- Department of Geology, Portland State University, Portland, OR, United States
We characterize the topographic evolution of the Pacific Northwest, United States, during the Cenozoic. New paleosol carbonate stable isotope (δ18O) results from central Oregon are presented, along with published proxy data, including fossil teeth, smectites, and carbonate concretions. We interpret a polygenetic history of Cascade Mountain topographic uplift along-strike, characterized by: 1) Steady uplift of the Washington Cascades through the Cenozoic due long-term arc rotation and shortening against a Canadian buttress, and 2) Uplift of the Oregon Cascades to similar-to-modern elevations by the late Oligocene, followed by topographic stagnation as extension developed into the Neogene. Since the Miocene, meteoric water δ18O values have decreased in Oregon, possibly due to emergence of the Coast Range and westward migration of the coastline. Spatial variability in isotopic change throughout the Pacific Northwest suggests that secular global climate change is not the primary forcing mechanism behind isotopic trends, though Milankovitch cycles may be partly responsible for relatively short-term variation.
Introduction
Stable isotope ratios of water illuminate temporal changes in a region’s climate and topography (e.g. Savin, 1982; Garzione et al., 2000; Rowley and Garzione, 2007). Oxygen (δ18O) isotope ratios in pedogenic carbonates have been utilized to constrain paleoclimate (Cerling and Quade, 1993; Quade et al., 2007). While studies have looked at Cascade Mountain uplift using single proxies for analysis (e.g. Kohn et al., 2002; Kohn and Law, 2006; Bershaw et al., 2019), few comprehensive studies have taken a multi-proxy approach.
Considering multi-proxy stable isotope data can reduce uncertainty by constraining forcing mechanisms of isotopic change (e.g. Gébelin et al., 2012; Currie et al., 2016). We present new soil carbonate δ18O data and synthesize existing paleowater proxy data to provide a more comprehensive interpretation of spatial (Pacific Northwest) and temporal (Cenozoic) patterns. Further, by comparing temporal patterns of stable oxygen isotopes in the Pacific Northwest to the record of global Cenozoic δ18O change and Milankovitch-scale variation (e.g. Zachos et al., 2001), the influence of global climate relative to local topography changes is investigated.
Background
The Pacific Northwest is in the mid-latitudes of the northern hemisphere. Orographic precipitation is significant in mountains across the region, including the Cascade Volcanic Arc (Cascades), the Olympic Mountains in Washington, and the Oregon Coast Range. The Cascade Mountains’ rainshadow is associated with significant differences in precipitation amount on either side of the range. Average precipitation along at roughly −122°longitude (windward) ranges from 150–210 cm/year, while average precipitation at −121°longitude (lee) ranges from 22–25 cm/year (Figure 1A). Westerly air-flow patterns in the region are interpreted to have remained relatively constant during the Cenozoic based on depositional patterns of wind-blown ash (Robinson et al., 1990).
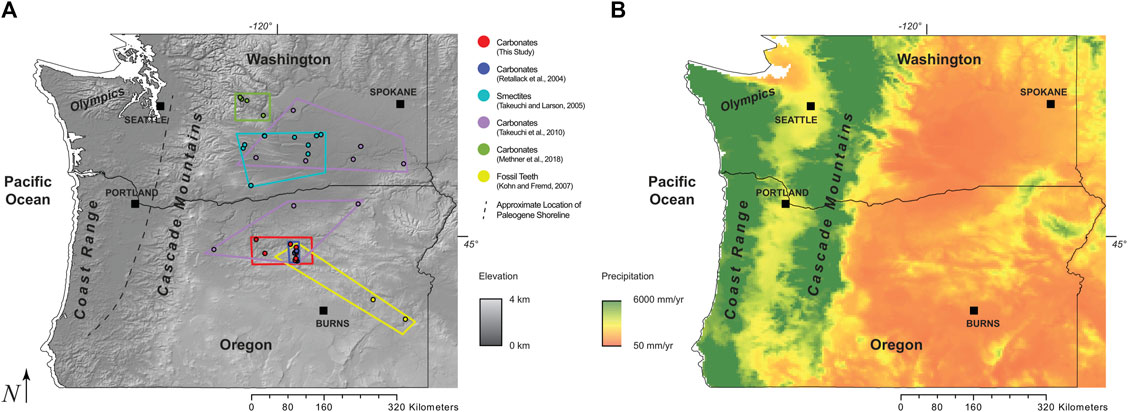
FIGURE 1. (A) Location map showing the extent of new data (red) and published datasets throughout the Pacific Northwest. Elevation is shown as greyscale in km. Dashed line shows the inferred Paleogene shoreline prior to the emergence of the Oregon Coast Range based on paleogeographic maps by Orr and Orr (1999) and references therein. Digital Elevation Model (DEM) is based on the United States. Geological Survey Global 30 Arc-Second GTOPO30 (https://doi.org/10.5066/F7DF6PQS). (B) Map showing annual precipitation amount, averaged from 1981–2010 across the Pacific Northwest. Data Source: PRISM Climate Group (http://prism.oregonstate.edu).
In Oregon and Washington, the Cascades extend north-south from British Columbia, Canada, to CA, United States. The Cascades are a magmatic arc that formed from the subduction of the Juan de Fuca Plate under the North American Plate (Priest, 1990). The onset of Cascade volcanism is interpreted to have begun ∼45 Ma (Wells and McCaffrey, 2013). By ∼35 Ma, the entire magmatic arc had been established from northern California to Mt. Rainier (Priest, 1990). The Clarno Formation of central Oregon (Bestland et al., 1999), Siletz River, and Crescent Formations of Washington contain significant amounts of subaerial volcanic rock that erupted as early as 51 Ma (Wells et al., 2014), suggesting some terrestrial settings by that time. The first plutons of the Cascade arc appeared at ∼26 Ma (du Bray and John, 2011), with a significant volume of ignimbrites erupting starting at 39 Ma (Robinson et al., 1984). Volcanic activity slowed greatly between 17–10 Ma (Priest, 1990). In the mid-Miocene, the Columbia River Flood Basalt Group (CRBG) inundated much of Oregon and southern Washington (Beeson et al., 1989; Sherrod and Smith, 2000). They are absent in the Cascades themselves, suggesting the Cascades served as a topographic barrier. In the Late Miocene, further volcanic activity is evidenced by ash deposition and volcaniclastics of the Deschutes Formation and widespread Rattlesnake Tuff (Smith et al., 1987; Streck and Grunder 1995).
We present new δ18O from paleosol carbonates from the lee side of the Cascades in Central Oregon (Figure 1B). Additionally, we synthesize published stable isotope data from Washington and Oregon, including fossil teeth (Kohn and Fremd, 2007), paleosol carbonates (Retallack et al., 2004; Takeuchi et al., 2010; Lechler et al., 2018), smectites (Takeuchi and Larson, 2005), and carbonate concretions (Methner et al., 2016) (Figure 1, Supplementary Table S1).
The late Cenozoic topographic history of the Cascades is disputed. Of the publications compiled for this study, Takeuchi and Larson (2005), Takeuchi et al., (2010) and Methner et al. (2016) propose most uplift occurred in the Neogene. Kohn and Fremd (2007) present data that may reflect near modern elevations in the Cascades by the late Oligocene, with an additional ∼800 m of uplift in the last ∼6 Ma. This interpretation is consistent with an interpretation of volcanic glass data (Bershaw et al., 2019). Retallack (2004a) shows that late Oligocene paleosols track Milankovitch cycles, but also suggests that Cascade uplift and rainshadow development play roles in longer-term trends (Retallack, 2004b). Lastly, dating of igneous rocks and thermochronologic data suggest that the uplift history of the Cascades varies across space and time (Robinson et al., 1984; Verplanck and Duncan, 1987; Conrey et al., 2002; Pesek et al., 2020).
Methods
Paleosol carbonates throughout the John Day Formation of known age (∼30–16 Ma) were sampled from locations described in Retallack et al., (1999) (Figure 1B, Supplementary Figure S3). ∼100 g of sample was collected after removing the upper 30 cm of outcrop to reduce the influence of evaporation. Diagenetically altered samples, identified in the field by spar, calcite veins, and oxidation, were avoided (e.g. Bershaw et al., 2012).
Samples were crushed to powder and reacted with 30% H2O2 to remove organics. Analyses were carried out at the Iowa State University Stable Isotope Lab (SIL) on a ThermoFinnigan Delta Plus XL mass spectrometer in continuous-flow mode via Thermo GasBench II with a CombiPal autosampler. The long-term precision of the mass spectrometer for δ18O is 0.06‰. Isotopic results are reported in standard delta notation (δ18O) relative to Vienna Standard Mean Ocean Water (VSMOW) (Supplementary Table S1).
To compare different proxy material, we estimate the stable isotopic composition of meteoric water using empirically derived fractionation factors (O'Neil et al., 1969; Yeh and Savin, 1977). To reduce interpretive bias, we apply a consistent standard for estimating the temperature of carbonate formation using ancient flora (Wolfe, 1994, Supplementary Table S1) or Δ47 temperatures when available. Our estimates are consistent with published water values where available. Fossil tooth data was averaged over the length of teeth and converted to water using an empirical relationship between environmental water and tooth chemistry (Kohn and Fremd, 2007). Differences between proxy data may exist that are related to unique conditions of mineral formation, such as the season or temperature of precipitation (Quade et al., 2007; Breecker et al., 2009).
We collected and analyzed 20 carbonate samples in central Oregon which we report here (Supplementary Table S1). Sample ages were determined by comparing stratigraphic position with published ages based on radiometric dating of tuff beds (Retallack et al., 1999; Retallack, 2004a). Exceptions are COS197 and COS197b, which are dated based on their position atop the Dayville Basalt (16.1 Ma) and below the Mascall Tuff Bed (15.2 Ma) (Prothero et al., 2006; Bestland et al., 2008; Drewicz and Kohn, 2018).
Results
The average δ18O value of our data is −11.2‰ with a range from −14.6 to −7.0‰. This is 1.6‰ more positive than the average of modern meteoric water in the lee (east) of the Oregon Cascade Mountains (−12.8‰), but within the range (−16.2 to −8.8‰) (Supplementary Table S2).
We combine our results with published data throughout the region, including 172 paleosol carbonates from Washington and Oregon (Takeuchi et al., 2010), 367 paleosol carbonates from Oregon (Retallack et al., 2004), 22 smectites from Washington (Takeuchi and Larson, 2005), 243 fossil teeth from Oregon (Kohn and Fremd, 2007), 31 modern carbonates from Washington (Lechler et al., 2018), and 119 carbonate concretions from Washington (Methner et al., 2016) (Figures 1B2 and Supplementary Table S1). Published modern stream water δ18O values from Washington and Oregon are reported for comparison (Supplementary Table S2), though they integrate less time than mineral proxy material so are inherently more variable. Ages of Eocene carbonates in WA, United States represent maximum ages, as there is uncertainty on the timing of potential diagenesis (Methner et al., 2016).
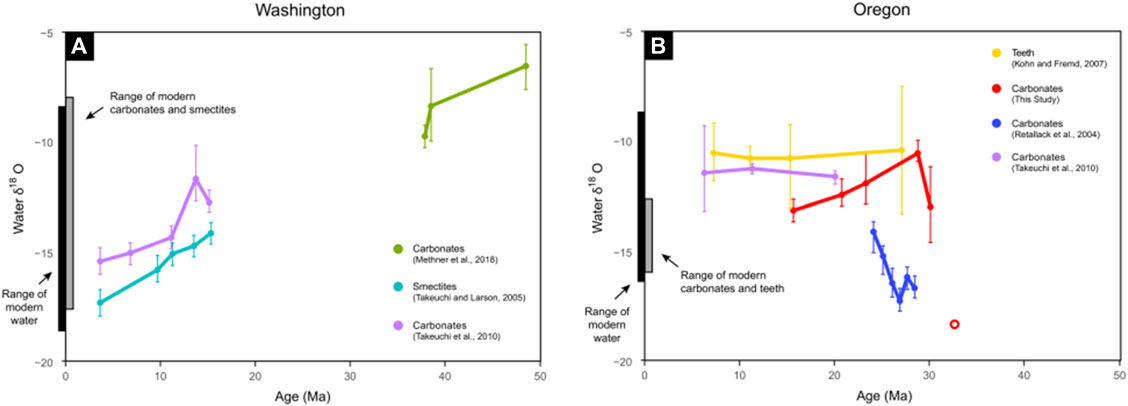
FIGURE 2. Plots showing temporal variations in meteoric water based on δ18O values of carbonates, smectites, and fossil teeth for central Washington at ∼47 latitude (A) and central Oregon at ∼45 latitude (B), both in the lee (east side) of the Cascade Mountains. Sample δ18O values are averaged within 2.5 million-year windows (shown as points) to emphasize trends across geologic time. Uncertainty bars represent the range of δ18O values found within each time-window. Samples are grouped (color-coded) by publication and sample type. The range of modern meteoric water δ18O east of the Cascade Mountains is shown (black bar) for both Washington and Oregon based on published stream data (Table S2). Ranges in published modern carbonates east of the Cascade Mountains (Takeuchi et al., 2010; Lechler et al., 2018 and this study), smectites (Takeuchi and Larson, 2005), and mammal teeth (Kohn and Fremd, 2007) δ18O data (Supplementary Table S1) are also shown for each state (gray bar). The open red circle (Oregon) represents sample JDRL_02, interpreted to be diagenetic carbonate.
Together, these data do not show significant relationships between isotopic composition and latitude, longitude, elevation, or age (R2 of 0.15, 0.00, 0.22, and 0.13, respectively). We separate datasets by state, as this provides a convenient latitudinal break between the northern Cascades of Washington and central Cascades of Oregon. In Washington, the average paleowater δ18O for all samples is −14.3 ± 2.8‰ (1σ SD) and range from −18.0 to −6.8‰. There is a weak, negative correlation between age and δ18O in samples from Washington, with an R2 of 0.40. The average δ18O in Oregon is −11.9 ± 2.1‰ (1σ SD), ∼2.4‰ more positive than Washington, with a range of −15.8 to −8.4‰. Oregon carbonates demonstrate negligible correlation between age and δ18O (R2 of 0.03). Sample δ18O values from individual studies are averaged within 2.5 million-year bins to emphasize trends in climate and topography across geologic time (Figure 2).
Discussion
Carbonates as a Proxy for Ancient Meteoric Water
Before interpreting geologic and climatic trends, we assess whether proxies accurately reflect meteoric water at the time of deposition. Several paleosol carbonate samples from central Oregon likely experienced some form of alteration which may change primary carbonate δ18O values, due to different formation water and/or temperatures of mineral precipitation. One Oligocene sample from our dataset (JDRL02, Figure A-6 of Supplementary Figure S3) has an anomalously low δ18O value (−18.2‰) (open red circle in Figure 2A). This sample is purple in hue and only weakly calcareous, so it is likely that it underwent oxidation weathering sometime after deposition, potentially obfuscating the isotopic signal with oxide minerals (Hoefs, 2009). In addition, pedogenic carbonate δ18O values from Retallack et al. (2004) across the late Oligocene (blue curve in Figure 2A) plot more negative (∼5‰) than roughly contemporaneous fossil teeth and paleosols. This difference may be explained by higher temperatures of carbonate precipitation. The Turtle Cove Member, which makes up samples 24 Ma and older (Retallack et al., 2004), contains significant amounts of celadonite, which is an alteration product forming between 27–100°C (Weaver, 1989; Lander and Hay, 1993). Hay (1963) provides a narrower range of water temperatures for this interaction (27–55°C) and an age estimate of when this occurred (24 ± 2 Ma). If the temperature of carbonate precipitation was >50°C, as observed in Paleogene carbonates from Washington (Methner et al., 2016), this would cause a decrease in δ18O of over 5‰ (Kim and O’Neil, 1997), explaining anomalously low δ18O values (blue curve in Figure 2) relative to contemporaneous proxy data.
Regional Synthesis: Oregon
Previous studies have proposed various interpretations of Cenozoic mountain uplift and climate change in Oregon based on proxy data, including late Neogene uplift of the Cascade Mountains (Takeuchi and Larson, 2005; Takeuchi et al., 2010), contrasted by interpretations of high topography by late Oligocene time (Kohn and Fremd, 2007; Bershaw et al., 2020), in addition to the assertion that global climate change exerts a first-order control on isotopic records in Oregon (Retallack et al., 2004). In our regional synthesis, we focus on tectonic (million-year scale) forcing of isotopic data and do not consider carbonates from Retallack et al. (2004) (blue curve in Figure 2A), or our sample JDRL02 (open red circle in Figure 2A), whose temperature of formation may be higher than we assumed.
Our new time-averaged carbonate data is within the range of other water proxy data in Oregon. The isotopic variability in contemporaneous paleowater proxy material is similar to that observed in modern soils and teeth (grey vertical bar in Figure 2A) and smaller than modern meteoric water (black vertical bar in Figure 2A). Our paleosol carbonate data and fossil tooth data of Kohn and Fremd (2007) (red and yellow curve in Figure 2A) are within the wide range of modern meteoric water (dark vertical bar in Figure 2A) in the Oregon Cascade rainshadow, supporting the interpretation that some topography, possibly similar to modern (∼1.5 km), existed by the late Oligocene. Additionally, modern coastal water δ18O values (average −6.8‰, Bershaw et al., 2020) are 5.3‰ more positive than the average δ18O of Paleogene, non-diagenetic carbonate in Oregon, which average −12.1‰ (n = 5). Our interpretation of near modern topography in the Oregon Cascades by Oligocene time is consistent with evidence of significant volcanism at that time (Verplanck and Duncan, 1987; Priest, 1990; Taylor, 1990) and volcanic glass δD data across the range (Bershaw et al., 2019).
Our new paleosol carbonate δ18O values decrease by ∼2.5‰ from 29 to 16 Ma. However, taken as a whole, our data is within the range of fossil teeth and previously published paleosol carbonate δ18O results whose average stays relatively constant across the Oligocene and early Miocene, suggesting Cascade Mountain topography was relatively stable during this time. Variation in values (particularly high among teeth) may reflect Milankovitch cycles and/or spatial variability among sample locations within the study area (Figure 1). This could reflect local topography and associated microclimates (Retallack, 2004b; Kukla et al., 2021 this volume), as observed in modern meteoric water across the same spatial extent (Bershaw et al., 2020).
Fossil tooth (Kohn and Fremd, 2007) and paleosol carbonate (Takeuchi et al., 2010) δ18O values from the late Miocene and Pliocene are 2–3‰ more positive than modern carbonates and teeth (Figure 2A) in central Oregon. This is likely not caused by global cooling at this time, as northern hemisphere ice sheet expansion resulted in a ∼1.5‰ increase in oceanic δ18O, whereas our data reflect a decrease in δ18O (Zachos et al., 2001). Instead, we suggest this negative shift in isotopic composition since the late Miocene may reflect the westward migration (>100 km) of the Oregon coast, driven by the Neogene emergence of a subaerial Oregon Coast Range (Figure 1B). A drop in δ18O values would be expected as the distance to the Pacific Ocean increased and a subaerial Coast Range created a modest moisture barrier, further depleting 18O in vapor. Emergence of the Coast Range at this time is independently constrained by an analysis of the Portland Basin, which shows a significant decrease in accommodation space sometime after the eruption of the CRBG in the mid-Miocene and coeval migration of basin depocenters eastward (Scanlon et al., 2019 in press). Modern coastal meteoric water δ18O values are ∼2.4‰ higher than those in the western foothills of the Cascade Mountains (Bershaw et al., 2020), where the coastline was prior to Coast Range uplift. This suggests that uplift of the Coast Range could account for the discrepancy between late Miocene and modern water proxy data.
Regional Synthesis: Washington
Estimated meteoric water δ18O values from Eocene carbonates in Washington (Methner et al., 2016) are relatively positive (average −8.8‰) compared to both modern water (average −14.6‰) and modern carbonates and smectites nearby (average −14.0‰) (Figure 2B and Supplementary Table S1). Instead, they are similar to the isotopic composition of modern stream water at low elevations on the west side of the Olympics (−7.1‰) and Cascades in WA, United States (−9.1‰) (Bershaw et al., 2020), suggesting the northern Cascades Mountains were not a significant topographic barrier to westerly derived moisture at that time.
Eocene paleowater δ18O values are also significantly more positive than Miocene and Pliocene smectite and paleosols (Figure 2B), resulting in a progressive trend towards more negative δ18O values across the Cenozoic. This is not likely the result of global cooling, as temperature change is moderated by an increase in oceanic δ18O of ∼1.8‰ from the late Eocene to Miocene (Zachos et al., 2001). We hypothesize that the northern Cascades in Washington have undergone progressive topographic uplift since the Paleogene. At what point uplift resulted in a rainshadow in central WA, United States is less clear, though paleofloral data from Idaho and paleosol carbonate δ13C data from Washington suggest arid conditions similar to modern were not established until the late Miocene or Pliocene (Takeuchi et al., 2010; Mustoe and Leopold, 2013). Progressive uplift through much of the Cenozoic is consistent with evidence that the northern Cascades were an active volcanic arc in the Paleogene (Tabor et al., 1984) and thermochronology that shows slow uplift of the Washington Cascades (<0.25 km/m.y.) from the Eocene to the late Miocene (Reiners et al., 2002; Pesek et al., 2020), with ongoing uplift today (Wells and McCaffrey, 2013).
Polygenetic History of Topography Across the Pacific Northwest
Our synthesis of water proxy datasets shows a polygenetic uplift history for the Cascade Mountains along strike. Oregon and Washington carbonates show distinct temporal trends, with differences as large as ∼5‰ across the late Miocene, where Oregon paleowaters are more enriched than contemporaneous water in Washington (Figure 2). These spatial discrepancies support our assertion that global climate change is not the primary forcing mechanism behind isotopic change in the Pacific Northwest on tectonic (million year) scales. Instead, isotopic proxy records suggest that the Northern Cascades in Washington were not a significant barrier to westerly airflow in the Eocene, but experienced progressive topographic uplift from the Paleogene to now, while the Cascades in Oregon formed a topographic barrier by the late Oligocene, but remained relatively unchanged into the Neogene. This interpretation is consistent with the tectonic framework of the region since mid-Miocene time, where east-west extension (subsidence) has developed in the Oregon Cascades (Conrey et al., 1997; Guffanti and Weaver, 1988) while uplift of the northern Cascades is due to subduction-related rigid block rotation (Figure 4 from Wells et al., 1998), delamination (Reiners et al., 2002), and/or erosional denudation (Enkelmann et al., 2015). Our interpretation of polygenetic uplift across the Oregon and Washington Cascades in consistent with volcanic glass δD values (Bershaw et al., 2019) and thermochronometry, which shows significant geographic variation in exhumation timing (Pesek et al., 2020), as opposed to contemporaneous, wholesale uplift of the entire Cascade Mountain chain.
Additionally, we hypothesize that the subaerial emergence of the Coast Range in Oregon and westward migration of the coastline (Scanlon et al., 2019 in press) caused a modest depletion in proxy material δ18O values that is not apparent in Washington proxy data, where the coastline remains at the base of the northern Cascades and the Puget Lowland remains connected to the ocean today. The migration of the coastline west and drying of the Willamette Valley (forearc) in the late Miocene coincides with aridification of central Washington and Idaho (Takeuchi et al., 2010; Mustoe and Leopold, 2013). We speculate that this shift in paleoclimate may reflect an increase in continentality (distance from oceanic water source) as opposed to wholesale Cascade Mountain uplift at that time.
Conclusion
We present new pedogenic carbonate δ18O data from central Oregon and synthesize this with published water proxy data from across the Pacific Northwest. We interpret a polygenetic history of Cascade Mountain topographic uplift along-strike, characterized by: 1) Progressive uplift of the Washington Cascades through the Cenozoic due long-term arc rotation and shortening against a Canadian buttress, and 2) Uplift of the Oregon Cascades to similar-to-modern elevations by the late Oligocene, followed by topographic stagnation as extension developed into Neogene time. Since the Miocene, meteoric water δ18O values have decreased in Oregon, possibly due to emergence of the Coast Range and westward migration of the coastline. Isotopic trends could also be influenced by changes in seasonality, the isotopic composition of the Pacific Ocean, and Blue or Klamath Mountain topography. Lastly, variability in δ18O values of proxy materials at any given time is likely forced by Milankovitch cycles, local topography (and associated microclimates), and volatility inherent in arid environments, where evaporation of surface water can be significant.
Data Availability Statement
The original contributions presented in the study are included in the article/Supplementary Material, further inquiries can be directed to the corresponding authors.
Author Contributions
There are two authors who contributed to this manuscript. AM (corresponding author) contributed to collecting and analyzing samples, writing the manuscript, interpreting the results and compiling figures and tables. JB contributed to collecting samples, writing the manuscript, interpreting results, and compiling figures. Both authors agree to be accountable for the content of the work.
Conflict of Interest
The authors declare that the research was conducted in the absence of any commercial or financial relationships that could be construed as a potential conflict of interest.
Acknowledgments
Samples were collected at John Day Fossil Beds National Monument under Permit JODA-2019-SCI-0009. The authors would like to thank Dr. Nicholas Famoso, The National Park Service, and Patrick Gamman for facilitating sample collection and providing insight. Publication of this article in an open access journal was funded by the Portland State University Library's Open Access Fund.
Supplementary Material
The Supplementary Material for this article can be found online at: https://www.frontiersin.org/articles/10.3389/feart.2021.624961/full#supplementary-material.
References
Beeson, M. H., Tolan, T. L., and Anderson, J. L. (1989). “The Columbia River Basalt Group in western Oregon; geologic structures and other factors that controlled flow emplacement patterns,” in Volancism and tectonism in the Columbia River flood-basalt province: Geological Society of America Special Paper. Editors S. P. Reidel, and P. R. Hooper (Boulder, Colorado: Geological Society of America), 239, 223–246.
Bershaw, J., Garzione, C. N., Schoenbohm, L., Gehrels, G., and Tao, L. (2012). Cenozoic evolution of the Pamir plateau based on stratigraphy, zircon provenance, and stable isotopes of foreland basin sediments at Oytag (Wuyitake) in the Tarim Basin (west China). J. Asian Earth Sci. 44, 136–148. doi:10.1016/j.jseaes.2011.04.020
Bershaw, J., Cassel, E. J., Carlson, T. B., Streig, A. R., and Streck, M. J. (2019). Volcanic glass as a proxy for Cenozoic elevation and climate in the Cascade Mountains, Oregon, USA. J. Volcanol. Geotherm. Res. 381, 157–167. doi:10.1016/j.jvolgeores.2019.05.021
Bershaw, J., Hansen, D. D., and Schauer, A. J. (2020). Deuterium excess and 17O-excess variability in meteoric water across the Pacific Northwest, USA. Tellus B: Chem. Phys. Meteorol. 72 (1), 1–17. doi:10.1080/16000889.2020.1773722
Bestland, E. A., Forbes, M. S., Krull, E. S., Retallack, G. J., and Fremd, T. (2008). Stratigraphy, paleopedology, and geochemistry of the middle Miocene Mascall Formation (type area, central Oregon, USA). PaleoBios. 28 (2), 41–61.
Bestland, E. A., Hammond, P. E., Blackwell, D. L. S., Kays, M. A., Retallack, G. J., and Stimac, J. (1999). Geologic framework of the clarno unit, John day fossil beds national monument, central Oregon. Oreg. Geol. 61 (1), 3–19.
Breecker, D. O., Sharp, Z. D., and McFadden, L. D. (2009). Seasonal bias in the formation and stable isotopic composition of pedogenic carbonate in modern soils from central New Mexico, USA. Geol. Soc. Am. Bull. 121, 630–640.
Cerling, T. E., and Quade, J. (1993). Climate change in continental isotopic records. Geophys. Monogr. 78, 217–231.
Conrey, R. M., Sherrod, D. R., Hooper, P. R., and Swanson, D. A. (1997). Diverse primitive magmas in the Cascade arc, northern Oregon and southern Washington. Can. Mineral. 35 (2), 367–396.
Conrey, R. M., Taylor, E. M., Donnelly-Nolan, J. M., and Sherrod, D. R. (2002). “North-central Oregon Cascades: exploring petrologic and tectonic intimacy in a propagating intra-arc rift,” in Field guide geologic process in cascadia; 98th annual meeting of the cordilleran section of the geological society of America. Editor G. W. Moore (Oregon: Oregon Department of Geology and Mineral Industries), 36, 47–90.
Currie, B. S., Polissar, P. J., Rowley, D. B., Ingalls, M., Li, S., Olack, G., et al. (2016). Multiproxy paleoaltimetry of the late Oligocene-Pliocene Oiyug basin, southern Tibet. Am. J. Sci. 316 (5), 401–436. doi:10.2475/05.2016.01
Drewicz, A. E., and Kohn, M. J. (2018). Stable isotopes in large herbivore tooth enamel capture a mid-Miocene precipitation spike in the interior Pacific Northwest. Palaeogeogr. Palaeoclimatol. Palaeoecol. 495, 1–12. doi:10.1016/j.palaeo.2017.11.022
du Bray, E., and John, D. A. (2011). Petrologic, tectonic, and metallogenic evolution of the Ancestral Cascades magmatic arc, Washington, Oregon, and northern California. Geosphere 7 (5), 1102–1133. doi:10.1130/ges00669.1
Enkelmann, E., Ehlers, T. A., Merli, G., and Methner, K. (2015). Thermal and exhumation history of the Eocene chumstick basin, Washington state, USA. Tectonics 34 (5), 951–969. doi:10.1002/2014tc003767
Garzione, C. N., Quade, J., DeCelles, P. G., and English, N. B. (2000). Predicting paleoelevation of Tibet and the Himalaya from δ18O vs. altitude gradients in meteoric water across the Nepal Himalaya. Earth Planet. Sci. Lett. 183 (1–2), 215–229. doi:10.1016/s0012-821x(00)00252-1
Gébelin, A., Mulch, A., Teyssier, C., Page Chamberlain, C., and Heizler, M. (2012). Coupled basin-detachment systems as paleoaltimetry archives of the western North American Cordillera. Earth Planet. Sci. Lett. 335–336, 36–47. doi:10.1016/j.epsl.2012.04.029
Guffanti, M., and Weaver, C. S. (1988). Distribution of late Cenozoic volcanic vents in the Cascade Range: volcanic arc segmentation and regional tectonic considerations. J. Geophys. Res. 93 (B6), 6513–6529. doi:10.1029/jb093ib06p06513
Hay, R. L. (1963). Stratigraphy and zeolitic diagenesis of the john day formation of Oregon. California: University of California Press
Hayes, J. M. (2001). Fractionation of carbon and hydrogen isotopes in biosynthetic processes. Rev. Mineral. Geochem. 43 (1), 225–277. doi:10.2138/gsrmg.43.1.225
Kim, S. T., and O'Neil, J. R. (1997). Equilibrium and nonequilibrium oxygen isotope effects in synthetic carbonates. Geochim. Cosmochim. Acta 61 (16), 3461–3475. doi:10.1016/s0016-7037(97)00169-5
Kohn, M. J., and Fremd, T. J. (2007). Tectonic controls on isotope compositions and species diversification, John Day Basin, central Oregon. PaleoBios. 27 (2), 48–61.
Kohn, M. J., and Law, J. M. (2006). Stable isotope chemistry of fossil bone as a new paleoclimate indicator. Geochim. Cosmochim. Acta. 70 (4), 931–946. doi:10.1016/j.gca.2005.10.023
Kohn, M. J., Miselis, J. L., and Fremd, T. J. (2002). Oxygen isotope evidence for progressive uplift of the Cascade Range, Oregon. Earth Planet. Sci. Lett. 204 (1–2), 151–165. doi:10.1016/s0012-821x(02)00961-5
Kukla, T., Ibarra, D. E., Caves, J. K., Gooley, J. T., Mullins, C. E., Kramer, S., et al. (2021). High-resolution stable isotope paleotopography of the John day region, Oregon, USA. Front. Earth Sci. 9, 30. doi:10.3389/feart.2021.635181
Lander, R. H., and Hay, R. L. (1993). Hydrogeologic control on zeolitic diagenesis of the White River sequence. Geol. Soc. Am. Bull. 105 (3), 361–376. doi:10.1130/0016-7606(1993)105<0361:hcozdo>2.3.co;2
Lechler, A. R., Huntington, K. W., Breecker, D. O., Sweeney, M. R., and Schauer, A. J. (2018). Loess-paleosol carbonate clumped isotope record of late Pleistocene-Holocene climate change in the Palouse region, Washington State, USA. Quat. Res. 90 (2), 331–347. doi:10.1017/qua.2018.47
Mack, G. H., Cole, D. R., and Treviño, L. (2000). The distribution and discrimination of shallow, authigenic carbonate in the Pliocene-Pleistocene Palomas Basin, southern Rio Grande rift. Geol. Soc. Am. Bull. 112 (5), 643–656. doi:10.1130/0016-7606(2000)112<643:tdados>2.0.co;2
Methner, K., Fiebig, J., Wacker, P., Umhoefer, J., Chamberlain, C. P., and Mulch, L. A. (2016). Eocene‐Oligocene proto‐Cascades topography revealed by clumped (Δ47) and oxygen isotope (δ18O) geochemistry (Chumstick Basin, WA, USA). Tectonics 35, 546–564.
Mulch, A., and Chamberlain, C. P. (2007). Stable isotope paleoaltimetry in orogenic belts the silicate record in surface and crustal geological archives. Rev. Mineral. Geochem. 66 (1), 89–118. doi:10.2138/rmg.2007.66.4
Mustoe, G. E., and Leopold, E. B. (2014). Paleobotanical evidence for the post-Miocene uplift of the Cascade Range. Can. J. Earth Sci. 51, 809–824.
O'Neil, J. R., Clayton, R. N., and Mayeda, T. K. (1969). Oxygen isotope fractionation in divalent metal carbonates. J. Chem. Phys. 51 (12), 5547–5558. doi:10.1063/1.1671982
Orr, E. L., and Orr, W. N. (1999). Geology of Oregon. 5th Edition. Dubuque, Iowa: Kendall Hunt Publishing Company
Pesek, M. E., Perez, N. D., Meigs, A., Rowden, C. C., and Giles, S. M. (2020). Exhumation timing in the Oregon Cascade Range decoupled from deformation, magmatic, and climate patterns. Tectonics 39 (9), e2020TC006078. doi:10.1029/2020tc006078
Priest, G. R. (1990). Volcanic and tectonic evolution of the Cascade volcanic arc, central Oregon. J. Geophys. Res. 95 (B12), 19583–19599. doi:10.1029/jb095ib12p19583
Prothero, D. R., Draus, E., and Foss, S. E. (2006). Magnetic stratigraphy of the lower portion of the middle Miocene Mascall Formation, central Oregon. PaleoBios 26 (1), 37–42.
Quade, J., Garzione, C., and Eiler, J. (2007). Paleoelevation reconstruction using pedogenic carbonates. Rev. Mineral. Geochem. 66 (1), 53–87. doi:10.2138/rmg.2007.66.3
Reidel, S. P., Camp, V. E., Tolan, T. L., and Martin, B. S. (2013). The Columbia River flood basalt province: stratigraphy, areal extent, volume, and physical volcanology. Geol. Soc. Am. Spec. Pap. 497, 1–43. doi:10.1130/2013.2497(1)
Reiners, P. W., Ehlers, T. A., Garver, J. I., Mitchell, S. G., Montgomery, D. R., Vance, J. A., et al. (2002). Late Miocene exhumation and uplift of the Washington cascade range. Geology 30 (9), 767–770. doi:10.1130/0091-7613(2002)030<0767:lmeauo>2.0.co;2
Retallack, G. J. (2004a). Late Oligocene bunch grassland and early Miocene sod grassland paleosols from central Oregon, USA. Palaeogeogr. Palaeoclimatol. Palaeoecol. 207 (3–4), 203–237. doi:10.1016/j.palaeo.2003.09.027
Retallack, G. J. (2004b). Late Miocene climate and life on land in Oregon within a context of Neogene global change. Palaeogeogr. Palaeoclimatol. Palaeoecol. 214 (1–2), 97–123. doi:10.1016/s0031-0182(04)00394-3
Retallack, G. J., Bestland, E. A., and Fremd, T. J. (1999). Eocene and Oligocene paleosols of central Oregon. Boulder, Colorado: Geological Society of America, 344
Retallack, G. J., Tanaka, S., and Tate, T. (2002). Late Miocene advent of tall grassland paleosols in Oregon. Palaeogeogr. Palaeoclimatol. Palaeoecol. 183 (3–4), 329–354. doi:10.1016/s0031-0182(02)00250-x
Retallack, G. J., Wynn, J. G., and Fremd, T. J. (2004). Glacial-interglacial-scale paleoclimatic change without large ice sheets in the Oligocene of central Oregon. Geology 32 (4), 297–300. doi:10.1130/g20247.1
Robinson, P. T., Brem, G. F., and McKee, E. H. (1984). John Day Formation of Oregon: a distal record of early Cascade volcanism. Geology 12 (4), 229–232. doi:10.1130/0091-7613(1984)12<229:jdfooa>2.0.co;2
Robinson, P. T., Walker, G. W., and McKee, E. H. (1990). “Eocene (?), Oligocene, and lower Miocene rocks of the blue mountains region,” in Geology of the blue mountains region of Oregon, Idaho, and Washington: cenozoic geology of the blue mountains region. Editor G. W. Walker (Reston, Virginia: US Geological Survey), 29–62.
Rowley, D. B., and Garzione, C. N. (2007). Stable isotope-based paleoaltimetry. Annu. Rev. Earth Planet. Sci. 35, 463–508. doi:10.1146/annurev.earth.35.031306.140155
Sanyal, P., Bhattacharya, S. K., and Prasad, M. (2005). Chemical diagenesis of Siwalik sandstone: isotopic and mineralogical proxies from Surai Khola section, Nepal. Sediment. Geol. 180 (1–2), 57–74. doi:10.1016/j.sedgeo.2005.06.005
Savin, S. M. (1982). “Stable isotopes in climatic reconstructions,” in Climate and Earth history. Washington, DC: Natl. Acad. Press, 164–171.
Scanlon, D. P., Bershaw, J., Wells, R. E., and Streig, A. R. (2019). The spatial and temporal evolution of the Portland and tualatin forearc basins, Oregon, USA. MS Thesis. Oregon: Portland State University.
Sherrod, D. R., and Smith, J. G. (2000). Geologic map of upper Eocene to Holocene volcanic and related rocks of the Cascade Range, Oregon. Washington, DC: US Geological Survey, 17
Smith, G. A., Snee, L. W., and Taylor, E. M. (1987). Stratigraphic, sedimentologic, and petrologic record of late Miocene subsidence of the central Oregon High Cascades. Geology 15 (5), 389–392. doi:10.1130/0091-7613(1987)15<389:ssapro>2.0.co;2
Snavely, P. D., MacLeod, N. S., and Wagner, H. C. (1973). Miocene tholeiitic basalts of coastal Oregon and Washington and their relations to coeval basalts of the Columbia Plateau. Geol. Soc. Am. Bull. 84 (2), 387–424. doi:10.1130/0016-7606(1973)84<387:mtboco>2.0.co;2
Streck, M. J., and Grunder, A. L. (1995). Crystallization and welding variations in a widespread ignimbrite sheet; the Rattlesnake Tuff, eastern Oregon, USA. Bull. Volcanol. 57 (3), 151–169. doi:10.1007/s004450050086
Tabor, R. W., Frizzell, V. A., Vance, J. A., and Naeser, C. W. (1984). Ages and stratigraphy of lower and middle Tertiary sedimentary and volcanic rocks of the central Cascades, Washington: application to the tectonic history of the Straight Creek fault. Geol. Soc. Am. Bull. 95 (1), 26–44. doi:10.1130/0016-7606(1984)95<26:aasola>2.0.co;2
Takeuchi, A., Hren, M. T., Smith, S. V., Chamberlain, C. P., and Larson, P. B. (2010). Pedogenic carbonate carbon isotopic constraints on paleoprecipitation: evolution of desert in the Pacific Northwest, USA, in response to topographic development of the Cascade Range. Chem. Geol. 277 (3–4), 323–335. doi:10.1016/j.chemgeo.2010.08.015
Takeuchi, A., and Larson, P. B. (2005). Oxygen isotope evidence for the late Cenozoic development of an orographic rain shadow in eastern Washington, USA. Geology 33 (4), 313–316. doi:10.1130/g21335.1
Taylor, E. M. (1990). Volcanic history and tectonic development of the Central High Cascade Range, Oregon. J. Geophys. Res. 95 (B12), 19611–19622. doi:10.1029/jb095ib12p19611
Verplanck, E. P., and Duncan, R. A. (1987). Temporal variations in plate convergence and eruption rates in the Western Cascades, Oregon. Tectonics 6 (2), 197–209. doi:10.1029/tc006i002p00197
Wells, R., Bukry, D., Friedman, R., Pyle, D., Duncan, R., Haeussler, P., et al. (2014). Geologic history of Siletzia, a large igneous province in the Oregon and Washington Coast Range: correlation to the geomagnetic polarity time scale and implications for a long-lived yellowstone hotspot. Geosphere 10 (4), 692–719. doi:10.1130/ges01018.1
Wells, R. E., and McCaffrey, R. (2013). Steady rotation of the Cascade arc. Geology 41 (9), 1027–1030. doi:10.1130/g34514.1
Wells, R. E., Weaver, C. S., and Blakely, R. J. (1998). Fore-arc migration in Cascadia and its neotectonic significance. Geology 26 (8), 759–762. doi:10.1130/0091-7613(1998)026<0759:famica>2.3.co;2
Wolfe, J. A. (1994). Tertiary climatic changes at middle latitudes of western North America. Palaeogeogr. Palaeoclimatol. Palaeoecol. 108 (3–4), 195–205. doi:10.1016/0031-0182(94)90233-x
Yeh, H.-W., and Savin, S. M. (1977). Mechanism of burial metamorphism of argillaceous sediments: 3. O-isotope evidence. Geol. Soc. Am. Bull. 88 (9), 1321–1330. doi:10.1130/0016-7606(1977)88<1321:mobmoa>2.0.co;2
Keywords: paleoclimate, cascades, stable isotope, topography, oregon coast range
Citation: McLean A and Bershaw J (2021) Molecules to Mountains: A Multi-Proxy Investigation Into Ancient Climate and Topography of the Pacific Northwest, USA. Front. Earth Sci. 9:624961. doi: 10.3389/feart.2021.624961
Received: 01 November 2020; Accepted: 18 February 2021;
Published: 26 March 2021.
Edited by:
Alexis Licht, University of Washington, United StatesReviewed by:
Aude Gebelin, University of Plymouth, United KingdomGregory Retallack, University of Oregon, United States
Copyright © 2021 McLean and Bershaw. This is an open-access article distributed under the terms of the Creative Commons Attribution License (CC BY). The use, distribution or reproduction in other forums is permitted, provided the original author(s) and the copyright owner(s) are credited and that the original publication in this journal is cited, in accordance with accepted academic practice. No use, distribution or reproduction is permitted which does not comply with these terms.
*Correspondence: Alexander McLean, YW1jbGVhbjE5MTdAZ21haWwuY29t