- 1CSIR - National Institute of Oceanography, Dona Paula, Goa, India
- 2School of Earth Ocean and Atmospheric Sciences, Goa University, Taleigao, Goa, India
In this study, we conducted a comprehensive investigation of rock magnetic, mineralogical, and sedimentological records of sediment cores supplemented by a high resolution seismic data to elucidate the controls of structural and diagenetic (early vs. late) processes on the sediment magnetism in active and relict cold seep sites in the Bay of Bengal. Two distinct sediment magnetic zones (Z-I and Z-II) are defined based on the down-core variations in rock magnetic properties. The sediment magnetism is carried by complex magnetic mineral assemblages of detrital (titanomagnetite, titanohematite) and authigenic (fine-grained greigite) minerals. Overall, the magnetic susceptibility varies over one order of magnitude with highest values found in relict core. Uppermost sediment magnetic zone (Z-I) is characterized by higher concentration of magnetite as seen through elevated values of magnetic susceptibility (χlf) and saturation isothermal remanent magnetization (SIRM). A systematic gradual decrease of χlf and IRM1T in Z-I is attributed to the progressive diagenetic dissolution of iron oxides and subsequent precipitation of iron sulfides. Magnetic grain size diagnostic (ARM/IRM1T) parameter decreases initially due to the preferential dissolution of fine-grained magnetite in the sulfidic zone (Z-I), and increases later in response to the authigenic formation of magnetite and greigite in methanic zone (Z-II). Distinct low S-ratio and χlf values in methanic zone of relict core is due to increased relative contribution from highly preserved coercive magnetic (titanohematite) grains of detrital origin which survived in the diagenetic processes. A strong linkage between occurrence of authigenic carbonates and greigite formation is observed. Two plausible mechanisms are proposed to explain the formation and preservation of greigite in Z-I and Z-II: 1) decline in methane flux due to massive hydrate accumulation within the active fault system and formation of authigenic carbonate crust in the sub-surface sediments hindered the supply of upward migrating fluid/gas; thereby limiting the sulfide production which preferentially enhanced greigite formation in Z-I and 2) restricted supply of downward diffusing sulfide by the carbonate layers in the uppermost sediments created a sulfide deficient zone which inhibited the pyritization and favoured the formation of greigite in the methanic zone (Z-II).
Introduction
At active cold seeps, methane and hydrogen sulfide fluids emanate from the seafloor. These fluids led to diagenesis of magnetic minerals at or below the sediment-water interface, wherein primary detrital iron-bearing magnetic minerals undergoes reductive dissolution and are converted into iron sulfides e.g., greigite, pyrrhotite, pyrite (Canfield and Berner, 1987; Boetius et al., 2000; Jørgensen et al., 2004; Rowan et al., 2009; Dewangan et al., 2013; Riedinger et al., 2014; Suess, 2014; Kars and Kodama 2015; Roberts, 2015; Lin et al., 2017; Rodelli et al., 2018; Amiel et al., 2020). Rock magnetic properties of methanic sediments from active cold seep and gas hydrate dominated sedimentary systems have been extensively studied to understand the magnetic mineral diagenesis. For example in Nankai trough, Japan (Kars and Kodama, 2015), Cascadia Margin (Housen and Musgrave, 1996; Larrasoaña et al., 2007), continental margin offshore of south-western Taiwan (Horng and Chen, 2006), continental margin off Argentina and Uruguay (Riedinger et al., 2014), Bay of Bengal (Badesab et al., 2017; Badesab et al., 2019; Badesab et al., 2020a; Badesab et al., 2020b; Badesab et al., 2020c), Southern Eastern Mediterranean continental shelf (Amiel et al., 2020), Niger deep-sea fan (Dillon and Bleil, 2006), Bulls eye vent off Vancouver Island, Canada (Novosel et al., 2005), and North-western Weddell Sea (Reilly et al., 2020).
Structural control on methane seepage at cold seep sites has been well reported using high resolution seismic and bathymetry data. For example Lomvi pockmark, Vestnesa Ridge, offshore Svalbard (Bünz et al., 2012; Berndt et al., 2014; Yao et al., 2019), Harstad Basin, southwest Barents Sea (Crémière et al., 2018), Bay of Bengal (Dewangan et al., 2020), Concepción Methane Seep Area, offshore Central Chile (Geersen et al., 2016), Taixinan Basin, South China Sea (Wang, et al., 2018) Central Nile deep sea fan (Römer et al., 2014), Nankai accretionary prism (Henry et al., 2002), Congo basin (Wenau et al., 2015) and Black Sea (Bohrmann et al., 2003). Extensive geological and geophysical studies established the presence of fracture-filled and massive-type gas hydrate deposits in the Krishna-Godavari (K-G) basin (Collett et al., 2008; Dewangan et al., 2010; Shankar and Riedel, 2010; Dewangan et al., 2011; Kumar et al., 2014). Fractures/faults, generated from shale-tectonism, provide an efficient gas plumbing system in the K-G basin (Dewangan et al., 2010). Fluid migration through these fractures/faults led to the occurrence of paleo-cold seeps in the K-G basin (Mazumdar et al., 2009; Dewangan et al., 2010). In some active faults, methane migrates up to the seafloor and form active/relict cold seep system associated with shallow gas hydrates (Gullapalli et al., 2019; Mazumdar et al., 2019; Figure 1).
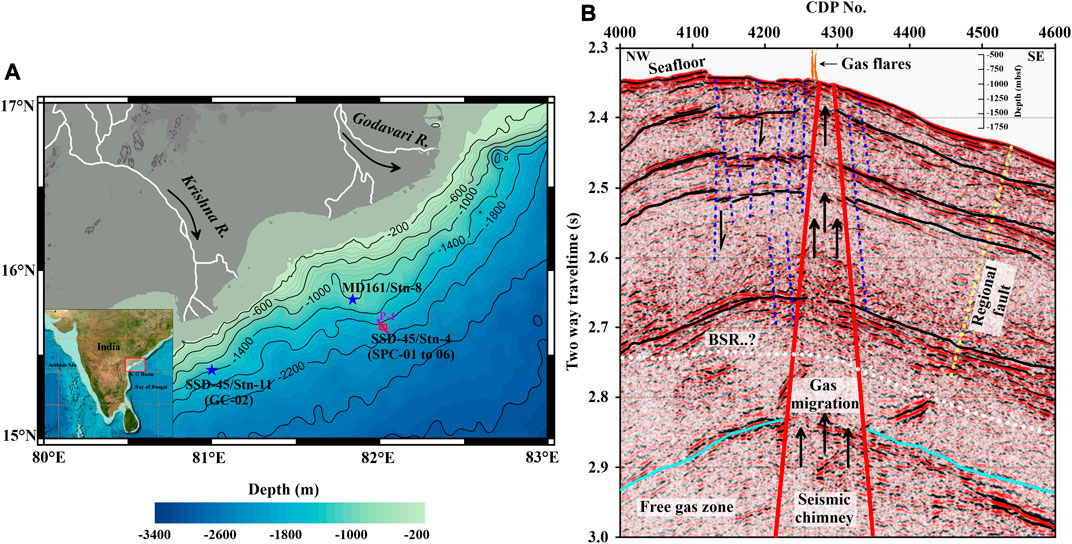
FIGURE 1. (A) Map showing the location of active (SSD-45/Stn-4), relict (SSD-45/Stn-11/GC-02) and paleo (MD161/Stn-08) seep sites in the Krishna-Godavari (K-G) basin, Bay of Bengal High resolution seismic profile (P1) highlighting (B) the normal faults (blue dashed lines), subsurface chimney like feature (solid red lines) and water column imaging showing gas flares (orange). Regional toe-thrust fault (yellow dashed line), feasible BSR (white dashed line), and subsurface horizons (solid black lines) are marked on the seismic section (modified after Dewangan et al., 2020). The spade sediment cores SPC-01, SPC-02, SPC-03, SPC-04, SPC-05, and SPC-06 were retrieved from active seep site SSD-45/Stn-4 and gravity core GC-02 was retrieved from relict site SSD-45/Stn-11. The location of the sediment core from paleo-seep site (MD161/Stn-8) is also marked. Bathymetry data of the studied area was obtained from GEBCO Compilation Group665 (2020) GEBCO 2020 Grid (10.5285/a29c5465-b138-234d-e053-6c86abc040b9).
Methane migrates from deep-seated gas reservoirs and interacts with downward diffusing seawater sulfate to trigger multiple zones of anaerobic oxidation of methane (AOM) (Jørgensen et al., 2004; Knittel and Boetius, 2009; Lin et al., 2018) driven by sulfate reduction (Mazumdar et al., 2019). At seep sites, non-steady and multiple diagenetic fronts would yield a complex pattern of magnetic mineral assemblages (Roberts, 2015; Reilly et al., 2020). Recent study by Reilly et al. (2020) provides new insights on magnetic mineral diagenesis through a shallow sulfate-methane transition zone (SMTZ) in a sediment core from Perseverance Drift, Northwestern Weddell Sea, Antartica. They reported that non-steady state disturbances including fluctuations in carbon flux and sedimentation can shift the pore-water geochemical profiles resulting in preservation of authigenic ferrimagnetic iron sulfides. In contrast, steady-state diagenetic processes at relict sites would lead to dissolution of detrital magnetic minerals followed by subsequent diagenetic formation of iron sulfide (pyrite) which can significantly reduce magnetic susceptibility of the bulk sediments (Canfield and Berner, 1987; Roberts et al., 2005; Roberts and Weaver, 2005; Mohamed et al., 2011; Kars and Kodama, 2015; Roberts, 2015; Amiel et al., 2020; Lin et al., 2020; Reilly et al., 2020).
Magnetic minerals are potential recorders of the geological and methane-related geochemical processes and provide vital information on the methane seepage dynamics and diagenetic processes in marine gas hydrate sedimentary system (Musgrave et al., 2006; Larrasoaña et al., 2007; Kars and Kodama, 2015; Roberts, 2015; Badesab et al., 2019). Previous rock magnetic studies on sediment cores overlying shallow and deep-seated gas hydrate deposits in the K-G basin mainly aimed at delineating the magnetic signatures of detrital and diagenetic processes associated with evolution of gas-hydrate system (Dewangan et al., 2013; Badesab et al., 2017). For example, a magnetic based proxy for deciphering paleo-methane seepage events and sediment dispersal patterns, tracking of the rapid sedimentation events and its control on the magnetic mineral diagenesis and evolution of gas hydrate deposits was developed (Usapkar et al., 2014; Badesab et al., 2017; Badesab et al., 2019; Badesab et al., 2020b). Furthermore, the mechanism of formation and preservation of greigite in deep methanic sediments were unravelled by Badesab et al. (2020c). The occurrence of silicate-hosted greigite inclusion were reported by Badesab et al. (2020a). They further highlighted its importance in understanding magnetic mineral diagenesis in the gas hydrate system. More recently, Badesab et al. (2020c) conducted a comprehensive rock magnetic and mineralogical investigation on a seep-impacted sediment core from a newly discovered site and successfully delineated the processes governing the methane seepage dynamics and evolution of shallow gas hydrate (2–3 mbsf) system in the K-G basin. However, a dedicated rock magnetic study focussing on understanding the complex diagenetic (early and late) processes controlled by variability in methane fluxes and underlying fault/fractures at active and relict seep dominated sedimentary systems is still lacking.
Sediment cores retrieved during gas hydrate discovery cruise in 2018 in the K-G basin provides an excellent opportunity to establish a magnetic mineral inventory and investigate the diagenetic processes at active/relict and paleo-seep sites associated with shallow and deep-seated marine gas hydrates respectively. The active seep sites (SPC-01 to SPC-06) are mainly characterized by gas flares as observed in the water-column images, higher methane flux and presence of live chemosynthesis dependent organisms predominantly Bivalvia, Gastropods, and Polychaete (Mazumdar et al., 2019; Dewangan et al., 2020). Relict seep site (Stn-11/GC-02) is characterized by the absence of gas flares, presence of non-living shell fragment, Calyptogena Sp. and occurrence light brown colored authigenic carbonates at 48 cm below sea floor at this site. Contrastingly, paleo-seep site (MD161/Stn-8) is marked by the presence of methane-derived carbonates, non-living with chemosynthetic clams (Calyptogena sp.) and authigenic carbonates within 17–23 mbsf in this core which provided evidence of seepage of methane and sulfide-bearing fluids to the seafloor in the geological past (Mazumdar et al., 2009). In this study, we conducted a comprehensive investigation of rock magnetic, mineralogical, and sedimentological records of sediment cores supplemented by high resolution seismic data to elucidate the controls of structural and diagenetic (early vs. late) processes on the sediment magnetism in an active (SSD-45/Stn-4/SPC-01 to SPC-06) and relict (SSD-45/Stn-11/GC-02) cold seep sites in the Bay of Bengal.
Geology of Krishna-Godavari Basin
Krishna-Godavari (K-G) basin is situated along the eastern continental margin of India (Figure 1). The K-G basin covers onshore area of 28,000 km2 and offshore area about 145,000 km2 and extends from Ongole in South to Vishakhapatnam in north (Ojha and Dubey, 2006). Average sediment thickness is about 3–5 km in onshore region, which increases to about 8 km in the offshore region (Prabhakar and Zutshi, 1993; Bastia, 2007). The Krishna and Godavari rivers and their tributaries deliver major sediment load to the K-G basin. Annual sediment load delivered by the Krishna and Godavari rivers is estimated to be around 67.7 × 106 and 170 × 106 metric ton respectively (Biksham and Subramanian, 1988; Ramesh and Subramanian, 1988). Both the river systems flows through the Deccan trap basalt and Precambrian metamorphic rocks and supplies large amount of detrital magnetite-rich sediments to the K-G basin (Ramesh and Subramanian 1988; Sangode et al., 2007). Sediments in the K-G basin are primarily composed of montmorillonite clay with traces of illite and kaolinite (Rao, 1991). Neo-tectonic activities in the K-G basin resulted in the formation of geomorphic structures including shale diapirs, mounds, and faults. Migration of methane through the fault system favoured the accumulation of gas hydrates in the K-G basin (Dewangan et al., 2010).
Methodology
Sampling and Measurements
During gas hydrate exploration cruise (SSD-45) of Council of Scientific and Industrial Research -National Institute of Oceanography (CSIR-NIO) onboard R/V Sindhu Sadhana, six short spade cores (SPC-01 to SPC-06; Table. 1) were retrieved from active site (SSD-45/Stn-4) and one gravity core (Stn-11/GC-02) from relict seep site (as evident through the presence of non-living shell fragment, Calyptogena Sp. and authigenic carbonates at 48 cmbsf in this core) in the K-G basin (Figure 1). Upon recovery, sediment cores from active sites smelled strongly of hydrogen sulfide (H2S) suggesting a shallow SMTZ that motivated this study. Magnetic samples were taken onboard immediately after collection of pore water samples to minimize sediment alteration due to warmer temperatures and oxygen exposure. The cores were sub-sampled in the presence of high purity nitrogen flushing to minimize the exposure of samples to atmospheric conditions, which could oxidize hydrogen sulfide or iron mono-sulfide. Samples for magnetic analysis were collected by pushing non-magnetic plastic bottles into the split halves at ∼2 cm intervals. After sub-sampling, magnetic samples were transferred to a cold room (4°C) for storage. Magnetic measurements were carried out on wet sediment samples (packed in 25 mm plastic sample bottled) at the Paleomagnetic laboratory of CSIR-NIO and specialized rock magnetic measurements on dry samples at Center for Advanced Marine Core Research (CMCR), Kochi University, Japan.
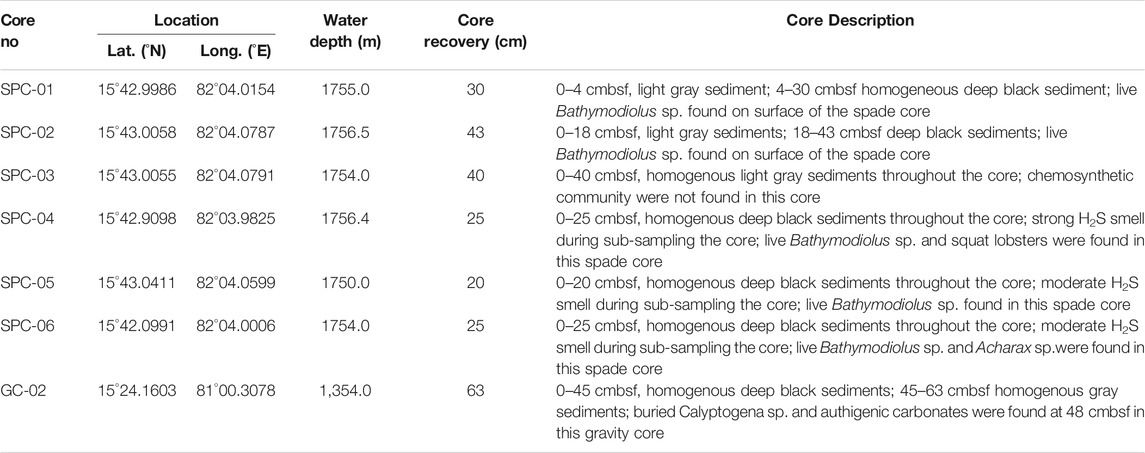
TABLE 1. Information of spade sediment cores retrieved from an active seep site in the Krishna-Godavari (K-G) basin, Bay of Bengal.
Magnetic Measurements
Low-field magnetic susceptibility measurements were carried out using a Bartington Instruments MS2B dual frequency susceptibility meter. The susceptibility was measured at low (lf) and high (hf) frequencies χlf = 0.47 kHz and χhf = 4.7 kHz. The frequency-dependent susceptibility was calculated as χfd % = (χlf − χhf)/χlf × 100%. All remanent magnetizations were measured using an AGICO JR-6A spinner magnetometer. An anhysteretic remanent magnetization (ARM) was imparted using a 100 mT alternating magnetic field superimposed on a fixed direct current (DC) bias field of 50 μT. An isothermal remanent magnetization (IRM) was imparted using an inducing field of +1T in the forward direction by a MMPM10 pulse magnetizer. S-ratio is calculated as the ratio of IRM at −300 mT and SIRM (IRM−300 mT/IRM1T) (Thompson and Oldfield, 1986). Thermomagnetic measurements were conducted on eight selected samples using a Natsuhara Giken (Model NMB-89) magnetic balance at CMCR with a heating rate of 10°C/min in a 0.3-T field.
Hysteresis loops, first-order reversal curves (FORC) (Pike et al., 1999), and back-field curves were also measured for 14 selected samples with a maximum field of 1T (number of loops per FORC: 91, averaging time: 200 ms, field increment: 4 mT, and a slew rate limit: 1T/s) at CMCR. Processing of FORC diagrams were done using the FORCinel software (Harrison and Feinberg, 2008). FORC distributions were processed using a smoothing factor (SF) of 5, but SF = 6 were used where possible. Low-temperature magnetic measurements were conducted on selected samples using a Quantum Design Magnetic Properties Measurement System (MPMS) at CMCR. A room temperature SIRM (RT-SIRM) was imparted at room temperature (300 K) in 2.5 T. Samples were then cooled to 5 K and warmed back to 300 K in a zero magnetic field. A low temperature SIRM (LT-SIRM) was then imparted at 5 K in 2.5 T. Samples were warmed up to 300 K in a zero magnetic field (termed “ZFC” for zero field-cooled). Samples were then cooled to 5 K in the presence of a 2.5 T magnetic field. A LT-SIRM was again imparted at 5 K, and samples were warmed to 300 K in a zero magnetic field (termed “FC” for field-cooled).
Bulk Sediment Grain Size Analyses
Sediment grain size measurements were carried out using a Malvern Mastersizer 2000 Laser Particle Size Analyzer at CSIR-NIO. Before analyses, desalination of sediment samples were carried out by washing repeatedly with deionized water followed by decarbonisation using dilute HCl (1N) acid. For removal of organic carbon, samples were treated with 10% H2O2 and later, Sodium hexametaphosphate was added to sediments for better dispersal of the sediment fractions. Grain size values are reported as volume%.
Mineralogical Analysis
Magnetic particles were extracted from bulk sediments following the separation method of Petersen et al. (1986). Bulk sediment samples were disintegrated into 500 ml of distilled water with addition of 200 mg of a dispersing agent (sodium hexametaphosphate) and suspensions were treated with ultrasonics. Further, sediment suspension was allowed to continuously flow through the magnetic extraction set-up. The extraction method is based on the principle that high magnetic field gradients are created by inserting magnets on the teflon coated magnetic finger. During continuous circulation, magnetic particles within the bulk sediments get attracted towards the magnetic finger. These magnetic fractions are removed and washed several times in a small volume of distilled water to remove clay fractions and other contaminants. Magnetic particles were mounted on a carbon tape and were carbon coated for imaging. Images of magnetic grains were captured in secondary electron (SE) imaging mode at energy levels between 15 and 20 keV using a scanning electron microscope (SEM; JEOL JSM-5800 LV) at CSIR-NIO. Energy dispersive X-ray spectroscopy (EDS) probe attached to the microscope was used to determine the composition of magnetic minerals. Magnetic mineralogy was determined on the extracted magnetic particles using a Rigaku X-Ray Diffractometer (Ultima IV). The samples were allowed to run from 15° to 65° of 2θ at 1°/min scan speed using Cu Kα radiation (λ = 1.5414 Å).
Results
Down-Core Changes in Rock Magnetic Parameters
The magnetic susceptibility (χlf) profile of all spade cores (SPC-01 to SPC-06) from active seep and a gravity core from relict (SSD-45/Stn-11/GC-02) sites along with IRM1T, ARM/IRM1T, IRM1T/χlf and S-ratio are shown in Figure 2. Two distinct sediment magnetic zones (Z-I and Z-II) are defined based on down-core variations of χlf in all cores (Figures 2A,G,M,S,Y,A5,A11). In active methane-seep influenced sediment cores, Z-I is marked by relatively higher values of χlf and IRM1T indicating high concentration of magnetic minerals as compared to that observed in Z-II. Magnetic grain size diagnostic proxy (ARM/IRM1T) showed variations in Z-I and Z-II of all analyzed sediment cores (Figure 2; Maher and Thompson, 1999; Peters and Dekkers, 2003). A general trend of downcore decrease suggesting coarsening in magnetic grain size is observed till the bottom of Z-I in all cores except SPC-02 (Figure 2I). In SPC-01, SPC-03, SPC-04, SPC-06, and GC-02 ARM/IRM1T first decreases probably due to preferential dissolution of fine-grained magnetite in Z-I, and rises downcore in response to the authigenic formation of greigite (Figures 2C,O,U,A7, A13) or fine-grained magnetite (Rodelli et al., 2019; Lin et al., 2020) in Z-II. A mixed trend of ARM/IRM1T in SPC-02 and SPC-05 reflects the dominance of both fine and coarse magnetic particles in Z-II (Figures 2I,A1). Two distinct patterns in grain size indicator of magnetic iron sulfides (IRM1T/χlf) are observed (Figure 2; Maher and Thompson, 1999; Peters and Dekkers, 2003; Snowball, 1991; Snowball and Thompson, 1990). A trend of initial rise in IRM1T/χlf values are noticed in Z-I of cores SPC-01, SPC-02, SPC-03, SPC-05, and GC-02 suggest the presence of fine grained magnetic particles (Figures 2D,J,P,A2). After initial rise, a gradual decline in IRM1T/χlf values till end of Z-II of the same cores is noticed (Figures 2D,J,P,A2,A14). Overall, S-ratios vary between 0.82 and 0.99 for majority of the samples suggesting that bulk magnetic mineralogy is characterized by both soft as well as hard coercive minerals in the cores from active seep sites (Figures 2E,K,Q,W,A3,A9; Frank and Nowaczyk, 2008). A systematic downcore decrease in χfd (%) is seen in all cores from active sites (Figure 2F,L,R,X,A4,A10). High values in Z-I indicate higher concentration of fine-grained magnetic particles, while relatively lower values in Z-II suggest the relative dominance of coarse magnetic particles. Interestingly, we noticed an opposite trend in χfd (%) profile of relict site. Lower χfd (%) values were found in Z-I and vice-versa (Figure 2A16).
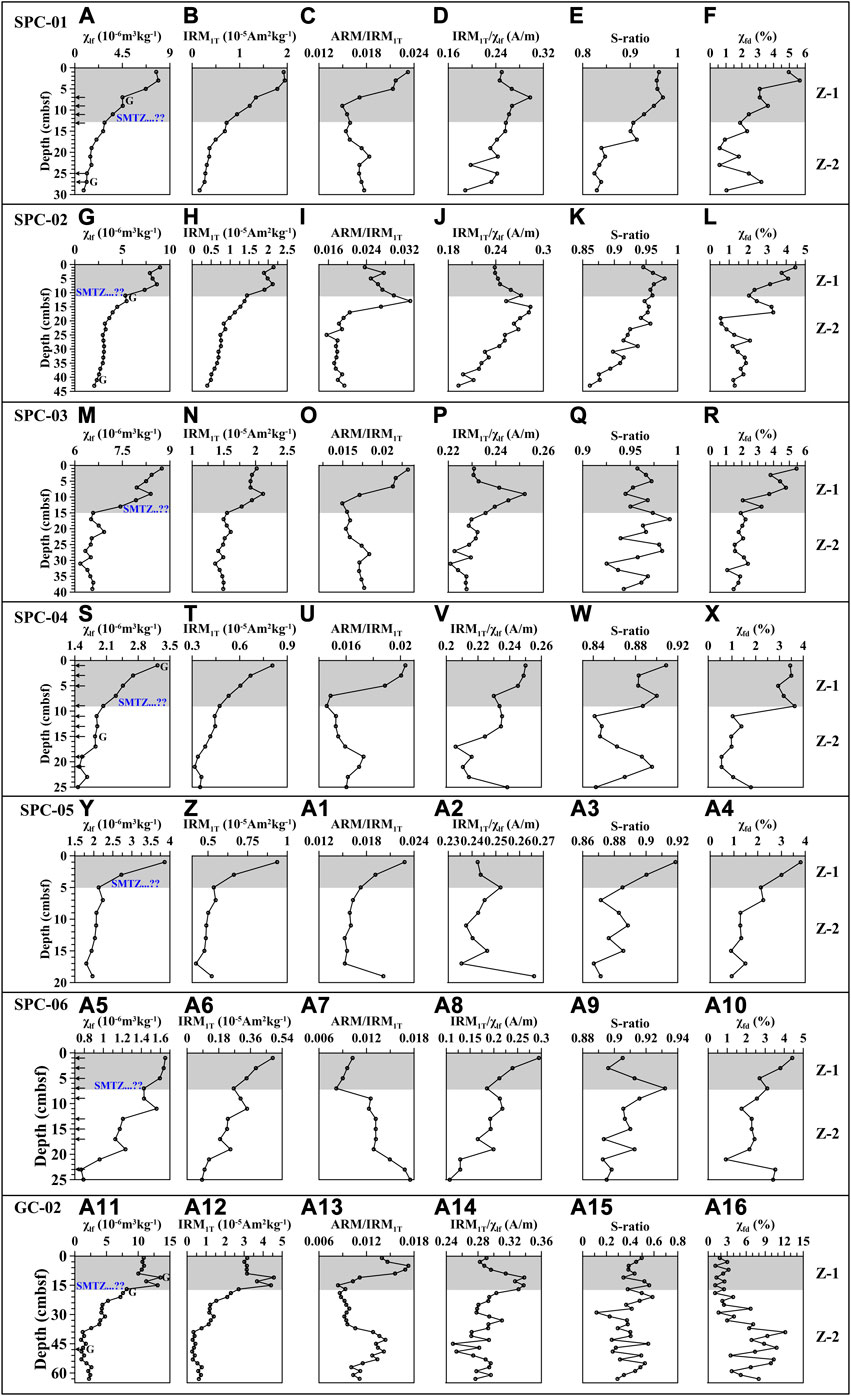
FIGURE 2. Down-core variations of selected rock magnetic data of spade (A–E): SPC-01, (F–J): SPC-02, (K–O): SPC-03, (P–T): SPC-04, (U–Y): SPC-05, (Z–A4): SPC-06, and gravity sediment cores (A5–A9): GC-02. Based on magnetic susceptibility variations two sedimentary magnetic zones Z-I and Z-II are demarcated. Z-I highlighted with gray shading. (Please note that χlf, low frequency magnetic susceptibility; ARM, anhysteretic remanent magnetization; and IRM1T, isothermal remanent magnetization; χfd%, frequency dependent susceptibility). Depth of carbonate occurrence are highlighted in solid black arrows (horizontal). The depth of greigite occurrence is marked by “G.” Sediment magnetic zones (Z-I, Z-II) in each profile are marked.
Magnetic record of relict site (SSD-45/Stn-11/GC-02) showed similar magnetic zonation (Z-I, Z-II), but exhibit different downcore trends in ARM/IRM1T, IRM1T/χlf and S-ratio (Figure 2A11–A16). Three sediment intervals (11 cmbsf, 13 cmbsf, 15 cmbsf) within Z-I showed higher χlf, IRM1T and IRM1T/χlf suggesting the presence of fine-grained ferrimagnetic iron sulfides in these samples (Figures 2A11,A12,A14). An anomalous increase in ARM/IRM1T between 39 and 57 cmbsf suggests the dominance of fine-grained magnetic particles in this interval (Figure 2A13). A major shift in S-ratio at 29 cmbsf followed by a minor rise in IRM1T downcore (29–33 cmbsf) indicates that the relict site has much higher concentration of high-coercive (coarse-grained) minerals (Figures 2A12,A15) as compared to other sites.
Magnetic Mineralogy and Grain Size Diagnostic Parameters
Thermomagnetic Measurements
Figures 3A–H show the thermomagnetic curves of the representative sediment samples from Z-I to Z-II of cores SPC-01/02/03/05. A steady decrease in χ between 552 and 633°C in majority of the samples imply that the bulk magnetic mineralogy is governed by magnetite (Dunlop et al., 1997). Two samples from SPC-01 (21 cmbsf) (Figure 3B) and SPC-02 (41 cmbsf) (Figure 3D) showed a major drop in χ at 695 and 673°C respectively. A minor increase in χ between 357 and 473°C for most of the samples could be either due to the presence of titanomagnetite with a wide range of Ti-contents (Lattard et al., 2006) or because of conversion of paramagnetic minerals into magnetite during heating process (Hirt and Gehring, 1991; Pan et al., 2000; Passier et al., 2001; Figures 3A–H).
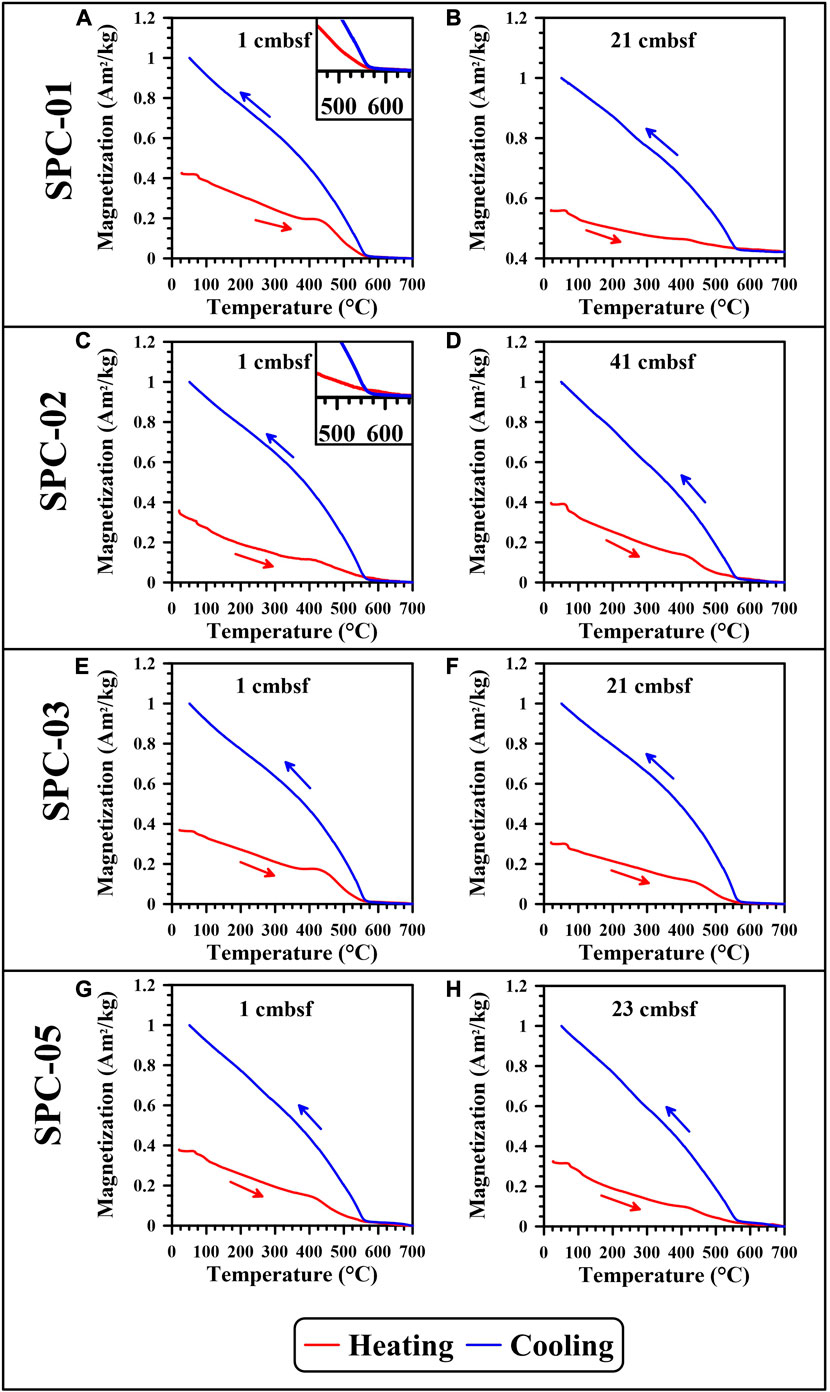
FIGURE 3. Thermomagnetic profiles of selected representative samples representing two sedimentary magnetic zones of the studied spade sediment cores. (A–B): SPC-01, (C–D): SPC-02, (E–F): SPC-03, and (G–H): SPC-05. Solid red lines indicate heating curves, and blue lines indicate cooling curves respectively.
FORC Diagrams
FORC diagram provided additional information on the type of magnetic minerals and their domain states. Representative FORC diagrams for Z-I and Z-II samples of cores SPC-01/02/03/06 are shown in Figure 4A–N. In majority of the samples, FORC distributions are characterized by closed contours with a peak at Bc ∼10–12 mT which suggest that magnetic particles exhibit vortex state to multidomain (MD) type behavior (Figures 4A–N; Roberts et al., 2017; Lascu et al., 2018; Roberts et al., 2020).
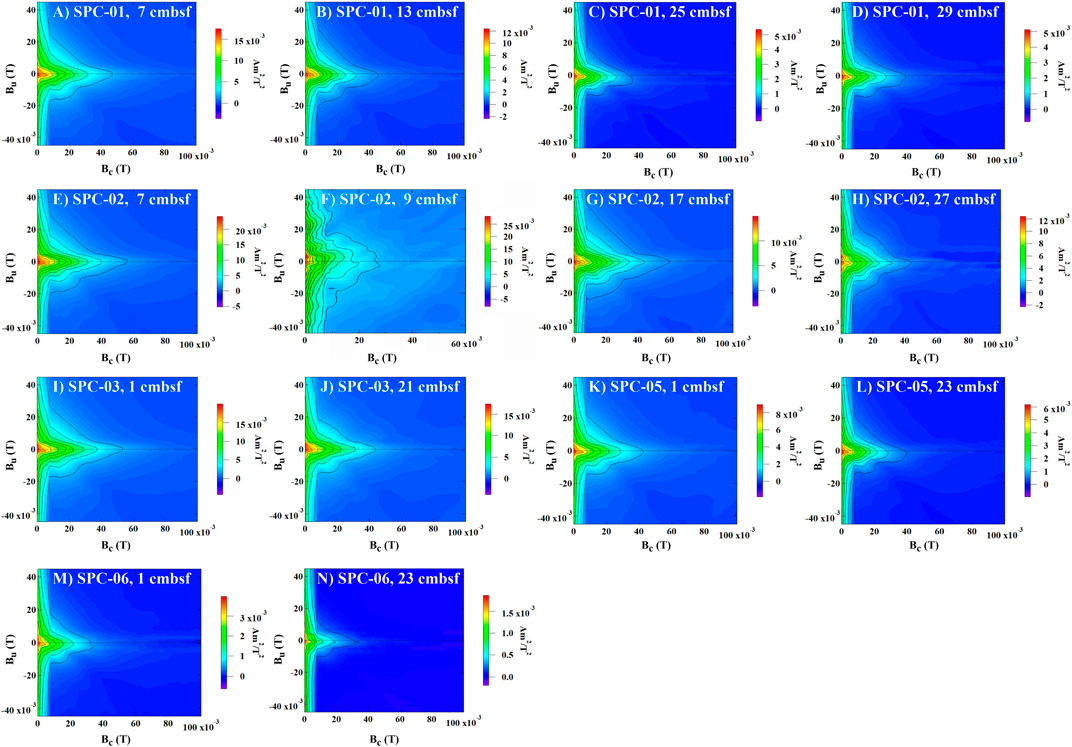
FIGURE 4. First-order reversal curve diagrams for selected representative samples from spade cores. (A–D): SPC-01, (E–H): SPC-02, (I–J): SPC-03, (K–L): SPC-05, and (M–N): SPC-06.
Low-Temperature Measurements
Results of the low-temperature magnetic measurements displaying characteristic magnetic signatures with respect to the sediment magnetic zones (Z-I, Z-II) (Figure 5). A typical Verwey transition (Tv) temperature indicative of presence of magnetite (Figures 5A,B,D–F) is noticed in RT-SIRM, ZFC-FC, and in the first derivative of magnetization curves (Verwey, 1939; Verwey, 1947; Özdemir et al., 2002; Chang et al., 2016a). Samples from magnetically reduced zone (Z-II) do not exhibit any Tv. The displayed LT magnetization curve (Figure 5C) at 29 cmbsf of core SPC-01 is indicative of maghemite (Özdemir and Dunlop, 2010). Approximately 62% loss of RT-SIRM induced at 5 K is seen during warming between 5 and 30 k (Figure 5C) indicating the presence of SP size magnetic particles. We do not observe any Besnus transition (typical for pyrrhotite; Besnus and Meyer, 1964; Dekkers, 1989; Larrasoaña et al., 2007) in the studied samples.
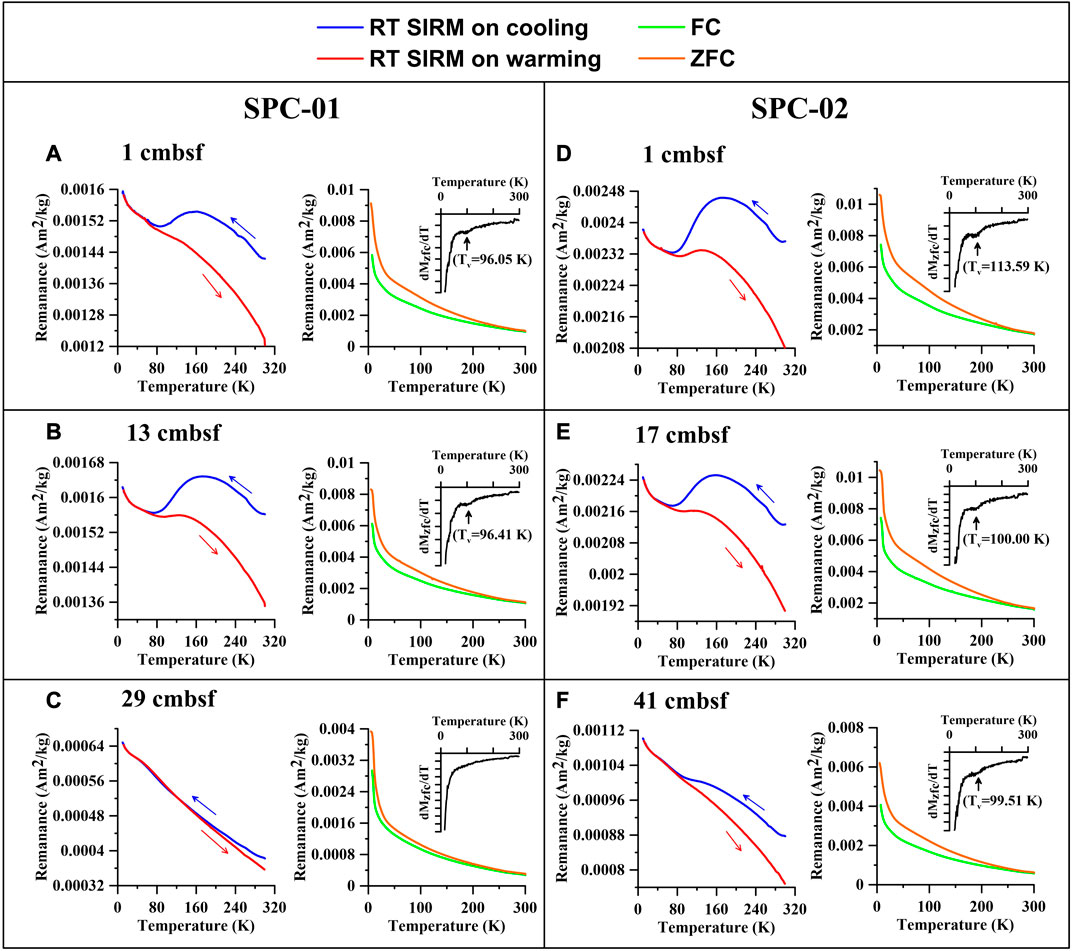
FIGURE 5. Low-temperature magnetization curves for selected representative samples from the two spade cores (A–C): SPC-01 and (D–F): SPC-02. FC, field-cooled; RTSIRM, saturation isothermal remanent magnetization at room temperature; ZFC, zero field-cooled. The corresponding values of Verwey transition (Tv) are placed near the first derivative of magnetization curves.
Identification of Magnetic Mineralogy Using X-Ray Diffraction Analysis and Scanning Electron Microscopy
Titanomagnetite and greigite are the dominant magnetic minerals identified in all the sediment magnetic zones (Figure 6A–M). Greigite is present in both magnetic zones (Z-I and Z-II) of the cores SPC-01 (Figures 6B,C), SPC-02 (Figures 6E,F), and SPC-04 (Figures 6J,K). The electron microscopic images and respective EDS spectra of magnetic particles extracted from different sediment depth intervals of spade cores SPC-01–04 are presented in Figures 7A–L. Ferrimagnetic iron oxides (detrital) and sulfides (diagenetic) minerals of different sizes and shapes are identified in all spade cores (Figures 7A–L). Well-preserved (Figures 7A,D,G,I) as well as diagenetically altered (Figures 7C,H) titanomagnetite grains are found in both sediment magnetic zones. EDS data for these grains indicate the presence of titanium, iron, and oxygen with minor amount of silicon, aluminum, calcium, potassium, manganese and magnesium. Numerous diagenetically formed iron sulfides occurring as framboidal crystals and individual grains are observed (Figures 7B,K,L) and EDS data indicate the presence of sulfur and iron along with minor amounts of aluminum and silicon (Figures 7A–L). Greigite was found to occur as fine-grained minerals only in few samples and the EDS results of these grains show the presence of sulfur and iron with minor traces of calcium, potassium, silicon, aluminum, and magnesium (Figures 7E,F,J). The titanomagnetite grains in Figures 7C,L show a shrinkage-cracks typical of low-temperature mathematization (Nowaczyk, 2011). The cracks on the grains most likely indicate the areas where dissolution might have started. In the relict seep core (GC-02), magnetic mineralogy is carried by titanomagnetite, titanohematite and greigite particles (Figures 8I–P). Z-I is dominated by coarse-grained titanomagnetite and titanohematite grains. A skeletal type titanohematite grains exhibiting the dissolution features are observed in Z-I (Figure 8J) and Z-II (Figures 8M,O,P). Numerous titanomagnetite grains showing the overgrowth of fine-grained greigite particles are noticed in Z-I (Figures 8K,L) and Z-II (Figure 8O).
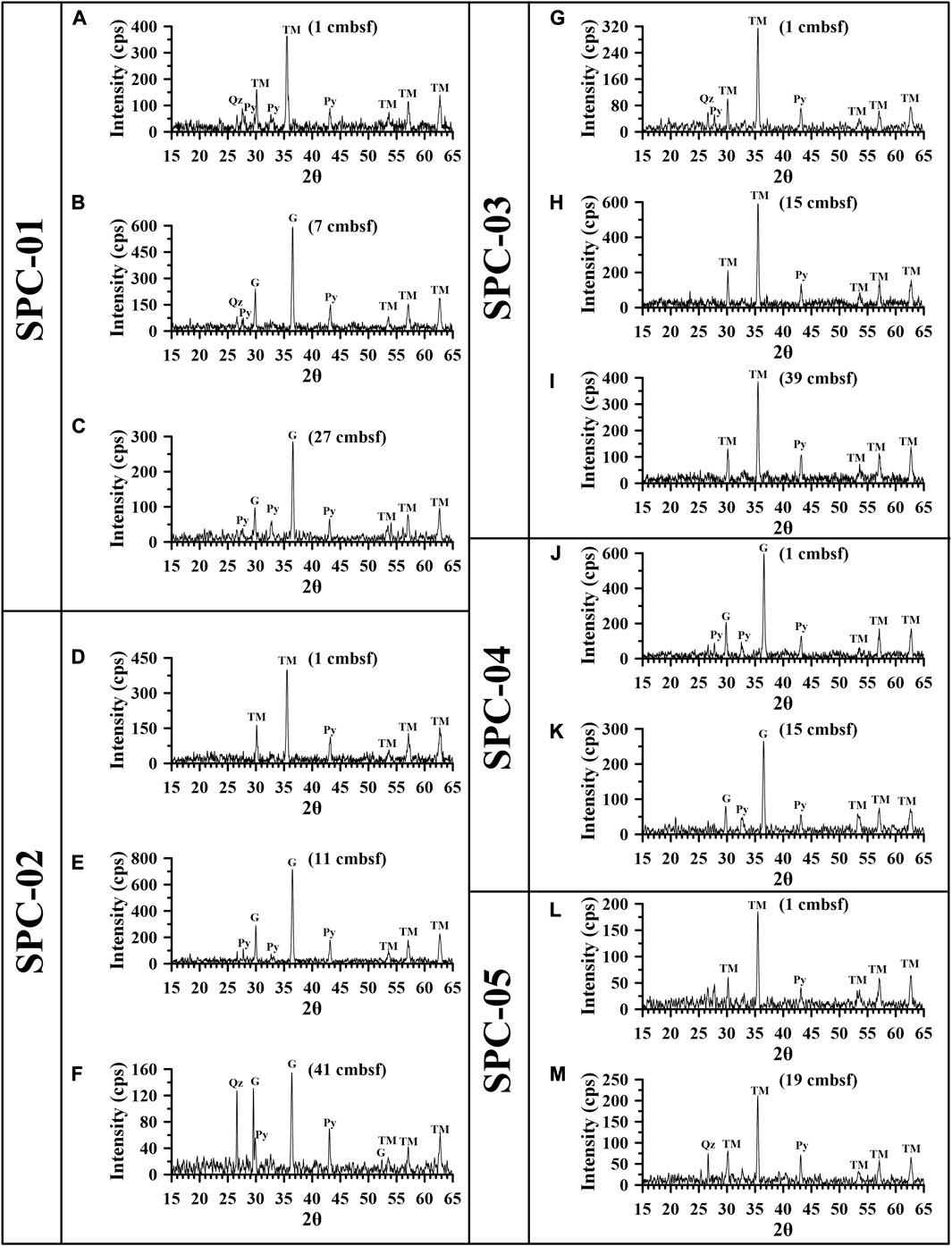
FIGURE 6. X-ray diffraction spectra for magnetic minerals extracted from different sediment core depth intervals of spade cores. (A–C): SPC-01, (D–F): SPC-02, (G–I): SPC-03, (J–K): SPC-04, and (L–M): SPC-05. TM, titanomagnetite; P, pyrite; Qz, quartz, and G, greigite.
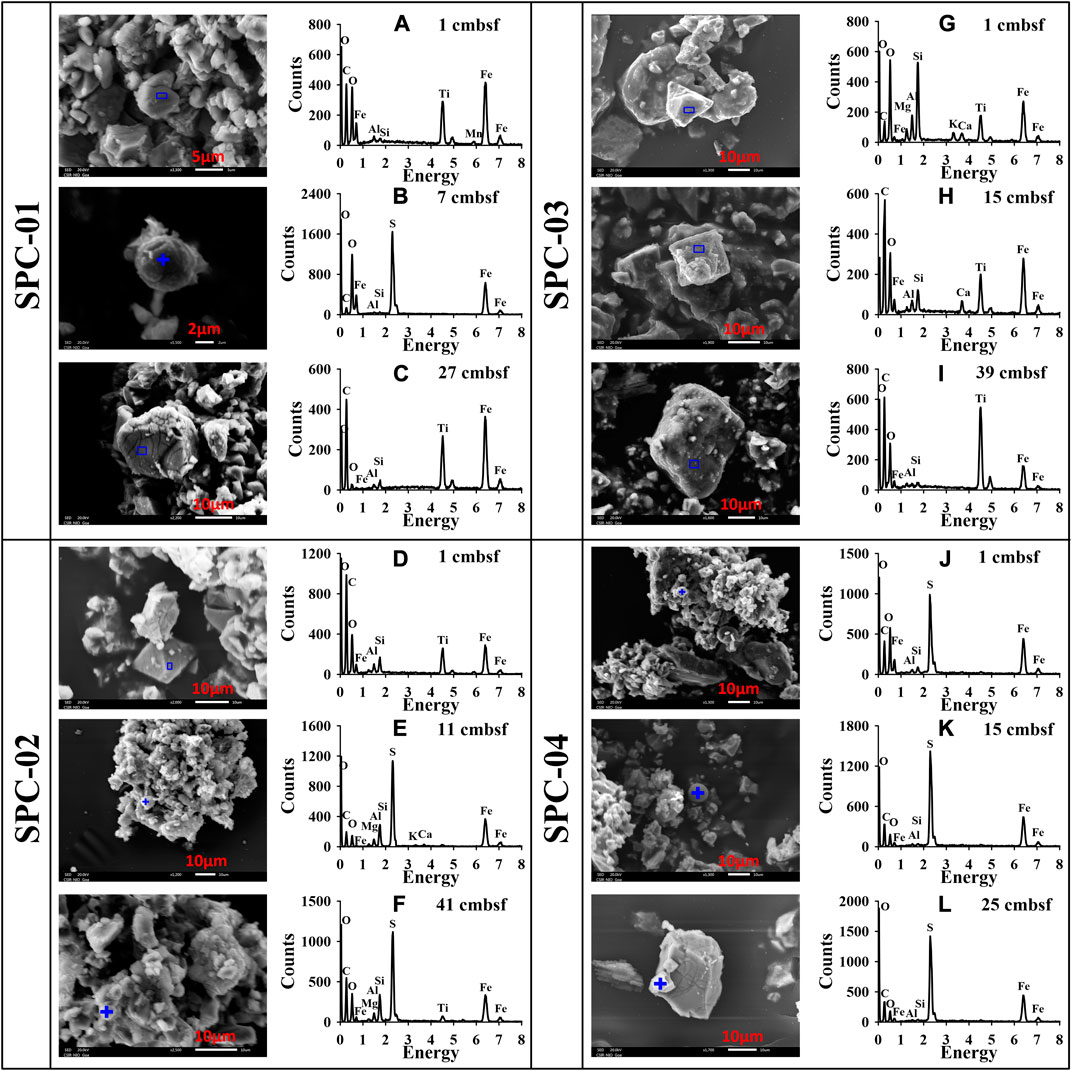
FIGURE 7. Scanning electron microscopy on magnetic extracts from different sediment core depth intervals. Energy dispersive x-ray spectroscopy spectra are placed adjacent to the respective images. (A–C): SPC-01, (D–F): SPC-02, (G–I): SPC-03, and (J–L): SPC-04. Iron (Fe), titanium (Ti), sulfur (S), oxygen (O), calcium (Ca), silicon (Si), carbon (C), aluminium (Al), potassium (K), magnesium (Mg), and manganese (Mn) peaks are indicated.
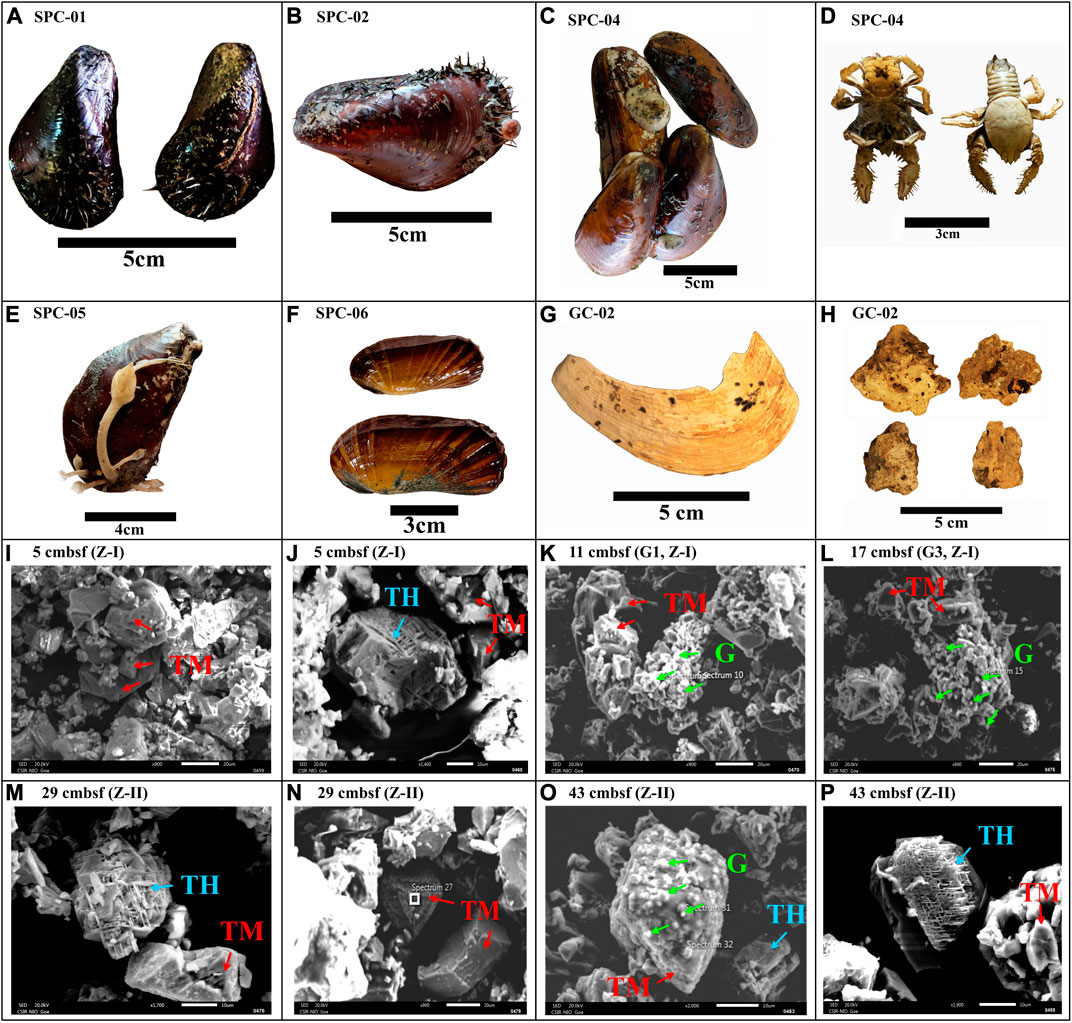
FIGURE 8. Photographs showing occurrence of (A,B,C,E): Bathymodiolus sp (D): Decapod crustaceans (squat lobsters) (F): Acharax sp. found at the spade core (surface) locations (G) calyptogena sp., shell fragment, and (H) authigenic carbonates found at 48 cmbsf in GC-02 location (I–P): Scanning electron microscopy on magnetic extracts from different sediment core depth intervals (Relict seep site: SSD-45/Stn-11/GC-02). TM, titanomagnetite; TH, titanohematite; and G, greigite).
Grain Size Distributions of Sediments, Chemosynthetic Communities and Authigenic Carbonates
Bulk sediment grain size in the studied cores mainly composed of silt and clay size fractions (Figure 9). Sand content is relatively low and varies between 0.99 and 18.58 vol%. Sand bearing sediment layers are observed throughout the core only in SPC-04/05/06 (Figures 9D–F). Higher magnetic susceptibility was found only in silt and clay size fractions (Figures 9A–F). Characteristic chemosynthetic living communities (Mazumdar et al., 2019) including Bathymodiolus sp., (Figures 8A–C,E), Acharax sp. shell (Figure 8F) and Decapod crustaceans (squat lobsters) belonging to the family Galatheidae and Munidopsidae (Figure 8D) are abundantly found at the active methane seep sites. At relict seep site (SSD-45/Stn-11/GC-02), light brown colored authigenic carbonates showing various morphologies and a non-living shell fragments (Calyptogena sp.) were found at 48 cmbsf (Z-II) in this core (Figures 8G,H).
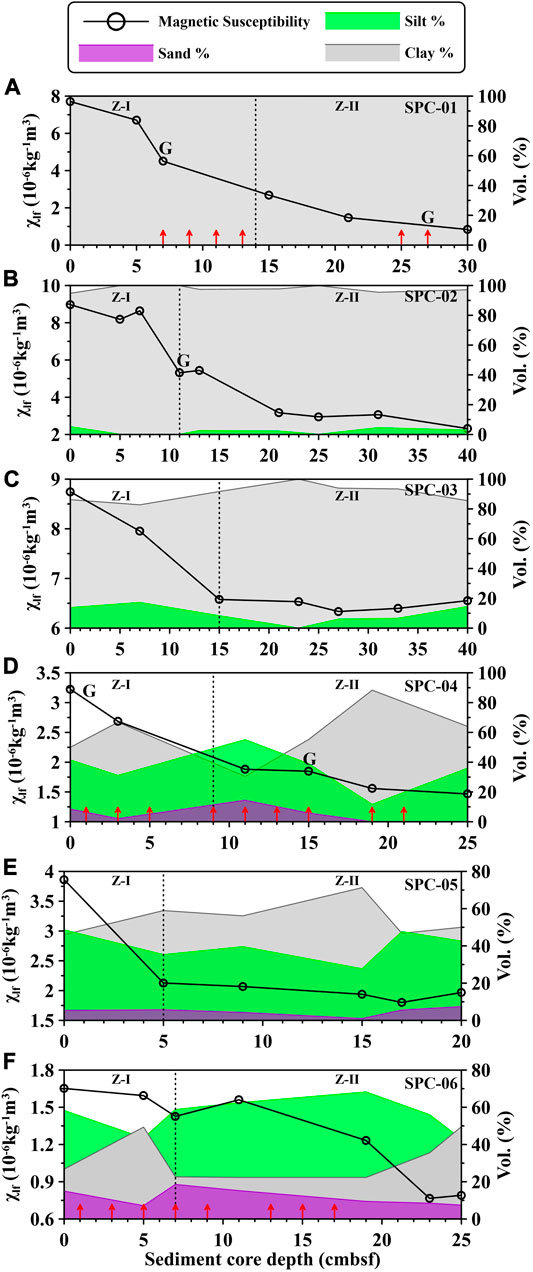
FIGURE 9. (A–F) Grain size distribution and magnetic susceptibility data of all spade (SPC-01 to SPC-06) sediment cores retrieved from active methane seep site SSD-45/Stn-4. Depths of carbonate occurrence are highlighted in solid red arrows (vertical). The depth of greigite occurrence is marked by “G”. Sediment magnetic zones (Z-I, Z-II) in each profile are marked.
Correlation Between Magnetic Parameters of Active and Relict Methane-Seep Sites
A clear distinction in terms of magnetic mineral concentration, grain size and mineralogy is seen between active and relict seep sites (Figure 10). A positive correlation between χlf and IRM1T is observed in all the samples, but showed different slopes which can be attributed to the presence of different magnetic mineralogies (Figure 10A). Contrastingly, anomalous lower values (0.11–0.55) of S-ratio and wide range of χlf displayed by samples from relict site indicates the predominance of highly coercive minerals (Figure 10C). Coupling between magnetic grain size (ARM/IRM1T) and physical grain size is noticed in majority of samples from active and relict seep sites (Figure 10D). We observed two populations (A and B). The samples from group-A showed uniformity in physical grain size, but exhibit wide range of magnetic grain size, while group-B samples follow equivalent trend and showed slight variation in magnetic and large scattering in physical grain size (Figure 10D).
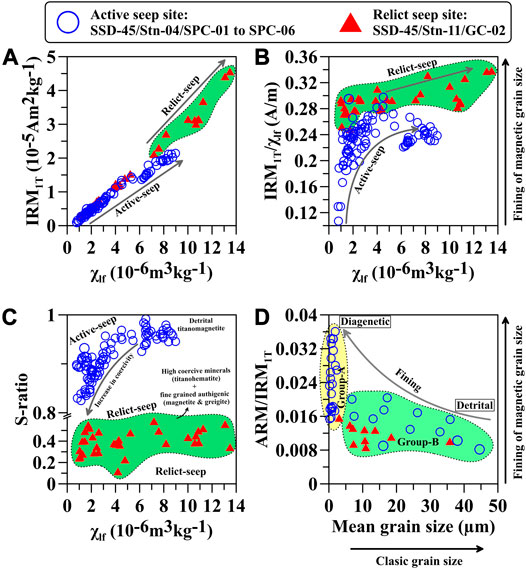
FIGURE 10. (A–D): Bivariate scatter plots of magnetic parameters magnetic susceptibility (χlf), saturation isothermal remanent magnetization (IRM1T), IRM1T/χ, S-ratio, ARM/IRM1T and mean grain size for spade cores (SPC-01 to SPC-06) from active (SSD-45/Stn-4) and gravity core (GC-02) from relict seep site (SSD-45/Stn-11) in the Krishna-Godavari (K-G) basin, Bay of Bengal. The gray arrows are used to highlight the trends in plots.
Discussion
Room, low and high temperature based rock magnetic data, FORC diagrams, XRD and SEM-EDS observations provide vital information about complex magnetic mineral assemblages in the studied cores. Data revealed that the sediment cores from active seep sites have undergone significant diagenetic dissolution in Z-I, while antigenically formed fine-grained magnetite and greigite is predominant in Z-II. Contrastingly, sulfitic (Z-I) and methanic (Z-II) zones of relict core are devoid of authigenic magnetite and mainly dominated by large amount of coarse-grained titanomagnetite and highly coercive (titanohematite) grains of detrital origin which most likely survived the diagenetic dissolution and remained preserved in these zones (Figures 8I–P). Here, we analyse these information to elucidate the controls of structural and diagenetic (early vs. late) processes on the sediment magnetic signals in an active/relict cold seep sites in the Bay of Bengal.
Magnetic Mineral Inventory of Sediments at Active/Relict Cold Seep Sites in the K-G Basin
Magnetic mineral assemblages in the sediment cores from active and relict seep sites from K-G basin, primarily consists of detrital (titanomagnetite, titanohematite), diagenetic (greigite/pyrite) and authigenic (magnetite) origin and are present in various proportions (Figures 2, 3, 5–7, 8I–P). Higher values of χlf in Z-I can be attributed to the presence of mixture of fine and coarse grained detrital titanomagnetite and titanohematite primarily sourced from Deccan trap basalts (Ramesh and Subramanian, 1988; Sangode et al., 2001; Badesab et al., 2019; Figures 2, 7, 8I–P). Higher values of grain size indicative (ARM/IRM1T) ratio (Maher, 1988) in Z-II of cores SPC-01/03/04/06, and GC-02 reveals high concentrations of fine SD/PSD magnetite (Figures 2C,O,U,A7,A13). ARM/IRM1T first decreases because of preferential dissolution of fine-grained magnetite in the upper part of the reduced zone (Z-I), and increases later in response to the authigenic formation of fine-grained authigenic magnetite (Rodelli et al., 2019; Lin et al., 2020) and greigite in deeper positions downcore (Z-II). Diagenetically formed magnetic iron sulfide (greigite) are also found to co-exist with detrital titanomagnetite grains and are spatially distributed in both sediment magnetic zones (Z-I and Z-II) of cores from active and relict seep sites (Figures 6, 7, 8I–P, 11). These can be explained based on the fact that few titanomagnetite grains might have survived the early diagenetic dissolution and got rapidly buried and remain preserved due to increased sedimentation.
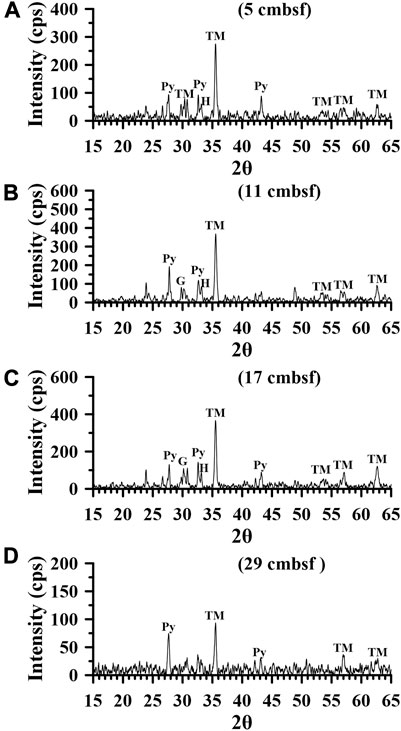
FIGURE 11. (A–D) X-ray diffraction spectra for magnetic minerals extracted from different sediment intervals of relict seep site: SSD-45/Stn-11/GC-02. TM, titanomagnetite; H, hematite; Py, pyrite; and G, greigite.
Verwey transition (Tv) temperatures has been successfully utilized as a diagnostic for discriminating detrital magnetite in sulfitic marine sedimentary systems. For example in Oman continental margin (Chang et al., 2016a), offshore of Western Australia (Heslop et al., 2013), and Ria de Vigo, Spain (Mohamed et al., 2011). Low-temperature magnetic data of SPC-01 and SPC-02 revealed the presence of magnetite (Figures 5A,B,D–F; Moskowitz et al., 1993; Özdemir et al., 1993; Verwey, 1947; Muxworthy and McClelland, 2000; Prozorov et al., 2007; Chang et al., 2013; Chang et al., 2014; Chang et al., 2016a; Chang et al., 2016b). At relict seep site (GC-02), magnetic mineralogy in the high χlf zone (Z-I) comprised of abundant detrital coarse-grained titanomagnetite and titanohematite particles (Figures 8I,J). Within sulfidic zone, minor peak of high χlf, χfd%, and IRM1T/χlf between 11 and 13 cmbsf reflect the diagenetic formation of greigite in this interval (Figures 2A11,A14,A16, 8K–L). Reduced methane flux due to either closure of underlying fractures/fault or cut-off in the supply of downward diffusing sulfate concentrations most likely interrupted the reductive diagenetic process and rather favored the formation and preservation of greigite by limiting H2S production at this site (Jørgensen et al., 2004; Neretin et al., 2004). Overgrowth of sub-micron sized particles on the larger titanomagnetite grain in Z-II is suggestive of authigenic growth of greigite probably due to weakening of anaerobic oxidation of methane (AOM) process in GC-02 (Figure 8O).
Magnetic granulometry (ARM/IRM1T, IRM1T/χlf; Snowball and Thompson, 1990; Roberts, 1995; Maher and Thompson, 1999; Sagnotti and Winkler, 1999; Nowaczyk et al., 2012) and mineralogy (S-ratio; Bloemendal et al., 1992) diagnostic parameters exhibits different trends in Z-1 and Z-II, respectively (Figure 2). A trend of down-core decrease of ARM/IRM1T in SPC-01/03/04/05/06 and GC-02 indicates the increase in abundance of coarser magnetic particles in Z-I (Figures 2C,O,U,A1,A7,A13). Loss of finer magnetic fraction in Z-I can be explained by selective diagenetic dissolution of fine-grained magnetic minerals due to their high reactivity and large surface area (Karlin and Levi, 1983; Rodelli et al., 2019; Tarduno, 1995). In contrast, core SPC-02 showed an opposite trend of down-core increase in ARM/IRM1T upto 11 cmbsf in Z-I (Figure 2I). Fining of magnetic particles in Z-I (Figure 2I) can be attributed to diagenetically induced fining mechanism (Chang et al., 2016b) or due to increased production of ultra-fine magnetic grains during magnetite reduction processes (Tarduno, 1995; Rodelli et al., 2019). The titanomagnetite grain in Z-II (Figures 7C,L) showed shrinkage-cracks typical of low-temperature maghemitization (Nowaczyk, 2011). The cracks on the grains indicate the areas where dissolution might have started. Hence, the fining mechanism in methanic Z-II can also be linked to the partial dissolution of large titanomagnetite grains (Nowaczyk, 2011). Down-core variations in χlf and IRM1T/χlf illustrate the spatial distribution of magnetic iron sulfides in the studied spade and gravity cores (Figure 2). Apparent linkage between high IRM1T/χlf, χfd%, and greigite occurrence was clearly observed for SPC-01/02/04 (Figures 2D,F,L,X,J,V, 6B,C,E,F,J,K, 7B,E,F,J,K,L). Several peaks of IRM1T/χlf and χfd%, predominantly in Z-I manifested by presence of non-uniformly distributed greigite (confirmed through SEM-EDS and XRD; Peters and Dekkers, 2003; Fu et al., 2008; Rowan et al., 2009; Figures 2, 6, 7) can be attributed to non-steady state magnetic mineral diagenesis driven by fluctuations in diffusive methane flux at these sites.
Variability in rock magnetic parameters in each core reflects the different degree of diagenetic disturbances created by methane seepage within the studied sites in the K-G basin. Similar observations were reported from Vestnesa Ridge, Svalbard margin (Sztybor and Rasmussen, 2017) and Oregon continental margin (Karlin, 1990). Variations in S-ratio can be attributed to the changes in magnetic mineralogy (Figures 2E,K,Q,W,A3,A9,A15). High S-ratio in Z-I is due to the presence of detrital titanomagnetite (Figures 2E,K,Q,W,A3,A9,A15, 6A,D,G,J,L, 7A,D,G). In spade cores (SPC-01 to SPC-06), we did not observe any signatures of higher coercivity (hematite) in SEM-EDS and XRD data (Figures 6, 7). In fact, XRD data confirmed the presence of greigite in samples exhibiting low S-ratio in both Z-I and Z-II (Figures 6B,C,E,F,K). The S-ratio drop in Z-II of relict core can be well-explained by the occurrence of numerous titanohematite grains (Figure 2A15, 8M,O,P). Preservation of titanomagnetite grains (Figures 8M–P) in Z-II could be either due to weakened AOM (Reidinger et al., 2005) or higher resistance against dissolution (Nowaczyk, 2011; Rodelli et al., 2019) which might have hindered the early diagenetic processes and led to the preservation of detrital magnetic particles. Significant drop in S-ratio (Figure 2A15) followed by high χfd% (Figure 2A16) in Z-II of relict core (SSD-45/Stn-11/GC-02) can be attributed to the presence of mixture of detrital titanohematite (Figures 8M,O,P), authigenic greigite (Dillon and Bleil, 2006; Larrasoaña et al., 2007; Rodelli et al., 2019) and hexagonal 3C pyrrhotite (Horng, 2018; Horng and Roberts, 2018). Similar observations were recently reported from Perseverance Drift, North-western Weddell Sea (Reilly et al., 2020).
Authigenically formed ferrimagnetic iron sulfides (greigite and pyrrhotite) are well-reported in methane-rich sedimentary system, for instance in Nankai Trough (Horng, 2018; Kars and Kodama, 2015), Hydrate Ridge (Larrasoaña et al., 2007), and offshore Taiwan (Horng and Roberts, 2018). FORC diagrams and low-temperature magnetic measurements provided additional constraints on the magnetic mineralogy and domain states. FORC distributions showed the mixture of vortex to multi-domain magnetic particles (Figures 4A–N). In natural system, greigite can commonly occur as magnetostatically interacting framboids. A micromagnetic modelling study involving hysteresis and FORC simulations demonstrated that magnetostatic interactions could remarkably alter the FORC response of greigite framboids and can form patterns similar to vortex and MD (Rodelli et al., 2018; Valdez-Grijalva et al., 2020). It is quite possible that greigite clusters observed in SPC-02 (Figures 7E,F) and SPC-04 (Figure 7J) might be contributing significantly towards the observed FORC (vortex to multi-domain) distributions. Low temperature magnetic data indicated the presence of magnetite in Z-I and Z-II (Figures 5A,B,D–F). Similar to our findings, Korff et al. (2016) reported cyclic magnetite dissolution and preservation of detrital magnetite in methanic sediments of the abyssal northwest Pacific Ocean. Sample from the zone of reduced magnetic susceptibility (Z-II) of SPC-01 did not show any indication of a Verwey transition in RT-SIRM, ZFC-FC, and the first derivative of magnetization curves (Figure 5C). We observed that nearly 62% of the LT-SIRM applied at 5 K is lost during warming between 5 and 30 K (Figure 5C). This observation indicates the presence of superparamagnetic (SP) magnetic particles in these samples (Tarduno, 1995; Passier and Dekkers, 2002). Similar to our observation, Kars and Kodama (2015) reported a related pattern of sudden decrease in LT-SIRM from 5 to ∼30 K and about ∼50–60% loss of the applied remanence in the sediment core samples from Nankai Trough, offshore Japan. They linked the loss of remanence to the presence of SP particles which get unblocked very rapidly. The SP magnetic particles in this sample (Figure 5C) is most likely iron sulfides like greigite, pyrrhotite, titanohematite (Horng, 2018; Rodelli et al., 2019; Roberts et al., 2020). This interpretation can be explained by the fact that fine-grained magnetic particles in this sample would get readily dissolved in the sulfitic environment (as seen through lowest χlf in Z-II). Contrastingly, the SP greigite and pyrrhotite nanoparticles are thermodynamically more stable compared to the SP magnetite nanoparticles and would rather remain preserved (Rowan et al., 2009; Roberts et al., 2018; Rodelli et al., 2018). Lack of LT transitions in the magnetization derivation curves in this sample (Figure 5C) is persistent and further confirms the presence of greigite (through high χfd%, Figure 2F) and pyrrhotite (Chang et al., 2009; Roberts et al., 2011).
Early Versus Late Diagenesis of Magnetic Minerals in Active and Relict Methane Seep Sites in the Bay of Bengal: Constraints From Rock Magnetism and Mineralogical Proxies
In active cold seep sedimentary system, temporal movement of diagenetic fronts due to variability in methane fluxes and sulfate reduction rates favours non-steady state diagenesis. Such process could generate complex magnetic signatures in sediments involving magnetite reduction and authigenic formation of magnetite (Amiel et al., 2020; Lin et al., 2020; Riedinger et al., 2014) and iron sulfides (Canfield and Berner, 1987; Larrasoaña et al., 2003; Liu et al., 2012; Riedinger et al., 2014; Roberts, 2015). Rock magnetic data showed progressive downcore dissolution of magnetite in sediment cores from active (Figures 2A–A10) and relict (Figures 2A11–A16; Karlin and Levi, 1983) sites in K-G basin. A gradual downcore decrease in ARM/IRM1T and χlf within Z-I in cores SPC-01/03/04/05/06 suggest substantial dissolution of fine-grained magnetic particles due to early diagenesis (Figures 2C,O,U,A1,A7; Rodelli et al., 2019). A slight increase in ARM/IRM1T in Z-II of SPC-01/03/04/06 (Figures 2C,O,U,A7) can be attributed to the presence of fine-grained authigenic magnetite (Karlin et al., 1987; Roberts, 2015; Roberts et al., 2018; Rodelli et al., 2019; Lin et al., 2020). A similar steady increase of ARM/IRM1T in sulfidic zone of a sediment cores from deep methanic zone of Southern Eastern Mediterranean continental shelf (Amiel et al., 2020) and Niger deep-sea fan was attributed to the gradual authigenic growth of SD magnetite (Dillon and Bleil, 2006). A downcore increase in ARM/IRM1T in Z-II of active seep sites (SPC-01/03/04/06) could also be due to presence of authigenic magnetite formed as a result of microbial iron reduction process in methanic sediments which might have permitted the newly formed magnetite to survive the sulfidic dissolution and favoured preservation (Rodelli et al., 2018; Rodelli et al., 2019; Lin et al., 2020; Figures 2C,O,U,A7). SEM-EDS and XRD data of cores showed the presence of greigite in methanic zone (Z-II) from active seep sites (Figures 6C,E,F,K, 7E,F,K,L). Following the concept put forward by Lin et al. (2020), we propose that following mechanism for authigenic greigite formation in Z-II of active seep sites. Increased supply of reactive iron (secondary iron oxyhydroxides) produced during iron-sulfide mineral oxidation due to decline in methane seepage intensities (Lin et al., 2016; Lin et al., 2020) and limitation of sulfide in methanic zone (below SMTZ) probably favoured the greigite formation in this zone.
During early diagenesis, sulfate gets readily depleted through decomposition of organic matter (Karlin, 1990a). We put forward the plausible mechanism for the preservation of fine-grained authigenic magnetite in Z-II as seen through a trend of high ARM/IRM1T in SPC-01/03/04/06 (Figure 2C,O,U,A7). Fine-grained magnetite (SD-type) could also occur as magnetic inclusions in silicates which most likely protected the magnetite from diagenetic dissolution (Hounslow and Morton 2004; Feinberg et al., 2005; Tarduno et al., 2006; Muxworthy and Evans, 2013; Chang et al., 2016a; Chang et al., 2016b) and could explain the preservation of fine-grained magnetite in Z-II. Another possibility is that authigenic magnetite formation coupled to microbial iron reduction (Lin et al., 2020) within methanic sediments might have allowed the freshly formed fine-grained magnetite to survive sulfidic dissolution fronts and rather favoured preservation as evident through high ARM/IRM1T values in Z-II (methanic) of cores SPC-01/03/04/06 from active seep sites. However, we do not rule out the possibility of presence of fine-grained titanomagnetite in Z-II. Similar findings were reported from rock magnetic studies on sediment cores from continental margin of Oman north-western Arabian Sea (Chang et al., 2016b) and northeast part of Japan Sea (Yamazaki et al., 2003). At relict site, an anomalous (39–57 cmbsf) interval within (Z-II) showed increased ARM/IRM1T values and significant drop in S-ratio (29–63 cmbsf) indicating the preservation of numerous highly coercive magnetic particles as confirmed through SEM observations (Figures 2A13,A15, 8M−P). We proposed that overgrowth of sub-micron sized authigenically formed SP greigite particles on the larger titanomagnetite grain (at 43 cmbsf, Figure 8O) might have contributed towards the observed increase in IRM1T/χlf (Figure 2A14). The presence of SP greigite is further confirmed by the observed increase in χfd% in Z-II of GC-02 (Figure 2A16).
Magnetic iron sulfides including greigite and pyrrhotite forms within the sulfidic pore water produced by upward migrating SMTZ fronts (Kasten et al., 1998; Horng, 2018). Significant downcore decrease in magnetite concentration (χlf) and grain size (ARM/IRM1T) diagnostic parameters in SPC-01/03/04/05/06 and GC-02 suggests that fine-grained magnetic particles were selectively removed by diagenetic dissolution (Figures 2A,C,M,O,S,U,Y,A1,A5,A7,A11,A13; Chang et al., 1987; Karlin et al., 1987; Roberts, 2015; Rodelli et al., 2019; Lin et al., 2020). Rock magnetic (Figure 2), SEM-EDS (Figures 7, 8I–P) observations, and mineralogical (Figures 6, 11) records provide evidence on the spatial distribution of diagenetically formed iron sulfides (greigite, pyrite) in Z-I and Z-II of all analyzed cores. A noticeable high IRM1T/χlf and χfd% values in samples from Z-I are exhibited by fine-grained greigite particles formed during early diagenesis (Figure 2). FORC diagrams for majority of samples provided evidences of two important magnetic (fine-grained, SD + SP) populations in Z-I and Z-II (Figures 4A–N). SP sized ultra-fine authigenically formed ferrimagnetic greigite particles (as seen through high χfd% and rapid decrease of ZFC and FC remanences upon warming from 5 to 30 K (Banerjee et al., 1993; Muxworthy and Roberts, 2007; Figures 2, 5C) and SD-type (as seen through negative regions in the horizontal axis, Sagnotti et al., 2005; Rowan and Roberts, 2006; Sagnotti et al., 2010; Roberts et al., 2011; Figures 4A–M) indicate the preservation of fine-grained SD magnetite particles.
Reduced magnetization at gas hydrate vents has been established in Cascadia accretionary margin off Vancouver Island (Novosel et al., 2015). At active seep sites, methane is being transported from deep-seated gas reservoir up to the seafloor and the intensities of methane flux governs the SMTZ location. Presence of abundant live chemosynthetic communities (Bathymodiolus sp. and Acharax sp.) provide evidences of active methane seepage at the studied site (Figures 8A–F). Elevated methane flux will shift the SMTZ fronts upward and vice-versa (Borowski et al., 1996). Production of hydrogen sulfide via AOM at SMTZ causes the dissolution of iron oxides, and precipitation of iron sulfides (Reidinger et al., 2014). Diagenetic dissolution of magnetite followed by the subsequent transformation to pyrite will create a distinct χlf minima compared to ferrimagnetic greigite which would significantly increase χlf (Dewangan et al., 2013; Kars and Kodama, 2015; Badesab et al., 2019). Several researchers established that variability in the SMTZ depth can be used to quantify methane flux (Housen and Musgrave, 1996; Neretin et al., 2004; Novosel et al., 2005; Reidinger et al., 2005; Musgrave et al., 2006; Larrasoaña et al., 2007; Roberts, 2015). A close linkage between the depth of SMTZ and minima in χlf has been well-established and increasingly utilized as a proxy for detecting SMTZ fronts in methanic sediments for example in Cascadia margin (Housen and Musgrave, 1996; Larrasoaña et al., 2007), offshore of Argentina and Uruguay (Riedinger et al., 2014), Nankai trough, Japan (Kars and Kodama, 2015), and offshore New Zealand (Rowan and Roberts, 2006). In the analyzed sediment cores, the distinct χlf minima most probably reflect recent SMTZ front, which varies between 5 cmbsf and 16 cmbsf (Figure 2). The shallowest χlf minima is noticed in active seep core SPC-05 (Figure 2Y) and deepest at SPC-03 (Figure 2M) and relict site GC-02 (Figure 2A11), respectively. Such variability in SMTZ depth provides evidences on the differential rate of diagenesis constrained by fluctuations in methane fluxes. In such non-steady state diagenetic scenario, for instance at active seep sites (SPC-01 to SPC-06), different degree of diagenetic disturbances created by changing methane flux could variably modify and preserve the primary magnetic mineral assemblages in sediments. SEM observations indicate the presence of abundant coarse-grained titanomagnetite and titanohematite in Z-I (Figures 7A,D,G, 8I–P) and Z-II (Figures 7C,I, 8M–P). More recently Amiel et al. (2020) conducted a detailed magnetic investigation on the sediment cores from SE Mediterranean Continental Shelf to examine the influence of early diagenesis in methanic environment. They reported that rapid sedimentation and non-steady state conditions triggered by variability in methane flux favoured the survival of abundant iron-oxide minerals in the sulfidic and deep methanic zone (Riedinger et al., 2014). In the present study, survival and preservation of these minerals in Z-I and Z-II can be explained by the fact that titanohematite and titanomagnetite are more stable and offers strong resistance to reductive dissolution induced by early and late diagenetic processes (Channell and Hawthorne, 1990; Poulton et al., 2004; Nowaczyk, 2011; Rodelli et al., 2019) or due to increased sedimentation (Riedinger et al., 2014; Amiel et al., 2020). Stability against diagenetic alteration increases together with elevated Ti content and, in turn, results in a higher magnetic stability. Dissolution of titanohematite involves a noticeably slower reaction compared to magnetite (Cornell and Schwertmann, 1996; Yamazaki et al., 2003; Liu et al., 2004; Rodelli et al., 2019). Previous studies have demonstrated that increasing titanium content stabilizes titanomagnetite compounds because Ti4+ substitution correspondingly reduces the number of Fe3+, the acting electron acceptor under anaerobic conditions (Karlin, 1990; Garming et al., 2005). During non-steady diagenesis, formation of secondary minerals takes places whenever pore-water chemistry changes due to upward or downward diffusion of dissolved hydrogen sulfide (Roberts and Weaver, 2005) and could lead to the formation and preservation of authigenic magnetite and greigite. Microbial iron reduction within methanic sediments is the most likely mechanism for authigenic magnetite formation (Rodelli et al., 2018; Lin et al., 2020).
More recently Reilly et al. (2020) and Rodelli et al. (2019) revealed that high coercivity magnetic minerals respond slowly to the diagenetic dissolution through entire SMTZ and can be a potential source for ferric iron via microbial iron reduction process coupled to AOM (Beal et al., 2009; Riedinger et al., 2014; Amiel et al., 2020). They further concluded that increased concentration of high coercivity minerals in methanic zone (below SMTZ) can produce large amount of ferric (Fe3+) via iron reduction where sulfide is limited and favours the authigenic precipitation of greigite. In the relict core (SSD-45/Stn-11/GC-02) greigite and titanohematite are found to occur throughout the sulfidic (Z-I) and methanic (Z-II) (Figures 2A11,A16, 8I–P, 11A–D). It is highly possible that diagenetic dissolution of titaohematites during iron reduction generated enough (Fe3+) and provided conducive environment for greigite formation in the intervals where sulfide got exhausted in core SSD-45/Stn-11/GC-02. Our SEM (Figures 8K,L,O) and XRD (Figures 11B,C) observations followed by high values of χfd% (Figure 2A16) support the findings of Reilly et al. (2020). Bivariate magnetic plots (Figure 10) and FORC diagrams (Figure 12) also helped to evaluate the influence of steady and non-steady state diagenetic processes on the magnetic record of sediment cores from active (SPC-01 to SPC-06), relict (Stn-11/GC-02) and paleo (MD161/Stn-8; Mazumdar et al., 2009) seep sites in the K-G basin. Scattered data plot between IRM1T/χlf and χlf reflects a broad range of SP greigite (Figures 2A16, 8K,L,O) particles and a larger variations in their concentrations (Figure 10B). A scattered plot between magnetic (ARM/IRM1T) and physical (mean) grain size showed a systematic pattern of fining in magnetic grain size with increase in diagenetic dissolution of detrital magnetic minerals (Figure 10D).
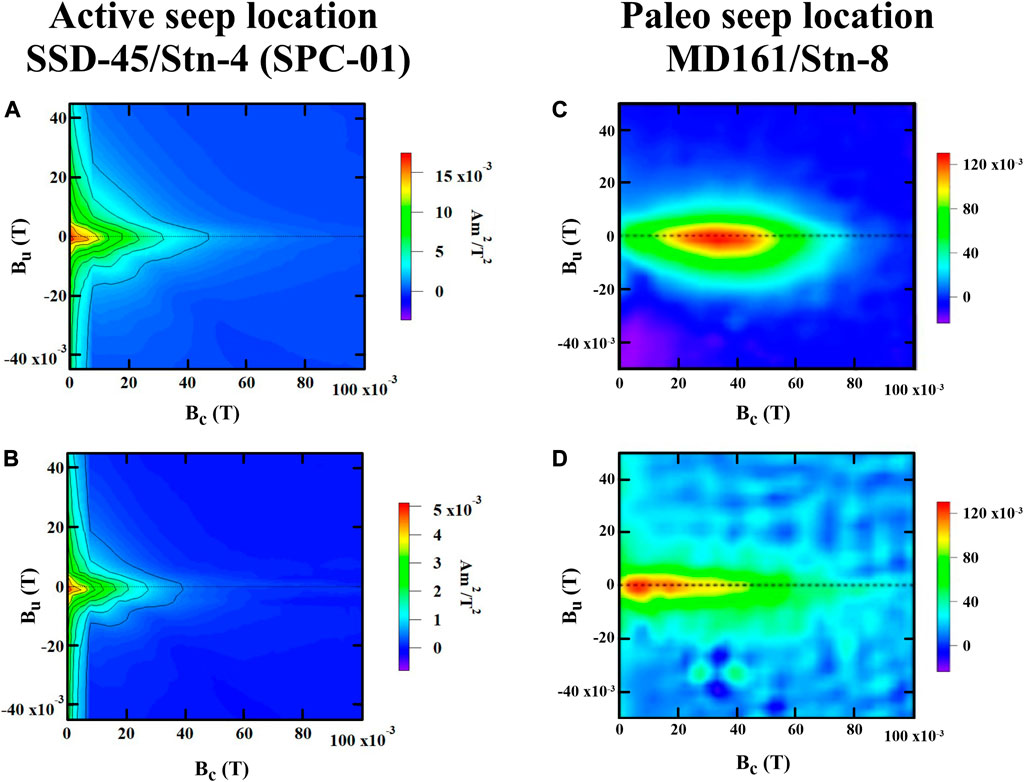
FIGURE 12. Comparative FORC diagrams of greigite bearing sediment intervals from (A,B): active (SSD-45/Stn-4/SPC-01) and (C,D) of paleo-seep location (MD161-Station-08; modified from Badesab et al., 2020a) in the Krishna-Godavari (K-G) basin, Bay of Bengal. The dashed (black) lines along Bc axis highlights the negative region on the horizontal axis.
Non-steady state diagenetic processes can potentially affect the sediment magnetic parameters. For example, abrupt change in the sedimentation rate can cause migration of the SMTZ (e.g., Fu et al., 2008; Riedinger et al., 2014), temporal changes in the organic matter load into the sediment can affect the depths of the different respiration processes (Larrasoaña et al., 2003; Rowan et al., 2009), changes in the sediment composition can lead to the accumulation of magnetic minerals in the sediment and change in the magnetic parameters. These mechanisms require time-variations in the flux of the sediments from Krishna and Godavari rivers, which are main source of detrital magnetic particles to the studied area. Multiple layers of mass transport deposits (MTD’s) generated as a result of frequently occurring high sedimentation events have been identified in the shallow and deep offshore regions of the K-G basin (Ramana et al., 2007; Ramprasad et al., 2011; Yamamoto et al., 2018). Recently Badesab et al. (2019) reported a high resolution sediment magnetic record of high sedimentation events in the K-G basin. Samples from relict site (SSD-45/Stn-11/GC-02) showed remarkably high χlf and IRM1T (Figures 2A11,A12) compared to active seep sites (SPC-01 to SPC-06) probably due to non-steady state diagenesis driven by high sedimentation events. It is most likely that coarse titanomagnetite and titanohematite grains survived diagenesis due to 1) its inherent property to resist the dissolution (Nowaczyk, 2011; Rodelli et al., 2019), and 2) rapid burial due to increased sedimentation (Badesab et al., 2019). Detailed rock magnetic and microscope analyses conducted on the sediment cores from continental margin of Oman and northern California by Rowan et al. (2009) reported that newly formed greigite particles are small initially and would display thermally unstable SP behaviour unless it grows fully through the stable SD blocking volume. They attributed the magnetic enhancement to the late diagenetic growth of magnetically stable greigite. Greigite can display both SD-type behaviour (Roberts, 1995) as well as ultrafine-grained SP (Rowan and Roberts, 2006; Rowan et al., 2009). Differences in the growth of greigite particles from active (SPC-01 to SPC-06) and paleo (MD161/Stn-8; Badesab et al., 2020a) samples is clearly reflected in the FORC diagrams (Figures 12A–D). FORC diagram of samples from active seep site (SPC-01) showed the magnetic particles exhibit a mixed (vortex state to multidomain) type behavior (Figures 12A,B; Roberts et al., 2017; Roberts et al., 2020). In contrast, FORC diagrams of paleo-seep samples showed broad distribution with concentric and elongated contour indicative of presence of magnetically stable greigite probably formed as a result of late diagenetic growth (Figure 12C; Rowan et al., 2009) and biogenic mineralization (Figure 12D; Rodelli et al., 2018; Badesab et al., 2020a) at site MD161/Stn-8.
Structural Control on the Formation and Preservation of Greigite
Methane venting episodes linked to active and paleo-cold seeps are well documented in the K-G basin (Mazumdar et al., 2009; Dewangan et al., 2020). High-resolution seismic data across active seep site (SSD-45/Stn-4; present study) showed the presence of well-developed seismic chimney and faults, which facilitated the migration of deep-seated gas through the gas hydrate stability zone (GHSZ) up to the sea floor and favored gas hydrate accumulation in the K-G basin (Dewangan et al., 2020; Figure 1B). At the same site, shallow (2–3 mbsf) gas hydrates were recovered during a gas hydrate exploration cruise in 2018 (Mazumdar et al., 2019). Vertical migration of methane through the sedimentary column produces multiple AOM’s resulting in the growth of diagenetic minerals including pyrite, greigite and pyrrhotite (Roberts, 2015). Interplay between the rates downward diffusing sulfate and upward migrating methane concentrations control the amount of hydrogen sulfide which is a key factor constraining the greigite formation (Kasten et al., 1998; Jørgensen et al., 2004; Neretin et al., 2004). For instance, enhanced methane flux would generate large amount of hydrogen sulfide which could drive pyritization process to complete resulting in the precipitation of pyrite, while limitation of sulfide due to decline in methane would favor greigite/pyrrhotite formation (Liu et al., 2004).
At active site, presence of greigite in both sediment magnetic zones of cores SPC-01/02/04 has been confirmed through SEM-EDS and XRD data (Figures 2, 6, 7). We try to investigate the structural control on the variability in methane fluxes and subsequent diagenetic formation of greigite in the studied cores. Control of opening and closing dynamics of the underlying fracture/fault system on methane migration in the K-G basin has been well-established (Dewangan et al., 2011; Dewangan et al., 2020). Spatial distribution of authigenic carbonates in cores SPC-01, SPC-04, SPC-06 (Figure 13) provide evidence on several AOM’s and is indicative of past fluctuations in methane fluxes. We put forward following plausible mechanisms to explain the formation and preservation of greigite in Z-I and Z-II: 1) decline in methane flux either due to massive hydrate accumulation (Mazumdar et al., 2019; Dewangan et al., 2020) or hindering of upward migrating fluid/gas by the carbonate layers in the subsurface sediments most likely resulted in low sulfide production and preferentially favoured formation and preservation of greigite in the Z-I. Similar observations were reported in fault-controlled cold seep-hydrate Woolsey Mound in the Northern Gulf of Mexico (Simonetti et al., 2013), gas hydrate field offshore Vancouver Island (Riedel et al., 2002), Vestnesa Ridge, W-Svalbard Margin (Singhroha et al., 2020), and in the K-G basin (Badesab et al., 2017; Dewangan et al., 2011). They explained that plugging of an active fault system due to massive hydrate formation can also cause significant drop in methane flux. 2) Occurrence of greigite below the carbonate layers in cores SPC-01, SPC-04, SPC-06 (Figure 13) in Z-II suggest that supply of downward diffusing sulfide is most likely hindered and subsequently a sulfide deficient zone is formed below the carbonate layer which inhibited the pyritization and rather favoured the formation and preservation of greigite. A detailed rock magnetic analysis of a 30 m long sediment core from paleo-seep site in the Bay of Bengal reported the presence of both diagenetic and biogenic greigite (within 17–23 mbsf) i.e., beneath a thick layer of authigenic carbonate formed as a result of intense methane seepage event (Mazumdar et al., 2009; Dewangan et al., 2013; Badesab et al., 2020a). More recent study has demonstrated that elevated supply of Fe2+ via iron reduction produced by high coercivity minerals in sulfide limited zone (Z-II) can also favour the authigenic growth of greigite (Rodelli et al., 2019; Reilly et al., 2020). Greigite presence in low S-ratio intervals of Z-II in relict core (SSD-45/Stn-11/GC-02) can also be linked with such process (Figures 2A15, 8O, 11C,D). The presence of greigite is further confirmed by the observed increase in χfd% (Figure 2A16). However additional geochemical and microbiological data are warranted to resolve the mechanism.
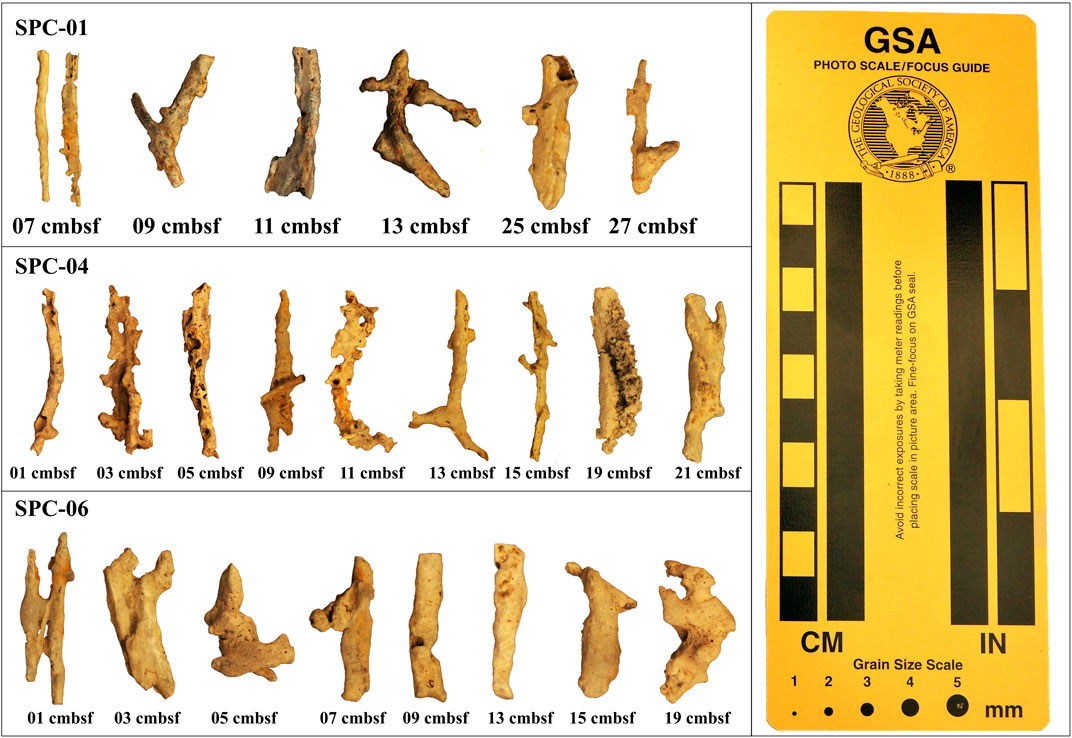
FIGURE 13. Photographs showing methane-derived authigenic carbonates found in an active seep sites SSD-45/Stn-04/SPC-01, SPC-04 and SPC-06. Respective depths of the carbonate occurrence are mentioned.
A conceptual model explaining the evolution of magnetic mineralogies at active (Stn-4/SPC-01 to SPC-06) and relict (Stn-11/GC-02) seep sites is developed (Figure 14). Shallow gas hydrates (2-3 mbsf) and authigenic carbonates (throughout the cores) were recovered at active seep sites (Figure 13; Mazumdar et al., 2019). In sulfidic (Z-I), detrital magnetic particles minerals supplied through Krishna and Godavari river systems reacted with the H2S produced by bacterial activity via organic matter decomposition and AOM-coupled sulfate reduction which led to the diagenetic dissolution of magnetic particles and subsequent precipitation of iron sulfide (pyrite) with intermediate precursors such as greigite and pyrrhotite (Berner, 1984; Karlin, 1990a). SEM-EDS and XRD data coupled with χfd% confirmed the presence of greigite in both sulfidic (Z-I) and methanic (Z-II) (Figures 2, 6, 7). Most likely mechanism which can explain the formation and preservation of greigite in Z-I and Z-II is as follows. Decline in methane flux due to massive hydrate accumulation along the active fault system and formation of authigenic carbonate crust in the sub-surface sediments might have hindered the supply of upward migrating fluid/gas and limited the sulfide production which preferentially enhanced greigite formation in Z-I. Secondly, restricted supply of downward diffusing sulfide by the carbonate layers in the uppermost sediments might be a responsible process for creating a sulfide deficient zone beneath. These might have inhibited the pyritization process and rather favoured the formation of greigite in methanic zone (Z-II). Samples from relict site (SSD-45/Stn-11/GC-02) showed notably high χlf and IRM1T (Figure 2A11,A12) compared to active seep sites which is most probably due to non-steady state diagenesis driven by high sedimentation events in the K-G basin. Coarse titanomagnetite and titanohematite grains might have survived diagenesis either due to its inherent property to resist the dissolution (Nowaczyk, 2011; Rodelli et al., 2019), or because of rapid burial due to increased sedimentation (Badesab et al., 2019).
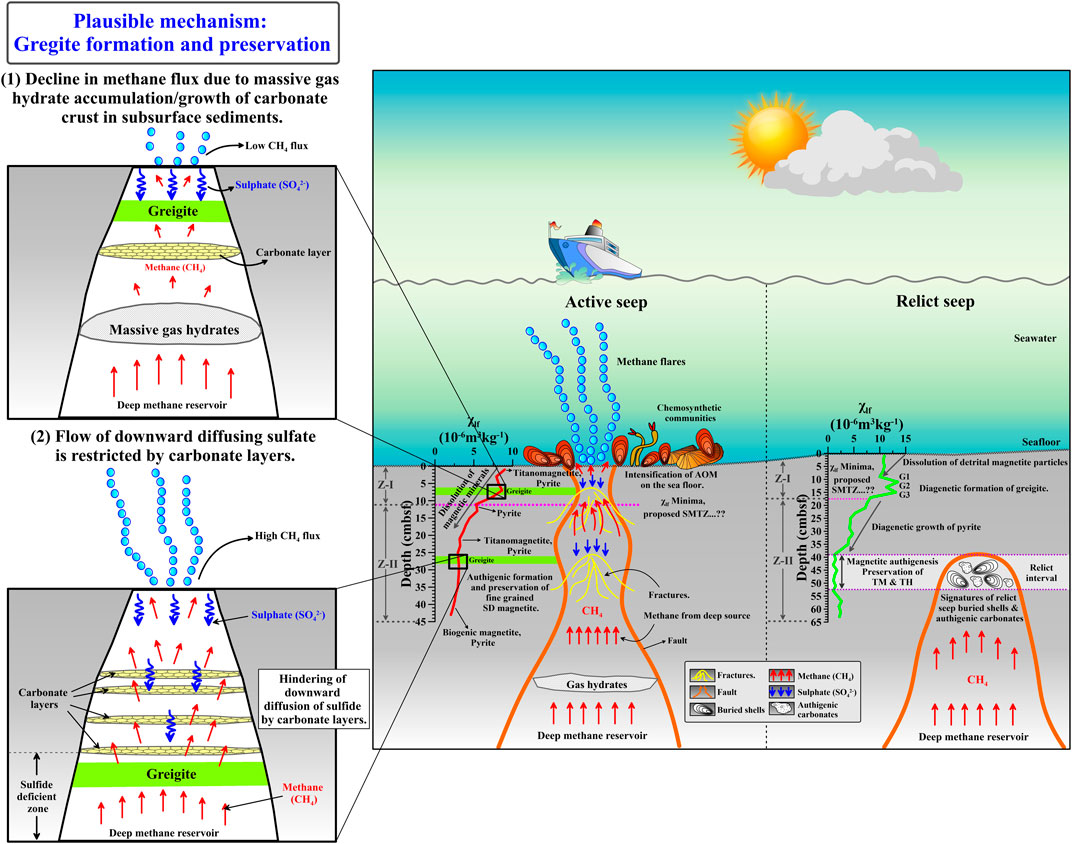
FIGURE 14. Conceptual model explaining the structural and diagenetic controls on the evolution of magnetic mineralogies at active and relict methane seep site in the Krishna-Godavari (K-G) basin, Bay of Bengal.
Conclusion
A set of rock magnetic, mineralogical and sedimentological records of the sediment cores were used to characterize the changes in the magnetic concentration, grain size, and mineralogy in a newly discovered active (Stn-4/SPC-01 to SPC-06) and relict (Stn-11/GC-02) cold seep sites in the K-G basin, Bay of Bengal. Sediment magnetism is mainly carried by complex magnetic mineral assemblages of detrital (titanomagnetite, titanohematite) and authigenic (magnetite, greigite) minerals. Topmost sulfidic sediment magnetic zone (Z-I) is marked by a higher concentration of magnetic minerals as evident in rock magnetic parameters. A systematic downcore decrease in χlf and IRM1T in this zone is due to the progressive diagenetic dissolution of iron oxide and subsequent precipitation of iron sulfides. Magnetogranulometric proxy (ARM/IRM1T) provided valuable insights on the preferential dissolution of fine-grained magnetite in the sulfidic zone (Z-I), and authigenic formation of magnetite in methanic zone (Z-II) of all studied cores. At relict site, elevated concentration of highly coercive magnetic (titanohematite) grains relative to soft minerals (as seen through lower S-ratio and χlf) which survived diagenesis are found in the methanic zone. A strong linkage between occurrence of authigenic carbonates and greigite formation and preservation is noticed. We summarized our observations into a conceptual model which provides insights on the evolution of different magnetic mineralogies constrained by variable diagenetic processes at active and relict seep sites in the K-G basin, Bay of Bengal.
Data Availability Statement
The datasets presented in this study can be found in online repositories. The names of the repository/repositories and accession number(s) can be found below: CSIR-NIO’s data repository (https://publication-data.nio.org/s/pb7ZQHybKm5DqDj).
Author Contributions
VG, FB, PD, and MK developed the concept for the manuscript. PD and VG have contributed towards sediment core collection and sampling during the research expedition (SSD-045) onboard R/V Sindhu Sadhana. Analyses were performed by VG, FB, and PD. All authors contributed equally to the writing and editing of the manuscript and preparation of figures and table.
Funding
This work is a contribution to the GAP-2303 research project “Studies on Gas Hydrate Exploration and Technology Development for its exploitation” supported by Ministry of Earth Sciences. Govt. of India. Research visit of FB to Center for Advanced Marine Core Research (CMCR), Kochi University, Japan for carrying our specialized rock magnetic analyses at Paleomagnetic Laboratory were supported by DST-JSPS under the scheme JSPS Postdoctoral Fellowship for Overseas Researchers (Strategic Program; JSPS grant GR 17202) and Establishment of Young Researcher Fellowship Program 2017–2018 (IA/Indo-Japanese/F-1/2018) scheme awarded to FB. We acknowledge funding support by SERB‐DST, Government of India, under the Early Career Research Award scheme (DST no. ECR/2016/000528) to FB.
Conflict of Interest
The authors declare that the research was conducted in the absence of any commercial or financial relationships that could be construed as a potential conflict of interest.
Acknowledgments
Director, CSIR–NIO, Secretary MOES, and National Gas Hydrate Program (NGHP) are thanked for supporting this study. We greatly acknowledge the support of Dr Aninda Mazumdar, participants of scientific cruise SSD-045 onboard research vessel R/V Sindhu Sadhana of CSIR-NIO, ship cell team and crews onboard for providing assistance in collection of sediment cores and sampling. Director of the Center for Advanced Marine Core Research (CMCR), Kochi University, Japan is thanked for allowing access to the Paleomagnetic laboratory during a research visit of FB as a part of INSA-JSPS fellowship. Myriam Kars is thanked for providing support to FB during the fellowship period at CMCR. High-end rock magnetic analyses at CMCR were supported by DST-JSPS under the scheme JSPS Postdoctoral Fellowship for Overseas Researchers (Strategic Program; JSPS grant GR 17202) and Establishment of Young Researcher Fellowship Program 2017–2018 (IA/Indo-Japanese/F-1/2018) scheme awarded to F. B. We thank Chisa Nishimori for providing laboratory and technical support in Kochi. Thanks to Areef Sardar, Girish Prabhu, Kaveri Salunke and Prem Piyush Panda for their support in carrying out mineralogical analyses at CSIR-NIO. We thank associate editor (Luigi Jovane) and all three reviewers for constructive comments that improved the paper. The data used in the present study are available on CSIR-NIO’s data repository (https://publication-data.nio.org/s/pb7ZQHybKm5DqDj). This is CSIR-NIO publication no. 6671.
References
Amiel, N., Shaar, R., and Sivan, O. (2020). The effect of early diagenesis in methanic sediments on sedimentary magnetic properties: case study from the SE mediterranean continental shelf. Front. Earth Sci. 8, 283. doi:10.3389/feart.2020.00283
Badesab, F., Dewangan, P., Gaikwad, V., Kars, M., Kocherla, M., Krishna, K. S., et al. (2019). Magnetic mineralogical approach for exploration of gas hydrates in the Bay of Bengal. J. Geophys. Res. Solid Earth. 24 (5), 4428–4451. doi:10.1029/2019JB017466
Badesab, F., Dewangan, P., and Gaikwad, V. (2020c). Magnetic mineral diagenesis in a newly discovered active cold seep site in the Bay of Bengal. Front. Earth Sci. doi:10.3389/feart.2020.592557
Badesab, F., Dewangan, P., Gaikwad, V., Sebastian, J. G., and Venkateshwarlu, M. (2020b). A rock magnetic perspective of gas hydrate occurrences in a high-energy depositional system in the Krishna-Godavari basin, Bay of Bengal. Geo-mar. Lett. 40. 1–15. doi:10.1007/s00367-020-00646-8
Badesab, F., Dewangan, P., Usapkar, A., Kocherla, M., Peketi, A., Mohite, K., et al. (2017). Controls on evolution of gas-hydrate system in the Krishna-Godavari basin, offshore India. Geochem. Geophys. Geosys. 18 (1), 52–74. doi:10.1002/2016GC006606
Badesab, F., Gaikwad, V., and Dewangan, P. (2020a). Controls on greigite preservation in a gas hydrate system of the Krishna-Godavari basin, Bay of Bengal. Geo-mar. Lett. 40 (4), 439–452. doi:10.1007/s00367-019-00604-z
B. A. Maher, and R. Thompson (1999). (editors) Quaternary climates, environments and magnetism. .Norwich,UK: (Cambridge University Press). doi:10.1017/CBO9780511535635
Banerjee, S. K., Hunt, C. P., and Liu, X. M. (1993). Separation of local signals from the regional paleomonsoon record of the Chinese Loess Plateau: a rock‐magnetic approach. Geophys. Res. Lett. 20 (9), 843–846. doi:10.1029/93GL00908
Bastia, R. (2007). Geologic settings and petroleum systems of India's east coast offshore basins: concepts and applications. Bangalore, India: Technology Publications.
Beal, E. J., House, C. H., and Orphan, V. J. (2009). Manganese- and iron-dependent marine methane oxidation. Science. 325 (5937), 184–187. doi:10.1126/science.1169984
Berndt, C., Feseker, T., Treude, T., Krastel, S., Liebetrau, V., Niemann, H., et al. (2014). Temporal constraints on hydrate-controlled methane seepage off Svalbard. Science. 343 (6168), 284–287. doi:10.1126/science.1246298
Besnus, M. J., and Meyer, A. J. (1964). Nouvelles données expérimentales sur le magnétisme de la pyrrhotine naturelle. Proc. Int. Conf. Mag. 20, 507–511.
Biksham, G., and Subramanian, V. (1988). Sediment transport of the Godavari River basin and its controlling factors. J.Hydrol. 101 (1-4), 275–290. doi:10.1016/0022‐1694(88)90040‐6
Bloemendal, J., King, J. W., Hall, F. R., and Doh, S. J. (1992). Rock magnetism of late Neogene and Pleistocene deep-sea sediments: relationship to sediment source, diagenetic processes, and sediment lithology. J. Geophys. Res. Solid Earth. 96, 4361–4375. doi:10.1029/91JB03068
Boetius, A., Ravenschlag, K., Schubert, C. J., Rickert, D., Widdel, F., Gieseke, A., et al. (2000). A marine microbial consortium apparently mediating anaerobic oxidation of methane. Nature. 407, 623–626. doi:10.1038/35036572
Bohrmann, G., Ivanov, M., Foucher, J. P., Spiess, V., Bialas, J., Greinert, J., et al. (2003). Mud volcanoes and gas hydrates in the Black Sea: new data from Dvurechenskii and Odessa mud volcanoes. Geo-mar. Lett. 23 (3-4), 239–249. doi:10.1007/s00367-003-0157-7
Borowski., W. S., Paull, C. K., and Ussler, W. I. I. I. (1996). Marine pore‐water sulfate profiles indicate in situ methane flux from underlying gas hydrate. Geology. 24 (7), 655–658. doi:10.1130/0091‐7613(1996)024<0655:MPWSPI>2.3.CO;2
Bünz, S., Polyanov, S., Vadakkepuliyambatta, S., Consolaro, C., and Mienert, J. (2012). Active gas venting through hydrate‐bearing sediments the vastness ridge. Offshore W‐svalbard. Mar. Geol. 332–334, 189–197. doi:10.1016/j.margeo.2012.09.012
Canfield, D. E., and Berner, R. A. (1987). Dissolution and pyritization of magnetite in anoxie marine sediments. Geochim. Cosmochim. Acta 51 (3), 645–659. doi:10.1016/0016‐7037(87)90076‐7
Chang, L., Heslop, D., Roberts, A. P., Rey, D., and Mohamed, K. J. (2016b). Discrimination of biogenic and detrital magnetite through a doubleVerwey transition temperature. J. Geophy. Res. Solid Earth 121 (1), 3–14. doi:10.1002/2015JB012485
Chang, L., Roberts, A. P., Heslop, D., Hayashida, A., Li, J., Zhao, X., et al. (2016a). Widespread occurrence of silicate-hosted magneticmineral inclusions in marine sediments and their contribution topaleomagnetic recording. J. Geophys. Res. Solid Earth 121 (12), 8415–8431. doi:10.1002/2016JB013109
Chang, L., Roberts, A. P., Rowan, C. J., Tang, Y., Pruner, P., Chen, Q., et al. (2009). Low-temperature magnetic properties of greigite (Fe3S4). Geochem. Geophy. Geosy. 10, Q01Y04. doi:10.1029/2008GC002276
Chang, L., Roberts, A. P., Winklhofer, M., Heslop, D., Dekkers, M. J., Krijgsman, W., et al. (2014). Magnetic detection and characterization of biogenic magnetic minerals: a comparison of ferromagneticresonance and first-order reversal curve diagrams. J. Geophys. Res. Solid Earth 119 (8), 6136–6158. doi:10.1002/2014JB011213
Chang, L., Winklhofer, M., Roberts, A. P., Heslop, D., Florindo, F., Dekkers, M. J., et al. (2013). Low‐temperature magnetic properties of pelagic carbonates: oxidation of biogenic magnetite and identification of magnetosome chains. J. Geophys. Res. Solid Earth. 118 (12), 6049–6065. doi:10.1002/2013JB010381
Chang, S. B. R., Kirschvink, J. L., and Stolz, J. F. (1987). Biogenic magnetite as a primary remanence carrier in limestone deposits. Phys. Earth Planet. Inter. 46, 289–303. doi:10.1016/0031-9201(87)90191-9
Channell, J. E. T., and Hawthorne, T. (1990). Progressive dissolution of titanomagnetite at ODP site 653 (tyrrhenian sea). Earth Planet. Sci. Lett. 96 (3‐4), 469–480. doi:10.1016/0012‐821X(90)90021‐O
Collett, T., Riedel, M., Cochran, J. R., Boswell, R., Presley, J., Kumar, P., et al. (2008). the NGHP Expedition 01 ScientistsInitial report, Directorate General of HydrocarbonsIndian national gas hydrate Program, expedition ‐ 01. India: Ministry of Petroleum and Natural gas. doi:10.3133/sir20125054
Cornell, R. M., and Schwertmann, U. (1996). The iron oxides. Switzerland, VCH. doi:10.1002/3527602097
Crémièrea, A., Chanda, S., Sahyc, D., Thorsnesa, T., Martmad, T., Noblec, S. R., et al. (2018). Structural controls on seepage of thermogenic and microbial methane sincere last glacial maximum in the harstad basin, southwest Barents Sea. Mar. Petrol. Geol. 98, 569–658. doi:10.1016/j.marpetgeo.2018.07.010
Dekkers, M. J. (1989). Magnetic properties of natural pyrrhotite. II. High‐ and low‐temperature behaviour of Jrs and TRM as function ofgrain size. Phys. Earth Planet. Inter. 57, 266–283. doi:10.1016/0031‐9201(89)90116‐7
Dewangan, P., Basavaiah, N., Badesab, F. K., Usapkar, A., Mazumdar, A., Joshi, R., et al. (2013). Diagenesis of magneticminerals in a gas hydrate/cold seep environment off the Krishna–Godavari basin, Bay of Bengal. Mar. Geol. 340, 57–70. doi:10.1016/j.margeo.2013.04.016
Dewangan, P., Ramprasad, T., Ramana, M. V., Mazumdar, A., Desa, M., and Badesab, F. K. (2010). Seabed morphology and gas venting features in the continental slope region of Krishna‐Godavari basin, Bay of Bengal: implications in gas‐hydrate exploration. Mar. Petrol. Geol. 27 (7), 1628–1641. doi:10.1016/j.marpetgeo.2010.03.015
Dewangan, P., Sriram, G., Kumar, A., Mazumdar, A., Peketi, A., Mahale, V., et al. (2020). Widespread occurrence of methane seeps in deep-water regions of Krishna-Godavari basin, Bay of Bengal. Mar. Petrol. Geol. 124. 104783. doi:10.1016/j.marpetgeo.2020.104783
Dewangan, P., Sriram, G., Ramprasad, T., Ramana, M. V., and Jaiswal, P. (2011). Fault system and thermal regime in the vicinity of siteNGHP‐01‐10, Krishna–Godavari basin, Bay of Bengal. Mar. Petrol. Geol. 28, 1899–1914. doi:10.1016/j.marpetgeo.2011.03.009
Dillon, M., and Bleil, U. (2006). Rock magnetic signatures in diagenetically altered sediments from the niger deep‐sea fan. J. Geophys. Res. Solid Earth. 111 (B3). doi:10.1029/2004JB003540
Dunlop, D. J., Özdemir, Ö., and Schmidt, P. W. (1997). Paleomagnetism and paleothermometry of the Sydney Basin 2. Origin of anomalously high unblocking temperatures. J. Geophys. Res. Solid Earth 102 (B12), 27285–27295. doi:10.1029/97JB02478
Feinberg, J. M., Scott, G. R., Renne, P. R., and Wenk, H. R. (2005). Exsolved magnetite inclusions in silicates: features determining their remanence behavior. Geology. 33 (6), 513–516. doi:10.1130/G21290.1
Frank, U., and Nowaczyk, N. R. (2008). Mineral magnetic properties of artificial samples systematically mixed from haematite and magnetite. Geophy. J. Inter. 175 (2), 449–461. doi:10.1111/j.1365-246X.2008.03821.x
Fu, Y., Von Dobeneck, T., Franke, C., Heslop, D., and Kasten, S. (2008). Rock magnetic identification and geochemical process models of greigite formation in quaternary marine sediments from the Gulf of Mexico (IODP Hole U1319A). Earth Planet. Sci. Lett. 275 (3-4), 233–245. doi:10.1016/j.epsl.2008.07.034
Garming, J. F. L., Bleil, U., and Riedinger, N. (2005). Alteration of magnetic mineralogy at the sulfate–methane transition: analysis of sediments from the argentine continental slope. Phys. Earth Planet. Inter. 151 (3‐4), 290–308. doi:10.1016/j.pepi.2005.04.001
Geersen, J., Scholz, F., Linke, P., Schmidt, M., Lange, D., Behrmann, J. H., et al. (2016). Fault zone controlled seafloor methane seepage in the rupture area of the 2010 maule earthquake, central chile. Geochem. Geophy., Geosys. 17 (11), 4802–4813. doi:10.1002/2016GC006498
Gullapalli, S., Dewangan, P., Kumar, A., Dakara, G., and Mishra, C. K. (2019). Seismic evidence of free gas migration through the gas hydrate stability zone (GHSZ) and active methane seep in Krishna-Godavari offshore basin. Mar. Petrol. Geol. 110, 695–705. doi:10.1016/j.marpetgeo.2019.07.052
Harrison, R. J., and Feinberg, J. M. (2008). FORCinel: an improved algorithm for calculating first order reversal curve distributions using locally weighted regression smoothing. Geochem. Geophy. Geosys. 9, Q05016. doi:10.1029/2008GC001987
Henry, P., Lallemant, S., Nakamura, K. I., Tsunogai, U., Mazzotti, S., and Kobayashi, K. (2002). Surface expression of fluid venting at the toe of the Nankai wedge and implications for flow paths. Mar. Geol. 187 (1-2), 119–143. doi:10.1016/S0025-3227(02)00262-1
Heslop, D., Roberts, A. P., Chang, L., Davies, M., Abrajevitch, A., and Deckker, P. (2013). Quantifying magnetite magnetofossil contributions to sedimentary magnetizations. Earth Planet. Sci. Lett. 382, 58–65. doi:10.1016/j.epsl.2013.09.011
Hirt, A. M., and Gehring, A. U. (1991). Thermal alteration of the magnetic mineralogy in ferruginous rocks. J. Geophys. Res. Solid Earth 96 (B6), 9947–9953. doi:10.1029/91JB00573
Horng, C. S., and Chen, K. H. (2006). Complicated magnetic mineral assemblages in marine sediments offshore southwestern Taiwan: possible influences of methane flux on the early diagenetic process. Terr., Atmos. Ocean. Sci. 17, 1009–1026. doi:10.3319/TAO.2006.17.4.1009
Horng, C. S., and Roberts, A. P. (2018). The low‐temperature Besnus magnetic transition: signals due to monoclinic and hexagonal pyrrhotite. Geochem. Geophy., Geosys. 19, 3364–3375. doi:10.1029/2017GC007394
Horng, C. S. (2018). Unusual magnetic properties of sedimentary pyrrhotite in methane seepage sediments: comparison with metamorphic pyrrhotite and sedimentary greigite. J. Geophys. Res. Solid Earth 123 (6), 4601–4617. doi:10.1002/2017JB015262
Hounslow, M. W., and Morton, A. C. (2004). Evaluation of sediment provenance using magnetic mineral inclusions in clastic silicates: comparison with heavy mineral analysis. Sediment. Geology. 171 (1-4), 13–36. doi:10.1016/j.sedgeo.2004.05.008
Housen, B. A., and Musgrave, R. J. (1996). Rock‐magnetic signature of gas hydrates in accretionary prism sediments. Earth Planet. Sci. Lett. 139 (3‐4), 509–519. doi:10.1016/0012‐821X(95)00245‐8
Jørgensen, B. B., Böttcher, M. E., Lüschen, H., Neretin, L. N., and Volkov, I. I. (2004). Anaerobic methane oxidation and a deep H2S sink generate isotopically heavy sulfides in Black Sea sediments. Geochim. Cosmochim. Acta. 68 (9), 2095–2118. doi:10.1016/j.gca.2003.07.017
Karlin, R., and Levi, S. (1983). Diagenesis of magnetic minerals in recent hemipelagic sediments. Nature 303 (5915), 327. doi:10.1038/303327a0
Karlin, R., Lyle, M., and Heath, G. R. (1987). Authigenic magnetite formation in suboxic marine sediments. Nature 326 (6112), 490–493. doi:10.1038/326490a0
Karlin, R. (1990). Magnetic mineral diagenesis in suboxic sediments at bettis site W‐N, NE Pacific Ocean. J. Geophys. Res. Solid Earth 95 (B4), 4421–4436. doi:10.1029/JB095iB04p04421
Karlin, R. (1990a). Magnetite diagenesis in marine sediments from the Oregon continental margin. J. Geophys. Res. Solid Earth 95 (B4), 4405–4419. doi:10.1029/JB095iB04p04405
Kars, M., and Kodama, K. (2015). Autogenesis of magnetic minerals in gas hydrate bearing sediments in the nankai trough. Offshore Japan Geochem. Geophy. Geosys. 16, 947–961. doi:10.1002/2014GC005614
Kasten, S., Freudenthal, T., Gingele, F. X., and Schulz, H. D. (1998). Simultaneous formation of iron‐rich layers at different redox boundaries sediments of the Amazon deep‐sea fan. Geochim. Cosmochim. Acta. 62, 2253–2264. doi:10.1016/S0016‐7037(98)00093‐3
Knittel, K., and Boetius, A. (2009). Anaerobic oxidation of methane: progress with an unknown process. Annu. Rev. Microbiol. 63, 311–334. doi:10.1038/296643a010.1146/annurev.micro.61.080706.093130
Korff, L., von Dobeneck, T., Frederichs, T., Kasten, S., Kuhn, G., Gersonde, R., et al. (2016). Cyclic magnetite dissolution in pleistocene sediments of the abyssal northwest Pacific Ocean: evidence for glacial oxygen depletion and carbon trapping. Paleoceanography 31 (5), 600–624. doi:10.1002/2015PA002882
Kumar, P., Collett, T. S., Boswell, R., Cochran, J. R., Lall, M., Mazumdar, A., et al. (2014). Geologic implications of gas hydrates in theoffshore of India: krishna‐godavari basin, mahanadi basin, andaman sea, Kerala–konkan basin. Mar. Petrol. Geol. 58, 29–98. doi:10.1016/j.marpetgeo.2014.07.031
Larrasoaña, J. C., Roberts, A. P., Musgrave, R. J., Gràcia, E., Piñero, E., Vega, M., et al. (2007). Diagenetic formation ofgreigite and pyrrhotite in gas hydrate marine sedimentary systems. Earth Planet. Sci. Lett. 261 (3‐4), 350–366. doi:10.1016/j.epsl.2007.06.032
Larrasoaña, J. C., Roberts, A. P., Stoner, J. S., Richter, C., and Wehausen, R. (2003). A new proxy for bottom-water ventilation in the eastern mediterranean based on diagenetically controlled magnetic properties of sapropel-bearing sediments. Palaeogeogr. Palaeoclimatol. Palaeoecol. 190, 221–242. doi:10.1016/S0031-0182(02)00607-7
Lascu, I., Einsle, J. F., Ball, M. R., and Harrison, R. J. (2018). The vortex state in geologicmaterials: a micromagnetic perspective. J. Geophys. Res. Solid Earth 123, 7285–7304. doi:10.1029/2018JB015909
Lattard, D., Engelmann, R., Kontny, A., and Sauerzapf, U. (2006). Curie temperatures of synthetic titanomagnetite in the Fe‐Ti‐O system: effects of composition, crystal chemistry, and thermomagnetic methods. J. Geophy. Res. Solid Earth 111 (B12), S28. doi:10.1029/2006JB004591
Lin, Q., Wang, J., Algeo, T. J., Su, P., and Hu, G. (2016). Formation mechanism of authigenic gypsum in marine methane hydrate settings: evidence from the northern South China Sea. Deep-Sea Res. Oceanographic Res. Pap. 115, 210–220. doi:10.1016/j.dsr.2016.06.010
Lin, Z., Sun, X., Lu, Y., Strauss, H., Xu, L., Gong, J., et al. (2017). The enrichment of heavy iron isotopes in authigenic pyrite as a possible indicator of sulfate-driven anaerobic oxidation of methane: insights from the South China Sea. Chem. Geol. 449, 15–29. doi:10.1016/j.chemgeo.2016.11.032
Lin, Z., Sun, X., Roberts, A. P., Strauss, H., Lu, Y., Yang, X., et al. (2020). A novel authigenic magnetite source for sedimentary magnetization. Geology. 49. doi:10.1130/G48069.1
Lin, Z., Sun, X., Strauss, H., Lu, Y., Böttcher, M. E., Teichert, B. M., et al. (2018). Multiple sulfur isotopic evidence for the origin of elemental sulfur in an iron-dominated gas hydrate-bearing sedimentary environment. Mar. Geol. 403, 271–284. doi:10.1016/j.margeo.2018.06.010
Liu, Q., Jackson, M. J., Yu, Y., Chen, F., Deng, C., and Zhu, R. (2004). Grain size distribution of pedogenic magnetic particles in Chinese loess/paleosols. Geophy. Res. Lett. 31 (22). doi:10.1029/2004GL021090
Liu, Q., Roberts, A. P., Larrasoaa, J. C., Banerjee, S. K., Guyodo, Y., Tauxe, L., et al. (2012). Environmental magnetism: principles and applications. Rev. Geophys. 50, RG4002. doi:10.1029/2012RG000393
Maher, B. A. (1988). Magnetic properties of some synthetic sub-micron magnetites. Geophy. J. Inter. 94 (1), 83–96. doi:10.1111/j.1365-246X.1988.tb03429.x
Mazumdar, A., Dewangan, P., Joäo, H. M., Peketi, A., Khosla, V. R., Kocherla, M., et al. (2009). Evidence of paleo–cold seep activity from theBay of Bengal, offshore India. Geochem. Geophy., Geosys., 10 (6), 10Q06005. doi:10.1029/2008GC002337
Mazumdar, A., Dewangan, P., Peketi, A., Gullapalli, S., Kalpana, M. S., Naik, G. P., et al. (2019). The first record of active methane (cold) seep ecosystem associated with shallow methane hydrate from the Indian EEZ. J. Earth Sys. Sci. 128, 18. doi:10.1007/s12040-018-1044-y
Mohamed, K. J., Rey, D., Rubio, B., Dekkers, M. J., Roberts, A. P., and Vilas, F. (2011). Onshore–offshore gradient in reductive early diagenesis in coastal marine sediments of the Ria de Vigo, Northwest Iberian Peninsula. Cont. Shelf Res. 31 (5), 433–447. doi:10.1016/j.csr.2010.06.006
Moskowitz, B. M., Frankel, R. B., and Bazylinski, D. A. (1993). Rock magnetic criteria for the detection of biogenic magnetite. Earth Planet. Sci. Lett. 120 (3‐4), 283–300. doi:10.1016/0012‐821X(93)90245‐5
Musgrave, R. J., Bangs, N. L., Larrasoaña, J. C., Gràcia, E., Hollamby, J. A., and Vega, M. E. (2006). Rise of the base of the gas hydrate zonesince the last glacial recorded by rock magnetism. Geology. 34 (2), 117–120. doi:10.1130/G22008.1
Muxworthy, A. R., and Evans, M. E. (2013). Micromagnetics and magnetomineralogy of ultrafine magnetite inclusions in the Modipe Gabbro. Geochem. Geophy. Geosys. 14 (4), 921–928. doi:10.1029/2012GC004445
Muxworthy, A. R., and McClelland, E. (2000). Review of the low‐temperature magnetic properties of magnetite from a rock magnetic perspective. Geophy. J. Inter. 140 (1), 101–114. doi:10.1046/j.1365‐246x.2000.00999.x
Muxworthy, A. R., and Roberts, A. P. (2007). “First-order reversal curve (FORC) diagrams,” in Encycl. Geomag. Paleomag.. Editors D. Gubbins, and E. Herrero-Bervera (New York, NY: Springer). 266–272. doi:10.1016/j.jmmm.2018.09.086
Neretin, L. N., Böttcher, M. E., Jørgensen, B. B., Volkov, I. I., Lüschen, H., and Hilgenfeldt, K. (2004). Pyritization processes and greigiteformation in the advancing sulfidization front in the upper pleistocene sediments of the Black Sea 1. Geochim. Cosmochim. Acta. 68 (9), 2081–2093. doi:10.1016/S0016‐7037(03)00450‐2
Novosel, I., Spence, G. D., and Hyndman, R. D. (2005). Reduced magnetization produced by increased methane flux at a gas hydrate vent. Mar. Geol. 216 (4), 265–274. doi:10.1016/j.margeo.2005.02.027
Novosel, N., Galić, S., Pajić, D., Zadro, K., and Babić, E. (2015). Enhancing superconducting properties of MgB 2 by addition of magnetic particles. J. Supercond.novel Magnet. 28 (2), 425–430. doi:10.1007/s10948-014-2807-6
Nowaczyk, N. R., Arz, H. W., Frank, U., Kind, J., and Plessen, B. (2012). Dynamics of the laschamp geomagnetic excursion from Black Sea sediments. Earth Planet. Sci. Lett. 351, 54–69. doi:10.1016/j.epsl.2012.06.050
Nowaczyk, N. R. (2011). Dissolution of titanomagnetite and sulphidization in sediments from Lake Kineret, Israel. Geophys. J. Inter. 187 (1), 34–44. doi:10.1111/j.1365-246X.2011.05120.x
Ojha, P. S., and Dubey, M. (2006). Gaint hydrocarbon fields of offshore Krishna-Godavari Basin. Petroview. 1, 26–30.
Özdemir, Ö., and Dunlop, D. J. (2010). Hallmarks of mathematization in low‐temperature remanence cycling of partially oxidized magnetite nanoparticles. J. Geophy. Res. Solid Earth 115 (B2). doi:10.1029/2009JB006756
Özdemir, Ö., Dunlop, D. J., and Moskowitz, B. M. (2002). Changes in remanence, coercivity and domain state at low temperature in magnetite. Earth Planet. Sci. Lett 194 (3‐4), 343–358. doi:10.1016/S0012‐821X(01)00562‐3
Özdemir, Ö., Dunlop, D. J., and Moskowitz, B. M. (1993). The effect of oxidation on the verwey transition in magnetite. Geophy. Res. Lett. 20 (16), 1671–1674. doi:10.1029/93GL01483
Pan, Y. X., Zhu, R. X., Banerjee, S. K., Gill, J., and Williams, Q. (2000). Rock magnetic properties related to thermal treatment of siderite: behavior and interpretation. J. Geophys. Res. Solid Earth 105, 783–794. doi:10.1029/1999JB900358
Passier, H. D., De Lange, G. J., and Dekkers, M. J. (2001). Magnetic properties and geochemistry of the active oxidation front and the youngestsapropel in the eastern Mediterranean Sea. Geophy. J. Inter. 145 (3), 604–614. doi:10.1046/j.0956‐540x.2001.01394.x
Passier, H. F., and Dekkers, M. J. (2002). Iron oxide formation in the active oxidation front above sapropel S1 in the eastern MediterraneanSea as derived from low‐temperature magnetism. Geophy .J. Inter. 150 (1), 230–240. doi:10.1046/j.1365‐246X.2002.01704.x
Peters, C., and Dekkers, M. J. (2003). Selected room temperature magnetic parameters as a function of mineralogy, concentration and grain size. Phys. Chem. Earth, Parts A/B/C. 28 (16-19), 659–667. doi:10.1016/S1474-7065(03)00120-7
Petersen, N., Von Dobeneck, T., and Vali, H. (1986). Fossil bacterial magnetite in deep‐sea sediments from the South Atlantic Ocean Nature 320, 611–615. doi:10.1038/320611a0
Pike, C. R., Roberts, A. P., and Verosub, K. L. (1999). Characterizing interactions infine magnetic particle systems using first order reversal curves. J. Appl. Phys. 85 (9), 6660–6667. doi:10.1063/1.370176
Poulton, S. W., Krom, M. D., and Raiswell, R. (2004). A revised scheme for the reactivity of iron (oxyhydr) oxide minerals towards dissolved sulfide. Geochim. Cosmochim. Acta. 68 (18), 3703–3715. doi:10.1016/j.gca.2004.03.012
Prabhakar, K. N., and Zutshi, P. L. (1993). Evolution of southern part of IndianEast Coast basin. J. Geol. Soc. Ind. 41, 215–230.
Prozorov, T., Mallapragada, S. K., Narasimhan, B., Wang, L., Palo, P., Nilsen‐Hamilton, , et al. (2007). Protein‐mediated synthesis of uniform super paramagnetic magnetite nanocrystals. Adv. Funct. Mater. 17 (6), 951–957. doi:10.1002/adfm.200600448
Ramana, M. V., Ramprasad, T., Kamesh Raju, K. A., and Desa, M. (2007). Occurrence of gas hydrates along the continental margins of India, particularly the Krishna‐Godavari off shore basin. Int. J.Env. Stu. 64 (6), 675–693. doi:10.1080/00207230701476321
Ramesh, R., and Subramanian, V. (1988). Temporal, spatial and size variation in the sediment transport in the Krishna River basin, India. J. Hydrol. 98, 53–65.
Ramprasad, T., Dewangan, P., Ramana, M. V., Mazumdar, A., Karisiddaiah, S. M., Ramya, E. R., et al. (2011). Evidence of slumping/sliding in Krishna–Godavari offshore basin due to gas/fluid movements. Mar. Petrol. Geol. 28, 1806–1816. doi:10.1016/j.marpetgeo.2011.02.007
Rao, V. P. (1991). Clay mineral distribution in the continental shelf sediments from Krishna to Ganges river mouth, east coast of India. Ind. J. Mar. Sci. 20, 7–12.
Reidinger, N., Pfeifer, K., Kasten, S., Garming, J. F. L., Vogt, C., and Hensen, C. (2005). Diagenetic alteration of magnetic signals by anaerobic oxidation of methane related to a change in sedimentation rate. Geochim. Cosmochim. Acta. 69, 4117–4126. doi:10.1016/j.gca.2005.02.004
Reilly, B. T., McCormick, M. L., Brachfeld, S. A., and Haley, B. A. (2020). Authigenic ferrimagnetic iron sulfide preservation due to non‐steady state diagenesis: a perspective from perseverance drift, North western Weddell Sea. Geochem. Geophy., Geosys. 21 (11), e2020GC009380. doi:10.1029/2020GC009380
Riedel, M., Spence, G. D., Chapman, N. R., and Hyndman, R. D. (2002). Seismic investigations of a vent field associated with gas hydrates, offshore vancouver Island. J. Geophys. Res. Solid Earth 107 (B9), EPM 5-1–EPM 5-16. doi:10.1029/2001JB000269
Riedinger, N., Formolo, M. J., Lyons, T. W., Henkel, S., Beck, A., and Kasten, S. (2014). An inorganic geochemical argument for coupled anaerobic oxidation of methane and iron reduction in marine sediments. Geobiology 12 (2), 172–181. doi:10.1111/gbi.12077
Roberts, A. P., Almeida, T. P., Church, N. S., Harrison, R. J., Heslop, D., Li, Y., et al. (2017). Resolving the origin of pseudo‐single domain magnetic behavior. J. Geophys. Res. Solid Earth 122, 9534–9558. doi:10.1002/2017JB014860
Roberts, A. P., Chang, L., Rowan, C. J., Horng, C. S., and Florindo, F. (2011). Magnetic properties of sedimentary greigite (Fe3S4): an update. Rev. Geophy. 49, RG1002. doi:10.1029/2010RG000336
Roberts, A. P., Jiang, W. T., Florindo, F., Horng, C. S., and Laj, C. (2005). Assessing the timing of greigite formation and the reliability of the upper olduvai polarity transition record from the crostolo river, Italy. Geophy. Res. Lett. 32 (5), L05307. doi:10.1029/2004GL022137
Roberts, A. P. (2015). Magnetic mineral diagenesis. Earth‐sci. Rev. 151, 1–47. doi:10.1016/j.earscirev.2015.09.010
Roberts, A. P. (1995). Tectonic rotation about the termination of a major strike‐slip fault, marlborough fault system, New Zealand. Geophy. Res. Lett. 22 (3), 187–190. doi:10.1029/94GL02582
Roberts, A. P., and Weaver, R. (2005). Multiple mechanisms of remagnetization involving sedimentary greigite (Fe3S4). Earth Planet. Sci. Lett. 231, 263–277. doi:10.1016/j.epsl.2004.11.024
Roberts, A. P., Zhao, X., Harrison, R. J., Heslop, D., Muxworthy, A. R., Rowan, C. J., et al. (2018). Signatures of reductive magnetic mineral diagenesis from unmixing of first order reversal curves. J. Geophys. Res. Solid Earth 123, 4500–4522. doi:10.1029/2018JB015706
Roberts, A. P., Zhao, X., Heslop, D., Abrajevitch, A., Chen, Y. H., Hu, P., et al. (2020). Hematite (α-Fe2O3) quantification in sedimentary magnetism: limitations of existing proxies and ways forward. Geosci. Lett. 7 (1), 1–11. doi:10.1186/s40562-020-00157-5
Rodelli, D., Jovane, L., Giorgioni, M., Rego, E. S., Cornaggia, F., Benites, M., et al. (2019). Diagenetic fate of biogenic soft and hard magnetite in chemically stratified sedimentary environments of Mamanguá Ría, Brazil. J. Geophys. Res. Solid Earth 124 (3), 2313–2330. doi:10.1029/2018JB016576
Rodelli, D., Jovane, L., Roberts, A. P., Cypriano, J., Abreu, F., and Lins, U. (2018). Fingerprints of partial oxidation of biogenic magnetite from cultivated and natural marine magnetotactic bacteria using synchrotron radiation. Environ. Microbiol. Rep. 10 (3), 337–343. doi:10.1111/1758-2229.12644
Römer, M., Sahling, H., Pape, T., dos Santos Ferreira, C., Wenzhöfer, F., Boetius, A., et al. (2014). Methane fluxes and carbonate deposits at a cold seep area of the central nile deep sea fan, eastern mediterranean sea. Mar. Geol. 347, 27–42. doi:10.1016/j.margeo.2013.10.011
Rowan, C. J., Roberts, A. P., and Broadbent, T. (2009). Reductive diagenesis, magnetite dissolution, greigite growth and paleomagnetic smoothing in marine sediments: a new view. Earth Planet. Sci. Lett. 277 (1‐2), 223–235. doi:10.1016/j.epsl.2008.10.016
Rowan, C. J., and Roberts, A. P. (2006). Magnetite dissolution, diachronous greigite formation, and secondary magnetizations from pyrite oxidation: unravelling complex magnetizations in Neogene marine sediments from New Zealand. Earth Planet. Sci. Lett. 241 (1‐2), 119–137. doi:10.1016/j.epsl.2005.10.017
Sagnotti, L., Cascella, A., Ciaranfi, N., Macrì, P., Maiorano, P., Marino, M., et al. (2010). Rock magnetism and paleomagnetism of the montalbano jonico section (Italy): evidence for late diagenetic growth of greigite and implications for magnetostratigraphy. Geophy. J. Int. 180 (3), 1049–1066. doi:10.1111/j.1365-246X.2009.04480.x
Sagnotti, L., Roberts, A. P., Weaver, R., Verosub, K. L., Florindo, F., Pike, C. R., et al. (2005). Apparent magnetic polarity reversals due to remagnetization resulting from late diagenetic growth of greigite from siderite. Geophy J. Int. 160 (1), 89–100. doi:10.1111/j.1365-246X.2005.02485.x
Sagnotti, L., and Winkler, A. (1999). Rock magnetism and paleomagnetism ofgreigite-bearing mudstones in the Italian peninsula. Earth Planet. Sci. Lett. 165, 67–80. doi:10.1016/s0012-821x(98)00248-9
Sangode, S. J., Sinha, R., Phartiyal, B., Chauhan, O. S., Mazari, R. K., Bagati, T. N., et al. (2007). Environmental magnetic studies on some quaternary sediments of varied depositional settings in the Indian sub‐continent. Quat. Int. 159, 102–118. doi:10.1016/j.quaint.2006.08.015
Sangode, S. J., Suresh, N., and Bagati, T. N. (2001). Godavari source in the Bengal fan sediments: results from magnetic susceptibility dispersal pattern. Curr. Sci., 80 (5), 660–664.
Shankar, U., and Riedel, M. (2010). Seismic and heat flow constraints from the gas hydrate system in the Krishna‐Godavari basin, India. Mar. Geol. 276 (1–4), 1–13. doi:10.1016/j.margeo.2010.06.006
Simonetti, A., Knapp, J. H., Sleeper, K., Lutken, C. B., Macelloni, L., and Knapp, C. C. (2013). Spatial distribution of gas hydrates from higher solutionseismic and core data, Woolsey Mound, Northern Gulf of Mexico. Mar. Petrol. Geol. 44, 21–33. doi:10.1016/j.marpetgeo.2013.04.004
Singhroha, S., Bünz, S., Plaza‐Faverola, A., and Chand, S. (2020). Detection of gas hydrates in faults using azimuthal seismic velocity analysis, vastness ridge, W‐svalbard margin. J. Geophys. Res. Solid Earth 125 (2), e2019JB017949. doi:10.1029/2019JB017949
Snowball, I. F. (1991). Magnetic hysteresis properties of greigite (Fe3S4) and a new occurrence in Holocene sediments from Swedish Lappland. Phys. Earth Planet. Inter. 68 (1–2), 32–40. doi:10.1016/0031-9201(91)90004-2
Snowball, I., and Thompson, R. (1990). A stable chemical remanence in holocene sediments. J. Geophys. Res. Solid Earth 95 (B4), 4471–4479. doi:10.1029/JB095iB04p04471
Suess, E. (2014). Marine cold seeps and their manifestations: geological control, biogeochemical criteria and environmental conditions. Int. J. Earth Sci. 103 (7), 1889–1916. doi:10.1007/s00531-014-1010-0
Sztybor, K., and Rasmussen, T. L. (2017). Diagenetic disturbances of marine sedimentary records from methane‐influenced environments in the fram strait as indications of variation in seep intensity during the last 35000 years. Boreas 46 (2), 212–228. doi:10.1111/bor.12202
Tarduno, J. A., Cottrell, R. D., and Smirnov, A. V. (2006). The paleomagnetism of single silicate crystals: recording geomagnetic field strength during mixed polarity intervals, super chrons, and inner core growth. Rev. Geophy. 44 (1), RG1002. doi:10.1029/2005RG000189
Tarduno, J. A. (1995). Superparamagnetism and reduction diagenesis in pelagic sediments: enhancement or depletion?. Geophy. Res. Lett. 22 (11), 1337–1340. doi:10.1029/95GL00888
Usapkar, A., Dewangan, P., Kocherla, M., Ramprasad, T., Mazumdar, A., and amd Ramana, M. V. (2014). Enhanced methane flux event and sediment dispersal pattern in the Krishna–Godavari offshore basin: evidences from rock magnetic techniques. Mar. Petrol. Geol. 58, 461–475. doi:10.1016/j.marpetgeo.2014.08.008
Valdez-Grijalva, M. A., Nagy, L., Muxworthy, A. R., Williams, W., Roberts, A. P., and Heslop, D. (2020). Micromagnetic simulations of first-order reversal curve (FORC) diagrams of framboidal greigite. Geophys. J. Int. 222 (2), 1126–1134. doi:10.1093/gji/ggaa241
Verwey, E. J. W. (1939). Electronic conduction of magnetite (Fe3O4) and its transition point at low temperatures. Nature 144 (3642), 327. doi:10.1038/144327b0
Verwey, E. J. W. (1947). Theory of the stability of lyophobic colloids. J. Phys. Chem. 51 (3), 631–636. doi:10.1021/j150453a001
Wang, X., Liu, B., Qian, J., Zhang, X., Guo, Y., Su, P., et al. (2018). Geophysical evidence for gas hydrate accumulation related to methane seepage in the Taximan basin, South China Sea. J.f Asian Earth Sci. 168, 27–37. doi:10.1016/j.jseaes.2017.11.011
Wenau, S., Spiess, V., Pape, T., and Fekete, N. (2015). Cold seeps at the salt front in the lower congo basin II: the impact of spatial and temporal evolution of salt-tectonics on hydrocarbon seepage. Mar. Petrol. Geol. 67, 880–893. doi:10.1016/j.marpetgeo.2014.09.021
Yamamoto, Y., Chiyonobu, S., Kanamatsu, T., Ahagon, N., Aoike, K., Kamiya, N., et al. (2018). Repeated large scale masstransport deposits and consequent rapid sedimentation in the western part of the Bay of Bengal, India. Geol. Soc. Spec. Publ. 477, SP477.12. doi:10.1144/SP477.12
Yamazaki, T., Abdeldayem, A. L., and Ikehara, K. (2003). Rock-magnetic changes with reduction diagenesis in Japan Sea sediments and preservation of geomagnetic secular variation in inclination during the last 30,000 years. Earth Planet. Space 55, 327–340. doi:10.1186/BF03351766
Keywords: cold seep, methane hydrate, rock magnetism, diagenesis, Bay of Bengal
Citation: Gaikwad V, Badesab F, Dewangan P and Kotha M (2021) Diagenesis of Magnetic Minerals in Active/Relict Methane Seep: Constraints From Rock Magnetism and Mineralogical Records From Bay of Bengal. Front. Earth Sci. 9:638594. doi: 10.3389/feart.2021.638594
Received: 07 December 2020; Accepted: 25 January 2021;
Published: 04 March 2021.
Edited by:
Luigi Jovane, University of São Paulo, BrazilReviewed by:
Zhiyong Lin, Sun Yat-sen University, ChinaSteffen Wiers, Uppsala University, Sweden
Ron Shaar, Hebrew University of Jerusalem, Israel
Copyright © 2021 Gaikwad, Badesab, Dewangan and Kotha. This is an open-access article distributed under the terms of the Creative Commons Attribution License (CC BY). The use, distribution or reproduction in other forums is permitted, provided the original author(s) and the copyright owner(s) are credited and that the original publication in this journal is cited, in accordance with accepted academic practice. No use, distribution or reproduction is permitted which does not comply with these terms.
*Correspondence: Firoz Badesab, Zmlyb3pAbmlvLm9yZw==