). Binder et al. (2017) examined the dynamic and thermodynamic evolution of a winter 2015–16 extreme Arctic warm event in detail, finding that three air streams with distinct origins and warmed by different processes contributed to the warm episode: warm low-level air of subtropical origin, initially cold low-level air of polar origin heated by surface fluxes, and descending air heated by adiabatic compression. These airstreams were transported poleward by a low-level jet positioned between an anticyclone and a series of cyclones, with further warming by latent heating in a region of continuous warm conveyor belt ascent into the upper part of the anticyclone. Papritz (2020) extended this type of Lagrangian air mass evolution analysis to all Arctic lower-tropospheric warm (and cold) extreme events and found that blocking plays a critical role in generating warm extremes in both summer and winter. Subsidence from the Arctic middle troposphere in blocking anticyclones was found to be the most important warming process, with anomalous blocking in the Barents, Kara, and Laptev Seas favoring warm extremes during winter and blocks located in the high Arctic during summer, when subsidence within blocks is particularly important for warming. Poleward transport of already warm air masses contributes around 20% to warming during both summer and winter, while about 40% of the air masses originate from the Arctic during winter and are heated diabatically by ocean-atmosphere fluxes in marine cold air outbreaks.
These recent studies expand upon and complicate the traditional view that cyclonic moisture intrusions directly transport already warm, moist air from lower latitudes to warm the Arctic and impact sea ice. It instead appears likely that lower latitude atmospheric processes contribute to Arctic warm events through complex planetary- and synoptic-scale dynamics that involve two-way interactions between the Arctic and lower latitudes in response to initial extratropical forcing, with critical contributions to final warming from intra-Arctic coupling between ocean and land surfaces, the atmospheric boundary layer, and the middle and upper troposphere. A further question concerns the vertical distribution of moisture transport in the Arctic atmosphere and its impact on amplification and near-surface warming. Corridors of direct moisture advection from the mid-latitudes to the Arctic tend to ascend from the surface to the middle troposphere along moist isentropes (Laliberté and Kushner, 2014; Wernli and Papritz, 2018; Hao et al., 2021), and Feldl et al. (2020) find that greater warming and moistening of the upper troposphere does not directly contribute to Arctic amplification as it does not affect the near-surface lapse rate feedback. However, transport of moist air masses from the mid-latitude near-surface layer to the Arctic middle and upper troposphere likely still contributes to Arctic warming indirectly, as latent heat release aids the formation of blocking anticyclones (Pfahl et al., 2015; Grams and Archambault, 2016; Zhang and Wang, 2018; Sánchez et al., 2020) that deflect cyclones poleward (Papritz and Dunn-Sigouin, 2020) and warm the lower troposphere through subsidence (Laliberté and Kushner, 2014; Ding et al., 2017; Wernli and Papritz, 2018; Papritz, 2020).
Further research is needed to clarify the complex interrelationships between cyclone-driven moisture intrusions, blocking, and sea ice in a rapidly changing Arctic, along with the contribution of these phenomena to overall Arctic amplification. These investigations should address the seasonality of cyclonic moisture intrusions and blocking and their role in Arctic warming, as Arctic-amplified warming is most pronounced during winter, while the warming contribution of subsidence within anticyclones is maximized during the summer according to Papritz (2020). Arctic blocking is less studied than in the mid-latitudes, and future studies should ensure that any blocking detection algorithms employed in this emerging area of research are suitable to detect Arctic blocks, which are generally weaker than their mid-latitude counterparts and may not be detected by geopotential height-based algorithms (see Blocking Definition, Identification, and Northern Hemisphere Climatology), that require poleward westerlies (Tyrlis et al., 2020). The vertical structure of moisture transport should be carefully examined in relation to blocking and influences on warming, as Arctic moistening in lower levels likely contributes directly to the lapse rate feedback, while middle- and upper-tropospheric moisture transport may contribute to warming through more indirect pathways including latent heat release and blocking development, and/or may act to dampen Arctic amplification due to a negative upper-level lapse rate feedback (Feldl et al., 2020; Hao et al., 2021). The relative importance of and relationships between lower-latitude and within-Arctic processes during Arctic warming events should also be further investigated. Recent studies reviewed here show that a large proportion of air masses originate from within the Arctic during these warm events, but the thermodynamic processes occurring within local and remote air masses to produce warming are linked to extra-Arctic atmospheric circulation features, thus the interactions between variability in the extratropical storm tracks and Arctic circulation extremes are an important avenue for future research. These questions regarding local and remote drivers of episodes of anomalous Arctic warming mirror the broader debate over local and remote influences on Arctic amplification, and future research should assess the overall significance of remotely forced cyclonic moisture intrusions and blocking to Arctic-amplified warming, including their interactions with mechanisms that have traditionally been conceptualized as intra-Arctic processes such as the ice-albedo, lapse rate, and cloud and water vapor feedbacks.
Tropical-High Latitude Subseasonal Teleconnections
Intraseasonal Influences on Extratropical Rossby Waves
In order to understand the potential future evolution of high-latitude circulation and its association with Arctic amplification, it is critical to understand lower-latitude and tropical processes. Tropical upper-tropospheric heating produced by thunderstorms in the convectively active region of the leading mode of atmospheric subseasonal variability, the Madden–Julian Oscillation (MJO; Madden and Julian, 1971; Madden and Julian, 1972; Madden and Julian, 1994), has been found to be an effective source of Rossby wave generation to the extratropics and Arctic (Hoskins and Karoly, 1981; Sardeshmukh and Hoskins, 1988; Bladé and Hartmann, 1995; Jin and Hoskins, 1995; Hendon and Salby, 1996). To generate these Rossby waves, diabatic heating produced by MJO deep convection leads to a divergent component of the upper-tropospheric tropical and subtropical wind field. That divergent wind then advects absolute vorticity toward the poles and leads to the formation of a wave train (Kiladis and Weickmann, 1992; Knutson and Weickmann, 1987; Sardeshmukh and Hoskins, 1988; Hendon and Salby, 1994; Higgins and Mo, 1997; Matthews et al., 2004; L’Heureux and Higgins, 2008; Lin et al., 2009; Seo and Son, 2012; Riddle et al., 2013) that can be traced (Seo and Lee, 2017; Barrett, 2019). The Rossby wave train’s subtropical origins depend on the longitude of the MJO convection, and the origin of the wave train can move east, in tandem with the diabatic heating source (Johnson and Feldstein, 2010; Hamill and Kiladis, 2014; Stan et al., 2017). The Rossby wave source region can extend across nearly the entire tropical Indian Ocean and the Pacific Ocean west of 180° (Lukens et al., 2017), and that allows the MJO to modulate tropical cyclones in the Atlanitc Ocean (Barrett and Leslie, 2009). One proposed physical mechanism for Rossby wave generation is as follows: when the MJO convection is over the Indian Ocean, horizontal convergence in the tropics and the advection of absolute vorticity in the subtropics develops a cyclonic anomaly over southeast Asia. As MJO convection moves eastward, a Rossby wave train departs the subtropics in the exit region of the subtropical Pacific jet (Lukens et al., 2017). Another proposed physical mechanism for Rossby wave generation involves modulation of the local Walker and Hadley cell circulations (Schwendike et al., 2014) over the Pacific. The subtropical jet over the Pacific is enhanced in regions of anomalous upper-tropospheric (anticyclonic; Roundy, 2012) divergence located poleward of MJO convection (Matthews and Kiladis, 1999; Moore et al., 2010). This jet shifts eastward with the MJO propagation, and regions of enhanced convection strengthen the local Hadley circulation, and as upper-tropospheric flow accelerates poleward due to pressure gradient forces, geostrophic adjustments yield troughing downstream of ridging, thereby producing midlatitude Rossby waves that propagate poleward across the Pacific Ocean in both the Northern and Southern Hemispheres (Schwendike et al., 2021).
Because of this important influence, predicting the future state of high-latitude circulation, precipitation, temperature, and cryosphere (snow and ice) depends on the correctly capturing the temporal evolution and geographical distribution of tropical convection of the MJO (Jones et al., 2004; Marshall et al., 2011; Riddle et al., 2013; Rodney et al., 2013; Goss and Feldstein, 2015; Tseng et al., 2018; Lin and Brunet, 2018; Zheng et al., 2019; Zheng and Chang, 2019; Zheng and Chang, 2020; Tseng et al., 2020). Indeed, the broader topic of polar amplification of surface temperature and precipitation has been linked to poleward-propagating Rossby waves excited by MJO-related tropical convection (Figure 3) (Lee et al., 2011). Furthermore, there is evidence that many of the blocked atmospheric circulations in the Arctic that were reviewed in Blocking Definition, Identification, and Northern Hemisphere Climatology are have connections to tropical convection on the subseasonal time scale (Henderson et al., 2016; Henderson and Maloney 2018), and even the moisture pathways and atmospheric rivers reviewed in Blocking Definition, Identification, and Northern Hemisphere Climatology and Arctic Moisture Intrusions: Impacts on Sea Ice and Relationships With Blocking may also have connections to the MJO on the subseasonal time scale (Mundhenk et al., 2016).
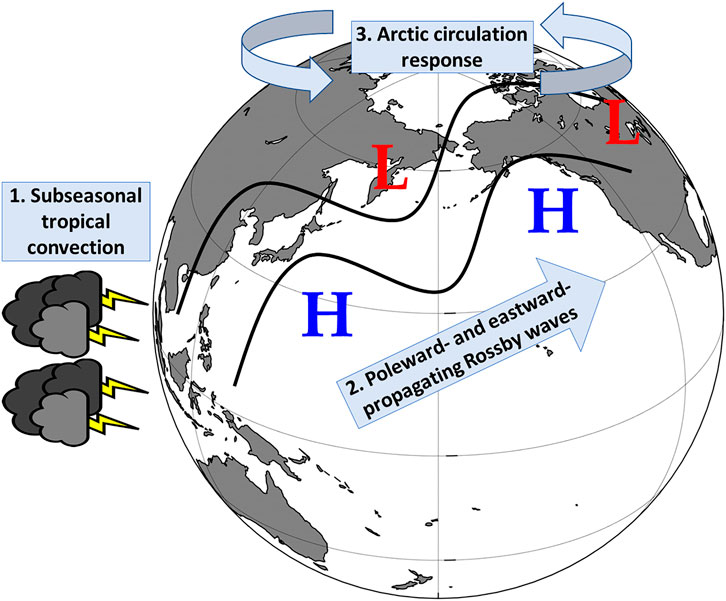
FIGURE 3. Schematic representing the sequence of processes by which subseasonal tropical convection can influence the poleward and eastward propagation of Rossby waves, resulting in an Arctic circulation response.
It remains an open question whether the extratropical Rossby wave train depends more on characteristics of the source region or either the tropical or extratropical flow (Roundy, 2021). Regardless, the Rossby wave response seems to be strongest when the MJO diabatic heating structure resembles a dipole but with asymmetries that are not yet fully understood (Lin and Brunet, 2018). Moreover, stronger MJO events do not necessarily produce stronger extratropical responses or in the same location as weaker MJO events (Lafleur et al., 2015). Another uncertainty comes in the timing of the lagged response, with studies suggesting the lag can be anywhere from 1 to 3 weeks (Higgins et al., 2000; Lin and Brunet, 2009; Riddle et al., 2013; Rodney et al., 2013; Schreck et al., 2013; Matsueda and Takaya, 2015; Seo et al., 2016; Hu et al., 2019).
Approximately 30% of the variability of the subseasonal upper-tropospheric extratropical circulation can be linked with MJO-induced teleconnections (Figure 3) (Matthews et al., 2004; Seo and Son, 2012; Seo et al., 2016). One of the critical consequences of the diabatic heating of the MJO is that it is able to produce long-lasting responses in the high latitudes that last much longer than their tropical forcing (Matthews et al., 2004; Kim et al., 2006; Branstator, 2014; Franzke et al., 2019). This suggests the low-frequency evolution of the MJO in the future will affect many of the processes responsible for Arctic amplification. One other important open question regarding MJO-linked Rossby waves centers on seasonality. There is much research that suggests the MJO is strongest in extended boreal winter (November-May; see reviews by Zhang, 2005 and Zhang et al., 2013). However, in extended boreal summer months (June-September), the tropical intraseasonal oscillation exhibits multiple propagation characteristics: east and north over the Indian monsoon region (Murakami and Nakazawa, 1985; Hartmann and Michelsen, 1989; Gadgil and Srinivasan, 1990) and northwest over the western North Pacific Ocean (Murakami, 1980; Lau and Chan, 1986; Chen and Murakami, 1988). This change in propagation has led to the MJO being referred to as the boreal summer intraseasonal oscillation (BSISO; Krishnamurti and Subrahmanyam, 1982; Yasunari, 1979). The ability of the BSISO to act as a Rossby wave source into the midlatitudes remains significantly understudied when compared to the MJO (Wang et al., 2020a; Wang et al., 2020b). Thus, studies of Arctic amplification in summer months should also consider the teleconnected influence from the BSISO in the tropics, just as studies of Arctic amplification in winter should consider the influence from the tropical MJO.
Despite the remaining questions on the seasonality and strength of the MJO-driven Rossby wave trains, it is very clear that the MJO modulates a wide range of weather phenomena located in the tropics, the mid-latitudes and even in the high latitudes.
Subseasonal Influence on Mid-to-High Latitude Circulation and Surface Variables
In addition to the Rossby wave pathways discussed in Intraseasonal Influences on Extratropical Rossby Waves, another primary way by which the MJO influences surface variables is by strongly modulating the extratropical North Atlantic Oscillation (NAO) and Pacific–North American (PNA) pattern. The PNA and NAO are two of the leading modes of Northern Hemisphere extratropical variability (Blackmon et al., 1984; Hurrell et al., 2001). Over the Pacific, the PNA pattern has been found to be related to the MJO. The MJO’s modulation of the PNA pattern is caused by two Rossby wave sources: a negative source north of MJO-enhanced convection over the Indian Ocean and a positive source north of the MJO-enhanced convection over the western Pacific Ocean (Seo and Lee, 2017). When the MJO convection is in the Pacific Ocean, it tends to excite the positive PNA phase, which translates into above-normal geopotential heights over western North American, below-normal heights over eastern North America (Higgins and Mo, 1997; Mori and Watanabe, 2008; Franzke et al., 2011), and colder-than-normal temperatures over the northeastern United States (Leathers et al., 1991).
Flatau and Kim (2013) noted that the MJO forces the annular modes (the AO and NAO) on intraseasonal time scales. The MJO and NAO have a statistically significant time-lagged relationship. Between 7 and 10 days after MJO convection is over the Indian Ocean (phases 2–3), the probability of a positive NAO is increased significantly from the background climatology. Similarly, between 7 and 10 days after MJO convection is over the western Pacific Ocean (phases 6–7), the probability of a negative NAO is increased significantly (Cassou, 2008; Lin et al., 2009; Yadav and Straus, 2017). This pathway, called by Barnes et al. (2019) the “tropospheric pathway”, is one way that the MJO can influence the NAO. Another mechanism is via the polar stratosphere, and that is not reviewed here, beyond noting that the tropospheric pathway may depend on the prevailing direction of tropical stratospheric winds of the quasi-biennial oscillation (QBO) (when QBO is westerly, the MJO-NAO tropospheric pathway is more robust; Barnes et al., 2019; Feng and Lin, 2019). A positive NAO is associated with anomalous ridging over the eastern United States and North Atlantic Ocean and thus with warmer surface air temperatures and less precipitation (Hurrell et al., 2001; Hurrell et al., 2003). Conversely, a negative NAO is associated with anomalous troughing over the eastern United States and North Atlantic Ocean and thus colder surface air temperatures and more precipitation. Thus, above-average surface temperatures and below-normal precipitation can be expected over the eastern United States after MJO convection is over the Indian Ocean. Similarly, colder temperatures and above-normal precipitation can be expected after MJO convection is over the western Pacific Ocean.
The MJO’s impacts on surface weather can be explained by the extratropical circulation anomalies forced by the tropical MJO convection (Figure 3). Zheng et al. (2018) noted that impacts on extratropical sea-level pressure, and thereby cyclone activity, may be greatest over the eastern North Pacific, the southeastern United States, Canada, and the north-central Atlantic Ocean. Temperature impacts, however, could be more significant over the eastern U.S. (Zheng and Chan, 2019). The anomalies in pressure and temperature have been definitively linked to the Rossby wave trains excited by the MJO, given that the upper-tropospheric circulation anomalies are equivalent barotropic (Zheng and Chan, 2019), thereby allowing any enhancement or suppression of the wind field to cause temperature advection. Zheng and Chan (2019) conclude: “the upper-level Rossby wave train is very important as it connects the MJO and extratropical surface weather anomalies.”
One of the most important ways in which the MJO’s influence extends to the Northern Hemisphere high latitudes is via its influence on surface air temperature (Lin and Brunet 2009; Yao et al., 2011; Yoo et al., 2011; Yoo et al., 2012; Zhou et al., 2012; Rodney et al., 2013; Johnson et al., 2014; Yoo et al., 2014; Lin 2015; Oliver 2015) and precipitation (Bond and Vecchi 2003; Jeong et al., 2008; Lin et al., 2010; Becker et al., 2011; He et al., 2011; Baxter et al., 2014; Jones and Carvalho 2014). Vecchi and Bond (2004) found that geopotential height, specific humidity, and surface air temperature in the Arctic varied by phase of the MJO, while Lin and Brunet (2009) confirmed a similar response of surface air temperature in Canada. Modulation of the Arctic atmosphere specifically, by phase of MJO has also been documented (L’Heureux and Higgins 2008; Yoo et al., 2011), while Yoo et al. (2012) further confirmed the MJO-driven, poleward propagating wave train drove changes in the Arctic overturning circulation, heat flux, and downward infrared radiation. Barrett et al. (2015), Klotzbach et al. (2016), Henderson et al. (2017), and Barrett (2019) noted that the MJO’s influence on circulation and temperature extends to snowfall, and that for the eastern parts of North America, snowfall, snow depth change, and snow water equivalent change all tended to be above normal (or snowier) after MJO convection in phases 8 and 1. This relationship between the MJO and NAO appears to be strongest when the El Niño–Southern Oscillation (ENSO) is in negative (La Niña) phase (Roundy et al., 2010), and as discussed above, when the stratospheric QBO is in its easterly phase (Yoo and Son, 2016).
In addition to documented relationships between MJO and high-latitude temperature and precipitation tendencies via modulation of large-scale circulation, the MJO’s influence has already been found to extend to Arctic sea ice (Henderson et al., 2014), by projecting onto the Arctic atmosphere in both boreal winter and summer seasons. Indeed, sea ice concentration is lower in both the Barents and Kara Seas one to two weeks after convection over the Maritime Continent, as a result of stationary wave interference and subsequent Arctic warming (Goss et al., 2016). Variability in sea ice concentration by phase of MJO was supported by corresponding lower troposphere atmospheric anomalies, with evidence of sea ice modulation occurring regionally. Daily changes in Northern Hemisphere (NH) spring snow depth by phase of MJO was explored by Barrett et al. (2015), with statistically significant depth anomalies found in March, april and May for both North America and Eurasia. In October, correlations between patterns of snow water equivalent (SWE) variability over Eurasia and mid-tropospheric geopotential heights were largest during MJO phases 4–7, indicating that tropical convection anomalies over the Indian Ocean and Maritime continent had the most impact on October circulation and snow variability (Henderson et al., 2017). These studies therefore provide additional evidence for connections between the tropics and the extratropics on subseasonal timescales.
Future Implications for Blocking, Moisture Transport, Subseasonal Variability, and Arctic Amplification
Arctic Amplification & Northern Hemisphere Blocking
There are several working hypotheses for how Arctic Amplification may influence mid-latitude weather extremes, and we refer the reader to existing reviews of the subject for a more thorough examination of the mechanisms behind both winter (Cohen et al., 2014; Cohen et al., 2020) and summer (Coumou et al., 2018) circulation response to Arctic change. Here, we briefly summarize some of these theoretical frameworks as they pertain to blocking. One prominent hypothesis to explain changes in mid-to high-latitude atmospheric circulation follows from the observation that Arctic amplification weakens the near-surface meridional temperature gradient, thus reducing the strength of the jet stream aloft (Francis and Vavrus, 2012; Francis and Vavrus, 2015; Cvijanovic and Caldeira, 2015; Deser et al., 2015; Vavrus et al., 2017). It is proposed that, under a weaker jet, Rossby waves become more amplified, and their westward progression slows, creating conditions that are conducive to blocking (Francis and Vavrus, 2012). It has also been argued that changes in snow cover or sea ice extent can exert an influence on blocking by altering the stationary wave pattern, thus bringing the background state of the atmosphere in certain regions closer to blocked conditions (Matsumura and Yamazaki, 2011; Wu et al., 2013; Cohen et al., 2014; Kim et al., 2014; Nakamura and Huang, 2018). Regarding winter circulation response, research efforts have also placed focus on a stratospheric pathway, where reduced sea ice along the Siberian coast may work in concert with increased snow cover over Eurasia in fall to elicit an increase in the upward propagation of Rossby waves that then disrupts the stratospheric polar vortex and exerts a downward influence on the troposphere, causing a negative AO by late winter (Cohen et al., 2007; Kim et al., 2014; Sun et al., 2015; McKenna et al., 2018; Henderson et al., 2018, Siew et al., 2020). This is relevant to blocking as the negative AO is associated with increased blocking throughout the Northern Hemisphere (Thompson and Wallace, 1998; Hassanzadeh and Kuang, 2015). In summer, it is thought that the enhanced thermal contrast between land and ocean under Arctic amplification increases the formation of wave guides that can encourage wave-amplifying resonance between free and forced Rossby waves, in turn promoting high-amplitude, persistent weather patterns such as those associated with atmospheric blocking (Coumou et al., 2018 and references therein).
Observed and Projected Trends in Northern Hemisphere Blocking, the Greenland Ice Sheet, and Poleward Moisture Transport
Documenting observational support of the theoretical linkages between Arctic amplification and blocking outlined above is an active area of research. While there is no current consensus about observed trends in overall Northern Hemisphere blocking (Woollings et al., 2018), regional trends have been documented despite differences among both blocking metrics and datasets (Barnes et al., 2014).
An analysis of Local Wave Activity has suggested increases in such activity correspond to increases in blocking (Martineau et al., 2017), where cyclonic events are most influential for the development of high-latitude blocks (e.g., Woollings et al., 2018). Martineau et al. (2017) show an increase in local wave amplitudes (i.e., waviness) over East Asia, noting, however, trends in teleconnections such as El Nino/Southern Oscillation (ENSO), the Arctic Oscillation (AO)/North Atlantic Oscillation (NAO), and Pacific-North American teleconnections (PNA) may explain this connection (e.g., Cohen et al., 2012; Kosaka and Xie 2013). In particular, the negative phase of the AO/NAO is associated with both an increased frequency and higher latitude of blocking highs throughout the Northern Hemisphere (Thompson and Wallace, 1998; Hassanzadeh and Kuang, 2015).
Belleflamme et al. (2015) and Ballinger et al. (2014) show that increased anticyclonic activity over the Beaufort Sea and Greenland regions from 1980–2014 may have both contributed to and been the result of Arctic sea ice loss. In particular, the period of 2007–2012 experienced significant anticyclonic circulation anomalies in these regions, which cannot be attributed to either natural climate variability or anthropogenic climate change (Belleflamme et al., 2015; Ballinger et al., 2014; and references therein). In their review paper, Woollings et al. (2018) indicate historical increases in JJA blocking, depending on the metric used, particularly over Greenland and the North Atlantic as well as the Bering Sea during the 1958–2012 period.
GCMs included in the Coupled Model Intercomparison Project phase 3 (CMIP3) and phase 5 (CMIP5) intercomparisons have suggested an overall decrease in blocking frequency in response to climate change in the Northern Hemisphere (e.g., Barnes et al., 2012; Dunn-Sigouin and Son, 2013; Matsueda and Endo, 2017). Most CMIP5 models qualitatively simulate the blocking climatology locations, but underestimate blocking frequency under the RCP8.5 scenario (Dunn-Sigouin and Son, 2013). However, the trends appear to vary both regionally and seasonally. Kennedy et al. (2016) show a decrease in blocking over Eurasia, which is consistent with findings from other studies (e.g., Masato et al., 2013a; Hanna et al., 2018a); Woollings et al., 2018. Under the RCP8.5 scenario, there will likely be a decrease in blocking frequency in both the North Atlantic and North Pacific in fall and winter (Dunn-Sigouin and Son, 2013). Dunn-Sigouin and Son (2013) also show an increase in Ural blocking in future scenarios, although this result is not robust, as described in Woollings et al. (2018). Interestingly, they suggest an increase in European blocking. Additionally, the spatial extent of blocking events is expected to increase, where this increase is greatest for summer blocking (Nabizadeh et al., 2019).
More recently, there has been significant improvement in blocking representation from CMIP5 to CMIP6, though the extent of this improvement still remains dependent on individual models, selected blocking metrics, regions, and seasons (e.g., Davini and D’Andrea, 2020; Schiemann et al., 2020; Simpson et al., 2020). For example, Simpson et al. (2020), Supplementary Information report that overall CMIP6 models appear to better represent the observed spatial climatology compared to CMIP5 although systematic biases in the mean climate state maybe the cause of readily apparent underestimation of wintertime European blocks, and under-(over-)estimation of summer Ural (Eastern Russia) blocking frequency. Similarly, Davini and D’Andrea (2020) confirm the improved overall representation compared to CMIP3. Current CMIP6 projections suggest an overall decrease in blocking more generally in winter and summer, with possible increases in the (summer) Ural and (winter) western North American blocking (Davini and D’Andrea, 2020). Schiemann et al. (2020) report that the regional CMIP6 blocking bias magnitude is sensitive to the blocking metric used.
Given the impact on GrIS SMB, there is a clear motivation to understand long-term variability in Greenland blocking frequency. On an interannual basis, blocking frequency over Greenland has been shown to be strongly related to the North Atlantic Oscillation (NAO). The frequency of blocking highs over Greenland is greater when the NAO is in its negative phase, i.e., when the difference between its two pressure centers, the Azores and Iceland, is minimized (Hanna et al., 2014; Hanna et al., 2015; Hanna et al., 2018a; Hofer et al., 2017). As such, both the NAO and Greenland blocking have been linked to GrIS melt variability, with the latter explaining more of the variance in surface melt (Mote, 1998a; Hanna et al., 2013; McLeod and Mote, 2016).
A growing body of work has highlighted an increase in summer Greenland blocking over the past 2 decades, particularly as measured by the GBI (e.g., Hanna et al., 2016; Hanna et al., 2018a; Hanna et al., 2018b; McLeod and Mote, 2016; Barrett et al., 2020; Wachowicz et al., 2021). Wachowicz et al. (2021) confirmed this summer increase using a 5-years running mean of blocking frequency as measured using both geopotential-height-reversal-based and potential-vorticity-based blocking indices. As one might expect given relationship outlined above, this increase in blocking frequency has coincided with a period of a more negative NAO conditions (Fettweis et al., 2013; van Angelen et al., 2014; Bevis et al., 2019), all of which has occurred against a backdrop of dramatic sea ice and snow cover loss (Derksen and Brown, 2012; Cohen et al., 2014; Pithan and Mauritsen, 2014; Stroeve and Notz, 2018). The coincident nature of these events has spurred interest in the possibility that the change in circulation may be a feature of arctic amplification.
Several studies have invoked either declining sea ice or snow cover as a potential explanation for anomalous anticyclonic conditions over Greenland. A collection of modeling and observational studies has demonstrated anomalous anticyclonic conditions over Greenland in years of low sea ice extent (Screen, 2013; Wu et al., 2013; Petrie et al., 2015; Liu et al., 2016), suggesting the additional heat flux from the ice-free ocean may act to enforce the ridge aloft. Supporting this theory, Wu et al. (2013) tied the summer circulation response to sea ice in the seas west of Greenland by tracking a stationary wave that originates at the surface in spring then persists into summer. Alternatively, Overland et al. (2012) pointed to the observed decline in Northern Hemisphere snow cover extent as a potential explanation for a recent shift in Arctic circulation that features positive pressure anomalies spanning the Arctic coastline of North America, including all of Greenland. Later work demonstrated a possible physical mechanism for this theory where low spring Eurasian snow cover extent increases the poleward propagation of Rossby waves which acts to decelerate the polar jet and encourage a negative AO (Matsumura et al., 2014)—conditions that are associated with increased blocking frequency (Thompson and Wallace, 1998; Hanna et al., 2014; Hanna et al., 2015; Hanna et al., 2018b; Hassanzadeh and Kuang, 2015).
Collectively, GCMs from CMIP3 through CMIP6 have consistently projected a decline in North Atlantic blocking in both winter and summer under future climate scenarios (Davini and D’Andrea, 2020; Masato et al., 2013b; Hanna et al., 2018a). However, GCMs generally fail to adequately represent blocking frequency and duration (Vial and Osborn, 2012; Woollings et al., 2018) and, critically, historical GCM simulations fail to capture the recent positive trend in summertime Greenland blocking, undermining confidence in projections of future blocking in this region (Davini and D’Andrea, 2020; Hanna et al., 2018a) (Figure 4). This underrepresentation carries significant implications, as accurate representation of regional circulation over Greenland is essential for GrIS SMB projections. Indeed, underestimation of GrIS SMB loss is a primary contributor to the negative bias in GCM projections of sea-level rise to date (Slater et al., 2020). Furthermore, a recent idealized modeling analysis found that a continuation of the recent increase in Greenland blocking would cause SMB losses to be more than twice than what is currently estimated from GCM projections (Delhasse et al., 2018).
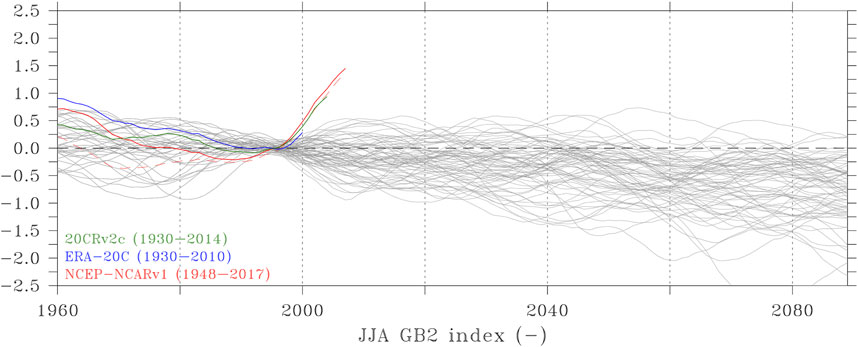
FIGURE 4. Time series of June, July and August Greenland Blocking Index 1 (dashed red line) and Greenland Blocking Index 2 (solid red line) indices over 1950–2,100 as simulated by NCEP/NCAR Reanalysis 1 (red line), by 20CRv2c reanalysis (green line), and by ERA-20C reanalysis in blue as well as by all the CMIP5 models (gray lines) for which both RCP4.5 and RCP8.5 scenarios are available. For the CMIP5-based time series, the historical scenario is used over 1900–2005 and both RCP4.5 and RCP8.5 afterward. A 20-years running mean has been applied to smooth the time series, and values have been normalized (average = 0 and standard deviation = 1) using 1986–2005 as the reference period, from Hanna et al. (2018a).
An increasing trend in episodes of warm and moist air intrusion into the Arctic has been observed. Several episodes of extreme winter warmth have extended latitudinally to the North Pole in recent years (Park D. S. R. et al., 2015; Cullather et al., 2016; Moore, 2016; Lee H. J. et al., 2017; Graham et al., 2017a; Binder et al., 2017; Kim et al., 2017; Kohnemann et al., 2017), and southerly winds contributed to the appearance of anomalous polynya north of Greenland during February–March and August–September 2018 (Moore et al., 2018; Ludwig et al., 2019; Lei et al., 2020). These extreme warm events are often coupled to Ural and Scandinavian blocking patterns (Luo et al., 2017; Rinke et al., 2017; Luo et al., 2019), and their frequent recurrence in recent winters may be linked to the increased incursion of warm, salty Atlantic Water—known as “Atlantification”—in the Atlantic sector of the Arctic Ocean (Alexeev et al., 2017; Polyakov et al., 2017; Polyakov et al., 2020a; Polyakov et al., 2020b; Barton et al., 2018; Tsubouchi et al., 2021). Dramatic sea ice decline and ocean warming have also been observed in the Pacific sector of the Arctic during recent years, alongside persistent atmospheric blocking and southerly moisture intrusions (Lee S. et al., 2017; Overland et al., 2018; Ballinger et al., 2019; Tachibana et al., 2019; Polyakov et al., 2020a; Huntington et al., 2020; Kodaira et al., 2020; Thoman et al., 2020).
Model simulations of future climate states indicate that poleward moisture transport into the Arctic will increase in the future, while dry static energy transport will decrease due to a decreased temperature gradient between the Arctic and lower latitudes (Hwang et al., 2011; Yoshimori et al., 2014b; Yoshimori et al., 2017; Graversen and Burtu, 2016; Feldl et al., 2017; Feldl et al., 2020; Graversen and Langen, 2019). In general, increased poleward water vapor flux can be expected to contribute to amplified Arctic warming through the greenhouse effect of water vapor and clouds as well as latent heat release from condensation. However, recent studies reviewed in Arctic Circulation Features: Blocking, Moisture Transport, and Coupling With the Cryosphere suggest that the warming impact of increasing moisture flux will not be spatially and temporally uniform, and will depend on the vertical structure of water vapor transport as well as its interaction with the atmospheric circulation. Further, changes in water vapor transport from outside the Arctic will likely interact in complex ways with changing within-Arctic hydroclimatological conditions resulting from sea ice loss and ocean warming. The influence of declining sea ice on cyclones, moisture transport, and blocking, and in turn the feedbacks of these atmospheric processes into sea ice and oceanic conditions, should be studied further. For example, Kim and Kim (2017) hypothesized that the increasing open water fraction from sea ice decline will reduce the ocean-atmosphere temperature gradient and suppress turbulent heat flux into the atmosphere, which will serve to slow Arctic warming and enhance the role of atmospheric heat and moisture transport from lower latitudes in Arctic climate.
Future Trends in Tropical-To-Arctic Teleconnections
High-latitude atmospheric circulation is strongly influenced by lower-latitude processes, including those from the tropical and subtropical Pacific, through the poleward propagation of Rossby wave trains (e.g., Ding et al., 2014; Feng et al., 2017; Jiménez-Esteve and Domeisen, 2018). In order to understand the potential future evolution of the high-latitude circulation and its association with Arctic amplification, it is necessary to understand the future evolution of those lower-latitude processes. Some studies suggest that recent Arctic amplification could be at least partially attributed to enhanced warm-air advection and moisture transport by Rossby waves associated with an increase in the frequency of MJO activity over the western Pacific and Maritime Continent (Lee et al., 2011; Yoo et al., 2011; Yoo et al., 2012; Seo et al., 2016; Jiang et al., 2020). However, there are more studies that examine changes in the MJO (both historical and future) than changes in MJO-driven teleconnections to the high latitudes. Here, we review aspects of both changes to the MJO and its teleconnections.
The MJO is strongly influenced by the atmospheric mean state in the tropics (Maloney and Hartmann, 2001; Inness and Slingo, 2003; Zhang and Dong, 2004; Maloney and Xie, 2013). Thus, changes in tropical sea-surface temperatures, wind shear, moisture availability, and stability driven by anthropogenic global warming all have the potential to influence the MJO, and by association, Arctic amplification. Some studies suggest that the amplitude of the MJO may intensify and its variance increase with increasing warming (Takahashi et al., 2011; Liu et al., 2013; Schubert et al., 2013; Subramanian et al., 2014; Arnold et al., 2015; Wolding et al., 2017; Haertel, 2018; Rushley et al., 2019). Others suggest the MJO may become spatially more expansive in the zonal direction, with greater surface convergence, enhanced deep convection, faster eastward propagation, and more heating (Caballero and Huber, 2010; Arnold et al., 2013; Liu, 2013; Liu et al., 2013; Chang et al., 2015; Song and Seo, 2016; Adames et al., 2017; Cui and Li 2019). While most studies suggest a more intense MJO over the 21st century, some suggest that an increase in tropical static stability may weaken the MJO’s generation of Rossby waves (Bui and Maloney, 2018; Bui and Maloney, 2019a) by weakening the wind response. This weakening would have important implications for Arctic amplification, since the divergent flow anomalies produced by the MJO’s anomalous heating are the source for Rossby wave generation into the extratropics (Sardeshmukh and Hoskins, 1988; Wolding et al., 2017; Maloney et al., 2019). Some modeling results suggest that teleconnected impacts in the Arctic may be weaker per unit of MJO precipitation anomaly over the next several decades (Jiang et al., 2020). This is further complicated because the MJO-Arctic link depends strongly on complex, nonlinear changes in extratropical static stability, storm track, North Pacific jet stream, and other aspects of the general circulation that all impact the receivership state of the Arctic to MJO teleconnections (Kang and Tziperman, 2018; Bui and Maloney, 2019b; Jiang et al., 2020).
Given all this, many questions remain unanswered regarding the role of tropical teleconnections and their impact on Arctic amplification. The extent to which MJO can influence atmospheric blocking is relatively understudied; however, preliminary work suggests that not only does MJO affect blocking frequency in particular at higher latitudes (Henderson et al., 2016), the relationship is also influenced by ENSO phase (Henderson et al., 2018). Future implications of this tropical-to-Arctic Amplification linkage are further complicated as studies disagree on projections of increased MJO precipitation under warmer climates (e.g., Schubert et al., 2013; Rushley et al., 2019). Moreover, few studies have examined either MJO teleconnections, in general, or MJO-Arctic linkages in warmer climates (e.g., Maloney et al., 2019; Jiang et al., 2020). More research is needed to fully understand the complex, multi-layered and non-linear processes that connect tropical and extratropical atmospheric circulation, its interaction with atmospheric blocking and moisture transport, and subsequent impacts on and amplification of changing Arctic atmospheric and surface variables.
Summary and Discussion
Arctic amplification is a fundamental feature of past, present, and modeled future climate. However, the causes of this amplification within Earth’s climate system are not fully understood. To date, warming in the Arctic has been most pronounced in autumn and winter seasons, with this trend predicted to continue based on model projections of future climate. The intra-Arctic feedbacks by which this is taking place are numerous and interconnected, while extra-Arctic processes, in particular changes to large-scale atmospheric circulation and moisture transport, are entangled with these internal feedbacks in complex ways.
A question we pose is whether external forcing from the tropics is appropriately considered in a discussion of Arctic amplification. If one defines Arctic amplification in a manner in which the Arctic specifies both the region in which the enhanced warming is observed and also all feedbacks involving amplification occur, then consideration of tropical forcing would fall outside the discussion. However, if we broaden the discussion to include mechanisms that import sensible and latent heat into the Arctic and thereby enhance local longwave forcing, in addition to mechanisms that modulate Arctic atmospheric circulation, triggering within-Arctic feedbacks (e.g., ice-albedo, lapse rate), then inclusion of forcing from lower latitudes becomes critical. We therefore have reviewed forcings acting within and external to the Arctic that lead to enhanced warming and cryospheric impacts in this region.
We have anchored our discussion of Arctic atmospheric circulation features on atmospheric blocking and moisture transport, as these phenomena have been widely studied and are interrelated mechanisms by which lower-latitude processes can couple with high-latitude Arctic climate. Blocking can broadly be defined as a quasi-stationary anomalous anticyclonic circulation pattern which disrupts the eastward propagation of cyclones and other systems, analogous to traffic congestion within the atmosphere (Nakamura and Huang, 2018). Poleward moisture transport into the Arctic is primarily accomplished by short-lived intrusion events associated with cyclones, which preferentially occur in certain regions and seasons as detailed in Arctic Moisture Transport Pathways and Climatology. Blocking and moisture transport are closely related, as poleward moisture transport is favored along the upstream flank of a blocking anticyclone, and latent heat release within the moisture plume often helps maintain or strengthen the block. These phenomena have important regional and global climate implications due to their influence on Greenland Ice Sheet surface mass balance and Arctic sea ice variability (Greenland Blocking: Impacts on Ice Sheet Surface Mass Balance and Relationships With Moisture Transport and Arctic Moisture Intrusions: Impacts on Sea Ice and Relationships With Blocking). Greenland blocking and episodes of extreme moisture transport into the Atlantic and Pacific sectors of the Arctic have shown increasing trends in recent years, highlighting the importance of further research to clarify the complex interrelationship between cyclone-driven moisture intrusions, blocking, and sea and land ice in a rapidly changing Arctic, along with the contribution of these phenomena to overall Arctic amplification.
With the nature of these Arctic moisture intrusions being associated with short-lived, intense events linked to cyclones (Sorteberg and Walsh, 2008; Dufour et al., 2016; Rinke et al., 2017; Villamil-Otero et al., 2018; Fearon et al., 2020) and Rossby wave breaking (Liu and Barnes, 2015), we have also reviewed the role of tropical subseasonal atmospheric variability in forcing extratropical and polar atmospheric circulation (Tropical-High Latitude Subseasonal Teleconnections). The MJO has been found to be an effective source of Rossby wave generation to the extratropics (Hoskins and Karoly, 1981; Sardeshmukh and Hoskins, 1988; Bladé and Hartmann 1995; Jin and Hoskins, 1995; Hendon and Salby, 1996), with poleward-propagating Rossby waves excited by MJO-related tropical convection being linked to polar amplification of surface air temperature (Lin and Brunet, 2009; Lee et al., 2011; Yao et al., 2011; Yoo et al., 2011; Yoo et al., 2012; Zhou et al., 2012; Rodney et al., 2013; Johnson et al., 2014; Yoo et al., 2014; Lin, 2015; Oliver, 2015), precipitation (Bond and Vecchi, 2003; Jeong et al., 2008; Lin et al., 2010; Becker et al., 2011; He et al., 2011; Baxter et al., 2014; Jones and Carvalho 2014), sea-level pressure, snow depth (Barrett et al., 2015; Henderson et al., 2017), and sea ice (Henderson et al., 2014).
Although our focus in this review is on subseasonal extra-Arctic forcing originating in the tropical Pacific and Indian Oceans, we also note that a number of studies have shown that Atlantic Ocean variability can influence Northern Hemisphere high latitude climate on interannual to multidecadal time scales (e.g., Kwon and Joyce, 2013; Hahn et al., 2018; Kwon et al., 2018; Kwon et al., 2020; Joyce et al., 2019; Athanasiadis et al., 2020). Future studies should examine the relative importance and characteristic time scales of these Indo-Pacific and Atlantic influences on Arctic climate in a coherent framework.
When considering future Arctic climate, a great deal of uncertainty exists due to rapid, and in some cases unprecedented, changes that have already occurred in recent decades. Here, we have focused on within- and extra-Arctic process drivers, specifically high-latitude atmospheric blocking, poleward moisture transport, and tropical-high latitude subseasonal teleconnections, and the role they have on the Arctic atmosphere, surface variables and amplification of changes in this region. Remaining questions arise from 1) the theoretical linkages between Arctic amplification and blocking, 2) the future evolution of poleward moist and dry static energy transport, and 3) future change in tropical subseasonal variability. Observed blocking trends are dependent on the metrics used to quantify such events and there are inconsistencies in current climate models’ abilities to fully capture blocking. Poleward moisture transport is projected to increase in a warming climate, but the vertical structure of moisture transport will likely determine the magnitude of its contribution to Arctic surface warming (Arctic Moisture Intrusions: Impacts on Sea Ice and Relationships With Blocking), and decreasing dry static energy transport due to sea ice loss may limit increases in total atmospheric heat transport to the Arctic (Audette et al., 2021). Similarly, divergent consensus on future trends of tropical subseasonal variability under a warmer climate makes projections of MJO-Arctic linkages under such climates challenging, and warrants more research to understand this complex and highly non-linear connection between the tropics and Arctic amplification.
Author Contributions
GH outlined the study scope, which was then further developed by all authors. Additionally, she co-led Intraseasonal Influences on Extratropical Rossby Waves, Subseasonal Influence on Mid-to-High Latitude Circulation and Surface Variables, and Future Trends in Tropical-To-Arctic Teleconnections with BB, and led Summary and Discussion. Additionally, Barrett produced the Figure 3 schematic. KM outlined Arctic Circulation Features: Blocking, Moisture Transport, and Coupling With the Cryosphere with LW and JP, and led the writing of Arctic Moisture Transport Pathways and Climatology, Arctic Moisture Intrusions: Impacts on Sea Ice and Relationships With Blocking and Observed and Projected Trends in Northern Hemisphere Blocking, the Greenland Ice Sheet, and Poleward Moisture Transport. LW and JP led the writing on Blocking Definition, Identification, and Northern Hemisphere Climatology, Greenland Blocking: Impacts on Ice Sheet Surface Mass Balance and Relationships With Moisture Transport and Arctic Amplification & Northern Hemisphere Blocking. TM helped frame the paper, and contributed to Summary and Discussion.
Funding
Funding for this research was provided by the Strategic Environmental Research and Development Program (SERDP), grant award number RC18-1658, and partially by the National Science Foundation Office of Polar Programs (OPP), award number 1821915.
Conflict of Interest
The authors declare that the research was conducted in the absence of any commercial or financial relationships that could be construed as a potential conflict of interest.
References
Adames, Á. F., Kim, D., Sobel, A. H., Del Genio, A., and Wu, J. (2017). Changes in the Structure and Propagation of the M JO with Increasing C O 2. J. Adv. Model. Earth Syst. 9 (2), 1251–1268. doi:10.1002/2017ms000913
Akers, P. D., Kopec, B. G., Mattingly, K. S., Klein, E. S., Causey, D., and Welker, J. M. (2020). Baffin Bay Sea Ice Extent and Synoptic Moisture Transport Drive Water Vapor Isotope (δ18O, δD, D-Excess) Variability in Coastal Northwest Greenland. Atmos. Chem. Phys. 20 (22), 13929–13955. doi:10.5194/acp-2020-340
Alexeev, V. A., and Jackson, C. H. (2013). Polar Amplification: Is Atmospheric Heat Transport Important?. Clim. Dyn. 41, 533–547. doi:10.1007/s00382-012-1601-z
Alexeev, V. A., Walsh, J. E., Ivanov, V. V., Semenov, V. A., and Smirnov, A. V. (2017). Warming in the Nordic Seas, North Atlantic Storms and Thinning Arctic Sea Ice. Environ. Res. Lett. 12, 084011. doi:10.1088/1748-9326/aa7a1d
Ali, S. M., and Pithan, F. (2020). Following Moist Intrusions into the Arctic Using SHEBA Observations in a Lagrangian Perspective. Q. J. R. Meteorol. Soc. 146, 3522–3533. doi:10.1002/qj.3859
American Meteorological Society (2020). Blocking. Glossary of Meteorology. US: Weatherwise. Available at: http://glossary.ametsoc.org/wiki/blocking.
Arnold, N. P., Branson, M., Kuang, Z., Randall, D. A., and Tziperman, E. (2015). MJO Intensification with Warming in the Superparameterized CESM. J. Clim. 28, 2706–2724. doi:10.1175/JCLI-D-14-00494.1
Arnold, N. P., Kuang, Z., and Tziperman, E. (2013). Enhanced MJO-like Variability at High SST. J. Clim. 26, 988–1001. doi:10.1175/jcli-d-12-00272.1
Aschwanden, A., Fahnestock, M. A., Truffer, M., Brinkerhoff, D. J., Hock, R., Khroulev, C., et al. (2019). Contribution of the Greenland Ice Sheet to Sea Level over the Next Millennium. Sci. Adv. 5, eaav9396. doi:10.1126/sciadv.aav9396
Athanasiadis, P. J., Yeager, S., Kwon, Y.-O., Bellucci, A., Smith, D. W., and Tibaldi, S. (2020). Decadal Predictability of North Atlantic Blocking and the NAO. Npj Clim. Atmos. Sci. 3, 20. doi:10.1038/s41612-020-0120-6
Audette, A., Fajber, R. A., Kushner, P. J., Wu, Y., Peings, Y., Magnusdottir, G., et al. (2021). Opposite Responses of the Dry and Moist Eddy Heat Transport into the Arctic in the PAMIP Experiments. Geophys. Res. Lett. 48 (9), e2020GL089990. doi:10.1029/2020GL089990
Baggett, C., Lee, S., and Feldstein, S. (2016). An Investigation of the Presence of Atmospheric Rivers over the North Pacific during Planetary-Scale Wave Life Cycles and Their Role in Arctic Warming. J. Atmos. Sci. 73, 4329–4347. doi:10.1175/JAS-D-16-0033.1
Baggett, C., and Lee, S. (2019). Summertime Midlatitude Weather and Climate Extremes Induced by Moisture Intrusions to the West of Greenland. Q. J. R. Meteorol. Soc. 145, 3148–3160. doi:10.1002/qj.3610
Ballinger, T. J., Hanna, E., Hall, R. J., Cropper, T. E., Miller, J., Ribergaard, M. H., et al. (2018). Anomalous Blocking over Greenland Preceded the 2013 Extreme Early Melt of Local Sea Ice. Ann. Glaciol. 59, 181–190. doi:10.1017/aog.2017.30
Ballinger, T. J., Lee, C. C., Sheridan, S. C., Crawford, A. D., Overland, J. E., and Wang, M. (2019). Subseasonal Atmospheric Regimes and Ocean Background Forcing of Pacific Arctic Sea Ice Melt Onset. Clim. Dyn. 52, 5657–5672. doi:10.1007/s00382-018-4467-x
Ballinger, T. J., Sheridan, S. C., and Hanna, E. (2014). Resolving the Beaufort Sea High Using Synoptic Climatological Methods. Int. J. Climatol. 34, 3312–3319. doi:10.1002/joc.3907
Barnes, E. A., Dunn-Sigouin, E., Masato, G., and Woollings, T. (2014). Exploring Recent Trends in Northern Hemisphere Blocking. Geophys. Res. Lett. 41, 638–644. doi:10.1002/2013GL058745
Barnes, E. A., Samarasinghe, S. M., Ebert‐Uphoff, I., and Furtado, J. C. (2019). Tropospheric and Stratospheric Causal Pathways between the MJO and NAO. J. Geophys. Res. Atmos. 124, 9356–9371. doi:10.1029/2019JD031024
Barnes, E. A., Slingo, J., and Woollings, T. (2012). A Methodology for the Comparison of Blocking Climatologies across Indices, Models and Climate Scenarios. Clim. Dyn. 38, 2467–2481. doi:10.1007/s00382-011-1243-6
Barrett, B. S. (2019). Connections between the Madden-Julian Oscillation and Surface Temperatures in winter 2018 over Eastern North America. Atmos. Sci. Lett. 20, e869. doi:10.1002/asl.869
Barrett, B. S., Henderson, G. R., McDonnell, E., Henry, M., and Mote, T. (2020). Extreme Greenland Blocking and High‐latitude Moisture Transport. Atmos. Sci. Lett. 21, e1002. doi:10.1002/asl.1002
Barrett, B. S., Henderson, G. R., and Werling, J. S. (2015). The Influence of the MJO on the Intraseasonal Variability of Northern Hemisphere Spring Snow Depth. J. Clim. 28, 7250–7262. doi:10.1175/JCLI-D-15-0092.1
Barrett, B. S., and Leslie, L. M. (2009). Links between Tropical Cyclone Activity and Madden-Julian Oscillation Phase in the North Atlantic and Northeast Pacific Basins. Monthly Weather Rev. 137, 727–744. doi:10.1175/2008MWR2602.1
Barriopedro, D., García-Herrera, R., and Trigo, R. M. (2010). Application of Blocking Diagnosis Methods to General Circulation Models. Part I: A Novel Detection Scheme. Clim. Dyn. 35, 1373–1391. doi:10.1007/s00382-010-0767-5
Barton, B. I., Lenn, Y.-D., and Lique, C. (2018). Observed Atlantification of the Barents Sea Causes the Polar Front to Limit the Expansion of Winter Sea Ice. J. Phys. Oceanogr. 48, 1849–1866. doi:10.1175/JPO-D-18-0003.1
Baxter, S., Weaver, S., Gottschalck, J., and Xue, Y. (2014). Pentad Evolution of Wintertime Impacts of the Madden-Julian Oscillation over the Contiguous United States. J. Clim. 27, 7356–7367. doi:10.1175/JCLI-D-14-00105.1
Becker, E. J., Berbery, E. H., and Higgins, R. W. (2011). Modulation of Cold-Season U.S. Daily Precipitation by the Madden-Julian Oscillation. J. Clim. 24, 5157–5166. doi:10.1175/2011JCLI4018.1
Belleflamme, A., Fettweis, X., and Erpicum, M. (2015). Recent Summer Arctic Atmospheric Circulation Anomalies in a Historical Perspective. The Cryosphere 9, 53–64. doi:10.5194/tc-9-53-2015
Bennartz, R., Shupe, M. D., Turner, D. D., Walden, V. P., Steffen, K., Cox, C. J., et al. (2013). July 2012 Greenland Melt Extent Enhanced by Low-Level Liquid Clouds. Nature 496, 83–86. doi:10.1038/nature12002
Bevis, M., Harig, C., Khan, S. A., Brown, A., Simons, F. J., Willis, M., et al. (2019). Accelerating Changes in Ice Mass within Greenland, and the Ice Sheet's Sensitivity to Atmospheric Forcing. Proc. Natl. Acad. Sci. USA 116, 1934–1939. doi:10.1073/pnas.1806562116
Binder, H., Boettcher, M., Grams, C. M., Joos, H., Pfahl, S., and Wernli, H. (2017). Exceptional Air Mass Transport and Dynamical Drivers of an Extreme Wintertime Arctic Warm Event. Geophys. Res. Lett. 44 (23), 12028–12036. doi:10.1002/2017GL075841
Bintanja, R., and van der Linden, E. C. (2013). The Changing Seasonal Climate in the Arctic. Sci. Rep. 3, 1556. doi:10.1038/srep01556
Blackmon, M. L., Lee, Y.-H., Wallace, J. M., and Hsu, H. H. (1984). Time Variation of 500 Mb Height Fluctuations with Long, Intermediate and Short Time Scales as Deduced from Lag-Correlation Statistics. J. Atmos. Sci. 41, 981–991. doi:10.1175/1520-0469(1984)041<0981:tvomhf>2.0.co;2
Bladé, I., and Hartmann, D. L. (1995). The Linear and Nonlinear Extratropical Response of the Atmosphere to Tropical Intraseasonal Heating. J. Atmos. Sci. 52, 4448–4471. doi:10.1175/1520-0469(1995)052<4448:tlaner>2.0.co;2
Boeke, R. C., Taylor, P. C., and Sejas, S. A. (2021). On the Nature of the Arctic's Positive Lapse‐Rate Feedback. Geophys. Res. Lett. 48, e2020GL091109. doi:10.1029/2020GL091109
Boisvert, L. N., Wu, D. L., and Shie, C.-L. (2015). Increasing Evaporation Amounts Seen in the Arctic between 2003 and 2013 from AIRS Data. J. Geophys. Res. Atmos. 120, 6865–6881. doi:10.1002/2015JD023258
Bond, N. A., and Vecchi, G. A. (2003). The Influence of the Madden-Julian Oscillation on Precipitation in Oregon and Washington*. Wea. Forecast. 18, 600–613. doi:10.1175/1520-0434(2003)018<0600:tiotmo>2.0.co;2
Box, J. E., Fettweis, X., Stroeve, J. C., Tedesco, M., Hall, D. K., and Steffen, K. (2012). Greenland Ice Sheet Albedo Feedback: Thermodynamics and Atmospheric Drivers. The Cryosphere 6, 821–839. doi:10.5194/tc-6-821-2012
Branstator, G. (2014). Long-Lived Response of the Midlatitude Circulation and Storm Tracks to Pulses of Tropical Heating. J. Clim. 27, 8809–8826. doi:10.1175/jcli-d-14-00312.1
Brunner, L., Schaller, N., Anstey, J., Sillmann, J., and Steiner, A. K. (2018). Dependence of Present and Future European Temperature Extremes on the Location of Atmospheric Blocking. Geophys. Res. Lett. 45, 6311–6320. doi:10.1029/2018GL077837
Budyko, M. I. (1969). The Effect of Solar Radiation Variations on the Climate of the Earth. The effect solar Radiat. variations Clim. Earth 21, 611–619. doi:10.1111/j.2153-3490.1969.tb00466.x
Bui, H. X., and Maloney, E. D. (2018). Changes in Madden‐Julian Oscillation Precipitation and Wind Variance under Global Warming. Geophys. Res. Lett. 45 (14), 7148–7155. doi:10.1029/2018gl078504
Bui, H. X., and Maloney, E. D. (2019b). Mechanisms for Global Warming Impacts on Madden-Julian Oscillation Precipitation Amplitude. J. Clim. 32 (20), 6961–6975. doi:10.1175/jcli-d-19-0051.1
Bui, H. X., and Maloney, E. D. (2019a). Transient Response of MJO Precipitation and Circulation to Greenhouse Gas Forcing. Geophys. Res. Lett. 46 (22), 13546–13555. doi:10.1029/2019gl085328
Caballero, R., and Huber, M. (2010). Spontaneous Transition to Superrotation in Warm Climates Simulated by CAM3. Geophys. Res. Lett. 37, L11701. doi:10.1029/2010GL043468
Cao, Y., Liang, S., Chen, X., He, T., Wang, D., and Cheng, X. (2017). Enhanced Wintertime Greenhouse Effect Reinforcing Arctic Amplification and Initial Sea-Ice Melting. Sci. Rep. 7, 8462. doi:10.1038/s41598-017-08545-2
Cassou, C. (2008). Intraseasonal Interaction between the Madden-Julian Oscillation and the North Atlantic Oscillation. Nature 455, 523–527. doi:10.1038/nature07286
Cess, R. D., Potter, G. L., Zhang, M. H., Blanchet, J. P., Chalita, S., Colman, R., et al. (1991). Interpretation of Snow-Climate Feedback as Produced by 17 General Circulation Models. Science 253, 888–892. doi:10.1126/science.253.5022.888
Chang, C. W. J., Tseng, W. L., Hsu, H. H., Keenlyside, N., and Tsuang, B. J. (2015). The Madden-Julian Oscillation in a Warmer World. Geophys. Res. Lett. 42 (14), 6034–6042. doi:10.1002/2015gl065095
Charney, J. G., Shukla, J., and Mo, K. C. (1981). Comparison of a Barotropic Blocking Theory with Observation. J. Atmos. Sci. 38, 762–779. doi:10.1175/1520-0469(1981)038<0762:coabbt>2.0.co;2
Chen, T. C., and Murakami, M. (1988). The 30-50 Day Variation of Convective Activity over the Western Pacific Ocean with Emphasis on the Northwestern Region. Mon. Wea. Rev. 116 (4), 892–906. doi:10.1175/1520-0493(1988)116<0892:tdvoca>2.0.co;2
Choi, Y. S., Kim, B. M., Hur, S. K., Kim, S. J., Kim, J. H., and Ho, C. H. (2014). Connecting Early Summer Cloud-Controlled Sunlight and Late Summer Sea Ice in the Arctic. J. Geophys. Res. Atmos. 119 (19), 11087–11099. doi:10.1002/2014JD022013
Christidis, N., and Stott, P. A. (2015). Changes in the Geopotential Height at 500 hPa under the Influence of External Climatic Forcings. Geophys. Res. Lett. 42, 798–810. doi:10.1002/2015GL066669
Cohen, J. L., Furtado, J. C., Barlow, M. A., Alexeev, V. A., and Cherry, J. E. (2012). Arctic Warming, Increasing Snow Cover and Widespread Boreal winter Cooling. Environ. Res. Lett. 7 (1), 014007. doi:10.1088/1748-9326/7/1/014007
Cohen, J., Screen, J. A., Furtado, J. C., Barlow, M., Whittleston, D., Coumou, D., et al. (2014). Recent Arctic Amplification and Extreme Mid-latitude Weather. Nat. Geosci 7, 627–637. doi:10.1038/ngeo2234
Cohen, J., Zhang, X., Francis, J., Jung, T., Kwok, R., Overland, J., et al. (2020). Divergent Consensuses on Arctic Amplification Influence on Midlatitude Severe winter Weather. Nat. Clim. Chang. 10, 20–29. doi:10.1038/s41558-019-0662-y
Cohen, L., Hudson, S. R., Walden, V. P., Graham, R. M., and Granskog, M. A. (2017). Meteorological Conditions in a Thinner Arctic Sea Ice Regime from winter to Summer during the Norwegian Young Sea Ice Expedition (N‐ICE2015). J. Geophys. Res. Atmos. 122, 7235–7259. doi:10.1002/2016JD026034
Cohen, J., Barlow, M., Kushner, P. J., and Saito, K. (2007). Stratosphere–Troposphere Coupling and Links with Eurasian Land Surface Variability. J. Climate 20, 5335–5343. doi:10.1175/2007JCLI1725.1
Coumou, D., Di Capua, G., Vavrus, S., Wang, L., and Wang, S. (2018). The Influence of Arctic Amplification on Mid-latitude Summer Circulation. Nat. Commun. 9, 2959. doi:10.1038/s41467-018-05256-8
Cox, C. J., Uttal, T., Long, C. N., Shupe, M. D., Stone, R. S., and Starkweather, S. (2016). The Role of Springtime Arctic Clouds in Determining Autumn Sea Ice Extent. J. Clim. 29, 6581–6596. doi:10.1175/JCLI-D-16-0136.1
Cox, C. J., Walden, V. P., Rowe, P. M., and Shupe, M. D. (2015). Humidity Trends Imply Increased Sensitivity to Clouds in a Warming Arctic. Nat. Commun. 6, 10117. doi:10.1038/ncomms10117
Crook, J. A., Forster, P. M., and Stuber, N. (2011). Spatial Patterns of Modeled Climate Feedback and Contributions to Temperature Response and Polar Amplification. Amplification 24, 3575–3592. doi:10.1175/2011JCLI3863.1
Cui, J., and Li, T. (2019). Changes of MJO Propagation Characteristics under Global Warming. Clim. Dyn. 53 (9), 5311–5327. doi:10.1007/s00382-019-04864-4
Cullather, R. I., Andrews, L. C., Croteau, M. J., Digirolamo, N. E., Hall, D. K., Lim, Y. K., et al. (2020). Anomalous Circulation in July 2019 Resulting in Mass Loss on the Greenland Ice Sheet. Geophys. Res. Lett. 47, e2020GL087263. doi:10.1029/2020GL087263
Cullather, R. I., Lim, Y.-K., Boisvert, L. N., Brucker, L., Lee, J. N., and Nowicki, S. M. J. (2016). Analysis of the Warmest Arctic winter, 2015-2016. Geophys. Res. Lett. 43, 10808–10816. doi:10.1002/2016GL071228
Cullather, R. I., and Nowicki, S. M. J. (2018). Greenland Ice Sheet Surface Melt and its Relation to Daily Atmospheric Conditions. J. Clim. 31, 1897–1919. doi:10.1175/jcli-d-17-0447.1
Cvijanovic, I., and Caldeira, K. (2015). Atmospheric Impacts of Sea Ice Decline in CO2 Induced Global Warming. Clim. Dyn. 44, 1173–1186. doi:10.1007/s00382-015-2489-1
Dai, A., Luo, D., Song, M., and Liu, J. (2019). Arctic Amplification Is Caused by Sea-Ice Loss under Increasing CO2. Nat. Commun. 10, 121. doi:10.1038/s41467-018-07954-9
Davini, P., and D’Andrea, F. (2020). From CMIP3 to CMIP6: Northern Hemisphere Atmospheric Blocking Simulation in Present and Future Climate. J. Clim. 33, 10021–10038. doi:10.1175/JCLI-D-19-0862.110.1175/JCLI-D-12-00032.1
Delhasse, A., Fettweis, X., Kittel, C., Amory, C., and Agosta, C. (2018). Brief Communication: Impact of the Recent Atmospheric Circulation Change in Summer on the Future Surface Mass Balance of the Greenland Ice Sheet. The Cryosphere 12, 3409–3418. doi:10.5194/tc-12-3409-2018
Derksen, C., and Brown, R. (2012). Spring Snow Cover Extent Reductions in the 2008-2012 Period Exceeding Climate Model Projections. Geophys. Res. Lett. 39, L19504. doi:10.1029/2012GL053387
Deser, C., Tomas, R. A., and Sun, L. (2015). The Role of Ocean-Atmosphere Coupling in the Zonal-Mean Atmospheric Response to Arctic Sea Ice Loss. J. Clim. 28, 2168–2186. doi:10.1175/JCLI-D-14-00325.1
Ding, Q., Schweiger, A., L’Heureux, M., Battisti, D. S., Po-Chedley, S., Johnson, N. C., et al. (2017). Influence of High-Latitude Atmospheric Circulation Changes on Summertime Arctic Sea Ice. Nat. Clim. Change 7, 289–295. doi:10.1038/nclimate3241
Ding, Q., Schweiger, A., L’Heureux, M., Steig, E. J., Battisti, D. S., Johnson, N. C., et al. (2019). Fingerprints of Internal Drivers of Arctic Sea Ice Loss in Observations and Model Simulations. Nat. Geosci 12, 28–33. doi:10.1038/s41561-018-0256-8
Ding, Q., Wallace, J. M., Battisti, D. S., Steig, E. J., Gallant, A. J. E., Kim, H. J., et al. (2014). Tropical Forcing of the Recent Rapid Arctic Warming in Northeastern Canada and Greenland. Nature 509, 209–212. doi:10.1038/nature13260
Dole, R. M., and Gordon, N. D. (1983). Persistent Anomalies of the Extratropical Northern Hemisphere Wintertime Circulation: Geographical Distribution and Regional Persistence Characteristics. Mon. Wea. Rev. 111 (8), 1567–1586. doi:10.1175/1520-0493(1983)111<1567:paoten>2.0.co;2
Doyle, J. G., Lesins, G., Thackray, C. P., Perro, C., Nott, G. J., Duck, T. J., et al. (2011). Water Vapor Intrusions into the High Arctic during winter. Geophys. Res. Lett. 38, L12806. doi:10.1029/2011gl047493
Dufour, A., Zolina, O., and Gulev, S. K. (2016). Atmospheric Moisture Transport to the Arctic: Assessment of Reanalyses and Analysis of Transport Components. J. Clim. 29, 5061–5081. doi:10.1175/JCLI-D-15-0559.1
Dunn‐Sigouin, E., and Son, S.-W. (2013). Northern Hemisphere Blocking Frequency and Duration in the CMIP5 Models. J. Geophys. Res. Atmos. 118, 1179–1188. doi:10.1002/jgrd.50143
Duynkerke, P., and Vandenbroeke, M. (1994). Surface Energy Balance and Katabatic Flow over Glacier and Tundra during GIMEX-91. Glob. Planet. Change 9, 17–28. doi:10.1016/0921-8181(94)90004-3
Else, B. G. T., Papakyriakou, T. N., Raddatz, R., Galley, R. J., Mundy, C. J., Barber, D. G., et al. (2014). Surface Energy Budget of Landfast Sea Ice during the Transitions from winter to Snowmelt and Melt Pond Onset: The Importance of Net Longwave Radiation and Cyclone Forcings. J. Geophys. Res. Oceans 119, 3679–3693. doi:10.1002/2013JC009672
Fang, Z. F. (2004). “Statistical Relationship between the Northern Hemisphere Sea Ice and Atmospheric Circulation during Wintertime,” in Observation, Theory and Modeling of Atmospheric Variability World Scientific Series on Asia-Pacific Weather and Climate. Editors Z. Xun, C. Ming, Z. Shuntai, Z. Yuejian, J. Fei-Fei, and Z. Xiaolei (Singapore: World Scientific Publishing Co), 131–141. doi:10.1142/9789812791139_0006
Fausto, R. S., As, D., Box, J. E., Colgan, W., Langen, P. L., and Mottram, R. H. (2016a). The Implication of Nonradiative Energy Fluxes Dominating Greenland Ice Sheet Exceptional Ablation Area Surface Melt in 2012. Geophys. Res. Lett. 43, 2649–2658. doi:10.1002/2016GL067720
Fausto, R. S., van As, D., Box, J. E., Colgan, W., and Langen, P. L. (2016b). Quantifying the Surface Energy Fluxes in South Greenland during the 2012 High Melt Episodes Using In-Situ Observations. Front. Earth Sci. 4, 82. doi:10.3389/feart.2016.00082
Fearon, M. G., Doyle, J. D., Ryglicki, D. R., Finocchio, P. M., and Sprenger, M. (2021). The Role of Cyclones in Moisture Transport into the Arctic. Geophys. Res. Lett. 48, e2020GL090353. doi:10.1029/2020GL090353
Feldl, N., Anderson, B. T., and Bordoni, S. (2017). Atmospheric Eddies Mediate Lapse Rate Feedback and Arctic Amplification. J. Clim. 30, 9213–9224. doi:10.1175/JCLI-D-16-0706.1
Feldl, N., Po-Chedley, S., Singh, H. K. A., Hay, S., and Kushner, P. J. (2020). Sea Ice and Atmospheric Circulation Shape the High-Latitude Lapse Rate Feedback. Npj Clim. Atmos. Sci. 3, 41. doi:10.1038/s41612-020-00146-7
Feng, J., Chen, W., and Li, Y. (2017). Asymmetry of the winter Extra-tropical Teleconnections in the Northern Hemisphere Associated with Two Types of ENSO. Clim. Dyn. 48, 2135–2151. doi:10.1007/s00382-016-3196-2
Feng, P. N., and Lin, H. (2019). Modulation of the MJO‐Related Teleconnections by the QBO. J. Geophys. Res. Atmos. 124, 12022–12033. doi:10.1029/2019JD030878
Fettweis, X., Hanna, E., Lang, C., Belleflamme, A., Erpicum, M., and Gallée, H. (2013). Brief Communication "Important Role of the Mid-tropospheric Atmospheric Circulation in the Recent Surface Melt Increase over the Greenland Ice Sheet". The Cryosphere 7, 241–248. doi:10.5194/tc-7-241-2013
Fettweis, X., Tedesco, M., Broeke, M., and Ettema, J. (2011). Melting Trends over the Greenland Ice Sheet (1958–2009) from Spaceborne Microwave Data and Regional Climate Models. EU: Publications and ResearchAvailable at: https://academicworks.cuny.edu/cc_pubs/673.
Flatau, M., and Kim, Y. J. (2013). Interaction Between the MJO and Polar Circulations. J. Climate 26 (11), 3562–3574. doi:10.1175/JCLI-D-11-00508.1
Francis, J. A., and Vavrus, S. J. (2015). Evidence for a Wavier Jet Stream in Response to Rapid Arctic Warming. Environ. Res. Lett. 10, 014005. doi:10.1088/1748-9326/10/1/014005
Francis, J. A., and Vavrus, S. J. (2012). Evidence Linking Arctic Amplification to Extreme Weather in Mid-latitudes. Geophys. Res. Lett. 39, L19804. doi:10.1029/2012GL051000
Franzke, C., Feldstein, S. B., and Lee, S. (2011). Synoptic Analysis of the Pacific-North American Teleconnection Pattern. Q.J.R. Meteorol. Soc. 137, 329–346. doi:10.1002/qj.768
Franzke, C. L. E., Jelic, D., Lee, S., and Feldstein, S. B. (2019). Systematic Decomposition of the MJO and its Northern Hemispheric Extratropical Response into Rossby and Inertio‐gravity Components. Q.J.R. Meteorol. Soc. 145, 1147–1164. doi:10.1002/qj.3484
Gadgil, S., and Srinivasan, J. (1990). Low Frequency Variation of Tropical Convergence Zones. Meteorl. Atmos. Phys. 44 (1–4), 119–132. doi:10.1007/BF01026814
Gallagher, M. R., Shupe, M. D., and Miller, N. B. (2018). Impact of Atmospheric Circulation on Temperature, Clouds, and Radiation at Summit Station, Greenland, with Self-Organizing Maps. J. Clim. 31, 8895–8915. doi:10.1175/JCLI-D-17-0893.1
Geb, M. (1966). Synoptic-statistical Investigations into the Initiation of Blocking Action over the North Atlantic and Europe. Meteorologishe Abhandlungen 69, 89.
Gong, T., Feldstein, S., and Lee, S. (2017). The Role of Downward Infrared Radiation in the Recent Arctic Winter Warming Trend. J. Clim. 30, 4937–4949. doi:10.1175/JCLI-D-16-0180.1
Goosse, H., Kay, J. E., Armour, K. C., Bodas-Salcedo, A., Chepfer, H., Docquier, D., et al. (2018). Quantifying Climate Feedbacks in Polar Regions. Nat. Commun. 9, 1919. doi:10.1038/s41467-018-04173-0
Goss, M., Feldstein, S. B., and Lee, S. (2016). Stationary Wave Interference and its Relation to Tropical Convection and Arctic Warming. J. Clim. 29, 1369–1389. doi:10.1175/JCLI-D-15-0267.1
Goss, M., and Feldstein, S. B. (2015). The Impact of the Initial Flow on the Extratropical Response to Madden-Julian Oscillation Convective Heating. Monthly Weather Rev. 143 (4), 1104–1121. doi:10.1175/MWR-D-14-00141.1
Graham, R. M., Cohen, L., Petty, A. A., Boisvert, L. N., Rinke, A., Hudson, S. R., et al. (2017a). Increasing Frequency and Duration of Arctic winter Warming Events. Geophys. Res. Lett. 44, 6974–6983. doi:10.1002/2017GL073395
Graham, R. M., Itkin, P., Meyer, A., Sundfjord, A., Spreen, G., Smedsrud, L. H., et al. (2019). Winter Storms Accelerate the Demise of Sea Ice in the Atlantic Sector of the Arctic Ocean. Sci. Rep. 9, 9222. doi:10.1038/s41598-019-45574-5
Graham, R. M., Rinke, A., Cohen, L., Hudson, S. R., Walden, V. P., Granskog, M. A., et al. (2017b). A Comparison of the Two Arctic Atmospheric winter States Observed during N‐ICE2015 and SHEBA. J. Geophys. Res. Atmos. 122, 5716–5737. doi:10.1002/2016JD025475
Grams, C. M., and Archambault, H. M. (2016). The Key Role of Diabatic Outflow in Amplifying the Midlatitude Flow: A Representative Case Study of Weather Systems Surrounding Western North Pacific Extratropical Transition. Mon. Wea. Rev. 144, 3847–3869. doi:10.1175/MWR-D-15-0419.1
Graversen, R. G., and Burtu, M. (2016). Arctic Amplification Enhanced by Latent Energy Transport of Atmospheric Planetary Waves. Q.J.R. Meteorol. Soc. 142, 2046–2054. doi:10.1002/qj.2802
Graversen, R. G., and Langen, P. L. (2019). On the Role of the Atmospheric Energy Transport in 2 × CO2-Induced Polar Amplification in CESM1. J. Clim. 32, 3941–3956. doi:10.1175/JCLI-D-18-0546.1
Graversen, R. G., Mauritsen, T., Drijfhout, S., Tjernström, M., and Mårtensson, S. (2011). Warm Winds from the Pacific Caused Extensive Arctic Sea-Ice Melt in Summer 2007. Clim. Dyn. 36, 2103–2112. doi:10.1007/s00382-010-0809-z
Graversen, R. G., and Wang, M. (2009). Polar Amplification in a Coupled Climate Model with Locked Albedo. Clim. Dyn. 33, 629–643. doi:10.1007/s00382-009-0535-6
Haertel, P. (2018). Sensitivity of the Madden Julian Oscillation to Ocean Warming in a Lagrangian Atmospheric Model. Climate 6, 45. doi:10.3390/cli6020045
Hahn, L. C., Armour, K. C., Battisti, D. S., Donohoe, A., Pauling, A. G., and Bitz, C. M. (2020). Antarctic Elevation Drives Hemispheric Asymmetry in Polar Lapse Rate Climatology and Feedback. Geophys. Res. Lett. 47, e2020GL088965. doi:10.1029/2020GL088965
Hahn, L., Ummenhofer, C. C., and Kwon, Y.-O. (2018). North Atlantic Natural Variability Modulates Emergence of Widespread Greenland Melt in a Warming Climate. Geophys. Res. Lett. 45, 9171–9178. doi:10.1029/2018GL079682
Häkkinen, S., Hall, D. K., Shuman, C. A., Worthen, D. L., and DiGirolamo, N. E. (2014). Greenland Ice Sheet Melt from MODIS and Associated Atmospheric Variability. Geophys. Res. Lett. 41, 1600–1607. doi:10.1002/2013GL059185
Hamill, T. M., and Kiladis, G. N. (2014). Skill of the MJO and Northern Hemisphere Blocking in GEFS Medium-Range Reforecasts. Monthly Weather Rev. 142 (2), 868–885. doi:10.1175/MWR-D-13-00199.1
Hanna, E., Cropper, T. E., Hall, R. J., and Cappelen, J. (2016). Greenland Blocking Index 1851-2015: a Regional Climate Change Signal. Int. J. Climatol. 36, 4847–4861. doi:10.1002/joc.4673
Hanna, E., Cropper, T. E., Jones, P. D., Scaife, A. A., and Allan, R. (2015). Recent Seasonal Asymmetric Changes in the NAO (A Marked Summer Decline and Increased winter Variability) and Associated Changes in the AO and Greenland Blocking Index. Int. J. Climatol. 35, 2540–2554. doi:10.1002/joc.4157
Hanna, E., Fettweis, X., and Hall, R. J. (2018a). Brief Communication: Recent Changes in Summer Greenland Blocking Captured by None of the CMIP5 Models. The Cryosphere 12, 3287–3292. doi:10.5194/tc-12-3287-2018
Hanna, E., Fettweis, X., Mernild, S. H., Cappelen, J., Ribergaard, M. H., Shuman, C. A., et al. (2014). Atmospheric and Oceanic Climate Forcing of the Exceptional Greenland Ice Sheet Surface Melt in Summer 2012. Int. J. Climatol. 34, 1022–1037. doi:10.1002/joc.3743
Hanna, E., Hall, R. J., Cropper, T. E., Ballinger, T. J., Wake, L., Mote, T., et al. (2018b). Greenland Blocking index Daily Series 1851-2015: Analysis of Changes in Extremes and Links with North Atlantic and UK Climate Variability and Change. Int. J. Climatol 38, 3546–3564. doi:10.1002/joc.5516
Hanna, E., Jones, J. M., Cappelen, J., Mernild, S. H., Wood, L., Steffen, K., et al. (2013). The Influence of North Atlantic Atmospheric and Oceanic Forcing Effects on 1900-2010 Greenland Summer Climate and Ice Melt/runoff. Int. J. Climatol. 33, 862–880. doi:10.1002/joc.3475
Hao, M., Lin, Y., Luo, Y., Nath, R., and Zhao, Z. (2021). The Impact of Atmospheric Moisture Transport on winter Arctic Warming: Radiation versus Latent Heat Release. Int. J. Climatol 41, 3982–3993. doi:10.1002/joc.7054
Hao, M., Luo, Y., Lin, Y., Zhao, Z., Wang, L., and Huang, J. (2019). Contribution of Atmospheric Moisture Transport to winter Arctic Warming. Int. J. Climatol 39, 2697–2710. doi:10.1002/joc.5982
Hartmann, D. L., and Michelsen, M. L. (1989). Intraseasonal Periodicities in Indian Rainfall. J. Atmos. Sci. 46 (18), 2838–2862. doi:10.1175/1520‐0469(1989)046<2838:IPIIR>2.0.CO;2
Hassanzadeh, P., and Kuang, Z. (2015). Blocking Variability: Arctic Amplification versus Arctic Oscillation. Geophys. Res. Lett. 42, 8586–8595. doi:10.1002/2015GL065923
He, J., Lin, H., and Wu, Z. (2011). Another Look at Influences of the Madden-Julian Oscillation on the Wintertime East Asian Weather. J. Geophys. Res. 116, D03109. doi:10.1029/2010JD014787
Hegyi, B. M., and Deng, Y. (2017). Dynamical and Thermodynamical Impacts of High- and Low-Frequency Atmospheric Eddies on the Initial Melt of Arctic Sea Ice. J. Clim. 30, 865–883. doi:10.1175/JCLI-D-15-0366.1
Hegyi, B. M., and Taylor, P. C. (2018). The Unprecedented 2016-2017 Arctic Sea Ice Growth Season: The Crucial Role of Atmospheric Rivers and Longwave Fluxes. Geophys. Res. Lett. 45, 5204–5212. doi:10.1029/2017GL076717
Henderson, G. R., Barrett, B. S., and M. Lafleur, D. (2014). Arctic Sea Ice and the Madden-Julian Oscillation (MJO). Clim. Dyn. 43, 2185–2196. doi:10.1007/s00382-013-2043-y
Henderson, G. R., Barrett, B. S., and South, K. (2017). Eurasian October Snow Water Equivalent: Using Self‐organizing Maps to Characterize Variability and Identify Relationships to the MJO. Int. J. Climatol. 37, 596–606. doi:10.1002/joc.4725
Henderson, G. R., Peings, Y., Furtado, J. C., and Kushner, P. J. (2018). Snow-atmosphere Coupling in the Northern Hemisphere. Nat. Clim Change 8, 954–963. doi:10.1038/s41558-018-0295-6
Henderson, S. A., Maloney, E. D., and Barnes, E. A. (2016). The Influence of the Madden-Julian Oscillation on Northern Hemisphere Winter Blocking. J. Clim. 29, 4597–4616. doi:10.1175/JCLI-D-15-0502.1
Henderson, S. A., and Maloney, E. D. (2018). The Impact of the Madden-Julian Oscillation on High-Latitude Winter Blocking during El Niño-Southern Oscillation Events. J. Clim. 31, 5293–5318. doi:10.1175/JCLI-D-17-0721.1
Hendon, H. H., and Salby, M. L. (1996). Planetary-scale Circulations Forced by Intraseasonal Variations of Observed Convection. J. Atmos. Sci. 53, 1751–1758. doi:10.1175/1520-0469(1996)053<1751:pscfbi>2.0.co;2
Hendon, H. H., and Salby, M. L. (1994). The Life Cycle of the Madden‐Julian Oscillation. J. Atmos. Sci. 51, 1751–1758. doi:10.1175/1520-0469(1994)051<2225:tlcotm>2.0.co;2
Hermann, M., Papritz, L., and Wernli, H. (2020). A Lagrangian Analysis of the Dynamical and Thermodynamic Drivers of Greenland Melt Events during 1979–2017. Weather Clim. Dyn. Discuss. 1, 497–518. doi:10.5194/wcd-1-497-2020
Higgins, R. W., and Mo, K. C. (1997). Persistent North Pacific Circulation Anomalies and the Tropical Intraseasonal Oscillation. J. Clim. 10, 223–244. doi:10.1175/1520-0442(1997)010<0223:pnpcaa>2.0.co;2
Higgins, R. W., Schemm, J.-K. E., Shi, W., and Leetmaa, A. (2000). Extreme Precipitation Events in the Western United States Related to Tropical Forcing. J. Clim. 13, 793–820. doi:10.1175/1520-0442(2000)013<0793:epeitw>2.0.co;2
Hofer, S., Tedstone, A. J., Fettweis, X., and Bamber, J. L. (2017). Decreasing Cloud Cover Drives the Recent Mass Loss on the Greenland Ice Sheet. Sci. Adv. 3, e1700584. doi:10.1126/sciadv.1700584
Hong, J.-Y., Kim, B.-M., Baek, E.-H., Kim, J.-H., Zhang, X., and Kim, S.-J. (2020). A Critical Role of Extreme Atlantic Windstorms in Arctic Warming. Asia-pacific J. Atmos. Sci. 56, 17–28. doi:10.1007/s13143-019-00123-y
Horton, D. E., Johnson, N. C., Singh, D., Swain, D. L., Rajaratnam, B., and Diffenbaugh, N. S. (2015). Contribution of Changes in Atmospheric Circulation Patterns to Extreme Temperature Trends. Nature 522, 465–469. doi:10.1038/nature14550
Hoskins, B. (1997). A Potential Vorticity View of Synoptic Development. Meteorol. App. 4, 325–334. doi:10.1017/S1350482797000716
Hoskins, B. J., and Karoly, D. J. (1981). The Steady Linear Response of a Spherical Atmosphere to thermal and Orographic Forcing. J. Atmos. Sci. 38 (6), 1179–1196. doi:10.1175/1520‐0469(1981)038<1179:TSLROA>2.0.CO;2
Hoskins, B. J., McIntyre, M. E., and Robertson, A. W. (1985). On the Use and Significance of Isentropic Potential Vorticity Maps. Quar- terly J. R. Meteorol. Soc. 111, 877–946. doi:10.1002/qj.49711147002
Hu, W., Liu, P., Zhang, Q., and He, B. (2019). Dominant Patterns of winter-time Intraseasonal Surface Air Temperature over the CONUS in Response to MJO Convections. Clim. Dyn. 53, 3917–3936. doi:10.1007/s00382-019-04760-x
Huang, Y., Dong, X., Bailey, D. A., Holland, M. M., Xi, B., DuVivier, A. K., et al. (2019). Thicker Clouds and Accelerated Arctic Sea Ice Decline: The Atmosphere‐Sea Ice Interactions in Spring. Geophys. Res. Lett. 46, 6980–6989. doi:10.1029/2019GL082791
Huntington, H. P., Danielson, S. L., Wiese, F. K., Baker, M., Boveng, P., Citta, J. J., et al. (2020). Evidence Suggests Potential Transformation of the Pacific Arctic Ecosystem Is Underway. Nat. Clim. Chang. 10, 342–348. doi:10.1038/s41558-020-0695-2
Hurrell, J. W., Kushnir, Y., Ottersen, G., and Visbeck, M. (2003). An Overview of the North Atlantic Oscillation. The North Atlantic Oscillation: Climate Significance and Environmental Impact. Editors J. W. Hurrell, Y. Kushnir, G. Ottersen, and M. Visbeck, Geophysical Monograph Series, Washington, DC: American Geophysical Union, 134, 1–36, 279.
Hurrell, J. W., Kushner, Y., and Visbeck, M. (2001). CLIMATE: The North Atlantic Oscillation. Science 291, 603–605. doi:10.1126/science.1058761
Hwang, Y.-T., Frierson, D. M. W., and Kay, J. E. (2011). Coupling between Arctic Feedbacks and Changes in Poleward Energy Transport. Geophys. Res. Lett. 38, L17704. doi:10.1029/2011gl048546
Inness, P. M., and Slingo, J. M. (2003). Simulation of the Madden-Julian Oscillation in a Coupled General Circulation Model. Part I: Comparison with Observations and an Atmosphere-Only GCM. J. Clim. 16 (3), 345–364. doi:10.1175/1520-0442(2003)016<0345:sotmjo>2.0.co;2
Izeboud, M., Lhermitte, S., Van Tricht, K., Lenaerts, J. T. M., Van Lipzig, N. P. M., and Wever, N. (2020). The Spatiotemporal Variability of Cloud Radiative Effects on the Greenland Ice Sheet Surface Mass Balance. Geophys. Res. Lett. 47, e2020GL087315. doi:10.1029/2020GL087315
Jeong, J.-H., Kim, B.-M., Ho, C.-H., and Noh, Y.-H. (2008). Systematic Variation in Wintertime Precipitation in East Asia by MJO-Induced Extratropical Vertical Motion. J. Clim. 21, 788–801. doi:10.1175/2007JCLI1801.1
Jiang, X., Adames, Á. F., Kim, D., Maloney, E. D., Lin, H., Kim, H., et al. (2020). Fifty Years of Research on the Madden‐Julian Oscillation: Recent Progress, Challenges, and Perspectives. J. Geophys. Res. Atmospheres 125 (17), e2019JD030911. doi:10.1029/2019jd030911
Jiménez-Esteve, B., and Domeisen, D. I. V. (2018). The Tropospheric Pathway of the ENSO-North Atlantic Teleconnection. J. Clim. 31, 4563–4584. doi:10.1175/JCLI-D-17-0716.1
Jin, F., and Hoskins, B. J. (1995). The Direct Response to Tropical Heating in a Baroclinic Atmosphere. J. Atmos. Sci. 52(3), 307–319. doi:10.1175/1520-0469(1995)052<0307:TDRTTH>2.0.CO;2
Johansson, E., Devasthale, A., Tjernström, M., Ekman, A. M. L., and L'Ecuyer, T. (2017). Response of the Lower Troposphere to Moisture Intrusions into the Arctic. Geophys. Res. Lett. 44, 2527–2536. doi:10.1002/2017GL072687
Johnson, N. C., Collins, D. C. S. B. F., Feldstein, S. B., L’Heureux, M. L., and Riddle, E. E. (2014). Skillful Wintertime North American Temperature Forecasts Out to 4 Weeks Based on the State of ENSO and the MJO*. Wea. Forecast. 29, 23–38. doi:10.1175/WAF-D-13-00102.1
Johnson, N. C., and Feldstein, S. B. (2010). The Continuum of North Pacific Sea Level Pressure Patterns: Intraseasonal, Interannual, and Interdecadal Variability. J. Clim. 23, 851–867. doi:10.1175/2009jcli3099.1
Jones, C., and Carvalho, L. M. V. (2014). Sensitivity to Madden-Julian Oscillation Variations on Heavy Precipitation over the Contiguous United States. Atmos. Res. 147-148, 10–26. doi:10.1016/j.atmosres.2014.05.002
Jones, C., Waliser, D. E., Lau, K. M., and Stern, W. (2004). The Madden–Julian Oscillation and its Impact on Northern Hemisphere Weather Predictability. Monthly Weather Rev. 132 (6), 1462–1471. doi:10.1175/1520-0493(2004)132<1462:TMOAII>2.0.CO;2
Joyce, T. M., Kwon, Y., Seo, H., and Ummenhofer, C. C. (2019). Meridional Gulf Stream Shifts Can Influence Wintertime Variability in the North Atlantic Storm Track and Greenland Blocking. Geophys. Res. Lett. 46, 1702–1708. doi:10.1029/2018GL08108
Jun, S. Y., Ho, C. H., Jeong, J. H., Choi, Y. S., and Kim, B. M. (2016). Recent Changes in winter Arctic Clouds and Their Relationships with Sea Ice and Atmospheric Conditions. Tellus A: Dynamic Meteorol. Oceanography 68, 29130. doi:10.3402/tellusa.v68.29130
Kang, W., and Tziperman, E. (2018). The MJO‐SSW Teleconnection: Interaction between MJO‐forced Waves and the Midlatitude Jet. Geophys. Res. Lett. 45 (9), 4400–4409. doi:10.1029/2018GL077937
Kapsch, M. L., Graversen, R. G., Economou, T., and Tjernström, M. (2014). The Importance of spring Atmospheric Conditions for Predictions of the Arctic Summer Sea Ice Extent. Geophys. Res. Lett. 41, 5288–5296. doi:10.1002/2014GL060826
Kapsch, M. L., Graversen, R. G., Tjernström, M., and Bintanja, R. (2016). The Effect of Downwelling Longwave and Shortwave Radiation on Arctic Summer Sea Ice. Radiat. Arctic Summer Sea Ice 29, 1143–1159. doi:10.1175/JCLI-D-15-0238.1
Kapsch, M. L., Graversen, R. G., and Tjernström, M. (2013). Springtime Atmospheric Energy Transport and the Control of Arctic Summer Sea-Ice Extent. Nat. Clim Change 3, 744–748. doi:10.1038/nclimate1884
Kapsch, M. L., Skific, N., Graversen, R. G., Tjernström, M., and Francis, J. A. (2019). Summers with Low Arctic Sea Ice Linked to Persistence of spring Atmospheric Circulation Patterns. Clim. Dyn. 52, 2497–2512. doi:10.1007/s00382-018-4279-z
Kay, J. E., L’Ecuyer, T., Chepfer, H., Loeb, N., Morrison, A., and Cesana, G. (2016). Recent Advances in Arctic Cloud and Climate Research. Curr. Clim. Change Rep. 2, 159–169. doi:10.1007/s40641-016-0051-9
Kayser, M., Maturilli, M., Graham, R. M., Hudson, S. R., Rinke, A., Cohen, L., et al. (2017). Vertical Thermodynamic Structure of the Troposphere during the Norwegian Young Sea ICE Expedition (N-Ice2015). J. Geophys. Res. Atmos. 122, 10855–10872. doi:10.1002/2016JD026089
Kennedy, D., Parker, T., Woollings, T., Harvey, B., and Shaffrey, L. (2016). The Response of High-Impact Blocking Weather Systems to Climate Change. Geophys. Res. Lett. 43, 7250–7258. doi:10.1002/2016GL069725
Kiladis, G. N., and Weickmann, K. M. (1992). Circulation Anomalies Associated with Tropical Convection during Northern winter. Mon. Wea. Rev. 120, 1900–1923. doi:10.1175/1520-0493(1992)120<1900:caawtc>2.0.co;2
Kim, B. M., Hong, J. Y., Jun, S. Y., Zhang, X., Kwon, H., Kim, S. J., et al. (2017). Major Cause of Unprecedented Arctic Warming in January 2016: Critical Role of an Atlantic Windstorm. Sci. Rep. 7, 40051. doi:10.1038/srep40051
Kim, B. M., Lim, G. H., and Kim, K. Y. (2006). A New Look at the Midlatitude-MJO Teleconnection in the Northern Hemisphere winter. Q. J. R. Meteorol. Soc. 132, 485–503. doi:10.1256/qj.04.87
Kim, B. M., Son, S. W., Min, S. K., Jeong, J. H., Kim, S. J., Zhang, X., et al. (2014). Weakening of the Stratospheric Polar Vortex by Arctic Sea-Ice Loss. Nat. Commun. 5, 4646. doi:10.1038/ncomms5646
Kim, H. M., and Kim, B. M. (2017). Relative Contributions of Atmospheric Energy Transport and Sea Ice Loss to the Recent Warm Arctic Winter. J. Clim. 30, 7441–7450. doi:10.1175/JCLI-D-17-0157.1
Klotzbach, P. J., Oliver, E. C. J., Leeper, R. D., and Schreck, C. J. (2016). The Relationship between the Madden-Julian Oscillation (MJO) and Southeastern New England Snowfall. Monthly Weather Rev. 144 (4), 1355–1362. doi:10.1175/MWR-D-15-0434.1
Knutson, T. R., and Weickmann, K. M. (1987). 30-60 Day Atmospheric Oscillations: Composite Life Cycles of Convection and Circulation Anomalies. Mon. Wea. Rev. 115, 1407–1436. doi:10.1175/1520-0493(1987)115<1407:daoclc>2.0.co;2
Kodaira, T., Waseda, T., Nose, T., and Inoue, J. (2020). Record High Pacific Arctic Seawater Temperatures and Delayed Sea Ice advance in Response to Episodic Atmospheric Blocking. Sci. Rep. 10, 20830. doi:10.1038/s41598-020-77488-y
Kohnemann, S. H. E., Heinemann, G., Bromwich, D. H., and Gutjahr, O. (2017). Extreme Warming in the Kara Sea and Barents Sea during the Winter Period 2000-16. J. Clim. 30, 8913–8927. doi:10.1175/JCLI-D-16-0693.1
Komatsu, K. K., Alexeev, V. A., Repina, I. A., and Tachibana, Y. (2018). Poleward Upgliding Siberian Atmospheric Rivers over Sea Ice Heat up Arctic Upper Air. Sci. Rep. 8, 2872. doi:10.1038/s41598-018-21159-6
Kosaka, Y., and Xie, S. P. (2013). Recent Global-Warming Hiatus Tied to Equatorial Pacific Surface Cooling. Nature 501 (7467), 403–407. doi:10.1038/nature12534
Krishnamurti, T. N., and Subrahmanyam, D. (1982). The 30–50 Day Mode at 850 Mb during MONEX. J. Atmos. Sci. 39 (9), 2088–2095. doi:10.1175/1520‐0469(1982)039<2088:TDMAMD>2.0.CO;2
Kwon, Y. O., Camacho, A., Martinez, C., and Seo, H. (2018). North Atlantic winter Eddy-Driven Jet and Atmospheric Blocking Variability in the Community Earth System Model Version 1 Large Ensemble Simulations. Clim. Dyn. 51, 3275–3289. doi:10.1007/s00382-018-4078-6
Kwon, Y. O., and Joyce, T. M. (2013). Northern Hemisphere Winter Atmospheric Transient Eddy Heat Fluxes and the Gulf Stream and Kuroshio-Oyashio Extension Variability. J. Clim. 26, 9839–9859. doi:10.1175/JCLI-D-12-00647.1
Kwon, Y. O., Seo, H., Ummenhofer, C. C., and Joyce, T. M. (2020). Impact of Multidecadal Variability in Atlantic SST on Winter Atmospheric Blocking. Blocking 33, 867–892. doi:10.1175/JCLI-D-19-0324.1
L'Heureux, M. L., and Higgins, R. W. (2008). Boreal winter Links between the Madden‐Julian Oscillation and the Arctic Oscillation. J. Clim. 21, 3040–3050.doi:10.1175/2007JCLI1955.1
Lafleur, D. M., Barrett, B. S., and Henderson, G. R. (2015). Some Climatological Aspects of the Madden-Julian Oscillation (MJO). J. Clim. 28, 6039–6053. doi:10.1175/JCLI-D-14-00744.1
Laliberté, F., and Kushner, P. J. (2014). Midlatitude Moisture Contribution to Recent Arctic Tropospheric Summertime Variability*. J. Clim. 27, 5693–5707. doi:10.1175/JCLI-D-13-00721.1
Lang, A., Yang, S., and Kaas, E. (2017). Sea Ice Thickness and Recent Arctic Warming. Geophys. Res. Lett. 44, 409–418. doi:10.1002/2016GL071274
Lau, K. M., and Chan, P. H. (1986). Aspects of the 40–50 Day Oscillation during the Northern Summer as Inferred from Outgoing Longwave Radiation. Monthly Weather Rev. 114 (7), 1354–1367. doi:10.1175/1520‐0493(1986)114<1354:AOTDOD>2.0.CO;2
Leathers, D. J., Yarnal, B., and Palecki, M. A. (1991). The Pacific/North American Teleconnection Pattern and United States Climate. Part I: Regional Temperature and Precipitation Associations. J. Clim. 4, 517–528. doi:10.1175/1520-0442(1991)004<0517:tpatpa>2.0.co;2
Lee, H. J., Kwon, M. O., Yeh, S. W., Kwon, Y.-O., Park, W., Park, J. H., et al. (2017). Impact of Poleward Moisture Transport from the North Pacific on the Acceleration of Sea Ice Loss in the Arctic since 2002. J. Clim. 30, 6757–6769. doi:10.1175/JCLI-D-16-0461.1
Lee, S., Gong, T., Feldstein, S. B., Screen, J. A., and Simmonds, I. (2017). Revisiting the Cause of the 1989-2009 Arctic Surface Warming Using the Surface Energy Budget: Downward Infrared Radiation Dominates the Surface Fluxes. Geophys. Res. Lett. 44, 10654–10661. doi:10.1002/2017GL075375
Lee, S., Gong, T., Johnson, N., Feldstein, S. B., and Pollard, D. (2011). On the Possible Link between Tropical Convection and the Northern Hemisphere Arctic Surface Air Temperature Change between 1958 and 2001. J. Clim. 24 (16), 4350–4367. doi:10.1175/2011jcli4003.1
Lei, R., Gui, D., Yuan, Z., Pang, X., Tao, D., and Zhai, M. (2020). Characterization of the Unprecedented Polynya Events north of Greenland in 2017/2018 Using Remote Sensing and Reanalysis Data. Acta Oceanol. Sin. 39, 5–17. doi:10.1007/s13131-020-1643-8
Lejenas, H., and Øakland, H. (1983). Characteristics of Northern Hemisphere Blocking as Determined from Long Time Series of Observational Data. Tellus 35, 350–362. doi:10.1111/j.1600.0870.1983.tb00210.x
Lenaerts, J. T. M., Medley, B., Broeke, M. R., and Wouters, B. (2019). Observing and Modeling Ice Sheet Surface Mass Balance. Rev. Geophys. 57, 376–420. doi:10.1029/2018RG000622
Lin, H., Brunet, G., and Derome, J. (2009). An Observed Connection between the North Atlantic Oscillation and the Madden-Julian Oscillation. J. Clim. 22 (2), 364–380. doi:10.1175/2008JCLI2515.1
Lin, H., and Brunet, G. (2018). Extratropical Response to the MJO: Nonlinearity and Sensitivity to the Initial State. J. Atmos. Sci. 75 (1), 219–234. doi:10.1175/JAS-D-17-0189.1
Lin, H., Brunet, G., and Mo, R. (2010). Impact of the Madden-Julian Oscillation on Wintertime Precipitation in Canada. Mon. Wea. Rev. 138, 3822–3839. doi:10.1175/2010MWR3363.1
Lin, H., and Brunet, G. (2009). The Influence of the Madden-Julian Oscillation on Canadian Wintertime Surface Air Temperature. Mon. Wea. Rev. 137, 2250–2262. doi:10.1175/2009MWR2831.1
Lin, H. (2015). Subseasonal Variability of North American Wintertime Surface Air Temperature. Clim. Dyn. 45, 1137–1155. doi:10.1007/s00382-014-2363-6
Liu, C., and Barnes, E. A. (2015). Extreme Moisture Transport into the Arctic Linked to Rossby Wave Breaking. J. Geophys. Res. Atmos. 120, 3774–3788. doi:10.1002/2014JD022796
Liu, P. (2013). Changes in a Modeled MJO with Idealized Global Warming. Clim. Dyn. 40 (3–4), 761–773. doi:10.1007/s00382-012-1323-2
Liu, P., Li, T., Wang, B., Zhang, M., Luo, J.-j., Masumoto, Y., et al. (2013). MJO Change with A1B Global Warming Estimated by the 40-km ECHAM5. Clim. Dyn. 41 (3–4), 1009–1023. doi:10.1007/s00382-012-1532-8
Liu, P., Zhang, Q., Zhang, C., Zhu, Y., Khairoutdinov, M., Kim, H., et al. (2016). A Revised Real-Time Multivariate MJO Index. Monthly Weather Rev. 144 (2), 627–642. doi:10.1175/MWR-D-15-0237.1
Long, X., and Robinson, W. A. (2017). Dynamical Heating of the Arctic Atmosphere during the Springtime Transition. J. Clim. 30, 9539–9553. doi:10.1175/JCLI-D-17-0333.1
Lu, J., and Cai, M. (2009). Seasonality of Polar Surface Warming Amplification in Climate Simulations. Geophys. Res. Lett. 36, L16704. doi:10.1029/2009GL040133
Ludwig, V., Spreen, G., Haas, C., Istomina, L., Kauker, F., and Murashkin, D. (2019). The 2018 North Greenland Polynya Observed by a Newly Introduced Merged Optical and Passive Microwave Sea-Ice Concentration Dataset. The Cryosphere 13, 2051–2073. doi:10.5194/tc-13-2051-2019
Lukens, K. E., Feldstein, S. B., Yoo, C., and Lee, S. (2017). The Dynamics of the Extratropical Response to Madden-Julian Oscillation Convection. Q.J.R. Meteorol. Soc. 143, 1095–1106. doi:10.1002/qj.2993
Luo, B., Luo, D., Wu, L., Zhong, L., and Simmonds, I. (2017). Atmospheric Circulation Patterns Which Promote winter Arctic Sea Ice Decline. Environ. Res. Lett. 12, 054017. doi:10.1088/1748-9326/aa69d0
Luo, D., Chen, X., Overland, J., Simmonds, I., Wu, Y., and Zhang, P. (2019). Weakened Potential Vorticity Barrier Linked to Recent Winter Arctic Sea Ice Loss and Midlatitude Cold Extremes. J. Clim. 32, 4235–4261. doi:10.1175/JCLI-D-18-0449.1
Lupo, A. R. (2020). Atmospheric Blocking Events: A Review. New York: Annals of the New York Academy of Sciences. doi:10.1111/nyas.14557
Lupo, A. R., Jensen, A. D., Mokhov, I. I., Alexander, V. T., Timothy, E., et al. (2019). Changes in Global Blocking Character during the Most Recent Decades. Atmosphere 1–19. doi:10.3390/atmos10020092
Madden, R. A., and Julian, P. R. (1972). Description of Global-Scale Circulation Cells in the Tropics with a 40-50 Day Period. J. Atmos. Sci. 29, 1109–1123. doi:10.1175/1520-0469(1972)029<1109:dogscc>2.0.co;2
Madden, R. A., and Julian, P. R. (1971). Detection of a 40-50 Day Oscillation in the Zonal Wind in the Tropical Pacific. J. Atmos. Sci. 28, 702–708. doi:10.1175/1520-0469(1971)028<0702:doadoi>2.0.co;2
Madden, R. A., and Julian, P. R. (1994). Observations of the 40-50-Day Tropical Oscillation-A Review. Mon. Wea. Rev. 122, 814–837. doi:10.1175/1520-0493(1994)122<0814:ootdto>2.0.co;2
Maloney, E. D., Adames, Á. F., and Bui, H. X. (2019). Madden-Julian Oscillation Changes under Anthropogenic Warming. Nat. Clim Change 9 (1), 26–33. doi:10.1038/s41558-018-0331-6
Maloney, E. D., and Hartmann, D. L. (2001). The Madden-Julian Oscillation, Barotropic Dynamics, and North Pacific Tropical Cyclone Formation. Part I: Observations. J. Atmos. Sci. 58 (17), 2545–2558. doi:10.1175/1520-0469(2001)058<2545:tmjobd>2.0.co;2
Maloney, E. D., and Xie, S. P. (2013). Sensitivity of Tropical Intraseasonal Variability to the Pattern of Climate Warming. J. Adv. Model. Earth Syst. 5, 32–47. doi:10.1029/2012MS000171
Manabe, S., and Wetherald, R. T. (1975). The Effects of Doubling the CO2Concentration on the Climate of a General Circulation Model. J. Atmos. Sci. 32, 3–15. doi:10.1175/1520-0469(1975)032<0003:teodtc>2.0.co;2
Marshall, A. G., Hudson, D., Wheeler, M. C., Hendon, H. H., and Alves, O. (2011). Assessing the Simulation and Prediction of Rainfall Associated with the MJO in the POAMA Seasonal Forecast System. Clim. Dyn. 37, 2129–2141. doi:10.1007/s00382-010-0948-2
Martineau, P., Chen, G., and Burrows, D. A. (2017). Wave Events: Climatology, Trends, and Relationship to Northern Hemisphere winter Blocking and Weather Extremes. J. Clim. 30, 5675–5697. doi:10.1175/jcli-d-16-0692.1
Masato, G., Hoskins, B. J., and Woollings, T. J. (2012). Wave-breaking Characteristics of Midlatitude Blocking. Q.J.R. Meteorol. Soc. 138, 1285–1296. doi:10.1002/qj.990
Masato, G., Hoskins, B. J., and Woollings, T. (2013a). Wave-Breaking Characteristics of Northern Hemisphere Winter Blocking: A Two-Dimensional Approach. J. Clim. 26, 4535–4549. doi:10.1175/JCLI-D-12-00240.1
Masato, G., Hoskins, B. J., and Woollings, T. (2013b). Winter and Summer Northern Hemisphere Blocking in CMIP5 Models. J. Clim. 26, 7044–7059. doi:10.1175/JCLI-D-12-00466.1
Matsueda, M., and Endo, H. (2017). The Robustness of Future Changes in Northern Hemisphere Blocking: a Large Ensemble Projection with Multiple Sea Surface Temperature Patterns. Geophys. Res. Lett. 44, 5158–5166. doi:10.1002/2017gl073336
Matsueda, S., and Takaya, Y. (2015). The Global Influence of the Madden-Julian Oscillation on Extreme Temperature Events*. J. Clim. 28, 4141–4151. doi:10.1175/jcli-d-14-00625.1
Matsumura, S., and Yamazaki, K. (2012). Eurasian Subarctic Summer Climate in Response to Anomalous Snow Cover. J. Clim. 25, 1305–1317. doi:10.1175/2011JCLI4116.1
Matsumura, S., Zhang, X., and Yamazaki, K. (2014). Summer Arctic Atmospheric Circulation Response to spring Eurasian Snow Cover and its Possible Linkage to Accelerated Sea Ice Decrease. J. Clim. 27, 6551–6558. doi:10.1175/JCLI-D-13-00549.1
Matthews, A. J., Hoskins, B. J., and Masutani, M. (2004). The Global Response to Tropical Heating in the Madden-Julian Oscillation during the Northern winter. Q. J. R. Meteorol. Soc. 130, 1991–2011. doi:10.1256/qj.02.123
Matthews, A. J., and Kiladis, G. N. (1999). The Tropical-Extratropical Interaction between High-Frequency Transients and the Madden-Julian Oscillation. Mon. Wea. Rev. 127, 661–677. doi:10.1175/1520-0493(1999)127<0661:tteibh>2.0.co;2
Mattingly, K. S., Mote, T. L., and Fettweis, X. (2018). Atmospheric River Impacts on Greenland Ice Sheet Surface Mass Balance. J. Geophys. Res. Atmos. 123, 8538–8560. doi:10.1029/2018JD028714
Mattingly, K. S., Mote, T. L., Fettweis, X., van As, D., Van Tricht, K., Lhermitte, S., et al. (2020). Strong Summer Atmospheric Rivers Trigger Greenland Ice Sheet Melt through Spatially Varying Surface Energy Balance and Cloud Regimes. J. Clim. 33, 6809–6832. doi:10.1175/JCLI-D-19-0835.1
McKenna, C. M., Bracegirdle, T. J., Shuckburgh, E. F., Haynes, P. H., and Joshi, M. M. (2018). Arctic Sea Ice Loss in Different Regions Leads to Contrasting Northern Hemisphere Impacts. Geophys. Res. Lett. 45, 945–954. doi:10.1002/2017GL076433
McLeod, J. T., Ballinger, T. J., and Mote, T. L. (2018). Assessing the Climatic and Environmental Impacts of Mid-tropospheric Anticyclones over Alaska. J. Climatol 38, 351–364. doi:10.1002/joc.5180
McLeod, J. T., and Mote, T. L. (2016). Linking Interannual Variability in Extreme Greenland Blocking Episodes to the Recent Increase in Summer Melting across the Greenland Ice Sheet. Int. J. Climatol. 36, 1484–1499. doi:10.1002/joc.4440
Merkouriadi, I., Cheng, B., Graham, R. M., Rösel, A., and Granskog, M. A. (2017). Critical Role of Snow on Sea Ice Growth in the Atlantic Sector of the Arctic Ocean. Geophys. Res. Lett. 44, 10479–10485. doi:10.1002/2017GL075494
Merkouriadi, I., Cheng, B., Hudson, S. R., and Granskog, M. A. (2020). Effect of Frequent winter Warming Events (Storms) and Snow on Sea-Ice Growth - a Case from the Atlantic Sector of the Arctic Ocean during the N-Ice2015 Campaign. Ann. Glaciol. 61, 164–170. doi:10.1017/aog.2020.25
Messori, G., Woods, C., and Caballero, R. (2018). On the Drivers of Wintertime Temperature Extremes in the High Arctic. J. Clim. 31, 1597–1618. doi:10.1175/JCLI-D-17-0386.1
Mewes, D., and Jacobi, C. (2019). Heat Transport Pathways into the Arctic and Their Connections to Surface Air Temperatures. Atmos. Chem. Phys. 19, 3927–3937. doi:10.5194/acp-19-3927-2019
Mewes, D., and Jacobi, C. (2020). Horizontal Temperature Fluxes in the Arctic in CMIP5 Model Results Analyzed with Self-Organizing Maps. Atmosphere 11, 251. doi:10.3390/atmos11030251
Middlemas, E. A., Kay, J. E., Medeiros, B. M., and Maroon, E. A. (2020). Quantifying the Influence of Cloud Radiative Feedbacks on Arctic Surface Warming Using Cloud Locking in an Earth System Model. Geophys. Res. Lett. 47, e2020GL089207. doi:10.1029/2020GL089207
Moore, G. W. K., Schweiger, A., Zhang, J., and Steele, M. (2018). What Caused the Remarkable February 2018 North Greenland Polynya?. Geophys. Res. Lett. 45, 13342–13350. doi:10.1029/2018GL080902
Moore, G. W. K. (2016). The December 2015 North Pole Warming Event and the Increasing Occurrence of Such Events. Sci. Rep. 6, 39084. doi:10.1038/srep39084
Moore, R. W., Martius, O., and Spengler, T. (2010). The Modulation of the Subtropical and Extratropical Atmosphere in the Pacific Basin in Response to the Madden–Julian Oscillation. Monthly Weather Rev. 138 (7), 2761–2779. doi:10.1175/2010MWR3194.1
Mori, M., and Watanabe, M. (2008). The Growth and Triggering Mechanisms of the PNA: A MJO-PNA Coherence. J. Meteorol. Soc. Jpn. 86, 213–236. doi:10.2151/jmsj.86.213
Mortin, J., Svensson, G., Graversen, R. G., Kapsch, M. L., Stroeve, J. C., and Boisvert, L. N. (2016). Melt Onset over Arctic Sea Ice Controlled by Atmospheric Moisture Transport. Geophys. Res. Lett. 43, 6636–6642. doi:10.1002/2016GL069330
Mote, T. L. (1998a). Mid-tropospheric Circulation and Surface Melt on theGreenland Ice Sheet. Part I: Atmospheric Teleconnections. Int. J. Climatol. 18, 111–129. doi:10.1002/(sici)1097-0088(199802)18:2<111::aid-joc227>3.0.co;2-x
Mote, T. L. (1998b). Mid-tropospheric Circulation and Surface Melt on the Greenland Ice Sheet. Part II: Synoptic Climatology. Int. J. Climatol. 18, 131–145. doi:10.1002/(sici)1097-0088(199802)18:2<131::aid-joc228>3.0.co;2-s
Mouginot, J., Rignot, E., Bjørk, A. A., van den Broeke, M., Millan, R., Morlighem, M., et al. (2019). Forty-six Years of Greenland Ice Sheet Mass Balance from 1972 to 2018. Proc. Natl. Acad. Sci. USA 116, 9239–9244. doi:10.1073/pnas.1904242116
Mundhenk, B. D., Barnes, E. A., and Maloney, E. D. (2016). All-season Climatology and Variability of Atmospheric River Frequencies over the North Pacific. J. Clim. 29, 4885–4903. doi:10.1175/JCLI-D-15-0655.1
Murakami, T. (1980). Empirical Orthogonal Function Analysis of Satellite‐observed Outgoing Longwave Radiation during Summer. Monthly Weather Rev. 108 (2), 205–222. doi:10.1175/1520‐0493(1980)108<0205:EOFAOS>2.0.CO;2
Murakami, T., and Nakazawa, T. (1985). Tropical 45 Day Oscillations during the 1979 Northern Hemisphere Summer. J. Atmos. Sci. 42 (11), 1107–1122. doi:10.1175/1520‐0469(1985)042<1107:TDODTN>2.0.CO;2
Naakka, T., Nygård, T., Vihma, T., Sedlar, J., and Graversen, R. (2019). Atmospheric Moisture Transport between Mid‐latitudes and the Arctic: Regional, Seasonal and Vertical Distributions. Int. J. Climatol 39, 2862–2879. doi:10.1002/joc.5988
Nabizadeh, E., Hassanzadeh, P., Yang, D., and Barnes, E. A. (2019). Size of the Atmospheric Blocking Events: Scaling Law and Response to Climate Change. Geophys. Res. Lett. 46, 13488–13499. doi:10.1029/2019gl084863
Nakamura, N., and Huang, C. S. Y. (2018). Atmospheric Blocking as a Traffic Jam in the Jet Stream. Science 361, 42–47. doi:10.1126/science.aat0721
Nash, D., Waliser, D., Guan, B., Ye, H., and Ralph, F. M. (2018). The Role of Atmospheric Rivers in Extratropical and Polar Hydroclimate. J. Geophys. Res. Atmos. 123, 6804–6821. doi:10.1029/2017JD028130
Neff, W., Compo, G. P., Martin Ralph, F., and Shupe, M. D. (2014). Continental Heat Anomalies and the Extreme Melting of the Greenland Ice Surface in 2012 and 1889. J. Geophys. Res. Atmos. 119, 6520–6536. doi:10.1002/2014JD021470
Nghiem, S. V., Hall, D. K., Mote, T. L., Tedesco, M., Albert, M. R., Keegan, K., et al. (2012). The Extreme Melt across the Greenland Ice Sheet in 2012. Geophys. Res. Lett. 39, 2012GL053611. doi:10.1029/2012GL053611
Noël, B., van de Berg, W. J., Lhermitte, S., and van den Broeke, M. R. (2019). Rapid Ablation Zone Expansion Amplifies north Greenland Mass Loss. Sci. Adv. 5, eaaw0123. doi:10.1126/sciadv.aaw0123
Nygård, T., Naakka, T., and Vihma, T. (2020). Horizontal Moisture Transport Dominates the Regional Moistening Patterns in the Arctic. J. Clim. 33, 6793–6807. doi:10.1175/JCLI-D-19-0891.1
Ogi, M., and Wallace, J. M. (2012). The Role of Summer Surface Wind Anomalies in the Summer Arctic Sea Ice Extent in 2010 and 2011. Geophys. Res. Lett. 39, L09704. doi:10.1029/2012gl051330
Oliver, E. C. J. (2015). Multidecadal Variations in the Modulation of Alaska Wintertime Air Temperature by the Madden-Julian Oscillation. Theor. Appl. Climatol. 121, 1–11. doi:10.1007/s00704-014-1215-y
Oltmanns, M., Straneo, F., and Tedesco, M. (2019). Increased Greenland Melt Triggered by Large-Scale, Year-Round Cyclonic Moisture Intrusions. The Cryosphere 13, 815–825. doi:10.5194/tc-13-815-2019
Overland, J. E., Francis, J. A., Hanna, E., and Wang, M. (2012). The Recent Shift in Early Summer Arctic Atmospheric Circulation. Geophys. Res. Lett. 39, L19804. doi:10.1029/2012GL053268
Overland, J. E., Turet, P., and Oort, A. H. (1996). Regional Variations of Moist Static Energy Flux into the Arctic. J. Clim. 9, 54–65. doi:10.1175/1520-0442(1996)009<0054:rvomse>2.0.co;2
Overland, J. E., Wang, M., and Ballinger, T. J. (2018). Recent Increased Warming of the Alaskan marine Arctic Due to Midlatitude Linkages. Adv. Atmos. Sci. 35, 75–84. doi:10.1007/s00376-017-7026-1
Papritz, L. (2020). Arctic Lower-Tropospheric Warm and Cold Extremes: Horizontal and Vertical Transport, Diabatic Processes, and Linkage to Synoptic Circulation Features. J. Clim. 33, 993–1016. doi:10.1175/JCLI-D-19-0638.1
Papritz, L., and Dunn‐Sigouin, E. (2020). What Configuration of the Atmospheric Circulation Drives Extreme Net and Total Moisture Transport into the Arctic. Geophys. Res. Lett. 47, 14. doi:10.1029/2020GL089769
Park, D. S. R., Lee, S., and Feldstein, S. B. (2015). Attribution of the Recent Winter Sea Ice Decline over the Atlantic Sector of the Arctic Ocean*. J. Clim. 28, 4027–4033. doi:10.1175/JCLI-D-15-0042.1
Park, H. S., Lee, S., Kosaka, Y., Son, S. W., and Kim, S. W. (2015a). The Impact of Arctic Winter Infrared Radiation on Early Summer Sea Ice. J. Clim. 28, 6281–6296. doi:10.1175/JCLI-D-14-00773.1
Park, H. S., Lee, S., Son, S. W., Feldstein, S. B., and Kosaka, Y. (2015b). The Impact of Poleward Moisture and Sensible Heat Flux on Arctic Winter Sea Ice Variability*. J. Clim. 28, 5030–5040. doi:10.1175/JCLI-D-15-0074.1
Park, K., Kang, S. M., Kim, D., Stuecker, M. F., and Jin, F. F. (2018). Contrasting Local and Remote Impacts of Surface Heating on Polar Warming and Amplification. J. Clim. 31, 3155–3166. doi:10.1175/JCLI-D-17-0600.1
Pelly, J. L., and Hoskins, B. J. (2003). A New Perspective on Blocking. J. Atmos. Sci. 60, 743–755. doi:10.1175/1520-0469(2003)060<0743:anpob>2.0.co;2
Persson, P. O. G. (2012). Onset and End of the Summer Melt Season over Sea Ice: thermal Structure and Surface Energy Perspective from SHEBA. Clim. Dyn. 39, 1349–1371. doi:10.1007/s00382-011-1196-9
Persson, P. O. G., Shupe, M. D., Perovich, D., and Solomon, A. (2017). Linking Atmospheric Synoptic Transport, Cloud Phase, Surface Energy Fluxes, and Sea-Ice Growth: Observations of Midwinter SHEBA Conditions. Clim. Dyn. 49, 1341–1364. doi:10.1007/s00382-016-3383-1
Petrie, R. E., Shaffrey, L. C., and Sutton, R. T. (2015). Atmospheric Response in Summer Linked to Recent Arctic Sea Ice Loss. Q.J.R. Meteorol. Soc. 141, 2070–2076. doi:10.1002/qj.2502
Pfahl, S., Schwierz, C., Croci-Maspoli, M., Grams, C. M., and Wernli, H. (2015). Importance of Latent Heat Release in Ascending Air Streams for Atmospheric Blocking. Nat. Geosci 8, 610–614. doi:10.1038/ngeo2487
Pithan, F., and Mauritsen, T. (2014). Arctic Amplification Dominated by Temperature Feedbacks in Contemporary Climate Models. Nat. Geosci 7, 181–184. doi:10.1038/ngeo2071
Polyakov, I. V., Alkire, M. B., Bluhm, B. A., Brown, K. A., Carmack, E. C., Chierici, M., et al. (2020a). Borealization of the Arctic Ocean in Response to Anomalous Advection from Sub-arctic Seas. Front. Mar. Sci. 7, 491. doi:10.3389/fmars.2020.00491
Polyakov, I. V., Pnyushkov, A. V., Alkire, M. B., Ashik, I. M., Baumann, T. M., Carmack, E. C., et al. (2017). Greater Role for Atlantic Inflows on Sea-Ice Loss in the Eurasian Basin of the Arctic Ocean. Science 356, 285–291. doi:10.1126/science.aai8204
Polyakov, I. V., Rippeth, T. P., Fer, I., Alkire, M. B., Baumann, T. M., Carmack, E. C., et al. (2020b). Weakening of Cold Halocline Layer Exposes Sea Ice to Oceanic Heat in the Eastern Arctic Ocean. Ocean 33, 8107–8123. doi:10.1175/JCLI-D-19-0976.1
Previdi, M., Janoski, T. P., Chiodo, G., Smith, K. L., and Polvani, L. M. (2020). Arctic Amplification: A Rapid Response to Radiative Forcing. Geophys. Res. Lett. 47, e2020GL089933. doi:10.1029/2020GL089933
Rex, D. F. (1950). Blocking Action in the Middle Troposphere and its Effect upon Regional Climate. Tellus 2, 196–211. doi:10.1111/j.2153-3490.1950.tb00331.x10.3402/tellusa.v2i3.8546
Riddle, E. E., Stoner, M. B., Johnson, N. C., L’Heureux, M. L., Collins, D. C., and Feldstein, S. B. (2013). The Impact of the MJO on Clusters of Wintertime Circulation Anomalies over the North American Region. Clim. Dyn. 40, 1749–1766. doi:10.1007/s00382-012-1493-y
Rinke, A., Knudsen, E. M., Mewes, D., Dorn, W., Handorf, D., Dethloff, K., et al. (2019a). Arctic Summer Sea Ice Melt and Related Atmospheric Conditions in Coupled Regional Climate Model Simulations and Observations. J. Geophys. Res. Atmos. 124, 6027–6039. doi:10.1029/2018JD030207
Rinke, A., Maturilli, M., Graham, R. M., Matthes, H., Handorf, D., Cohen, L., et al. (2017). Extreme Cyclone Events in the Arctic: Wintertime Variability and Trends. Environ. Res. Lett. 12, 094006. doi:10.1088/1748-9326/aa7def
Rinke, A., Segger, B., Crewell, S., Maturilli, M., Naakka, T., Nygård, T., et al. (2019b). Trends of Vertically Integrated Water Vapor over the Arctic during 1979-2016: Consistent Moistening All over?. J. Clim. 32, 6097–6116. doi:10.1175/JCLI-D-19-0092.1
Rodney, M., Lin, H., and Derome, J. (2013). Subseasonal Prediction of Wintertime North American Surface Air Temperature during Strong MJO Events. Monthly Weather Rev. 141 (8), 2897–2909. doi:10.1175/MWR-D-12-00221.1
Roundy, P. E., MacRitchie, K., Asuma, J., and Melino, T. (2010). Modulation of the Global Atmospheric Circulation by Combined Activity in the Madden-Julian Oscillation and the El Niño-Southern Oscillation during Boreal Winter. J. Clim. 23 (15), 4045–4059. doi:10.1175/2010JCLI3446.1
Roundy, P. E. (2012). “Tropical-extratropical Interactions,” in Intraseasonal Variability of the Atmosphere–Ocean Climate System. Editors W. K. M. Lau, and D. E. Waliser. 2nd ed. (Berlin: Heidelberg), 497–512.
Roundy, P. E. (2021). “The Association between the Phase Speed of the Madden-Julian Oscillation and Atmospheric Circulation,” in The Multiscale Global Monsoon System. 4th ed. Editors C. P. Chang, K. J. Ha, R. H. Johnson, G. N. C. Lau, and B. Wang (Singapore: World Scientific Publishing Co), 301–314. doi:10.1142/9789811216602_0024
Rushley, S. S., Kim, D., and Adames, Á. F. (2019). Changes in the MJO under Greenhouse Gas-Induced Warming in CMIP5 Models. J. Clim. 32, 803–821. doi:10.1175/jcli-d-18-0437.1
Sánchez, C., Methven, J., Gray, S., and Cullen, M. (2020). Linking Rapid Forecast Error Growth to Diabatic Processes. Q. J. R. Meteorol. Soc. 146, 3548–3569. doi:10.1002/qj.3861
Sardeshmukh, P. D., and Hoskins, B. J. (1988). The Generation of Global Rotational Flow by Steady Idealized Tropical Divergence. J. Atmos. Sci. 45, 1228–1251. doi:10.1175/1520-0469(1988)045<1228:tgogrf>2.0.co;2
Schaller, N., Sillmann, J., Anstey, J., Fischer, E. M., Grams, C. M., and Russo, S. (2018). Influence of Blocking on Northern European and Western Russian Heatwaves in Large Climate Model Ensembles. Environ. Res. Lett. 13, 054015. doi:10.1088/1748-9326/aaba55
Schiemann, R., Athanasiadis, P., Barriopedro, D., Doblas-Reyes, F., Lohmann, K., Roberts, M. J., et al. (2020). Northern Hemisphere Blocking Simulation in Current Climate Models: Evaluating Progress from the Climate Model Intercomparison Project Phase 5 to 6 and Sensitivity to Resolution. Weather Clim. Dynam. 1, 277–292. doi:10.5194/wcd-1-277-2020
Schreck, C. J., Cordeira, J. M., and Margolin, D. (2013). Which MJO Events Affect North American Temperatures?. Mon Weather Rev. 141, 3840–3850. doi:10.1175/mwr-d-13-00118.1
Schubert, J. J., Stevens, B., and Crueger, T. (2013). Madden-Julian Oscillation as Simulated by the MPI Earth System Model: Over the Last and into the Next Millennium. J. Adv. Model. Earth Syst. 5, 71–84. doi:10.1029/2012MS000180
Schwendike, J., Berry, G. J., Fodor, K., and Reeder, M. J. (2021). On the Relationship between the Madden‐Julian Oscillation and the Hadley and Walker Circulations. Geophys. Res. Atmos. 126, e2019JD032117. doi:10.1029/2019JD032117
Schwendike, J., Govekar, P., Reeder, M. J., Wardle, R., Berry, G. J., and Jakob, C. (2014). Local Partitioning of the Overturning Circulation in the Tropics and the Connection to the Hadley and Walker Circulations. J. Geophys. Res. Atmos. 119, 1322–1339. doi:10.1002/2013JD020742
Screen, J. A. (2013). Influence of Arctic Sea Ice on European Summer Precipitation. Environ. Res. Lett. 8, 044015. doi:10.1088/1748-9326/8/4/044015
Screen, J. A., and Simmonds, I. (2010). Increasing Fall-winter Energy Loss from the Arctic Ocean and its Role in Arctic Temperature Amplification. Geophys. Res. Lett. 37, L16707. doi:10.1029/2010gl044136
Sedlar, J. (2018). Spring Arctic Atmospheric Preconditioning: Do Not Rule Out Shortwave Radiation Just yet. J. Clim. 31, 4225–4240. doi:10.1175/JCLI-D-17-0710.1
Seo, K. H., Lee, H. J., and Frierson, D. M. W. (2016). Unraveling the Teleconnection Mechanisms that Induce Wintertime Temperature Anomalies over the Northern Hemisphere Continents in Response to the MJO. J. Atmos. Sci. 73, 3557–3571. doi:10.1175/JAS-D-16-0036.1
Seo, K. H., and Son, S. W. (2012). The Global Atmospheric Circulation Response to Tropical Diabatic Heating Associated with the Madden-Julian Oscillation during Northern Winter. J. Atmos. Sci. 69 (1), 79–96. doi:10.1175/2011JAS3686.1
Seo, K., and Lee, H. (2017). Mechanisms for a PNA-like Teleconnection Pattern in Response to the MJO. J. Atmos. Sci. 74 (6), 1767–1781. doi:10.1175/JAS-D-16-0343.1
Serreze, M. C., Barrett, A. P., Stroeve, J. C., Kindig, D. N., and Holland, M. M. (2009). The Emergence of Surface-Based Arctic Amplification. The Cryosphere 9, 11–19. doi:10.5194/tc-3-11-2009
Serreze, M. C., and Barry, R. G. (2011). Processes and Impacts of Arctic Amplification: A Research Synthesis. Glob. Planet. Change 77, 85–96. doi:10.1016/j.gloplacha.2011.03.004
Serreze, M. C., and Francis, J. A. (2006). The Arctic Amplification Debate. Climatic Change 76, 241–264. doi:10.1007/s10584-005-9017-y
Siew, P. Y. F., Li, C., Sobolowski, S. P., and King, M. P. (2020). Intermittency of Arctic-Mid-Latitude Teleconnections: Stratospheric Pathway between Autumn Sea Ice and the winter North Atlantic Oscillation. Weather Clim. Dynam. 1, 261–275. doi:10.5194/wcd-1-261-2020
Simpson, I. R., Bacmeister, J., Neale, R. B., Hannay, C., Gettelman, A., Garcia, R. R., et al. (2020). An Evaluation of the Large‐Scale Atmospheric Circulation and its Variability in CESM2 and Other CMIP Models. J. Geophys. Res. Atmos. 125, e2020JD032835. doi:10.1029/2020JD032835
Slater, T., Hogg, A. E., and Mottram, R. (2020). Ice-sheet Losses Track High-End Sea-Level Rise Projections. Nat. Clim. Chang. 10, 879–881. doi:10.1038/s41558-020-0893-y
Södergren, A. H., McDonald, A. J., and Bodeker, G. E. (2018). An Energy Balance Model Exploration of the Impacts of Interactions between Surface Albedo, Cloud Cover and Water Vapor on Polar Amplification. Clim. Dyn. 51, 1639–1658. doi:10.1007/s00382-017-3974-5
Song, E.-J., and Seo, K.-H. (2016). Past- and Present-Day Madden-Julian Oscillation in CNRM-CM5. Geophys. Res. Lett. 43, 4042–4048. doi:10.1002/2016GL068771
Sorteberg, A., and Walsh, J. E. (2008). Seasonal Cyclone Variability at 70°N and its Impact on Moisture Transport into the Arctic. Tellus A 60, 570–586. doi:10.1111/j.1600-0870.2007.00314.x10.1111/j.1600-0870.2008.00314.x
Stan, C., Straus, D. M., Frederiksen, J. S., Lin, H., Maloney, E. D., and Schumacher, C. (2017). Review of Tropical-Extratropical Teleconnections on Intraseasonal Time Scales. Rev. Geophys. 55, 902–937. doi:10.1002/2016RG000538
Steinfeld, D., Boettcher, M., Forbes, R., and Pfahl, S. (2020). The Sensitivity of Atmospheric Blocking to Upstream Latent Heating - Numerical Experiments. Weather Clim. Dynam. 1 (2), 405–426. doi:10.5194/wcd-1-405-2020
Steinfeld, D., and Pfahl, S. (2019). The Role of Latent Heating in Atmospheric Blocking Dynamics: a Global Climatology. Clim. Dyn. 53, 6159–6180. doi:10.1007/s00382-019-04919-6
Stramler, K., Del Genio, A. D., and Rossow, W. B. (2011). Synoptically Driven Arctic Winter States. J. Clim. 24, 1747–1762. doi:10.1175/2010JCLI3817.1
Stroeve, J., and Notz, D. (2018). Changing State of Arctic Sea Ice across All Seasons. Environ. Res. Lett. 13, 103001. doi:10.1088/1748-9326/aade56
Stuecker, M. F., Bitz, C. M., Armour, K. C., Proistosescu, C., Kang, S. M., Xie, S.-P., et al. (2018). Polar Amplification Dominated by Local Forcing and Feedbacks. Nat. Clim. Change 8, 1076–1081. doi:10.1038/s41558-018-0339-y
Subramanian, A., Jochum, M., Miller, A. J., Neale, R., Seo, H., Waliser, D., et al. (2014). The MJO and Global Warming: A Study in CCSM4. Clim. Dyn. 42 (7–8), 2019–2031. doi:10.1007/s00382-013-1846-1
Sun, L., Deser, C., and Tomas, R. A. (2015). Mechanisms of Stratospheric and Tropospheric Circulation Response to Projected Arctic Sea Ice Loss*. J. Clim. 28, 7824–7845. doi:10.1175/JCLI-D-15-0169.1
Tachibana, Y., Komatsu, K. K., Alexeev, V. A., Cai, L., and Ando, Y. (2019). Warm Hole in Pacific Arctic Sea Ice Cover Forced Mid-latitude Northern Hemisphere Cooling during winter 2017-18. Sci. Rep. 9, 5567. doi:10.1038/s41598-019-41682-4
Takahashi, C., Sato, N., Seiki, A., Yoneyama, K., and Shirooka, R. (2011). Projected Future Change of MJO and its Extratropical Teleconnection in East Asia during the Northern winter Simulated in IPCC AR4 Models. SOLA 7, 201–204. doi:10.2151/sola.2011-051
Taylor, P. C., Cai, M., Hu, A., Meehl, J., Washington, W., and Zhang, G. J. (2013). A Decomposition of Feedback Contributions to Polar Warming Amplification. J. Clim. 26, 7023–7043. doi:10.1175/JCLI-D-12-00696.1
Taylor, P., Hegyi, B., Boeke, R., and Boisvert, L. (2018). On the Increasing Importance of Air-Sea Exchanges in a Thawing Arctic: A Review. Atmosphere 9, 41. doi:10.3390/atmos9020041
Tedesco, M., Fettweis, X., Mote, T., Wahr, J., Alexander, P., Box, J. E., et al. (2013). Evidence and Analysis of 2012 Greenland Records from Spaceborne Observations, a Regional Climate Model and Reanalysis Data. The Cryosphere 7, 615–630. doi:10.7916/D8J38SGV.10.5194/tc-7-615-2013
Tedesco, M., and Fettweis, X. (2020). Unprecedented Atmospheric Conditions (1948-2019) Drive the 2019 Exceptional Melting Season over the Greenland Ice Sheet. The Cryosphere 14, 1209–1223. doi:10.5194/tc-14-1209-2020
Tedesco, M., Fettweis, X., van den Broeke, M. R., van de Wal, R. S. W., Smeets, C. J. P. P., van de Berg, W. J., et al. (2011). The Role of Albedo and Accumulation in the 2010 Melting Record in Greenland. Environ. Res. Lett. 6, 014005. doi:10.1088/1748-9326/6/1/014005
Tedesco, M., Mote, T., Fettweis, X., Hanna, E., Jeyaratnam, J., Booth, J. F., et al. (2016). Arctic Cut-Off High Drives the Poleward Shift of a New Greenland Melting Record. Nat. Commun. 7, 11723. doi:10.1038/ncomms11723
Thoman, R. L., Bhatt, U. S., Bieniek, P. A., Brettschneider, B. R., Brubaker, M., Danielson, S. L., et al. (2020). The Record Low Bering Sea Ice Extent in 2018: Context, Impacts, and an Assessment of the Role of Anthropogenic Climate Change. Bull. Am. Meteorol. Soc. 101, S53–S58. doi:10.1175/BAMS-D-19-0175.1
Thompson, D. W. J., and Wallace, J. M. (1998). The Arctic Oscillation Signature in the Wintertime Geopotential Height and Temperature fields. Geophys. Res. Lett. 25, 1297–1300. doi:10.1029/98GL00950
Tibaldi, S., and Molteni, F. (1990). On the Operational Predictability of Blocking. Tellus A 42, 343–365. doi:10.1034/j.1600-0870.1990.t01-2-00003.x
Tilinina, N., Gulev, S. K., and Bromwich, D. H. (2014). New View of Arctic Cyclone Activity from the Arctic System Reanalysis. Geophys. Res. Lett. 41, 1766–1772. doi:10.1002/2013GL058924
Tjernström, M., Shupe, M. D., Brooks, I. M., Achtert, P., Prytherch, J., and Sedlar, J. (2019). Arctic Summer Airmass Transformation, Surface Inversions, and the Surface Energy Budget. J. Clim. 32, 769–789. doi:10.1175/JCLI-D-18-0216.1
Tjernström, M., Shupe, M. D., Brooks, I. M., Persson, P. O. G., Prytherch, J., Salisbury, D. J., et al. (2015). Warm-air Advection, Air Mass Transformation and Fog Causes Rapid Ice Melt. Geophys. Res. Lett. 42, 5594–5602. doi:10.1002/2015GL064373
Treidl, R. A., Birch, E. C., and Sajecki, P. (1981). Blocking Action in the Northern Hemisphere: A Climatological Study. Atmosphere-Ocean 19 (1), 1–23. doi:10.1080/07055900.1981.9649096
Trenberth, K. E., and Solomon, A. (1994). The Global Heat Balance: Heat Transports in the Atmosphere and Ocean. Clim. Dynam. 10 (3), 107–134. 10.1007/BF00210625
Tseng, K. C., Barnes, E. A., and Maloney, E. D. (2018). Prediction of the Midlatitude Response to Strong Madden‐Julian Oscillation Events on S2S Time Scales. Geophys. Res. Lett. 45, 463–470. doi:10.1002/2017GL075734
Tseng, K., Maloney, E., and Barnes, E. A. (2020). The Consistency of MJO Teleconnection Patterns on Interannual Time Scales. J. Clim. 33 (9), 3471–3486. doi:10.1175/JCLI-D-19-0510.1
Tsubouchi, T., Våge, K., Hansen, B., Larsen, K. M. H., Østerhus, S., Johnson, C., et al. (2021). Increased Ocean Heat Transport into the Nordic Seas and Arctic Ocean over the Period 1993-2016. Nat. Clim. Chang. 11, 21–26. doi:10.1038/s41558-020-00941-3
Tyrlis, E., Bader, J., Manzini, E., and Matei, D. (2021). Reconciling Different Methods of High‐latitude Blocking Detection. Q. J. R. Meteorol. Soc. 147, 1070–1096. doi:10.1002/qj.3960
Tyrlis, E., and Hoskins, B. J. (2008). Aspects of a Northern Hemisphere Atmospheric Blocking Climatology. J. Atmos. Sci. 65, 1638–1652. doi:10.1175/2007JAS2337.1
van Angelen, J. H., van den Broeke, M. R., Wouters, B., and Lenaerts, J. T. M. (2014). Contemporary (1960-2012) Evolution of the Climate and Surface Mass Balance of the Greenland Ice Sheet. Surv. Geophys. 35, 1155–1174. doi:10.1007/s10712-013-9261-z
van den Broeke, M. R., and Gallée, H. (1996). Observation and Simulation of Barrier Winds at the Western Margin of the Greenland Ice Sheet. Q.J R. Met. Soc. 122, 1365–1383. doi:10.1002/qj.49712253407
Van Tricht, K., Lhermitte, S., Lenaerts, J. T. M., Gorodetskaya, I. V., L’Ecuyer, T. S., Noël, B., et al. (2016). Clouds Enhance Greenland Ice Sheet Meltwater Runoff. Nat. Commun. 7, 10266. doi:10.1038/ncomms10266
Vavrus, S. J., Wang, F., Martin, J. E., Francis, J. A., Peings, Y., and Cattiaux, J. (2017). Changes in North American Atmospheric Circulation and Extreme Weather: Influence of Arctic Amplification and Northern Hemisphere Snow Cover. J. Clim. 30, 4317–4333. doi:10.1175/JCLI-D-16-0762.1
Vázquez, M., Algarra, I., Eiras-Barca, J., Ramos, A. M., Nieto, R., and Gimeno, L. (2018). Atmospheric Rivers over the Arctic: Lagrangian Characterisation of Their Moisture Sources. Water 11, 41. doi:10.3390/w11010041
Vázquez, M., Nieto, R., Drumond, A., and Gimeno, L. (2016). Moisture Transport into the Arctic: Source-Receptor Relationships and the Roles of Atmospheric Circulation and Evaporation. J. Geophys. Res. Atmos. 121, 13493–13509. doi:10.1002/2016JD025400
Vecchi, G. A., and Bond, N. A. (2004). The Madden-Julian Oscillation (MJO) and Northern High Latitude Wintertime Surface Air Temperatures. Geophys. Res. Lett. 31, L04104. doi:10.1029/2003GL018645
Vial, J., and Osborn, T. J. (2012). Assessment of Atmosphere-Ocean General Circulation Model Simulations of winter Northern Hemisphere Atmospheric Blocking. Clim. Dyn. 39, 95–112. doi:10.1007/s00382-011-1177-z
Villamil-Otero, G. A., Zhang, J., He, J., and Zhang, X. (2018). Role of Extratropical Cyclones in the Recently Observed Increase in Poleward Moisture Transport into the Arctic Ocean. Adv. Atmos. Sci. 35, 85–94. doi:10.1007/s00376-017-7116-0
Wachowicz, L. J., Preece, J. R., Mote, T. L., Barrett, B. S., and Henderson, G. R. (2021). Historical Trends of Seasonal Greenland Blocking under Different Blocking Metrics. Int. J. Climatol 41, E3263–E3278. doi:10.1002/joc.6923
Wang, B., Lee, S.-S., Waliser, D. E., Zhang, C., Sobel, A., Maloney, E., et al. (2018). Dynamics-oriented Diagnostics for the Madden-Julian Oscillation. J. Clim. 31 (8), 3117–3135. doi:10.1175/JCLI-D-17-0332.1
Wang, J., Kim, H., Kim, D., Henderson, S. A., Stan, C., and Maloney, E. D. (2020a). MJO Teleconnections over the PNA Region in Climate Models. Part I: Performance- and Process-Based Skill Metrics. J. Clim. 33 (3), 1051–1067. doi:10.1175/JCLI-D-19-0253.1
Wang, J., Kim, H., Kim, D., Henderson, S. A., Stan, C., and Maloney, E. D. (2020b). MJO Teleconnections over the PNA Region in Climate Models. Part II: Impacts of the MJO and Basic State. J. Clim. 33 (12), 5081–5101. doi:10.1175/JCLI-D-19-0865.1
Wang, W., Zender, C. S., As, D., and Miller, N. B. (2019). Spatial Distribution of Melt Season Cloud Radiative Effects over Greenland: Evaluating Satellite Observations, Reanalyses, and Model Simulations against In Situ Measurements. J. Geophys. Res. Atmos. 124, 57–71. doi:10.1029/2018JD028919
Wang, W., Zender, C. S., and As, D. (2018). Temporal Characteristics of Cloud Radiative Effects on the Greenland Ice Sheet: Discoveries from Multiyear Automatic Weather Station Measurements. J. Geophys. Res. Atmos. 123, 11348–11361. doi:10.1029/2018JD028540
Ward, J. L., Flanner, M. G., and Dunn‐Sigouin, E. (2020). Impacts of Greenland Block Location on Clouds and Surface Energy Fluxes over the Greenland Ice Sheet. J. Geophys. Res. Atmos. 125, e2020JD033172. doi:10.1029/2020JD033172
Wernli, H., and Papritz, L. (2018). Role of Polar Anticyclones and Mid-latitude Cyclones for Arctic Summertime Sea-Ice Melting. Nat. Geosci 11, 108–113. doi:10.1038/s41561-017-0041-0
Wolding, B. O., Maloney, E. D., Henderson, S., and Branson, M. (2017). Climate Change and the M Adden‐ J Ulian O Scillation: A Vertically Resolved Weak Temperature Gradient Analysis. J. Adv. Model. Earth Syst. 9, 307–331. doi:10.1002/2016MS000843
Woods, C., Caballero, R., and Svensson, G. (2013). Large-scale Circulation Associated with Moisture Intrusions into the Arctic during winter. Geophys. Res. Lett. 40, 4717–4721. doi:10.1002/grl.50912
Woods, C., Caballero, R., and Svensson, G. (2017). Representation of Arctic Moist Intrusions in CMIP5 Models and Implications for Winter Climate Biases. J. Clim. 30, 4083–4102. doi:10.1175/JCLI-D-16-0710.1
Woods, C., and Caballero, R. (2016). The Role of Moist Intrusions in Winter Arctic Warming and Sea Ice Decline. J. Clim. 29, 4473–4485. doi:10.1175/JCLI-D-15-0773.1
Woollings, T., Barriopedro, D., Methven, J., Son, S.-W., Martius, O., Harvey, B., et al. (2018). Blocking and its Response to Climate Change. Curr. Clim. Change Rep. 4, 287–300. doi:10.1007/s40641-018-0108-z
Wu, B., Zhang, R., D'Arrigo, R., and Su, J. (2013). On the Relationship between Winter Sea Ice and Summer Atmospheric Circulation over Eurasia. J. Clim. 26, 5523–5536. doi:10.1175/JCLI-D-12-00524.1
Xu, D., Du, L., Ma, J., and Shi, H. (2020). Pathways of Meridional Atmospheric Moisture Transport in the central Arctic. Acta Oceanol. Sin. 39, 55–64. doi:10.1007/s13131-020-1598-9
Yadav, P., and Straus, D. M. (2017). Circulation Response to Fast and Slow MJO Episodes. Monthly Weather Rev. 145 (5), 1577–1596. doi:10.1175/MWR-D-16-0352.1
Yamanouchi, T. (2019). Arctic Warming by Cloud Radiation Enhanced by Moist Air Intrusion Observed at Ny-Ålesund, Svalbard. Polar Sci. 21, 110–116. doi:10.1016/j.polar.2018.10.009
Yang, W., and Magnusdottir, G. (2017). Springtime Extreme Moisture Transport into the Arctic and its Impact on Sea Ice Concentration. J. Geophys. Res. Atmos. 122, 5316–5329. doi:10.1002/2016JD026324
Yang, X.-Y., Fyfe, J. C., and Flato, G. M. (2010). The Role of Poleward Energy Transport in Arctic Temperature Evolution. Geophys. Res. Lett. 37, L14803. doi:10.1029/2010gl043934
Yao, W., Lin, H., and Derome, J. (2011). Submonthly Forecasting of winter Surface Air Temperature in North America Based on Organized Tropical Convection. Atmos. Ocean 49, 51–60. doi:10.1080/07055900.2011.556882
Yasunari, T. (1979). Cloudiness Fluctuations Associated with the Northern Summer Monsoon. J. Meteorol. Soc. Jpn. 57 (3), 16. doi:10.2151/jmsj1965.57.3_227
Yoo, C., Feldstein, S. B., and Lee, S. (2014). The Prominence of a Tropical Convective Signal in the Wintertime Arctic Temperature. Atmos. Sci. Lett. 15, 7–12. doi:10.1002/asl2.455
Yoo, C., Feldstein, S., and Lee, S. (2011). The Impact of the Madden-Julian Oscillation Trend on the Arctic Amplification of Surface Air Temperature during the 1979-2008 Boreal winter. Geophys. Res. Lett. 38, a–n. doi:10.1029/2011GL049881
Yoo, C., Lee, S., and Feldstein, S. B. (2012). Mechanisms of Arctic Surface Air Temperature Change in Response to the Madden-Julian Oscillation. J. Clim. 25, 5777–5790. doi:10.1175/JCLI-D-11-00566.1
Yoo, C., and Son, S. W. (2016). Modulation of the Boreal Wintertime Madden‐Julian Oscillation by the Stratospheric Quasi‐biennial Oscillation. Geophys. Res. Lett. 43, 1392–1398. doi:10.1002/2016GL067762
Yoshimori, M., Abe-Ouchi, A., and Laîné, A. (2017). The Role of Atmospheric Heat Transport and Regional Feedbacks in the Arctic Warming at Equilibrium. Clim. Dyn. 49, 3457–3472. doi:10.1007/s00382-017-3523-2
Yoshimori, M., Abe-Ouchi, A., Watanabe, M., Oka, A., and Ogura, T. (2014a). Robust Seasonality of Arctic Warming Processes in Two Different Versions of the MIROC GCM. J. Clim. 27, 6358–6375. doi:10.1175/JCLI-D-14-00086.1
Yoshimori, M., Watanabe, M., Abe-Ouchi, A., Shiogama, H., and Ogura, T. (2014b). Relative Contribution of Feedback Processes to Arctic Amplification of Temperature Change in MIROC GCM. Clim. Dyn. 42, 1613–1630. doi:10.1007/s00382-013-1875-9
You, C., Tjernström, M., and Devasthale, A. (2020). Warm-Air Advection over Melting Sea-Ice: A Lagrangian Case Study. Boundary-layer Meteorol. 179, 99–116. doi:10.1007/s10546-020-00590-1
Zhang, C., and Dong, M. (2004). Seasonality in the Madden-Julian Oscillation. J. Clim. 17 (16), 3169–3180. doi:10.1175/1520-0442(2004)017<3169:sitmo>2.0.co;2
Zhang, G., and Wang, Z. (2018). North Atlantic Extratropical Rossby Wave Breaking during the Warm Season: Wave Life Cycle and Role of Diabatic Heating. Mon. Wea. Rev. 146, 695–712. doi:10.1175/MWR-D-17-0204.1
Zhang, X., He, J., Zhang, J., Polyakov, I., Gerdes, R., Inoue, J., et al. (2013). Enhanced Poleward Moisture Transport and Amplified Northern High-Latitude Wetting Trend. Nat. Clim Change 3, 47–51. doi:10.1038/nclimate1631
Zheng, C., Chang, E. K. M., Kim, H., Zhang, M., and Wang, W. (2019). Subseasonal to Seasonal Prediction of Wintertime Northern Hemisphere Extratropical Cyclone Activity by S2S and NMME Models. J. Geophys. Res. Atmos. 124, 12057–12077. doi:10.1029/2019JD031252
Zheng, C., and Chang, E. K. M. (2019). The Role of MJO Propagation, Lifetime, and Intensity on Modulating the Temporal Evolution of the MJO Extratropical Response. J. Geophys. Res. Atmos. 124, 5352–5378. doi:10.1029/2019JD030258
Zheng, C., Kar-Man Chang, E., Kim, H.-M., Zhang, M., and Wang, W. (2018). Impacts of the Madden-Julian Oscillation on Storm-Track Activity, Surface Air Temperature, and Precipitation over North America. J. Clim. 31 (15), 6113–6134. doi:10.1175/JCLI‐D‐17‐0534.1
Zheng, C., and Chang, E. K. M (2020). The Role of Extratropical Background Flow in Modulating the MJO Extratropical Response. J. Clim. 33 (11), 4513–4536. doi:10.1175/JCLI-D-19-0708.1
Keywords: blocking, greenland, arctic amplification, moisture intrusions, intraseasonal
Citation: Henderson GR, Barrett BS, Wachowicz LJ, Mattingly KS, Preece JR and Mote TL (2021) Local and Remote Atmospheric Circulation Drivers of Arctic Change: A Review. Front. Earth Sci. 9:709896. doi: 10.3389/feart.2021.709896
Received: 14 May 2021; Accepted: 22 June 2021;
Published: 01 July 2021.
Edited by:
Peter L. Langen, Aarhus University, DenmarkReviewed by:
Hyo-Seok Park, Hanyang Universiy, South KoreaQinghua Ding, University of California, Santa Barbara, United States
Copyright © 2021 Henderson, Barrett, Wachowicz, Mattingly, Preece and Mote. This is an open-access article distributed under the terms of the Creative Commons Attribution License (CC BY). The use, distribution or reproduction in other forums is permitted, provided the original author(s) and the copyright owner(s) are credited and that the original publication in this journal is cited, in accordance with accepted academic practice. No use, distribution or reproduction is permitted which does not comply with these terms.
*Correspondence: Gina R. Henderson, Z2hlbmRlcnNAdXNuYS5lZHU=