- Department of Geosciences, Colorado State University, Fort Collins, CO, United States
Restoration aimed at rewetting the valley floor has the potential to increase organic carbon stock in the form of floodplain soil carbon, downed wood, and riparian vegetation. The primary goal of stream restoration is typically to restore habitat or maintain balance between natural ecosystem function and human land use. Although many benefits result from stream restoration, the carbon sequestration potential of different restoration approaches in diverse geographic settings has not yet been quantified. We investigate the carbon storage potential of restored stream segments (known as treatment segments) relative to otherwise analogous degraded and reference segments. We develop a conceptual framework to identify the conditions that maximize carbon storage in relation to characteristics of the river corridor and specific restoration practices and propose response surfaces for carbon storage. We illustrate application and quantification of the conceptual framework using data from a pilot study of treatment, degraded, and reference stream segments along two streams in Oregon, United States. The conceptual model is designed to help managers identify levels of hydrologic connectivity, channel and floodplain dynamics, floodplain vegetation, and other variables that may optimize carbon storage at a treatment site.
Introduction
Increasing concern about warming climate is driving increased interest in diverse forms of carbon sequestration (e.g., Lal, 2008; Villa and Bernal, 2018; Gifford, 2020). The saturated, reducing environment of wetlands limits microbial decay of organic material, and wetland soils therefore typically have much higher concentrations of organic carbon than nearby soils with lower soil moisture (e.g., Nahlik and Fennessy, 2016; Carnell et al., 2018). Wetlands can also sequester carbon at rates 30 to 50 times higher than forests (e.g., Tangen and Bansal, 2020). River floodplains that are hydrologically connected to the active channel can include substantial areas with ponds, lakes, and diverse types of wetlands (e.g., marshes, swamps, carrs, wet meadows). River floodplains can also be seasonally or perennially wet because of regional groundwater inputs associated with geologic structures (e.g., Tooth and McCarthy, 2007; Assine et al., 2015; Koltzer et al., 2019). Consequently, river floodplains can contain disproportionately large soil carbon stocks relative to adjacent uplands (Wohl et al., 2012).
Organic carbon stock in river corridors (active channel(s), floodplain, hyporheic zone) occurs primarily in the form of floodplain soil (here, soil refers to all floodplain sediment and includes litter and duff created by particulate organic matter smaller than large wood), downed dead wood pieces >10 cm in diameter and 1 m in length (hereafter, large wood), and living riparian vegetation (Sutfin et al., 2016). Active channels can contain substantial quantities of large wood (Triska, 1984; Wohl, 2014; Boivin et al., 2015), but the majority of carbon in most river corridors is found in the floodplain (e.g., Sutfin et al., 2016; Scott and Wohl, 2020). River restoration has traditionally been focused on the active channel but is gradually broadening to include an explicit focus on hydrologic connectivity within the river corridor. River restoration has also expanded to include processes that create and maintain desirable floodplain characteristics (Wohl et al., 2015), such as lateral channel migration that results in secondary or abandoned channels and associated habitat diversity (Hall et al., 2007). In the context of this broadening focus, river restoration strategies that have the potential to increase diverse forms of floodplain carbon stock may be justified as a mechanism of carbon sequestration.
The cumulative historic, contemporary, and potential future carbon stocks in river corridors worldwide have not been quantitatively estimated, but evidence suggests that this cumulative stock could be significant for the global carbon budget. The most recent estimates of this budget suggest fluxes of 9.6 Gt C per year from fossil-fuel emissions, 1.6 Gt C/y from land-use emissions, 2.5 Gt C/y into the oceans, and 3.4 Gt C/y into terrestrial C sequestration (Friedlingstein et al., 2020). Floodplains constitute about 9% of total land area (Nardi et al., 2019). Although the range of soil organic carbon stock (Mg C/ha) reported for floodplains is too large to justify assuming a single median value (e.g., Sutfin et al., 2016), soils are the largest terrestrial carbon reservoir (e.g., Scharlemann et al., 2014) and wetland soils have higher carbon concentrations than other soils (e.g., Nahlik and Fennessy, 2016). Wetlands store 20–30% of the estimated 1,500 Gt of global soil carbon, for example, but occupy 5–8% of land area (Nahlik and Fennessy, 2016). These numbers suggest that wetland floodplain soils can cumulatively create globally significant carbon sequestration.
Here, we propose a framework for using multiple lines of evidence to assess the carbon sequestration potential for a river corridor at the reach scale. We define a reach as a continuous length of river corridor that is at least several times the average bankfull channel width and has consistent channel and valley morphology as delineated based on valley floor width and gradient, channel planform, and flow regime. We also provide two case studies using recently completed stage 0 restoration sites in Oregon, United States. Stage 0 restoration refers to returning a river corridor to the stage 0 condition in the Cluer and Thorne (2014) stream evolution model. Stage 0 in this model is an anastomosing wet woodland or grassed wetland with a high floodplain water table that intersects the ground surface at least seasonally. Stream restoration to stage 0 conditions involves some combination of introducing in-channel obstacles such as large wood or beaver dam analogues to promote local aggradation and overbank flow (e.g., Pollock et al., 2014; Dixon et al., 2016) and regrading channel and floodplain topography to facilitate overbank flow and channel migration under an existing flow regime (Powers et al., 2019; Smith et al., 2020). Our case studies come from the South Fork McKenzie River and Deep Creek in Oregon, United States. At both sites large wood has been added and material from high surfaces was graded and used to fill previously straightened channel segments with the intent of facilitating more sinuous and multithread channel planforms. Our primary objective in this paper is to develop a methodology that can be used to identify river reaches with the greatest potential for carbon sequestration. We briefly illustrate the application of this methodology using preliminary data from the two case studies. We acknowledge that evaluating carbon stock at such recently restored sites does not effectively evaluate changes in carbon stock with time following river restoration. Rather, we use the case studies to illustrate the application and potential limitations of the method proposed here.
Materials and Methods
Conceptual Model of Factors Controlling Floodplain Carbon Stock
We start with a conceptual model of the factors that influence carbon stock (mass per unit area; e.g., Mg C/ha) in floodplain soil and large wood. We do not address carbon stock in living floodplain vegetation, which typically forms a smaller proportion of total floodplain carbon stock than does soil carbon (Wohl et al., 2012; Hanberrry et al., 2015; Sutfin et al., 2016). We also do not explicitly address human activities in the drainage basin or the river corridor, which can either increase or decrease carbon stock by modifying the variables included in the conceptual model (Wohl et al., 2017).
Floodplain Soil Carbon Stock
Biogeochemists conceptualize wetland carbon sequestration as a balance between carbon inputs and outputs, which can also be done for floodplain soil carbon as a whole (e.g., Wohl et al., 2017). Inputs are primarily carbon within organic matter from plants (autochthonous) and carbon dissolved and suspended in inflowing waters or deposited from hillslope sources during slope wash or mass movements (allochthonous; this can originate from rock weathering as petrogenic carbon or from biotic processes as biospheric carbon; Blattmann et al., 2018). Outputs consist of dissolved and suspended organic carbon in outflowing waters, and CO2 and CH4 emissions from microbial decomposition of organic matter (Villa and Bernal, 2018). Organic matter decomposition by microbes can be slowed by 1) scarcity of nutrients that limit microbial growth, 2) physical protection of organic matter contained within soil aggregates, 3) a high proportion of organic compounds that are recalcitrant and difficult for microbes to degrade, 4) cold temperatures that limit plant productivity but also microbial activity, and 5) anaerobic conditions that force microbes to use less efficient metabolic pathways than exist under aerobic conditions (Villa and Bernal, 2018). Although carbon dioxide and methane emissions from wetlands can form a significant source of wetland carbon loss, particularly as climate warms, the large carbon stocks in wetland soils suggest that naturally functioning (rather than degraded) wetlands in temperate and boreal latitudes typically have a positive carbon balance and sequester carbon over periods of decades to millennia (e.g., Whiting and Chanton, 2001). Consequently, the remainder of this paper focuses on influences on carbon sequestration. When referring to organic carbon present in soil, we are including carbon derived from autochthonous and allochthonous processes. We are using soil to refer to all floodplain sediments.
The carbon concentration of soil (% carbon) depends on multiple factors, including soil texture and the ability of carbon to sorb to soil particles; particulate and dissolved organic matter inputs; and temperature and moisture regimes, which influence microbial oxidation of soil carbon (Kaiser and Guggenberger, 2000; Rasmussen et al., 2018). In general, carbon concentration increases with clay content, organic matter inputs, and moisture, and correlates inversely with temperature (Jobbágy and Jackson, 2000; Falloon et al., 2011; Sutfin et al., 2016).
Starting with these immediate influences on soil carbon concentration, we can infer the reach-scale drivers of clay content, organic matter inputs, soil moisture, and temperature across various temporal and spatial scales.
At the largest scales of time and space, geology and climate interact to govern the physical configuration of the river corridor, the weathering of bedrock, the fluxes of material into and through the river corridor, and the biotic communities present (upper, central box in Figure 1). Geology and climate govern valley geometry and the space available for floodplain storage (physical configuration); as well as soil texture, floodplain water table, and soil organic matter (material fluxes). The influences of geology and climate on these properties are direct but are also mediated by geomorphic and biotic drivers as listed in the second tier of boxes in Figure 1. All of the factors mentioned thus far interact to determine the residence time of floodplain sediment. Residence time, along with soil texture, moisture, and organic matter content, determine soil organic carbon stock.
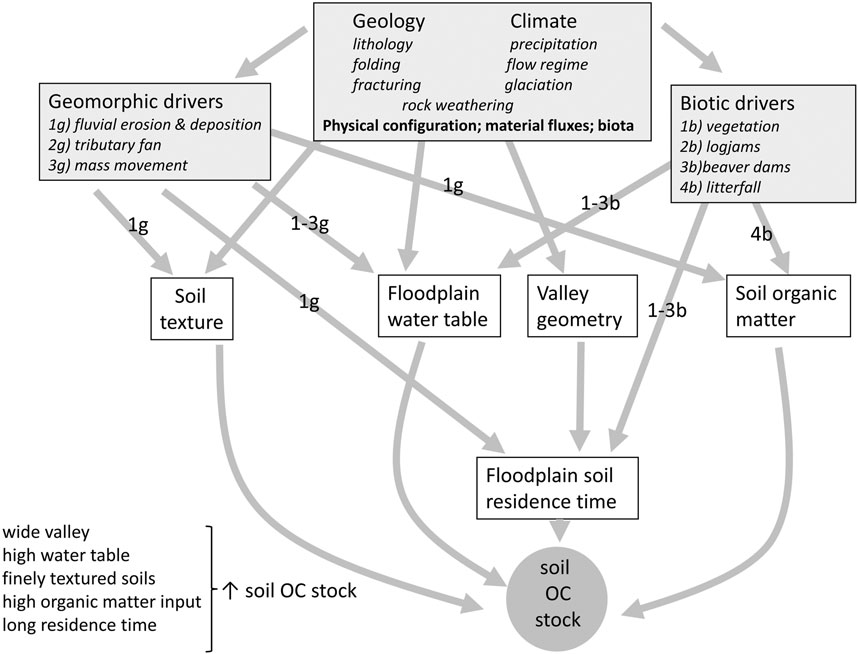
FIGURE 1. Conceptual model for influences on floodplain soil organic carbon stock. The numbers and letters superimposed on arrows represent the specific geomorphic (“g”) and biotic (“b”) drivers indicated by each arrow.
Human activities are not explicitly illustrated in the conceptual model but can alter the forms and processes of stream corridors in manners that influence floodplain carbon stock (e.g., floodplain drainage that lowers the water table and creates oxidizing conditions in floodplain soils).
Geologic characteristics influence soil carbon via controls on valley geometry, floodplain water table, and soil texture. 1) Valley geometry. Bedrock structure, glacial history, and the spatial density of bedrock fracturing influence the width and downstream gradient of the valley floor and thus the space available for floodplain development. Structures such as grabens (Koltzer et al., 2019), glacially eroded troughs (Montgomery, 2002; Livers and Wohl, 2015), and dense fracturing that reduces bedrock resistance to weathering and erosion (Ehlen and Wohl, 2002) promote the presence of a wide, low gradient valley floor at the reach scale and this valley geometry facilitates sediment storage in the floodplain. 2) Floodplain water table. Geologic characteristics can also promote the presence of a high water table, even in dry climates. Examples of such characteristics include structures that retain groundwater inputs from adjacent uplands (Koltzer et al., 2019); folded aquifer units (DesRoches et al., 2014); lithologic contacts between units with differing hydraulic conductivity (Muldoon et al., 2001); resistant lithologies that create a local base level and upstream alluvial depositional zone (Tooth and McCarthy, 2007); undulating bedrock topography that facilitates high water tables where bedrock is close to the surface (Hardie et al., 2012); and fractured bedrock that transmits groundwater readily to topographic lows (Briggs and Hare, 2018). 3) Soil texture. Lithology, in combination with climate and land cover, influences weathering regime and thus the soil texture of the floodplain.
Geomorphic processes influence soil carbon via controls on floodplain water table, soil texture, organic matter input, and sediment residence time in the floodplain. 1) Floodplain water table. Lateral and end moraines can influence groundwater fluxes and valley floor gradient in a manner that promotes a high water table (e.g., Christensen et al., 2020). Similarly, tributary alluvial fans and debris-flow fans, talus slopes, and landslide deposits can influence subsurface and surface water fluxes and create a high water table. Alluvial fans, for example, can serve as groundwater recharge zones, with a line of springs along the base of the fan (Miller et al., 2012). 2) Soil texture. The spatial pattern, rate, and frequency of fluvial erosion and deposition strongly influence the thickness, stratigraphy, and texture of floodplain alluvium (Bridge, 2003). 3) Organic matter input. Overbank deposition of particulate organic matter in transport within the channel reflects upstream sources of organic matter, but also the spatial details of deposition of upstream-sourced organic matter, as governed by hydrologic connectivity (Hupp et al., 2019). 4) Residence time. The balance between lateral and vertical accretion on the floodplain, as a function of active channel dynamics, exerts a fundamental control on the average residence time of sediment on the floodplain (Wittmann and von Blanckenburg, 2009; Wohl, 2015). A longer residence time can equate to higher soil carbon concentrations and stock if organic matter inputs continue through time (Lininger et al., 2018).
Biotic communities influence soil carbon via controls on floodplain water table, organic matter input via litterfall, and sediment residence time. 1) Floodplain water table. Living vegetation, beaver dams, and large wood can increase frictional resistance along channels and create obstructions to flow (Larsen and Harvey, 2010; Collins et al., 2012; Aberle and Järvelä, 2013). This can enhance hyporheic exchanges (Lautz et al., 2006; Sawyer and Cardenas, 2012; Doughty et al., 2020) and overbank flows (Westbrook et al., 2006; Oswald and Wohl, 2008) that result in a higher water table (Larsen et al., 2016). 2) Organic matter input. The primary productivity of floodplain vegetation and the rate of litterfall largely govern the organic matter added to floodplain soils along portions of a floodplain with dense vegetation and limited surface hydrologic connectivity with the active channel (Lininger et al., 2018; Hupp et al., 2019), although overbank deposition of fluvially transported organic matter can be important on some floodplains. 3) Sediment residence time. Sediment residence time is fundamentally a function of bank erosion and floodplain surface erosion, but these fluvial processes can be strongly mediated by floodplain vegetation, beaver dams, and large wood that increase streambank and floodplain erosional resistance and reduce the hydraulic forces of flow within the channel and across the floodplain (e.g., Micheli and Kirchner, 2002; Perignon et al., 2013).
Floodplain Large Wood Carbon Stock
The carbon concentration of large wood varies by tree species (Martin et al., 2018) but is typically on the order of 50%. The amount of large wood stored on the floodplain reflects a wood budget governed by inputs and outputs. Inputs occur as tree fall on the floodplain, mass movements that introduce large wood to the floodplain, and fluvial transport of large wood onto the floodplain (Wohl, 2020). Outputs occur as decay in situ and fluvial transport of large wood out of the floodplain. As for soil carbon, we can start with these immediate influences on floodplain large wood load (m3 wood/ha) and infer the reach-scale drivers of large wood inputs and outputs at larger temporal and spatial scales.
Geology and climate are the primary influences on valley configuration, material fluxes, and biotic communities, as explained in reference to Figure 1. Geology and climate also influence the geomorphic and biotic drivers that are particularly relevant to floodplain large wood load (Figure 2). In particular, geomorphic and biotic drivers influence the supply of wood to the floodplain and the retention of wood on the floodplains. Again, the conceptual model does not explicitly include human activities, although many human activities (e.g., timber harvest, channelization, beaver trapping) strongly influence floodplain large wood load.
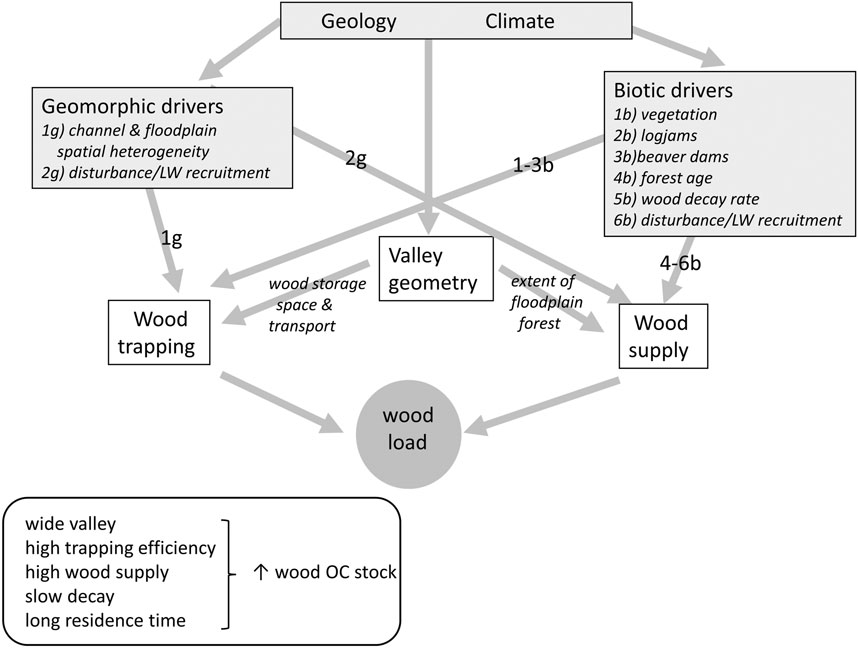
FIGURE 2. Conceptual model for influences on floodplain organic carbon stock in large wood. As in Figure 1, the numbers and letters superimposed on arrows represent the specific geomorphic (“g”) and biotic (“b”) drivers indicated by each arrow.
Geology and climate influence wood load via controls (weathering and erosion) on valley geometry, which then influences wood supply and wood trapping. The controls on valley geometry are as described for floodplain soil carbon. The width of the floodplain influences wood supply via the spatial extent of the floodplain forest and the potential for hillslope mass movements such as avalanches, landslides, or debris flows to directly introduce large wood to the floodplain (Wohl, 2020). The width of the floodplain and the channel planform also influence the retention of large wood moving down the river corridor. Wider reaches with anastomosing channel planform, bars and islands, and sinuous channels can be more effective at trapping and retaining large wood in transport than relatively straight, narrow channels (Gurnell et al., 2000; Wyzga and Zawiejska, 2005; Lassettre et al., 2008; Wohl and Cadol, 2011). Lateral channel migration and avulsion across broad floodplains can leave large wood that is buried and accreted to the floodplain (O’Connor et al., 2003; Guyette et al., 2008; Collins et al., 2012) and then sometimes exhumed and returned to the channel (Benda and Sias, 2003).
Geomorphic processes influence large wood load via controls on wood supply and wood trapping. 1) Wood supply. The supply of large wood to the floodplain partly reflects recruitment of living trees through bank erosion that topples the trees. Although many of these trees fall into the active channel and are carried downstream, others remain on the floodplain. Channel dynamics also indirectly influence wood supply by creating disturbances (erosion, deposition) that can limit the age of floodplain forests and create new germination sites for trees (e.g., Everitt, 1968). 2) Wood trapping. As noted in the description of interactions between valley geometry and wood trapping, the details of channel and floodplain spatial heterogeneity strongly influence how effectively wood being transported downstream in the active channel is trapped and retained on the floodplain (Scott and Wohl, 2018b). Numerous studies indicate that wood preferentially accumulates on geomorphic features such as bars, islands, meander bends, and secondary channels, as noted above, and these wood accumulations can be incorporated into the floodplain via lateral accretion.
Biotic communities influence floodplain large wood load through controls on wood supply and wood trapping. 1) Wood supply reflects the age of the forest and the processes of mortality and disturbance that change living trees to downed, dead wood. Individual tree mortality and mass mortality associated with wildfires, drought, insect infestations, and blowdowns can all recruit wood to the floodplain (Wohl, 2020). In regions with very rapid wood decay, however, such as the tropics (e.g., Clark et al., 2002), wood load may remain low despite substantial recruitment. 2) Wood trapping can reflect characteristics of floodplain vegetation, as well as geomorphically induced heterogeneity. Dense herbaceous or shrubby vegetation and closely spaced trees can limit and direct overbank transport of large wood (Wohl et al., 2018a,b) and significantly increase trapping and retention of large wood on floodplains.
Prediction of Floodplain Carbon Stock
Understanding the variables and interactions described in the preceding section can be used to identify reaches within a river network that have the potential for greater floodplain carbon stock in the form of soil carbon and/or large wood. Several sources of information can also be used to quantitatively constrain predictions of floodplain carbon stock. Among these are published values of carbon stock (Table 1) and rates (Table 2) of carbon inputs in diverse floodplains. This database is limited but steadily growing and at a minimum provides likely upper and lower bounds for floodplain carbon stock in a variety of field settings.
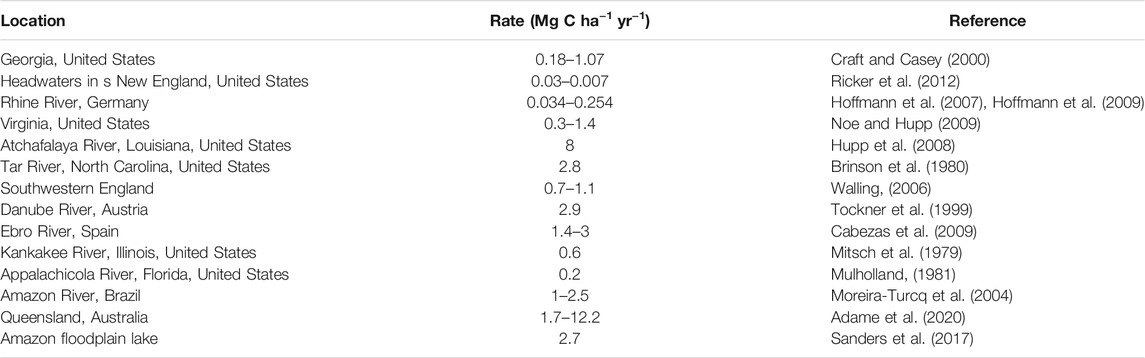
TABLE 2. Published values for soil organic carbon accumulation rates on floodplains (after Sutfin et al., 2016; Table 3).
We used the data in Table 1 to create preliminary illustrations of the magnitude of floodplain carbon stocks in the form of large wood and soil in relation to mean annual precipitation and temperature (Figure 3). These are preliminary versions for several reasons. First, precipitation and temperature are not necessarily the most important controls on either form of floodplain carbon stock, but they do represent variables that are commonly reported or relatively easily accessible for published field site locations. Both floodplain wood load and soil organic carbon concentration can increase with the age of the floodplain surface, for example, as a maturing floodplain forest provides a recruitment source for larger and more abundant downed wood and as litterfall from floodplain plants accumulates in reducing soils (Scott and Wohl, 2018a; Lininger et al., 2018). Similarly, within a spatially extensive floodplain on a large river, local differences in soil moisture can equate to significant differences in soil carbon stock, despite a consistent climate across the floodplain (e.g., Lininger et al., 2018). Consequently, differences in the ages or site-specific soil moisture of floodplain surfaces included in the dataset could produce trends in Figure 3 that might prove to be misleading as more quantifications of floodplain carbon stocks are published. Second, the number of published values is limited and these values do not fully represent the range of floodplain soil carbon or large wood carbon stocks likely to be present along rivers. Finally, we used median values to create a data point for each published case study. Most of the case studies included a range of values that reflect the local variation in floodplain carbon stock. Despite these caveats, we believe that in the absence of published floodplain carbon stocks for a particular geographic region, the data in Figure 3 provide a starting point for estimating potential floodplain carbon stocks (Supplementary Data Tables).
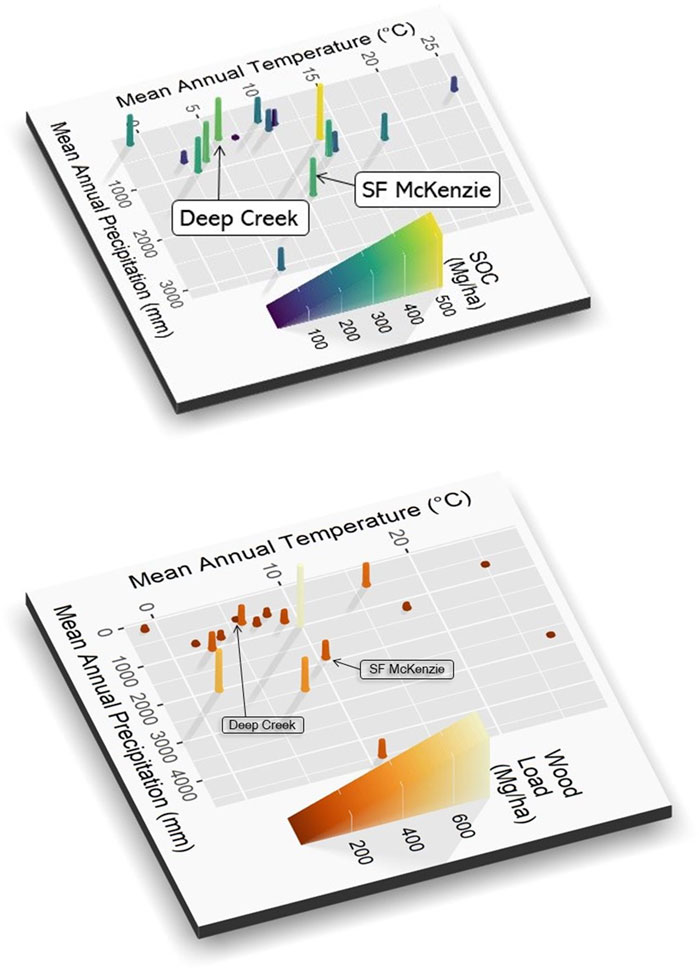
FIGURE 3. Magnitude of organic carbon stock in floodplain soil (top) and floodplain wood load (bottom). These distributions are based on limited data and thus likely to change as more field sites are characterized. Labels indicate the location of the reference-site values for the case studies in this paper. Data used to generate these plots are in supplementary tables.
Existing quantifications of floodplain carbon stocks indicate enormous variation based on site-specific geology, climate, biome, flow regime, and channel and valley morphology, even within a single river network (Sutfin et al., 2016; Scott and Wohl, 2018a; Sutfin and Wohl, 2019). In the context of river restoration, regional reference sites based on the least human-altered portions of a river can help to constrain estimates of the potential for enhanced carbon sequestration at a restoration site. This is the approach that we use in the case studies presented in this paper. The quantification of carbon stock at reference sites can be based on field sampling or existing databases.
Field sampling for soil organic carbon requires 1) collecting sediment samples that represent the lateral heterogeneity of the site and variation with depth, and analyzing the percent of organic carbon and 2) measuring or estimating soil bulk density (Structx, 2021). These measurements can then be used to calculate Mg C/ha of floodplain, commonly expressed as a volume based on 1 m depth of soil. The 1 m depth is based on the well-documented pattern of declines in organic carbon concentration with increasing depth in soils (e.g., Malone et al., 2009). Previous studies indicate that this depth decline is also common in floodplains (e.g., Lininger et al., 2018), in which most organic carbon comes from autochthonous sources such as litterfall from floodplain vegetation in portions of the floodplain that are farther from the active channel and have limited hydrologic connectivity (Hupp et al., 2019). In the floodplains of smaller rivers or on floodplains that have or are undergoing rapid deposition as a result of human activities such as altering land cover in the drainage basin, carbon-rich soil layers may be buried more deeply (Ricker and Lockaby, 2015; D’Elia et al., 2017). The potential for buried carbon-rich layers to exist in a floodplain should be assessed based on knowledge of site geomorphic and human history, and sampling depth for soil carbon should be modified if needed.
Previous work suggests that stratifying the floodplain into geomorphic units and then obtaining a minimum number of samples to adequately characterize the carbon concentration with depth in each unit (e.g., Lininger et al., 2018) can provide a basis for extrapolating carbon stock across an entire reach based on the spatial extent of each geomorphic unit (Sutfin and Wohl, 2017; Lininger et al., 2019; Scott and Wohl, 2020).
Field sampling for carbon in the form of large wood requires measuring the volume of individual wood pieces or logjams. This can be done for all pieces in the entire reach if the surface area is relatively small or wood loads are low. For larger areas or sites with substantial wood loads, stratified random sampling using floodplain transects (Wohl et al., 2018b) stratified on the basis of valley geometry, floodplain forest age or extent, or other relevant variables can provide sufficient data for extrapolation to the entire floodplain.
Existing databases can also be used to estimate floodplain carbon stock semi-quantitatively. Soil maps, including those available for much of the United States through the SSURGO online database (Soil Survey Staff, 2021), provide representative values for soil texture with depth, total organic carbon, bulk density, and soil thickness for a soil series, and these parameters can be used to calculate soil organic carbon stock (e.g., Wohl and Pfeiffer, 2018). Existing databases for large wood are largely nonexistent, although regional case studies that quantify expected wood loads based on position in the river network or channel width are available for a few locations, such as Washington State in the United States (Fox and Bolton, 2007). Remote imagery obtained via a drone, lidar data (Atha and Dietrich, 2016), Google Earth (Atha, 2014), aerial photographs, or other satellite imagery can be used to obtain at least a minimum count of large wood visible from the air. Ideally, ground measurements of piece diameters can be used to calibrate estimates of wood volume based on piece length, which is most easily measured in remote imagery. Where floodplain wood load is strongly influenced by fluvial transport, rather than just tree fall from the floodplain forest, the spatial distribution of large wood can be highly non-uniform (e.g., Piégay, 1993; Piégay and Gurnell, 1997; Wohl et al., 2018b), suggesting the need to obtain sufficiently large samples to include spatial heterogeneity of wood distribution.
Case Studies
Deep Creek
Deep Creek is located in Ochoco National Forest in the Ochoco Mountain range in central Oregon, United States (elevation 1,325 m) (Figure 4). The creek drains 224 km2 and flows southwest into the North Fork Crooked River immediately downstream of the study area. The catchment is dominated by old-growth ponderosa pine (Pinus ponderosa) forest. Average temperature and precipitation in Deep Creek are 5.7°C and 468 mm, respectively. Deep Creek represents a case study for potential carbon sequestration in a river corridor with mixed forest and beaver-modified willow carrs. Past grazing activities and artificial berms caused Deep Creek to incise up to 1 m below the floodplain and led to water table lowering and channel-floodplain disconnectivity. In an effort to restore habitat for Columbia River redband trout (Oncorhynchus mykiss gairdneri), Columbia spotted frog (Rana luteiventris), and other aquatic species, the U.S. Forest Service regraded the floodplain, added large wood, and reconnected relict secondary channels in 2018 and 2019 (Paul Powers, USDA Forest Service, June 2020, pers. comm.). The regional reference site used as a measure of carbon sequestration potential for Deep Creek is Gray’s Creek, located approximately 15 km from Deep Creek and also in the larger North Fork Crooked River watershed. The Gray’s Creek floodplain houses an active beaver complex that has been established for approximately 20 years (Jason Gritzner and Paul Powers, USDA Forest Service, June 2020, pers. comm.). We chose a degraded analog for Deep Creek just upstream of the restoration site, where the channel remains relatively incised with rows of alder (Alnus spp.) topping elevated surfaces surrounding the single active channel (Figure 5). Additional descriptive data for Deep Creek are in Supplementary Table S1.
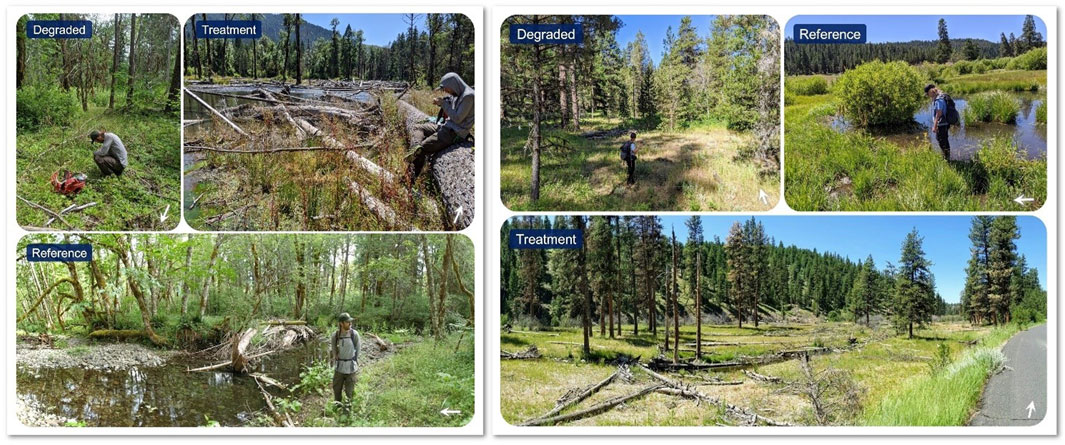
FIGURE 5. Field photos of the South Fork McKenzie River in Willamette National Forest (left panel) and Deep Creek in Ochoco National Forest (right panel), Oregon, United States. Each photo is labeled with its assigned class as degraded, treatment, and design reference (“Reference”), and arrows in the bottom right of each photo indicate flow direction. The person in the left panel is 1.86 m tall and the person in the right panel is 1.58 m tall.
South Fork McKenzie River
The South Fork McKenzie River (drainage area 560 km2) is located near Rainbow, Oregon in the Willamette National Forest in the western Cascade Mountains (elevation 340 m) (Figure 4). With average annual temperature and precipitation of 10.6°C and 1882 mm, respectively, this site represents a case study for carbon sequestration potential in a densely forested river corridor and a Mediterranean climate with significant hydrologic influence from an upstream dam. Common forest species here include western hemlock (Tsuga heterophylla), Douglas-fir (Pseudotsuga menziesii), and western redcedar (Thuja plicata), with red alder (Alnus rubra) and cottonwood (Populus trichocarpa) adjacent to streams. Following construction of Cougar Dam in 1963, the channel incised to a single thread with primarily boulder substrate. Restoration was initiated in 2018 and involved dense placement of large wood across the entire floodplain, lowering of high alluvial surfaces, and filling of the incised channel to reconnect the channel and floodplain and improve habitat conditions for Chinook salmon (Oncorhynchus tshawytscha), bull trout (Salvelinus confluentus), Pacific lamprey (Entosphenus tridentatus), and other species. Horse Creek, approximately 10 km away, provides the reference condition for this site. Horse Creek has an anastomosing planform with one primary and several secondary channels, each with high wood load, frequent channel avulsions, and abundant forested wetlands in the river corridor. We selected a reach of the South Fork McKenzie upstream of the restoration activities for a degraded site. This degraded site is planned to be included in future restoration actions. The degraded reach is single threaded with a pine-dominated forested floodplain and history of channel manipulation and levee construction (Figure 5). Additional descriptive data for the South Fork McKenzie are in Supplementary Table S1.
Methods
Our sampling design to estimate carbon sequestration potential includes three classes per study area. The three classes, characterized by floodplain condition and inferred history, are referred to as degraded, treatment, and design reference. We assume that degraded sites represent typical pre-restoration conditions where human activities have moved the site beyond the natural range of variability present prior to European settlement of the region (Rathburn et al., 2011; Wohl, 2011). Treatment sites represent conditions of recently implemented stream restoration treatments. Design reference sites represent relatively natural environments with minimal human alteration and carbon stocks that reflect the natural range of variability for a particular type of river corridor. Choice of degraded and design reference sites is subjective. The Deep Creek and South Fork McKenzie River sites are both on U.S. national forest lands and we worked with local Forest Service hydrologists and fish biologists to identify degraded and design reference sites. We also used our knowledge of analogous sites from other portions of the western US.
A beaver-modified willow carr in which beaver are still present is characterized by numerous dams, ponds, and beaver canals in various stages of activity (i.e., active, or abandoned for progressively longer times) (Laurel and Wohl, 2019). The channel and floodplain are hydrologically connected. Abundant surface water storage in ponds (Hood and Bayley, 2008) and a high floodplain water table (Westbrook et al., 2006) create reducing conditions throughout much of the floodplain, even in regions with a dry climate. Spatially dense woody vegetation attenuates downstream fluxes of water and sediment, and beaver dams promote hyporheic exchange flows (Lautz et al., 2006). This configuration, known as a beaver-meadow complex (Polvi and Wohl, 2012), represents design reference conditions for Deep Creek treatment site. In contrast, when beaver abandon a site and their dams fall into disrepair, river flow is more likely to concentrate into a single channel. Consequently, the channel incises and helps to lower the floodplain water table, hydrologically disconnecting the channel and floodplain and creating an alternative state known as an elk grassland (Wolf et al., 2007). This configuration represents degraded conditions for the Deep Creek treatment site.
A densely forested river corridor, such as that in the South Fork McKenzie River, has abundant recruitment sources for large wood. As described for other rivers in the U.S. Pacific Northwest, the continuing recruitment and storage of large wood facilitates formation of logjams that influence the distribution of hydraulics and sediment transport, commonly leading to a multithread channel planform and spatially heterogeneous floodplain geomorphology and floodplain forest (Fetherston et al., 1995; Collins et al., 2012). This represents design reference conditions for the South Fork McKenzie treatment site. When large wood is actively removed from the river corridor and/or deforestation removes wood recruitment sources, the loss of logjams in the channel and floodplain can lead to formation of a single channel flowing through a less diverse floodplain (Collins et al., 2002). This configuration represents degraded conditions for the South Fork McKenzie treatment site.
Soil Carbon
We anticipated within-reach heterogeneity in soil carbon content based on differences in hydrologic connectivity and soil moisture. Consequently, we differentiated wet and dry floodplain patches based on field conditions when sampling soil. We assigned these moisture conditions based on ground moisture at the time of field work and the type of vegetation present. For example, wetland vegetation such as sedges, rushes, or cattails characterize wet areas and more xeric vegetation such as reed canary grass (Phalaris arundinacea), cheatgrass (Bromus tectorum), or ponderosa pine (Pinus ponderosa) characterize dry areas. We randomized the exact sample location within a wet or dry area when choosing specific sample points.
We sampled soil carbon with a tubular soil corer of 3 cm diameter that collects 30-cm lengths of sample in each increment. At each soil sampling point, we sampled up to 1 m depth or refusal by cobbles in shallower soil. Previous research suggests that sampling bias and variance of organic carbon content cease to decrease after 11 samples within a floodplain along smaller rivers (Sutfin and Wohl, 2017) and within individual floodplain geomorphic units on larger rivers (Lininger et al., 2018). The case studies presented here involve smaller rivers, so we targeted 11 samples per moisture category in each floodplain class. This equates to 11 samples each in wet and dry soils per class per study area, for a total of 22 samples per class and 66 samples per study area. However, floodplains with degraded conditions were commonly hydrologically disconnected and dry. Consequently, fewer wet soil samples were collected from degraded floodplains. Additionally, some samples were lost during lab analysis. In total, we conducted statistical analyses on 59 soil samples from Deep Creek (12 dry and two wet from the degraded class, 11 dry and 13 wet from the treatment class, and 10 dry and 11 wet from the design reference class), and 53 samples from the South Fork McKenzie (11 dry from degraded, 11 dry and 10 wet from treatment, and 10 dry and 11 wet from design reference).
For final floodplain-scale estimation of soil carbon stocks, we weighted the estimated soil OC stocks for each moisture category by the approximate area of wet or dry soil in each floodplain. We determined wet versus dry conditions using National Agricultural Imagery Program (NAIP) aerial imagery from July 2020, the same month field samples were collected. Horse Creek, the design reference site for the South Fork McKenzie River, has too much forest vegetation to confidently estimate wet versus dry soil, so we used topography to estimate areas of wet and dry soil instead. Using a digital elevation model made from LiDAR data collected in 2016 and downloaded from Oregon Department of Geology and Mineral Resources, we assumed that soil below the average relative elevation above a channel surface (detrended by valley gradient) in the sampling area could be categorized as potentially wet. We validated this assumption with our sample locations of wet and dry soil. Through these analyses, we found the existing sample sizes to appropriately reflect area proportions of wet and dry soil, with roughly equal proportions in treatment and design reference floodplains, and little to no wet soil in degraded floodplains.
Soil samples were stored in a freezer and shipped to a commercial laboratory for analysis of organic carbon, total carbon, and texture with a hydrometer. Grain size percentages of silt, sand, and clay were used to categorize soil samples into soil types. Bulk density estimates were assigned based on soil type after evaluation of values according to soil type in comparison to two pedotransfer functions (Leonaviciute, 2000; Ruehlmann and Korschens, 2009), three regression analyses of data from Chaudhari et al. (2013), and a constant density value. Estimates of bulk density were the median result of the seven methods evaluated and offered the most reasonable estimates of carbon stocks. The following formula was used to calculate carbon stocks from percent organic carbon:
Large Wood Carbon
Wood load was estimated via transects following the protocol of Van Wagner, 1968) in the degraded and design reference reaches. Transects were evenly spaced where possible and extended either across the entire floodplain or across one half of the floodplain, depending on accessibility. We measured the diameter of each piece of large wood encountered along transects, and input diameters and lengths into the following equation to estimate volume per area of wood (Van Wagner, 1968):
Where V = volume of wood per area, d = diameter of a piece of large wood >10 cm diameter and >1 m length, L = transect length, and all metrics are in the same units of measurement. Wood volume in treatment floodplains was estimated with timber sale data from managing parties who completed the restoration projects, and from monitoring data in the South Fork McKenzie River (Hinshaw et al., in review). Monitoring data are from 2020 and include large wood volume measured in 23 plots randomly located in the South Fork McKenzie River treated floodplain. We calculated carbon stocks of large wood using average wood densities of the dominant tree species in each study area and the assumption that wood contains 50% organic carbon:
For the South Fork McKenzie River, we averaged densities for the five dominant tree species mentioned above. Ponderosa pine density was used for Deep Creek degraded and treatment sites, and willow density was used for the Deep Creek design reference floodplain.
Statistical Analyses
We used a type 3 ANOVA to determine whether there are differences between classes for each study site using a linear model of soil organic carbon stock predicted by class (degraded, treatment, and design reference). R version 4.0.3 was used to conduct the analysis with the car package (Fox and Weisberg, 2019; R Core Team, 2020). Separate models were fitted for the two study sites and two models were fit for Deep Creek: one model with class as the sole predictor, and one that included moisture. We used the emmeans package to conduct Tukey-adjusted pairwise comparisons between classes groups at the 95% confidence level (Lenth, 2020). Moisture was not included in the model for the South Fork McKenzie River because there were no wet samples in the degraded reach. Depth and texture, other potential predictors of carbon stocks, were not included because we assume they are adequately integrated into the calculation of soil organic carbon stocks. Moisture was included in a model for Deep Creek, but the sample size of n = 2 wet samples in the degraded reach precludes reliable interpretation of comparisons of wet samples from the degraded class to other classes.
Results
We first tested whether there were significant differences in percent organic carbon in relation to moisture category (wet vs dry) at each of the six sites. We found significant differences at only two of the sites: percent organic carbon is significantly higher in 1) wet soils of the design reference site for South Fork McKenzie (p value 0.0275) and 2) dry soils for the treatment site at Deep Creek (p value 0.036).
We then compared the six sites based on organic carbon stock. There were no significant differences between classes for Deep Creek when class was the sole predictor of soil organic carbon stock (Figure 6). When moisture and class were used to predict soil OC stocks, we found evidence of an interaction between class and moisture (p = 0.04697). Among dry samples, there was a notable, but not statistically significant, difference between degraded and treated floodplain carbon stocks (p = 0.0782), and a significant difference between treated and design reference floodplain soil OC stocks (p = 0.0150). The estimated difference between treated and degraded dry floodplain OC stocks is 268 Mg/ha, with higher estimated carbon stocks in treated floodplains. Our data in Deep Creek showed higher estimated OC stocks in dry treated than dry design reference conditions, with an estimated difference of 367 Mg/ha.
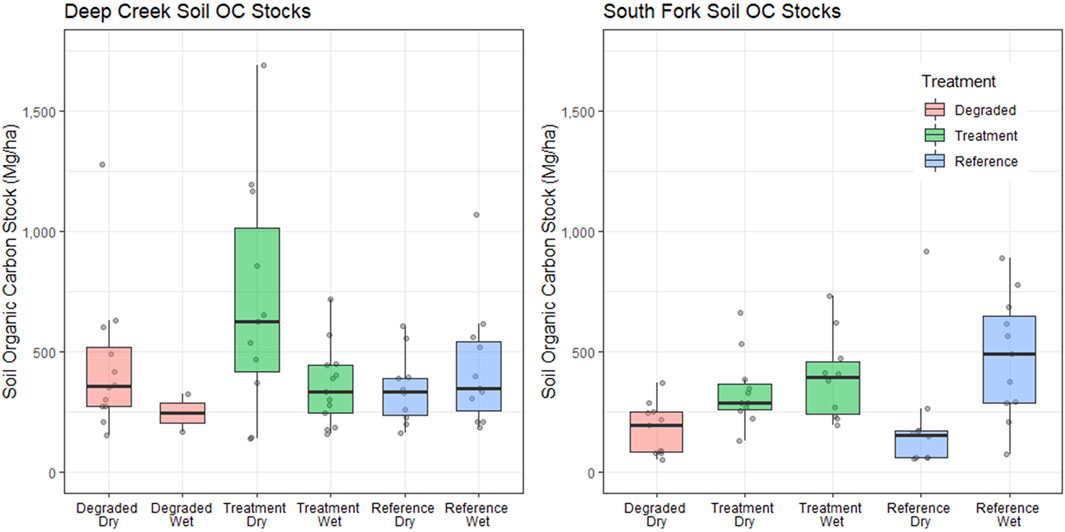
FIGURE 6. Box and whisker plots of soil organic carbon stock for wet and dry portions of each of the six classes. Line within each box indicates the median value, box ends are the upper and lower quartile, and whiskers are the 10th and 90th percentiles. Data points summarized by each box are also shown. Differences in South Fork McKenzie River organic carbon stocks were analyzed by class only due to a lack of wet samples from the degraded floodplain.
At the South Fork McKenzie site, there were no significant differences between class at the 95% confidence level, but there was a nonsignificant but notable estimated difference of 187 Mg/ha in mean values for OC stocks between the treated and degraded floodplains, with higher predicted soil OC stock in the treated floodplain (p = 0.0572). There was also a notable but nonsignificant difference between the degraded and design reference floodplains for wet and dry combined (p = 0.0886), with estimated mean OC stocks in the design reference site predicted to be higher than the degraded site by 171 Mg/ha.
Estimates of large wood load and large wood carbon stocks varied depending on the method used and are presented in Table 3. Total estimated OC stocks, estimated by the sums of the simple mean soil OC stock of each class and large wood OC stocks, were greatest for the treated floodplain reaches in both study areas, and are also presented in Table 3. Basic organic carbon data are in Supplementary Table S3.
Discussion and Conclusion
Our conceptual framework for quantifying carbon sequestration potential in river restoration involves the use of regional design reference and degraded floodplains for comparison to treated floodplains. The difference in measured carbon stocks between a degraded and design reference floodplain conceptually represents potential minimum differences in carbon storage that could be achieved through restoration actions, and measured carbon stocks in treatment areas indicate further potential for carbon storage. We illustrated application of the conceptual framework using two study areas, for both of which our results suggest higher organic carbon stocks in the treated areas than in their respective degraded and design reference sites. We also estimated higher carbon stock in the degraded reach of the Deep Creek study area than in the design reference site.
The importance of large wood as a contributor to total carbon stock varies in relation to valley morphology and the presence of wood-trapping sites (Figure 1B), and concentration of large wood in limited areas (e.g., beaver dams or very large logjams) can make it difficult to accurately estimate total wood load. We estimated the smallest carbon stocks in the form of large wood at the Gray’s Creek site, for example, which is an active beaver meadow complex and design reference floodplain for Deep Creek. We expected a low wood load in the anastomosing grassed wetland of Gray’s Creek, but the transect method of sampling large wood did not cross any beaver dams at this site, which may have caused us to underestimate wood load in the reach. Disproportionately high concentrations of wood are also located in large logjams at Horse Creek, the design reference floodplain for South Fork McKenzie. Because only one of five transects at Horse Creek crossed a logjam, the transect sampling method may have caused us to underestimate wood load at Horse Creek. In addition, high surface soil moisture and associated wood decay in Horse Creek likely kept us from measuring some buried, partially buried, decayed, and moss-covered pieces of wood from our transect surveys. Finally, the use of timber sale and project design data to estimate wood load in the treated Deep Creek floodplain likely led to an underestimate. Project design data provide an easy way to estimate wood loads in restoration reaches, but do not include pieces recruited since project construction.
Floodplain manipulation and other land use histories also influence large wood loads. The highest wood carbon stocks of our case studies occurred in the treated area of the South Fork McKenzie River. As part of the project implementation, large wood was placed in a lattice across the floodplain to create hydraulic roughness and encourage deposition. The use of large wood in restoration practices, such as the Stage 0 project at the South Fork McKenzie, increases the large wood carbon stocks in treated floodplains. At the degraded reach of the South Fork McKenzie, cut logs also increased measured wood loads in the study area. We observed that many of the logs measured along transects in the degraded site were cut and accompanied by burn piles, presumably due to forest management practices such as hazardous fuel control. These cut logs increased our measured wood loads, but have separate, more static, geomorphic roles than large wood that more regularly interacts with water and sediment in dynamic floodplains such as Horse Creek.
We interpret the range of carbon stocks measured at all three sites in each case study as reflecting the potential range of floodplain carbon storage for the geographic and geomorphic setting of each case study. As described in the conceptual model, numerous factors interact to govern floodplain carbon accumulation rates through time and carbon stock at any point in time. This makes it both difficult to precisely predict floodplain carbon storage and difficult to use degraded and design reference reaches to demonstrate the effects of river restoration on carbon storage over short timespans of a few years. However, it is encouraging that the values of wood load and soil organic carbon at each of the design reference sites seem reasonable relative to preliminary carbon storage plots and summary tables developed from published values of floodplain large wood loads and soil carbon (Figure 3 and Supplementary Material). This suggests that the type of data reported in Table 1 and Figure 3 may eventually be useful in assessing potential floodplain carbon stocks following restoration, when the available data are expanded to include a larger number of sample sites across a wider range of climate and geomorphic conditions.
The optimal timeframes for monitoring organic carbon stock after restoration are not presently known but would presumably be at least several years to decades after restoration activities cease (e.g., Schiefer et al., 2018). This would allow newly (re)saturated floodplain soils time to accumulate organic matter. It would also allow time for large wood introduced to the site during restoration or trapped by the enhanced spatial heterogeneity of the restored site to accumulate in natural trapping sites such as bars, islands, or forested floodplain margins. Depending on the size and spatial heterogeneity of the restored floodplain, we suggest that floodplain soil-carbon sampling be based on a stratified random design that, at a minimum, differentiates wet and dry floodplain surfaces and for larger rivers differentiates geomorphic patches (e.g., Lininger et al., 2018). Floodplain large-wood-carbon sampling using survey transects can also be based on a stratified random design where wood is known to be concentrated in large accumulations, including beaver dams.
Candidate sites for restoration are commonly chosen based on known or inferred historic conditions prior to excessive human disturbance and degradation. More specifically, in process-based and Stage 0 stream restoration, sites chosen for restoration activities are commonly wide, depositional valleys. This type of valley can be inferred, based on historic aerial imagery, local knowledge, relict hydric soils or floodplain landforms, to have historically possessed highly complex planforms with shallow groundwater tables, high degrees of lateral hydrologic connectivity, high wood loads, and floodplain wetlands. Abandoned beaver meadows are capable of retaining high carbon stocks for decades after abandonment (Laurel and Wohl, 2019). High measured soil carbon stocks of treatment floodplains in our case studies may primarily reflect past conditions, rather than the effects of recent restoration. In particular, the highest sampled soil carbon stocks from the Deep Creek treated floodplain (Figure 5) are from topographically high floodplain patches (referred to as “leave islands” during restoration) with old-growth ponderosa pines, suggesting the potential for prolonged organic matter input and accumulation.
There are many benefits associated with quantitatively estimating carbon stocks in degraded, treated, and design reference floodplains. We propose this conceptual model and study design as an example for practitioners, managers, scientists, investors, and other stakeholders to track changes of floodplains over time, collect baseline data in pre-treatment degraded areas, and build databases of design reference conditions that can be available to future stream restoration designers. At a broader scale, rivers play a role in the global carbon cycle, but the definition and quantification of carbon sequestration potential in rivers remains largely unknown. We suggest that the type of quantitative estimations summarized here can be used to enhance the use of river restoration as a tool for carbon sequestration.
Data Availability Statement
The original contributions presented in the study are included in the article/Supplementary Material, further inquiries can be directed to the corresponding author.
Ethics Statement
Written informed consent was obtained from the individual(s) for the publication of any potentially identifiable images or data included in this article.
Author Contributions
SH collected and analyzed the field data for the case studies. EW collected the background summary data for Tables 1, 2. Both authors collaborated on organization and writing of the text.
Funding
Funding for this work comes from a Geological Society of America student research grant to SH and from the Carbon Project of Patagonia’s RIVERS team.
Conflict of Interest
The authors declare that the research was conducted in the absence of any commercial or financial relationships that could be construed as a potential conflict of interest.
Publisher’s Note
All claims expressed in this article are solely those of the authors and do not necessarily represent those of their affiliated organizations, or those of the publisher, the editors and the reviewers. Any product that may be evaluated in this article, or claim that may be made by its manufacturer, is not guaranteed or endorsed by the publisher.
Acknowledgments
We thank Johan Hogervorst, Paul Powers, Paul Burns, Kate Meyer, Lisa Renan, and Lisa Kurian of the USDA Forest Service for help with site selection. We would like to acknowledge Juli Scamardo, Taylor Kenyon, and Anna Marshall for help collecting samples in the field.
Supplementary Material
The Supplementary Material for this article can be found online at: https://www.frontiersin.org/articles/10.3389/feart.2021.708895/full#supplementary-material
References
Aberle, J., and Järvelä, J. (2013). Flow Resistance of Emergent Rigid and Flexible Floodplain Vegetation. J. Hydraulic Res. 51, 33–45. doi:10.1080/00221686.2012.754795
Adame, M. F., Reef, R., Wong, V. N. L., Balcombe, S. R., Turschwell, M. P., Kavehei, E., et al. (2020). Carbon and Nitrogen Sequestration of Melaleuca Floodplain Wetlands in Tropical Australia. Ecosystems 23, 454–466. doi:10.1007/s10021-019-00414-5
Appling, A. P. (2012). Connectivity Drives Function: Carbon and Nitrogen Dynamics in a Floodplain-Aquifer ecosystemPhD Dissertation. Durham, North Carolina: Duke University.
Assine, M. L., Merino, E. R., Pupim, F. N., Warren, L. V., Guerreiro, R. L., and McGlue, M. M. (2015). “Geology and Geomorphology of the Pantanal Basin,” in IDynamics of the Pantanal Wetland in South America. Editors I. Bergier, and M.L. Assine (Cham, Switzerland: Springer), 23–50. doi:10.1007/698_2015_349
Atha, J. B., and Dietrich, J. T. (2016). Detecting Fluvial wood in Forested Watersheds Using LiDAR Data: a Methodological Assessment. River Res. Applic. 32, 1587–1596. doi:10.1002/rra.2989
Atha, J. B. (2014). Identification of Fluvial wood Using Google Earth. River Res. Applic. 30, 857–864. doi:10.1002/rra.2683
Benda, L. E., and Sias, J. C. (2003). A Quantitative Framework for Evaluating the Mass Balance of In-Stream Organic Debris. For. Ecol. Manage. 172, 1–16. doi:10.1016/s0378-1127(01)00576-x
Blattmann, T. M., Letsch, D., and Eglinton, T. I. (2018). On the geological and scientific legacy of petrogenic organic carbon. Am. J. Sci. 318, 861–881.
Boivin, M., Buffin-Bélanger, T., and Piégay, H. (2015). The Raft of the Saint-Jean River, Gaspé (Québec, Canada): A Dynamic Feature Trapping Most of the wood Transported from the Catchment. Geomorphology 231, 270–280. doi:10.1016/j.geomorph.2014.12.015
Briggs, M. A., and Hare, D. K. (2018). Explicit Consideration of Preferential Groundwater Discharges as Surface Water Ecosystem Control Points. Hydrological Process., 1–6. doi:10.1002/hyp.13178
Brinson, M. M., Bradshaw, H. D., Holmes, R. N., and Elkins, J. B. (1980). Litterfall, Stemflow, and Throughfall Nutrient Fluxes in an Alluvial Swamp forest. Ecology 61, 827–835. doi:10.2307/1936753
Cabezas, A., Comín, F. A., and Walling, D. E. (2009). Changing Patterns of Organic Carbon and Nitrogen Accretion on the Middle Ebro Floodplain (NE Spain). Ecol. Eng. 35, 1547–1558. doi:10.1016/j.ecoleng.2009.07.006
Carnell, P. E., Windecker, S. M., Brenker, M., Baldock, J., Masque, P., Brunt, K., et al. (2018). Carbon Stocks, Sequestration, and Emissions of Wetlands in South Eastern Australia. Glob. Change Biol. 24, 4173–4184. doi:10.1111/gcb.14319
Chaudhari, P. R., Ahire, D. V., Ahire, V. D., Chkravarty, M., and Maity, S. (2013). Soil bulk density as related to soil texture, organic matter content and available total nutrients of Coimbatore soil. Int. J. Sci. Res. Publ. 3, 1–8.
Christensen, C. W., Hayashi, M., and Bentley, L. R. (2020). Hydrogeological Characterization of an alpine Aquifer System in the Canadian Rocky Mountains. Hydrogeol J. 28, 1871–1890. doi:10.1007/s10040-020-02153-7
Cierjacks, A., Kleinschmit, B., Babinsky, M., Kleinschroth, F., Markert, A., Menzel, M., et al. (2010). Carbon Stocks of Soil and Vegetation on Danubian Floodplains. Z. Pflanzenernähr. Bodenk. 173, 644–653. doi:10.1002/jpln.200900209
Clark, D. B., Clark, D. A., Brown, S., Oberbauer, S. F., and Veldkamp, E. (2002). Stocks and Flows of Coarse Woody Debris across a Tropical Rain forest Nutrient and Topography Gradient. For. Ecol. Manage. 164, 237–248. doi:10.1016/s0378-1127(01)00597-7
Cluer, B., and Thorne, C. (2014). A Stream Evolution Model Integrating Habitat and Ecosystem Benefits. River Res. Applic. 30, 135–154. doi:10.1002/rra.2631
Collins, B. D., Montgomery, D. R., Fetherston, K. L., and Abbe, T. B. (2012). The Floodplain Large-wood Cycle Hypothesis: a Mechanism for the Physical and Biotic Structuring of Temperate Forested Alluvial Valleys in the North Pacific Coastal Ecoregion. Geomorphology 139-140, 460–470. doi:10.1016/j.geomorph.2011.11.011
Collins, B. D., Montgomery, D. R., and Haas, A. D. (2002). Historical Changes in the Distribution and Functions of Large wood in Puget Lowland Rivers. Can. J. Fish. Aquat. Sci. 59, 66–76. doi:10.1139/f01-199
Comiti, F., Andreoli, A., Mao, L., and Lenzi, M. A. (2008). Wood Storage in Three Mountain Streams of the Southern Andes and its Hydro-Morphological Effects. Earth Surf. Process. Landforms 33, 244–262. doi:10.1002/esp.1541
Craft, C. B., and Casey, W. P. (2000). Sediment and Nutrient Accumulation in Floodplain and Depressional Freshwater Wetlands of Georgia, USA. Wetlands 20, 323–332. doi:10.1672/0277-5212(2000)020[0323:sanaif]2.0.co;2
Craft, C., Vymazal, J., and Kröpfelová, L. (2018). Carbon Sequestration and Nutrient Accumulation in Floodplain and Depressional Wetlands. Ecol. Eng. 114, 137–145. doi:10.1016/j.ecoleng.2017.06.034
D’Elia, A. H., Liles, G. C., Viers, J. H., and Smart, D. R. (2017). Deep Carbon Storage Potential of Buried Floodplain Soils. Sci. Rep. 7, 8181. doi:10.1038/s41598-017-06494-4
DesRoches, A., Danielescu, S., and Butler, K. (2014). Structural Controls on Groundwater Flow in a Fractured Bedrock Aquifer Underlying an Agricultural Region of Northwestern New Brunswick, Canada. Hydrogeol J. 22, 1067–1086. doi:10.1007/s10040-014-1134-0
Dixon, S. J., Sear, D. A., Odoni, N. A., Sykes, T., and Lane, S. N. (2016). The Effects of River Restoration on Catchment Scale Flood Risk and Flood Hydrology. Earth Surf. Process. Landforms 41, 997–1008. doi:10.1002/esp.3919
Doughty, M., Sawyer, A. H., Wohl, E., and Singha, K. (2020). Mapping Increases in Hyporheic Exchange from Channel-Spanning Logjams. J. Hydrol. 587, 124931. doi:10.1016/j.jhydrol.2020.124931
Ehlen, J., and Wohl, E. (2002). Joints and Landform Evolution in Bedrock Canyons. Trans. Jpn. Geomorphol. Union 23, 237–255.
Everitt, B. L. (1968). Use of the cottonwood in an Investigation of the Recent History of a Flood plain. Am. J. Sci. 266, 417–439. doi:10.2475/ajs.266.6.417
Falloon, P., Jones, C. D., Ades, M., and Paul, K. (2011). Direct Soil Moisture Controls of Future Global Soil Carbon Changes: An Important Source of Uncertainty. Glob. Biogeochem. Cycles 25, 1–14. doi:10.1029/2010gb003938
Fetherston, K. L., Naiman, R. J., and Bilby, R. E. (1995). Large Woody Debris, Physical Process, and Riparian forest Development in Montane River Networks of the Pacific Northwest. Geomorphology 13, 133–144. doi:10.1016/0169-555x(95)00033-2
Fox, J., and Weisberg, S. (2019). An {R} Companion to Applied Regression. Third Edition. Thousand Oaks CA: Sage. Available at: https://socialsciences.mcmaster.ca/jfox/Books/Companion/.
Fox, M., and Bolton, S. (2007). A Regional and Geomorphic Reference for Quantities and Volumes of Instream Wood in Unmanaged Forested Basins of Washington State. North Am. J. Fish. Manage. 27, 342–359. doi:10.1577/m05-024.1
Friedlingstein, P., O’Sullivan, M., Jones, M. W., Andrew, R. M., Hauck, J., Olsen, A., et al. (2020). Global Carbon Budget 2020. Earth Syst. Sci. Data 12, 3269–3340.
Gifford, L. (2020). "You Can't Value what You Can't Measure": a Critical Look at forest Carbon Accounting. Climatic Change 161, 291–306. doi:10.1007/s10584-020-02653-1
Gurnell, A. M., Petts, G. E., Harris, N., Ward, J. V., Tockner, K., Edwards, P. J., et al. (2000). Large wood Retention in River Channels: the Case of the Fiume Tagliamento, Italy. Earth Surf. Process. Landforms 25, 255–275. doi:10.1002/(sici)1096-9837(200003)25:3<255::aid-esp56>3.0.co;2-h
Guyette, R. P., Dey, D. C., and Stambaugh, M. C. (2008). The Temporal Distribution and Carbon Storage of Large Oak wood in Streams and Floodplain Deposits. Ecosystems 11, 643–653. doi:10.1007/s10021-008-9149-9
Hall, J. E., Holzer, D. M., and Beechie, T. J. (2007). Predicting River Floodplain and Lateral Channel Migration for salmon Habitat Conservation. J. Am. Water Resour. Assoc. 43, 786–797. doi:10.1111/j.1752-1688.2007.00063.x
Hanberrry, B. B., Kabrick, J. M., and He, H. S. (2015). Potential Tree and Soil Carbon Storage in a Major Historical Floodplain forest with Disrupted Ecological Function. Perspect. Plant Ecol. Evol. Syst. 17, 17–23. doi:10.1016/j.ppees.2014.12.002
Hardie, M. A., Doyle, R. B., Cotching, W. E., and Lisson, S. (2012). Subsurface Lateral Flow in Texture-Contrast (Duplex) Soils and Catchments with Shallow Bedrock. Appl. Environ. Soil Sci., 861358. doi:10.1155/2012/861358
Hoffmann, T., Erkens, G., Cohen, K. M., Houben, P., Seidel, J., and Dikau, R. (2007). Holocene Floodplain Sediment Storage and Hillslope Erosion within the Rhine Catchment. The Holocene 17, 105–118. doi:10.1177/0959683607073287
Hoffmann, T., Glatzel, S., and Dikau, R. (2009). A Carbon Storage Perspective on Alluvial Sediment Storage in the Rhine Catchment. Geomorphology 108, 127–137. doi:10.1016/j.geomorph.2007.11.015
Hood, G. A., and Bayley, S. E. (2008). Beaver (Castor canadensis) Mitigate the Effects of Climate on the Area of Open Water in Boreal Wetlands in Western Canada. Biol. Conservation 141, 556–567. doi:10.1016/j.biocon.2007.12.003
Hupp, C. R., Demas, C. R., Kroes, D. E., Day, R. H., and Doyle, T. W. (2008). Recent Sedimentation Patterns within the central Atchafalaya Basin, Louisiana. Wetlands 28, 125–140. doi:10.1672/06-132.1
Hupp, C. R., Kroes, D. E., Noe, G. B., Schenk, E. R., and Day, R. H. (2019). Sediment Trapping and Carbon Sequestration in Floodplains of the Lower Atchafalaya Basin, LA: Allochthonous versus Autochthonous Carbon Sources. J. Geophys. Res. Biogeosci. 124, 663–677. doi:10.1029/2018jg004533
Jaramillo, V. c. J., Kauffman, J. B., Rentería-Rodríguez, L., Cummings, D. L., and Ellingson, L. J. (2003). Biomass, Carbon, and Nitrogen Pools in Mexican Tropical Dry forest Landscapes. Ecosystems 6, 609–629. doi:10.1007/s10021-002-0195-4
Jobbágy, E. G., and Jackson, R. B. (2000). The Vertical Distribution of Soil Organic Carbon and its Relation to Climate and Vegetation. Ecol. Appl. 10, 423–436. doi:10.1890/1051-0761(2000)010[0423:tvdoso]2.0.co;2
Kaiser, K., and Guggenberger, G. (2000). The Role of DOM Sorption to mineral Surfaces in the Preservation of Organic Matter in Soils. Org. Geochem. 31, 711–725. doi:10.1016/s0146-6380(00)00046-2
Koltzer, N., Scheck-Wenderoth, M., Cacace, M., Frick, M., and Bott, J. (2019). Regional Hydraulic Model of the Upper Rhine Graben. Adv. Geosci. 49, 197–206. doi:10.5194/adgeo-49-197-2019
Lal, R. (2008). Carbon Sequestration. Phil. Trans. R. Soc. B. 363, 815–830. doi:10.1098/rstb.2007.2185
Larsen, A., May, J.-H., Moss, P., and Hacker, J. (2016). Could Alluvial Knickpoint Retreat rather Than Fire Drive the Loss of Alluvial Wet Monsoon forest, Tropical Northern Australia. Earth Surf. Process. Landforms 41, 1583–1594. doi:10.1002/esp.3933
Larsen, L. G., and Harvey, J. W. (2010). How Vegetation and Sediment Transport Feedbacks Drive Landscape Change in the Everglades and Wetlands Worldwide. The Am. Naturalist 176, E66–E79. doi:10.1086/655215
Lassettre, N. S., Piégay, H., Dufour, S., and Rollet, A.-J. (2008). Decadal Changes in Distribution and Frequency of wood in a Free Meandering River, the Ain River, France. Earth Surf. Process. Landforms 33, 1098–1112. doi:10.1002/esp.1605
Laurel, D., and Wohl, E. (2019). The Persistence of beaver‐induced Geomorphic Heterogeneity and Organic Carbon Stock in River Corridors. Earth Surf. Process. Landforms 44, 342–353. doi:10.1002/esp.4486
Lautz, L. K., Siegel, D. I., and Bauer, R. L. (2006). Impact of Debris Dams on Hyporheic Interaction along a Semi-arid Stream. Hydrol. Process. 20, 183–196. doi:10.1002/hyp.5910
Lenth, R. V. (2020). Emmeans: Estimated Marginal Means, Aka Least-Squares Means. R package version 1.5.3. Available at: https://CRAN.R-project.org/package=emmeans Accessed: September 10, 2021.
Leonaviciute, N. (2000). Predicting soil bulk and particle densities by pedotransfer functions from existing soil data in Lithuania. Geografijos Metrastis 33, 317–330.
Lininger, K. B., Wohl, E., Sutfin, N. A., and Rose, J. R. (2018). Floodplain downed wood volumes: a comparison across three biomes. Earth Surf. Proc. Landforms 42, 1248–1261.
Lininger, K. B., Wohl, E., and Rose, J. R. (2018). Geomorphic Controls on Floodplain Soil Organic Carbon in the Yukon Flats, interior Alaska, from Reach to River basin Scales. Water Resour. Res. 54, 1934–1951. doi:10.1002/2017wr022042
Lininger, K. B., Wohl, E., Rose, J. R., and Leisz, S. J. (2019). Significant Floodplain Soil Organic Carbon Storage along a Large High‐Latitude River and its Tributaries. Geophys. Res. Lett. 46, 2121–2129. doi:10.1029/2018gl080996
Livers, B., and Wohl, E. (2015). An Evaluation of Stream Characteristics in Glacial versus Fluvial Process Domains in the Colorado Front Range. Geomorphology 231, 72–82. doi:10.1016/j.geomorph.2014.12.003
Malone, B. P., McBratney, A. B., Minasny, B., and Laslett, G. M. (2009). Mapping Continuous Depth Functions of Soil Carbon Storage and Available Water Capacity. Geoderma 154, 138–152. doi:10.1016/j.geoderma.2009.10.007
Martin, A. R., Doraisami, M., and Thomas, S. C. (2018). Global Patterns in wood Carbon Concentration across the World's Trees and Forests. Nat. Geosci 11, 915–920. doi:10.1038/s41561-018-0246-x
Micheli, E. R., and Kirchner, J. W. (2002). Effects of Wet Meadow Riparian Vegetation on Streambank Erosion. 2. Measurements of Vegetated Bank Strength and Consequences for Failure Mechanics. Earth Surf. Process. Landforms 27, 687–697. doi:10.1002/esp.340
Miller, J. R., Lord, M. L., Villarroel, L. F., Germanoski, D., and Chambers, J. C. (2012). Structural Organization of Process Zones in upland Watersheds of central Nevada and its Influence on basin Connectivity, Dynamics, and Wet Meadow Complexes. Geomorphology 139-140, 384–402. doi:10.1016/j.geomorph.2011.11.004
Mitsch, W. J., Dorage, C. L., and Wiemhoff, J. R. (1979). Ecosystem Dynamics and a Phosphorus Budget of an Alluvial cypress Swamp in Southern Illinois. Ecology 60, 1116–1124. doi:10.2307/1936959
M., M., Simo, J., and K., B. (2001). Correlation of Hydraulic Conductivity with Stratigraphy in a Fractured-Dolomite Aquifer, Northeastern Wisconsin, USA. Hydrogeology J. 9, 570–583. doi:10.1007/s10040-001-0165-5
Montgomery, D. R. (2002). Valley Formation by Fluvial and Glacial Erosion. Geol 30, 1047–1050. doi:10.1130/0091-7613(2002)030<1047:vfbfag>2.0.co;2
Moreira-Turcq, P., Jouanneau, J. M., Turcq, B., Seyler, P., Weber, O., and Guyot, J. L. (2004). Carbon sedimentation at Lago Grande de Curuai, a floodplain lake in the low Amazon region: insights into sedimentation rates. Palaeogeogr. Palaeoclimatol. Palaeoecol. 214, 27–40. doi:10.1016/j.palaeo.2004.06.013
Mulholland, P. J. (1981). Organic Carbon Flow in a Swamp‐Stream Ecosystem. Ecol. Monogr. 51, 307–322. doi:10.2307/2937276
Nahlik, A. M., and Fennessy, M. S. (2016). Carbon Storage in US Wetlands. Nat. Commun. 7, 13835. doi:10.1038/ncomms13835
Naiman, R. J., Melillo, J. M., Lock, M. A., Ford, T. E., and Reice, S. R. (1987). Longitudinal Patterns of Ecosystem Processes and Community Structure in a Subarctic River Continuum. Ecology 68, 1139–1156. doi:10.2307/1939199
Nardi, F., Annis, A., Di Baldassarre, G., Vivoni, E. R., and Grimaldi, S. (2019). GFPLAIN250m, a Global High-Resolution Dataset of Earth's Floodplains. Sci. Data 6, 180309. doi:10.1038/sdata.2018.309
Noe, G. B., and Hupp, C. R. (2009). Retention of Riverine Sediment and Nutrient Loads by Coastal Plain Floodplains. Ecosystems 12, 728–746. doi:10.1007/s10021-009-9253-5
O’Connor, J. E., Jones, M. A., and Haluska, T. L. (2003). Flood plain and Channel Dynamics of the Quinault and Queets Rivers, Washington, USA. Geomorphology 51, 31–59. doi:10.1016/S0169-555X(02)00324-0
Oswald, E. B., and Wohl, E. (2008). Wood-mediated Geomorphic Effects of a Jökulhlaup in the Wind River Mountains, Wyoming. Geomorphology 100, 549–562. doi:10.1016/j.geomorph.2008.02.002
Perignon, M. C., Tucker, G. E., Griffin, E. R., and Friedman, J. M. (2013). Effects of Riparian Vegetation on Topographic Change during a Large Flood Event, Rio Puerco, New Mexico, USA. J. Geophys. Res. Earth Surf. 118, 1193–1209. doi:10.1002/jgrf.20073
Piégay, H., and Gurnell, A. M. (1997). Large Woody Debris and River Geomorphological Pattern: Examples from S.E. France and S. England. Geomorphology 19, 99–116. doi:10.1016/s0169-555x(96)00045-1
Piégay, H. (1993). Nature, Mass and Preferential Sites of Coarse Woody Debris Deposits in the Lower Ain Valley (Mollon Reach), France. Regul. Rivers: Res. Mgmt. 8, 359–372. doi:10.1002/rrr.3450080406
Pollock, M. M., Beechie, T. J., Wheaton, J. M., Jordan, C. E., Bouwes, N., Weber, N., et al. (2014). Using beaver Dams to Restore Incised Stream Ecosystems. BioScience 64, 279–290. doi:10.1093/biosci/biu036
Polvi, L. E., and Wohl, E. (2012). The beaver Meadow Complex Revisited - the Role of Beavers in post-glacial Floodplain Development. Earth Surf. Process. Landforms 37, 332–346. doi:10.1002/esp.2261
Powers, P. D., Helstab, M., and Niezgoda, S. L. (2019). A Process‐based Approach to Restoring Depositional River Valleys to Stage 0, an Anastomosing Channel Network. River Res. Applic 35, 3–13. doi:10.1002/rra.3378
Rasmussen, C., Heckman, K., Wieder, W. R., Keiluweit, M., Lawrence, C. R., Berhe, A. A., et al. (2018). Beyond clay: towards an Improved Set of Variables for Predicting Soil Organic Matter Content. Biogeochemistry 137, 297–306. doi:10.1007/s10533-018-0424-3
Rathburn, S. L., Rubin, Z. K., and Wohl, E. E. (2011). Evaluating Channel Response to an Extreme Sedimentation Event in the Context of Historical Range of Variability: Upper Colorado River, USA. Earth Surf. Process. Landforms 38, 391–406.
RCoreTeam (2020). R: A Language and Environment for Statistical Computing. Vienna, Austria: R Foundation for Statistical Computing. Available at: https://www.R-project.org/.
Ricker, M. C., Donohue, S. W., Stolt, M. H., and Zavada, M. S. (2012). Development and Application of Multi-Proxy Indices of Land Use Change for Riparian Soils in Southern New England, USA. Ecol. Appl. 22, 487–501. doi:10.1890/11-1640.1
Ricker, M. C., and Lockaby, B. G. (2015). Soil Organic Carbon Stocks in a Large Eutrophic Floodplain Forest of the Southeastern Atlantic Coastal Plain, USA. Wetlands 35, 291–301. doi:10.1007/s13157-014-0618-y
Ruehlmann, J., and Korschens, M. (2009). Calculating the effect of soil organic matter concentration on soil bulk density. Soil Sci. Soc. Am. J. 73, 876–885.
Sanders, L. M., Taffs, K. H., Stokes, D. J., Sanders, C. J., Smoak, J. M., Enrich‐Prast, A., et al. (2017). Carbon Accumulation in Amazonian Floodplain Lakes: a Significant Component of Amazon Budgets. Limnol. Oceanogr. 2, 29–35. doi:10.1002/lol2.10034
Sawyer, A. H., and Cardenas, M. B. (2012). Effect of Experimental wood Addition on Hyporheic Exchange and thermal Dynamics in a Losing Meadow Stream. Water Resour. Res. 48, W10537. doi:10.1029/2011WR011776
Scharlemann, J. P., Tanner, E. V., Hiederer, R., and Kapos, V. (2014). Global Soil Carbon: Understanding and Managing the Largest Terrestrial Carbon Pool. Carbon Manage. 5, 81–91. doi:10.4155/cmt.13.77
Schiefer, J., Lair, G. J., Lüthgens, C., Wild, E. M., Steier, P., and Blum, W. E. H. (2018). The Increase of Soil Organic Carbon as Proposed by the "4/1000 Initiative" Is Strongly Limited by the Status of Soil Development - A Case Study along a Substrate Age Gradient in Central Europe. Sci. Total Environ. 628-629, 840–847. doi:10.1016/j.scitotenv.2018.02.008
Scott, D. N., and Wohl, E. E. (2018a). Geomorphic Regulation of Floodplain Soil Organic Carbon Concentration in Watersheds of the Rocky and Cascade Mountains, USA. Earth Surf. Dynam. 6, 1101–1114. doi:10.5194/esurf-6-1101-2018
Scott, D. N., and Wohl, E. E. (2018b). Natural and Anthropogenic Controls on wood Loads in River Corridors of the Rocky, Cascade, and Olympic Mountains, USA. Water Resour. Res. 54, 7893–7909. doi:10.1029/2018wr022754
Scott, D. N., and Wohl, E. (2020). Geomorphology and Climate Interact to Control Organic Carbon Stock and Age in Mountain River valley Bottoms. Earth Surf. Process. Landforms 45, 1911–1925. doi:10.1002/esp.4855
Smith, R. F., Neideigh, E. C., Rittle, A. M., and Wallace, J. R. (2020). Assessing Macroinvertebrate Community Response to Restoration of Big Spring Run: Expanded Analysis of Before‐after‐control‐impact Sampling Designs. River Res. Applic 36, 79–90. doi:10.1002/rra.3556
Soil Survey Staff (2021). Natural Resources Conservation Service. US Department of Agriculture. Web Soil Survey. Available at: https://websoilsurvey.nrcs.usda.gov/(Accessed February 5, 2021).
Structx (2021). Available at: https://structx.com/Soil_Properties_002.html (Accessed April 16, 2021).
Sutfin, N. A., Wohl, E. E., and Dwire, K. A. (2016). Banking Carbon: a Review of Organic Carbon Storage and Physical Factors Influencing Retention in Floodplains and Riparian Ecosystems. Earth Surf. Process. Landforms 41, 38–60. doi:10.1002/esp.3857
Sutfin, N. A., and Wohl, E. (2019). Elevational Differences in Hydrogeomorphic Disturbance Regime Influence Sediment Residence Times within Mountain River Corridors. Nat. Commun. 10, 2221. doi:10.1038/s41467-019-09864-w
Sutfin, N. A., and Wohl, E. (2017). Substantial Soil Organic Carbon Retention along Floodplains of Mountain Streams. J. Geophys. Res. Earth Surf. 122, 1325–1338. doi:10.1002/2016jf004004
Swinnen, W., Daniëls, T., Maurer, E., Broothaerts, N., and Verstraeten, G. (2020). Geomorphic Controls on Floodplain Sediment and Soil Organic Carbon Storage in a Scottish Mountain River. Earth Surf. Process. Landforms 45, 207–223. doi:10.1002/esp.4729
Tangen, B. A., and Bansal, S. (2020). Soil Organic Carbon Stocks and Sequestration Rates of Inland, Freshwater Wetlands: Sources of Variability and Uncertainty. Sci. Total Environ. 749, 141444. doi:10.1016/j.scitotenv.2020.141444
Tockner, K., Pennetzdorfer, D., Reiner, N., Schiemer, F., and Ward, J. V. (1999). Hydrological Connectivity, and the Exchange of Organic Matter and Nutrients in a Dynamic River-Floodplain System (Danube, Austria). Freshw. Biol. 41, 521–535. doi:10.1046/j.1365-2427.1999.00399.x
Tooth, S., and McCarthy, T. S. (2007). Wetlands in Drylands: Geomorphological and Sedimentological Characteristics, with Emphasis on Examples from Southern Africa. Prog. Phys. Geogr. Earth Environ. 31, 3–41. doi:10.1177/0309133307073879
Triska, F. J. (1984). Role of wood Debris in Modifying Channel Geomorphology and Riparian Areas of a Large lowland River under Pristine Conditions: A Historical Case Study. SIL Proc. 1922-2010 22, 1876–1892. doi:10.1080/03680770.1983.11897589
Villa, J. A., and Bernal, B. (2018). Carbon Sequestration in Wetlands, from Science to Practice: an Overview of the Biogeochemical Process, Measurement Methods, and Policy Framework. Ecol. Eng. 114, 115–128. doi:10.1016/j.ecoleng.2017.06.037
Walling, D. E. (2006). Human Impact on Land-Ocean Sediment Transfer by the World's Rivers. Geomorphology 79, 192–216. doi:10.1016/j.geomorph.2006.06.019
Walter, R. C., and Merritts, D. J. (2008). Natural Streams and the Legacy of Water-Powered Mills. Science 319, 299–304. doi:10.1126/science.1151716
Westbrook, C. J., Cooper, D. J., and Baker, B. W. (2006). Beaver Dams and Overbank Floods Influence Groundwater-Surface Water Interactions of a Rocky Mountain Riparian Area. Water Resour. Res. 42, W06404. doi:10.1029/2005WR004560
Whiting, G. J., and Chanton, J. P. (2001). Greenhouse Carbon Balance of Wetlands: Methane Emission versus Carbon Sequestration. Tellus B. 53, 521–528. doi:10.1034/j.1600-0889.2001.530501.x
Wittmann, H., and von Blanckenburg, F. (2009). Cosmogenic Nuclide Budgeting of Floodplain Sediment Transfer. Geomorphology 109, 246–256. doi:10.1016/j.geomorph.2009.03.006
Wohl, E. (2014). A Legacy of Absence. Prog. Phys. Geogr. Earth Environ. 38, 637–663. doi:10.1177/0309133314548091
Wohl, E., and Cadol, D. (2011). Neighborhood Matters: Patterns and Controls on wood Distribution in Old-Growth forest Streams of the Colorado Front Range, USA. Geomorphology 125, 132–146. doi:10.1016/j.geomorph.2010.09.008
Wohl, E., Cadol, D., Pfeiffer, A., Jackson, K., and Laurel, D. (2018a). Distribution of Large wood within River Corridors in Relation to Flow Regime in the Semiarid Western US. Water Resour. Res. 54, 1890–1904. doi:10.1002/2017wr022009
Wohl, E., Dwire, K., Sutfin, N., Polvi, L., and Bazan, R. (2012). Mechanisms of Carbon Storage in Mountainous Headwater Rivers. Nat. Commun. 3, 1263. doi:10.1029/ncomms227410.1038/ncomms2274
Wohl, E., Hall, R. O., Lininger, K. B., Sutfin, N. A., and Walters, D. M. (2017). Carbon Dynamics of River Corridors and the Effects of Human Alterations. Ecol. Monogr. 87, 379–409. doi:10.1002/ecm.1261
Wohl, E., Lane, S. N., and Wilcox, A. C. (2015). The Science and Practice of River Restoration. Water Resour. Res. 51, 5974–5997. doi:10.1002/2014wr016874
Wohl, E. (2015). Particle Dynamics: the Continuum of Bedrock to Alluvial River Segments. Geomorphology 241, 192–208. doi:10.1016/j.geomorph.2015.04.014
Wohl, E., and Pfeiffer, A. (2018). Organic Carbon Storage in Floodplain Soils of the U.S. Prairies. River Res. Applic. 34, 406–416. doi:10.1002/rra.3269
Wohl, E., Scott, D. N., and Lininger, K. B. (2018b). Spatial Distribution of Channel and Floodplain Large wood in Forested River Corridors of the Northern Rockies. Water Resour. Res. 54, 7879–7892. doi:10.1029/2018wr022750
Wohl, E. (2011). What Should These Rivers Look like? Historical Range of Variability and Human Impacts in the Colorado Front Range, USA. Earth Surf. Process. Landforms 36, 1378–1390. doi:10.1002/esp.2180
Wohl, E. (2020). Wood Process Domains and wood Loads on Floodplains. Earth Surf. Process. Landforms 45, 144–156. doi:10.1002/esp.4771
Wolf, E. C., Cooper, D. J., and Hobbs, N. T. (2007). Hydrologic Regime and Herbivory Stabilize an Alternative State in Yellowstone National Park. Ecol. Appl. 17, 1572–1587. doi:10.1890/06-2042.1
Keywords: floodplain, large wood, organic carbon, soil carbon, restoration, Oregon
Citation: Hinshaw S and Wohl E (2021) Quantitatively Estimating Carbon Sequestration Potential in Soil and Large Wood in the Context of River Restoration. Front. Earth Sci. 9:708895. doi: 10.3389/feart.2021.708895
Received: 12 May 2021; Accepted: 06 October 2021;
Published: 19 October 2021.
Edited by:
Peter Raymond, Yale University, United StatesReviewed by:
Erkan Istanbulluoglu, University of Washington, United StatesRobert Jacobson, United States Geological Survey, United States
Copyright © 2021 Hinshaw and Wohl. This is an open-access article distributed under the terms of the Creative Commons Attribution License (CC BY). The use, distribution or reproduction in other forums is permitted, provided the original author(s) and the copyright owner(s) are credited and that the original publication in this journal is cited, in accordance with accepted academic practice. No use, distribution or reproduction is permitted which does not comply with these terms.
*Correspondence: Ellen Wohl, RWxsZW4ud29obEBjb2xvc3RhdGUuZWR1