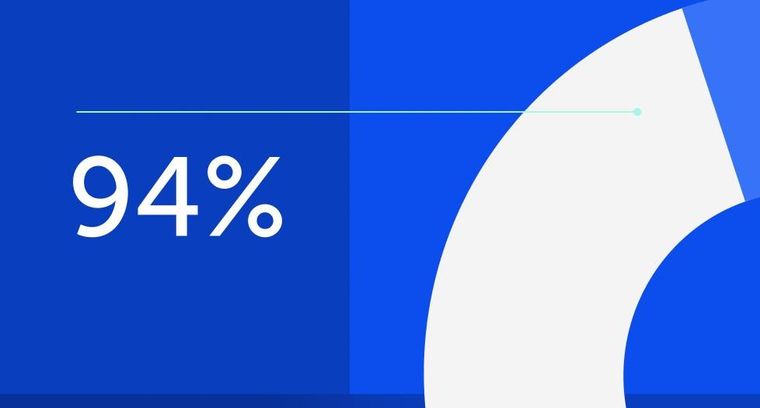
94% of researchers rate our articles as excellent or good
Learn more about the work of our research integrity team to safeguard the quality of each article we publish.
Find out more
BRIEF RESEARCH REPORT article
Front. Earth Sci., 05 March 2021
Sec. Biogeoscience
Volume 9 - 2021 | https://doi.org/10.3389/feart.2021.590257
This article is part of the Research TopicThe Biogeochemistry, Biophysics, Radiobiology, and Technical Challenges of Deep Subsurface ResearchView all 15 articles
The terrestrial subsurface offers privileged sites both to search for microbial life and to observe still mostly unknown characteristic lithologies. In particular, caves represent natural laboratories to investigate unique minerogenetic processes and biotic interactions, connected to these phenomena. Manganese mineralization in cave environments provides a window to understand the complex Mn cycle and the development of microbial communities in special conditions, such as low constant temperature, absence of light and, in particular, low-energy environments. In the current study, we isolated and characterized Mn-samples taken from the cave “Grotta Grande dei Cervi,” L’Aquila, Central Italy, and we used a multidisciplinary approach to characterize them, with the purpose of understanding the biogeochemical processes in extreme environments. A chemical characterization of the samples was done by EDS; further investigations are underway with other multidisciplinary methodologies to understand whether the Mn laminae are related to biological processes. SEM investigations revealed microbial imprints, showing cell-like structures and suggesting that the cell-like shapes occur within internal laminae. A culture-independent approach was used to assess the possibility that biotic factors may be involved in the production of these mineralizations and to investigate the nature of the microbial community in these materials. A molecular approach was the first step to investigate the role of microorganisms in forming manganese oxides associated with water bearing rocks. DNA from the black deposits was extracted and sequence analyses of specimens were performed. Our data support the hypothesis that microorganisms may contribute to the mineralizations of manganese in this environment, providing new encouraging insight into the role of microorganisms in the Mn cycle and the processes of energy acquisition in unfavorable conditions, with relevant implications for astrobiology.
Caves are still understudied habitats from both exploration and scientific perspectives. These limitations constitute a real knowledge gap, recently proposed by Ficetola et al. (2019) under the name of Racovitzan impediment. Subsurface hosts a real interdisciplinary laboratory in which it is possible to investigate chemical, physical, geological, ecological and biological processes (Barton and Northup, 2007; Miller et al., 2015; Tisato et al., 2015). Subterranean environments provide many redox interfaces and stable physical-chemical environments, which in turn may promote secondary mineral precipitation and microbial growth near starvation conditions. Microbes can trigger different reactions with the rock matrix which may enhance mineral formation and dissolution through different biochemical reactions, including redox transformations (Northup and Lavoie, 2001). These peculiar microbial reactions are involved in the formation of cave manganese deposits (Boston, 2000; Northup and Lavoie, 2001; Carmichael and Bräuer, 2015). Specifically, when electron transfer reactions take place at the microbe-mineral interface, these geomicrobiological interactions might leave residues of life throughout the geological records (Naylor, 2005; Noffke, 2009; Miot et al., 2014; Corenblit et al., 2019). Typically, manganese oxides can be found in the caves, as fine-grained, poorly crystalline black/dark brown coatings and crusts, manganese flowstones, manganese stromatolites or as biofilms on rock surfaces (Carmichael and Bräuer, 2015; Kimble et al., 2018).
The subsurface offers one of the best environment to search for extant, recently alive, and long-dead microbial lifeforms and their characteristic lithologies due to its sheltering from a large part of atmospheric events (Boston et al., 2001). Subsurface fabrics, fossil bacteria preserved in minerals, mineralized nano-sized structures and biologically generated textures represent some of the “biosignature suites” which have been proposed for exobiological research (Boston et al., 2001; Naylor, 2005; Northup et al., 2011). Biomineralization studies are therefore useful for the investigation of biogenic secondary mineral deposits, which can represent useful biosignatures for the search of early life on Earth and on other planets (Boston et al., 2001; Naylor, 2005; Miller et al., 2015; Tisato et al., 2015; Corenblit et al., 2019). The interest in these environments has also reached several space agencies, enough to develop research and training projects right inside the caves (e.g. PANGEA-X project, coordinated by ESA) (Miller et al., 2018; Miller et al., 2020). If these subsurface minerals are firmly recognized as biogenic activity indicators on Earth, then exobiological research is today focused on the millimeter-sized spherules revealed by NASA’s Opportunity rover in Meridiani Planum, a Martian region once covered by iron-rich waters.
As suggested by Boston et al. (2001), “biosignature suites” should be detectable at both macroscopic and microscopic level. This combination of features could be essential in the design of instrumentation and strategies for robotic, artificially intelligent and human-crewed extra-terrestrial missions (Boston, 2000; Boston et al., 2001). Thanks to recent developments of rover, orbiter and other technologies, now it is possible to observe, detect and quantify Martian landform characteristics at all scales (from mm to km) and in 2D/3D dimensions. The Perseverance rover (from NASA) launched in July 2020 and the ExoMars rover (from ESA/Roscosmos) scheduled to be launched in 2022, are equipped with devices capable of ascertaining surface and subsurface putative biogeomorphological signatures of life. With these instruments, it will be possible to investigate outcrops, rocks, and substrate debris and their macroscopic texture, structure, and layering (Coates et al., 2017; Vago et al., 2017; Corenblit et al., 2019).
Recently, the explorations on Mars with robotic tools increased the attention in Mn-rich rock varnishes of Earth, interesting for their involvement in the terrestrial and extra-terrestrial Mn biogeochemical cycling (Northup et al., 2010). One of the keys to establishing the biological origin for black rock deposits is to obtain more data from the laminae, containing a major concentration of manganese oxides. Currently, no detailed and systematic geomicrobiological explorations of most known caves have been carried out. This fragmentation of knowledge is primarily due to the difficulties of accessing a cave as well as to the lack of communication between different disciplines (Miot et al., 2014). In this context, highlytechnical sampling could represent a challenge to providing this information since the deepest areas are less sampled due to access difficulties. Thus, more complete and comprehensive knowledge of the geomicrobiology and biogeochemistry of caves, as well as biosignature identification, are needed. In this perspective, we applied an integrative and multi-scaled approach, applied to samples collected in a far, deep zone of a cave “Grotta Grande dei Cervi,” located in L’Aquila province in Central Italy. A series of tools from geology, mineralogy, geochemistry, microscopy, informatics and molecular microbiology was used: 1) to characterize particular black deposits on the wall of the cave; 2) to identify and characterize the microbial communities within black deposits using new powerful molecular tools; 3) to recognize microbial fabrics or fossil bacteria as valuable biosignatures for astrobiology by using innovative and experimental approaches.
The “Grotta Grande dei Cervi” is located in the middle portion of the Monti Carseolani ridge, 60 km NE of Rome, in Central Apennines (Italy). A well-structured cave system is found within the carbonatic ridge, known as Pietrasecca karst system (whose “Grotta Grande dei Cervi” and the Ovito di Pietrasecca represent the most important caves). This system evolved in a ridge formed by Upper Cretaceous-Miocene limestones and younger flysch deposits, bounded by a set of faults trending NE-SE Agostini and Piccini, 1994. The caves proceed along with these fractures, which occur transversely to the ridge. Part of both caves shows an evident vadose morphology. These features suggest a fast development under a high hydraulic gradient with significant erosion activity. The sampling of manganese deposits was conducted within “Grotta Grande dei Cervi”. The cave entrance opens at an altitude of 858 m a.s.l. (42°8′9.136″ N 13°7′43.558″ E), has an overall height difference of 107 m (+6/−113 m) and a linear extent of 1875 m. “Grotta Grande dei Cervi” cave also represents the paleoponor of the karst system, which is now active with the poor of the Ovito di Pietrasecca.
In this study, the geomicrobiology of the ferromanganese deposits was investigated within a poorly studied epigenetic cave system, located in the cave-rich Central Apennine karst region. Manganese oxides within “Grotta Grande dei Cervi” occur as black crusts on walls and floors and can be observed in the inner part of the cave (highlighted with a circle in Supplementary Figure S1). Five different samples replications were collected in the areas above a flow of water where was a Mn patina on the wall (Supplementary Figures S2, S3) during the fall season in 2019. The sampling area is located in the unsaturated zone, at 50 m of depth and 1 km from the entrance. Speleological techniques were used to reach the sampling site, with the scientific team backed by a group of expert speleologists. The portion of the cave holds a nearly constant air temperature, which is 8°C throughout the year and relative humidity close to saturation (97.7%). After the collection by sterile tools, all microbial samples were stored in Eppendorf tubes. The same specimens were replicated and filled with a solution of RNAlater (Ambion, Austin, TX, United States), according to the instructions of the manufacturer. A portable fridge was used for the carriage of the samples from the cave to the laboratory. All specimens were stored at −20°C until analyzed.
Black crusts samples from “Grotta Grande dei Cervi” were analyzed by scanning electron microscopy (SEM) combined with energy dispersive X-ray spectroscopy (EDS). Air-dried samples were directly mounted on sample stubs and prepared with a chromium sputter-coating to avoid surface charging, and at high vacuum, 7.00 kV. Samples were observed by a Gemini500 scanning electron microscope (SEM) (Zeiss, Germany) and EDS microanalysis were performed using a INCA X-ACT PELTIER COOLED detector (Aztec Energy, Oxford). For any sample, with GeminiSEM there is the possibility of work at variable pressure. Thanks to the Inlens detection signal at high performances, this system carries more signals and details, particularly at very low voltages. These features allow the fast acquisition of crisp images with minimum sample damage and the resolution of nanoscale details. The sample analyzed by SEM-EDS was cut, the surface polished and observed for microanalysis at low pressure. The microanalysis acquisitions were carried out at 12.00 kV.
A small portion from a SEM image, which presented microbial traces, was selected to produce 3D and 2D models. The small portion was processed in GIS environment to simplify each pixel value to a more general surface (x, y and z values represented simplified pixel values). These values were subsequently processed through Origin-pro software (Origin Lab Corporation, Northampton, MA). 3D Colormap and Contour tools were used to obtain both a planar 2D vision and a 3D graph, highlighting peaks and valleys occurring on the surface of the black crusts’ specimens.
Homogeneous samples were taken from the black deposits, stored in Eppendorf and fixed by a DNA fixer. Genomic DNA was extracted from about 0.5 g of solid phase with a bead beating technique using a “NucleoSpin® Soil kit” (Macherey Nagel, Germany), according to the manufacturer’s protocol. This kit is designed for the isolation of high molecular weight genomic DNA from microorganisms like Gram-positive and Gram-negative bacteria, archaea, fungi, and algae in soil, sludge, and sediment samples. The DNA was then filtered to remove humic acids that could inhibit the subsequent stages of amplification and sequencing. A specific 16S rRNA protocol has designed to amplify prokaryotes (bacteria and archaea) using paired-end 16S rRNA community sequencing on the Mi-Seq Illumina platform (Bio-Fab Research, Italy). The primers used in this protocol target the 16S rRNA V3 and V4 region (Mizrahi-Man et al., 2013; Choi et al., 2020). Illumina adapter overhang nucleotide sequences were added to the gene-specific sequences. The full-length primer sequences, using standard IUPAC nucleotide nomenclature, to follow the protocol targeting this region are: 16S Amplicon PCR Forward Primer = 5′ TCGTCGGCAGCGTCAGATGTGTATAAGAGACAGCCTACGGGNG GCWGCAG, 16S Amplicon PCR Reverse Primer = 5′ GTCTCGTGGGCTCG GAGATGTGTATAAGAGACAGGA CTACHVGGGTATCTAATCC. These primers were used to amplify template out of the DNA samples by PCR. MiSeq system provides on-instrument secondary analysis using the MiSeq Reporter software (MSR). The Metagenomics workflow obtained, classifies organisms from V3 and V4 amplicon using a database of 16S rRNA data. The classification was based on the SILVA 132 database (https://www.arb-silva.de/). The taxonomic assignments were then checked with LPSN service (https://lpsn.dsmz.de). The data were analyzed by QIIME2 (Bolyen et al., 2019). We analyzed the DNA of both samples separately, but since they were very similar, we then considered them as a single sample for the further elaborations.
To assess microbe-mineral interactions, biogenicity and to describe microbial features and unusual mineral formations, samples were investigated by SEM. Supplementary Figure S4 shows the optical microscope enlargements of the “Grotta Grande dei Cervi” sample analyzed by SEM and SEM-EDS. Figure 1 shows the SEM micrographs obtained from a broken Mn layer. The observations revealed microbial imprints, showing cell-like structures (Figures 1A,D) and suggesting that the cell-like shapes occur within internal laminae. Tubular empty sheaths, mineralized cells and pits or holes in the surface produced by bacterial cells also occurred on minerals (Figure 1C). Small micro borings, identified as putative paths carved by microorganisms, were observed (Figure 1B). Microscopically, the Mn patina shows a layered texture (Supplementary Figure S4) where layers of black Mn oxides, about 0.5 mm thick, alternates irregularly with interstices filled with silico-clastic materials. Furthermore, the Mn oxide layers also show an internal structure consisting of an alternation of thin laminae. SEM-EDS chemical map in Figure 2A shows that Mn is concentrated almost exclusively in the black laminated layers, but also Ca and, to a lesser extent, C seem to be enriched in these areas of the sample. This could be due to the coprecipitation of calcium carbonate (possibly in the calcite form) during the Mn oxides deposition. On the other hand, Al and Si are concentrated essentially in the interstices between the layers of Mn. This shows that the materials that fill the interstices are insoluble clastic aluminosilicates minerals (quartz, feldspars, clay minerals). Finally, Fe shows a fairly homogeneous distribution, at least in this EDS map. The SEM-EDS map in Figure 2B, is a detail of a single layer of Mn and shows that also the fine structure of these layers is an alternation of laminae of Mn oxides with small levels of clastic minerals. The deposition of Mn compounds in the cave could be a reaction to the changes in the hydrogeochemical conditions of the karst aquifer, caused by the formation of the cave and its drying. Based on chemical composition and considering the apparent correlation between Mn and Ca observed in EDS maps (Figures 2A,B), it could be inferred that the sample is composed by Mn minerals. Birnessite, todorokite or ranciéite could be the composing phases, as they are the most common minerals in this geological context.
FIGURE 1. Micrographs of rock sections under scanning electron microscope; (A) 15 K magnification that shows clear microbial imprints and pits (scale bar 1 µm); (B) 20 K magnification that shows micro borings, identified as putative paths carved by microorganisms (scale bar 1 µm); (C) 40 K magnification that shows a clear hole on the surface, probably produced by bacterial cells (scale bar 200 nm); (D) 70 K magnification that shows a microbial imprint on the surface, identified as a putative predivisional cell stage typical of staked bacteria (scale bar 200 nm).
FIGURE 2. Energy dispersive spectroscopy (EDS) elemental mapping images of a layer deposited on the rock wall, showing the distribution of chemical elements. (A) The area of the map is occupied essentially of Ca-bearing Mn oxides (MnOx on the combined map, Mn and Ca maps) intermixed with Fe-oxides and minor silicoclastic minerals (sm in the combined map and in Al map). (B) The combined map shows the layered structure of the sample where layers of quite pure Ca-bearing Mn oxides (MnOx on the combined map, Mn and Ca maps) intermixed with very minor siliciclastic minerals (sm in the combined map and in Al map). The grain qtz in the combined map is a grain of quartz, which is the most abundant minerals in the silicoclastic component. Notably, Fe appears homogeneously distributed on all the mapped area (Fe map).
Figure 3 shows the SEM image (Figure 3A) processed by Origin-pro software to obtain 2D (Figure 3B) and 3D models (Figure 3C). In the SEM images is underlined the area which presented microbial traces and that was selected for the construction of the models. The planar 2D model allows areas and perimeters measurements. These parameters can be useful for the evaluation of the potential microbial colonization area. The 3D model represents peaks and valleys. These microzones allow the calculation of 3D volumes. Thus, this 3D elaboration allowed us to simplify the SEM observations and the selection of the zones where microbial biosignatures were more probable found.
FIGURE 3. (A) micrograph of the inner section of the crust acquired by scanning electron microscopy (SEM). In the micrograph is highlighted the area processed through Origin-pro software. (B) The 2D model obtained from Origin-pro software that allowed to measure areas and perimeters (the units of measure of the axis are in pixels); (C) The 3D model obtained from Origin-pro software that allowed to represents microzones (peaks and valleys) useful for the calculation of the volumes and were useful for the detection of potential microbial colonization area (the bar colors and axes units are in pixels).
Sequencing and data processing revealed a rather large microbial community in which bacteria predominate. Bacteria accounted for 99% of the total abundance, while archaea were present to a very limited extent, less than 1%. Figure 4 shows the bar plot of genera found in the Mn-layer sample. The most common genus (31%) was represented by Bacillus, followed by Flavobacterium (5%), Massilia (3%), Nitrospira (3%), Paenisporosarcina (2%), Polaromonas (2%), Pseudomonas (2%), Lysinibacillus (2%) and Candidatus Methylomirabilis (1%). 19% was represented by unknown genera and 6% by uncultured bacteria. The remaining percentage of the community was represented by genera with an abundance of less than 1%. Among them, there was the presence of Mn-oxidazing bacteria genera, such as Caulobacter (0.4%), Pedomicrobium (0.2%), Rhodococcus (0.1%), and Hyphomicrobium (0.1%).
FIGURE 4. Taxonomy bar chart at the genus level. The genera relative percentages were calculated on the sequencing results obtained from both sequenced samples.
Caves offer the possibility to characterize and observe conversion from life to biosignature (Uckert et al., 2017). Indeed, microbes often leave detectable traces of their presence long after death (Boston et al., 2001). In addition to microfossils, microbial mats and biofilms can form biofabrics in rocks and bigger assemblies (e.g., microbialites). However, the interpretation of other life by-products (e.g., anomalous concentrations of elements, biologically fractionated isotopic signatures, and biominerals) is more difficult (Westall et al., 2015). Moreover, the detection of life traces could be further complicated by different degree of preservation and processes of post-fossilization alteration.
The implications related to the deposition of cave Mn compounds, in response to hydrogeochemical conditions’ changes, have been reported by several authors (Kotula et al., 2019 and references therein). The manganese in the cave might come from chemically reduced water infiltrated within the cave containing chemically reduced and dissolved manganese and soluble complex organic molecules. Mixing of this surface water with cave-stream water-oxygenated, neutral-to-slightly alkaline and bicarbonate-rich - may cause the water to become supersaturated with manganese. Manganese therefore may precipitate under the influence of microorganisms and under anaerobic conditions. Indeed, changes of the hydrogeochemical condition may produce an alkalinity increase and gradual conversion from reducing into oxidizing conditions. This conversion leads to Mn compounds precipitation, generally in the hydroxides form. Manganese bacteria can participate in the oxidation of Mn, exploiting the energy released from Mn(II) oxidation to Mn(III) and Mn(IV) (Kotula et al., 2019). In these environments, chemolithotrophic microorganisms may perform metal oxidation, such as manganese-oxidation, as a source of energy (Konhauser, 2007). As reported by Spilde et al. (2005), the Al-rich composition confirms that ferromanganese deposits are not speleogenesis left residues. The pH can have a role in the control of the presence of minerals inside the black deposits. Indeed, with a decrease of pH, there is an increased solubility of aluminum (higher solubility of Al3+). However, at neutrality and basic conditions, the control of pH is mainly due to Al(OH)-species. Near neutrality, Al hydroxides are almost insoluble; while, with an increase of pH there is an increase of Al hydroxides solubility. Thus, within a pH range of 6-8, Al is less soluble and hydroxy-aluminium forms are the main compounds present. With pH values lower than 6, the Al is mobilized by aluminosilicate minerals breakdown (e.g., feldspar), with a release of residues rich of Si. Furthermore, there is a pH buffer action by CaCO3-mineralized layer physical-chemically precipitated by CO2 degassing (Sjöberg et al., 2018). In summary, the abiotic Mn(III/IV) oxidation is not promoted by the acid/neutral pH that characterizes the majority of the surface environments (Spilde et al., 2005). Therefore, in the alkaline environments, there is the dominance of microbial Mn(III/IV) oxide production (Cloutier et al., 2017).
In the Mn deposit analyzed from “Grotta Grande dei Cervi,” an abundant and active microbial community was present with several genera already known to be implicated in Mn-oxidation. The most abundant genus in our sample was Bacillus, one of the most reported Mn-oxidizer genera (Spiro et al., 2010; Carmichael and Bräuer, 2015 and references therein). Among the other genera with an abundance less than 5%, those reported to be Mn-oxidizers are Flavobacterium (Sjöberg et al., 2018), Pseudomonas (Carmichael and Bräuer, 2015), Lysinibacillus (Johnson et al., 2012), Polaromonas (Northup et al., 2003), Rhodococcus (Ahmad et al., 2011), Caulobacter (Gregory and Staley, 1982), Pedomicrobium and Hyphomicrobium (Poindexter, 2006; Carmichael et al., 2013). Within this community, is interesting the presence of Caulobacter, Pedomicrobium and Hyphomicrobium genera. The imprint of a putative predivisional cell detected in the Mn layers analyzed by SEM (Figure 1D) could correspond to these dimorphic prosthecate bacteria. Pedomicrobium and Hyphomicrobium genera are both chemoheterotrophic aerobes. Moreover, Pedomicrobium Mn-oxidizers deposit manganese on their extracellular acidic polysaccharides (Spilde et al., 2006) and were found within manganese concretions and in the Lechuguilla Cave punk rock and ferromanganese deposits (Northup et al., 2003). The contribution of Archaea to the microbial communities from the manganese patinas was insignificant (0.6%). However, a few authors related their presence with manganese (Carmichael and Bräuer, 2015).
In this study, SEM-EDS investigations revealed microbial imprints, with characteristic features that showed interactions between microorganisms and minerals. These interactions can represent mineralogical signatures of life. Microbial communities in situ preservation via mineralization can be observed in cave environments, even when microorganisms are still alive (i.e., in vivo biomineralization) (Boston et al., 2001). The term biomineralization in this context refers to the intern and extern mineralization/lithification of microorganism cells and of the extracellular components of them (e.g., biofilm). Criteria for the identification of fossilized bacteria have been established by several studies (Boston et al., 2001; Iniesto et al., 2015; McMahon et al., 2018). The bacteria preservation in rocks is a valid tool for the exploration of the eldest sign of life on Earth, but also life beyond Earth. McKay et al. (1996) suggested that in Martian meteorites there is the presence of fossil bacteria. However, preparation for SEM analysis can cause microbial biomass loss, extracellular polymeric structures removal and/or dehydration, and mineral alteration. Furthermore, there is the oxidation of living cells organic compounds by manganese, many non-living elements with cell-like structures might create artefacts and microorganisms may leave only a few traces. For these reasons the determination of now alive, once alive, or never alive natural objects’ status can be ambiguous. Furthermore, SEM observations can be challenging due to artefacts created by sample preparation or operating conditions. The combination of SEM observations with a modeling program could represent a valid approach to ascertains microbial “macro-imprints” (i.e., putative paths carved by microorganisms and areas occupied by microbial clusters). Thus, the elaboration of SEM micrographs—or areas of them—with 3D models could be useful for the confirmation of biogenic imprints.
After microbe’s death, the manganese oxides-rich bacterial capsules seem to disintegrate into granules and be incorporated into the clay structures. The black deposits tubular forms look like Mn- and Fe-encrusted capsules fragments, probably produced by Mn- and Fe-oxidizing bacteria. Thus, the occurrence of Mn-rich black coatings is probably due to the activity of these bacteria (Šebela et al., 2015). For caves of North America, comparable features have been described by White et al. (2009).
A great research effort is needed in order to ascertain either the affiliation of these sheath-forming microorganisms and their metabolic capabilities, most likely related to the oxidation of mineral compounds, for tracing biological processes on early Earth. Nevertheless, the more frequently instances of dubious-fossils and pseudo-biosignatures will be documented and published by the astrobiology community, the more valued will be the speculation about biological biosignatures. Indeed, it is equally important to publish both valuable biosignature and biosignature imposters (Boston et al., 2001).
The study of primitive microbe-mineral interactions and biomineralization processes can be carried out in subsurface, an interesting environment from both a microbiological and mineralogical perspective. Several hypotheses argue that primordial life arisen in the dark early Earth and on other planets’ surfaces, thanks to protection from the UV radiation (Tisato et al., 2015). Recent results indicate traces of an early wet Mars and a volcanic activity relatively recent suggesting that organic molecules or traces of microbial life can be present on the subsurface of Mars (Miller et al., 2015). Recently, possible varnish coatings on Martian surface rocks have been discovered. These varnish coatings might protect from UV radiation and other conditions of hostile surface. This stimulates the understanding of the microorganisms’ role in the varnish formation (Northup et al., 2010). Moreover, rock varnish layers show microscopic cracks that over time would allow the percolation of any liquid water from fogs or dew over them. This aspect is of utmost interest for astrobiologists because it could allow the migration of any adventitious rock surface microorganisms into the cracks; these microorganisms might colonize the inner and underneath part of the porous rock varnish layers (DiGregorio, 2002). This migration can represent a very important mechanism of survival in hot or cold deserts and probably even on Mars. Thus, micro-borings, holes, tubes and other features related to black deposits can probably host microbial biofilms. Mats and biofilms formation tendency offers same valid benefits (e.g., cohesive community formation, environmental particles trap, immediate chemical, redox, and ionic environment change, needed materials sequester) to organisms that may be expected to live on other planets, under different biological conditions, and perhaps with other chemistries.
The literature on biogenically mediated mineral structures from caves is rather limited. Within Italy, as well as worldwide, new caves continue to be discovered, which may provide potentially unique geochemical environments to be investigated. In any case, the direct investigation of these low-accessible environments is essential, and add value to a research field that is usually more theoretical. Moreover, investigations in pristine environments, protected by law, entails low-impact methodologies similar to Planetary Protection Protocols for extraterrestrial missions. A holistic vision of the subterranean microbiome, assisted by the contribution of other disciplines, could thus clarify many elusive geomicrobiological phenomena and may represent concrete support for exobiological research. In our case, this multidisciplinary approach afforded us aclear evidence of the presence of Mn-oxidizers within the Mn-layer. More in deep studies, with a wider sampling campaign and petrographic and geochemical analyses, need be carried out. However, our preliminary findings demonstrate that the combination of SEM-EDS characterization, 16S rRNA gene sequencing and 3D modeling could represent a valid approach to evaluate the involvement of microbial component in the formation of Mn-secondary deposits.
The Sequencing results and dataset processed by QIIME2 can be found in the Figshare repository (https://doi.org/10.6084/m9.figshare.12771002.v1) as Samples “Grotte4” and “Grotte5.”
IV took the lead in data acquisition, processing, analyses and interpretation, and writing the manuscript. FM contributed to laboratory work and data acquisition. MP contributed to data acquisition, processing, manuscript writing and responding to reviews. FB collected samples and analyzed EDS data. MD coordinated and supervised the experimental work and contributed to the writing of the manuscript.
The Scientific committee of the “Club Alpino Italiano” supported the participation to the DULIA-bio Workshop 2019.
The authors declare that the research was conducted in the absence of any commercial or financial relationships that could be construed as a conflict of interest.
The authors wish to thank Maria Giammatteo and Lorenzo Arrizza for SEM-EDS analysis, the Group “Grotte e Forre Francesco de Marchi” for sampling support, the township of Carsoli (L’Aquila) and “Aliena Srl” for sampling authorization, the “uPIX—uNDER PIXel Fotografia Ipogea” for the cave pictures, Simone Bernardini (Roma Tre University) for the useful suggestions, and Cesareo Saiz-Jimenez and Valme Jurado (IRNAS-CSIC) for hosting and supporting IV.
The Supplementary Material for this article can be found online at: https://www.frontiersin.org/articles/10.3389/feart.2021.590257/full#supplementary-material.
Agostini, S., and Piccini, L. (1994). Aspetti geomorfologici ed evolutivi del sistema carsico di Pietrasecca (M. Carseolani ‐ Appennino Centrale, Italia). Ist. Ital. di Speleol. Mem. 5, 61–70.
Ahmad, M., Roberts, J. N., Hardiman, E. M., Singh, R., Eltis, L. D., and Bugg, T. D. H. (2011). Identification of DypB from Rhodococcus jostii RHA1 as a lignin peroxidase. Biochemistry 50, 5096–5107. doi:10.1021/bi101892z
Barton, H. A., and Northup, D. E. (2007). Geomicrobiology in cave environments: past, current and future perspectives. J. Cave Karst Stud. 69, 163–178.
Bolyen, E., Rideout, J. R., Dillon, M. R., Bokulich, N. A., Abnet, C. C., Al-Ghalith, G. A., et al. (2019). Reproducible, interactive, scalable and extensible microbiome data science using QIIME 2. Nat. Biotechnol. 37, 852–857. doi:10.1038/s41587-019-0209-9
Boston, P. J. (2000). “Bubbles in the rocks: natural and artificial caves and cavities as life support structures,” in Mars greenhouses: concepts and challenges: proceedings from a 1999 Workshop. Editors R. M. Wheeler, and C. Martin-Brennan (Cape Canaveral, Florida, USA: NASA Technical Memorandum), 9–17.
Boston, P. J., Spilde, M. N., Northup, D. E., Melim, L. A., Soroka, D. S., Kleina, L. G., et al. (2001). Cave biosignature suites: microbes, minerals, and Mars. Astrobiology 1, 25–55. doi:10.1089/153110701750137413
Carmichael, M. J., Carmichael, S. K., Santelli, C. M., Strom, A., and Bräuer, S. L. (2013). Mn(II)-oxidizing bacteria are abundant and environmentally relevant members of ferromanganese deposits in caves of the upper Tennessee river basin. Geomicrobiol. J. 30, 779–800. doi:10.1080/01490451.2013.769651
Carmichael, S. K., and Bräuer, S. L. (2015). “7. Microbial diversity and manganese cycling: a review of manganese-oxidizing microbial cave communities,” in In microbial life of cave systems. Editor A. S. Engel (Berlin, München, Boston: De Gruyter), 137–160. doi:10.1515/9783110339888-009
Choi, K., Choi, J., Lee, P. A., Roy, N., Khan, R., Lee, H. J., et al. (2020). Alteration of bacterial wilt resistance in tomato plant by microbiota transplant. Front. Plant Sci. 11, 1186. doi:10.3389/fpls.2020.01186
Cloutier, M. L. C., Carmichael, S. K., Carson, M. A., Madritch, M. D., and Bräuer, S. L. (2017). Carbon quantity and quality drives variation in cave microbial communities and regulates Mn(II) oxidation. Biogeochemistry 134, 77–94. doi:10.1007/s10533-017-0343-8
Coates, A. J., Jaumann, R., Griffiths, A. D., Leff, C. E., Schmitz, N., Josset, J.-L., et al. (2017). The PanCam instrument for the ExoMars rover. Astrobiology 17, 511–541. doi:10.1089/ast.2016.1548
Corenblit, D., Darrozes, J., Julien, F., Otto, T., Roussel, E., Steiger, J., et al. (2019). The search for a signature of life on Mars: a biogeomorphological approach. Astrobiology 19, 1279–1291. doi:10.1089/ast.2018.1969
DiGregorio, B. E. (2002). “Rock varnish as a habitat for extant life on Mars,” in Proc. SPIE 4495, instruments, methods, and missions for astrobiology IV. Editors R. B. Hoover, G. V. Levin, R. R. Paepe, and A. Y. Rozanov, 120–130. doi:10.1117/12.454750
Ficetola, G. F., Canedoli, C., and Stoch, F. (2019). The Racovitzan impediment and the hidden biodiversity of unexplored environments. Conserv. Biol. 33, 214–216. doi:10.1111/cobi.13179
Gregory, E., and Staley, J. T. (1982). Widespread distribution of ability to oxidize manganese among freshwater bacteria. Appl. Environ. Microbiol. 44, 509–551.
Iniesto, M., Zeyen, N., López-Archilla, A. I., Bernard, S., Buscalioni, Á. D., Guerrero, M. C., et al. (2015). Preservation in microbial mats: mineralization by a talc-like phase of a fish embedded in a microbial sarcophagus. Front. Earth Sci. 3. doi:10.3389/feart.2015.00051
Johnson, K. W., Carmichael, M. J., McDonald, W., Rose, N., Pitchford, J., Windelspecht, M., et al. (2012). Increased abundance of gallionella spp., leptothrix spp. and total bacteria in response to enhanced Mn and Fe concentrations in a disturbed southern appalachian high elevation wetland. Geomicrobiol. J. 29, 124–138. doi:10.1080/01490451.2011.558557
Kimble, J. C., Winter, A. S., Spilde, M. N., Sinsabaugh, R. L., and Northup, D. E. (2018). A potential central role of Thaumarchaeota in N-Cycling in a semi-arid environment, Fort Stanton Cave, Snowy River passage, New Mexico, United States. FEMS Microbiol. Ecol. 94, fiy173. doi:10.1093/femsec/fiy173
Konhauser, K. (2007). Introduction to geomicrobiology, microbial weathering. Oxford, Great Britain: Blackwell Publishing Ltd.
Kotula, P., Andreychouk, V., Pawlyta, J., Marynowski, L., and Jendrzejewska, I. (2019). Genesis of iron and manganese sediments in Zoloushka Cave (Ukraine/Moldova) as revealed by δ13C organic carbon. Int. J. Speleol. 48, 221–235. doi:10.5038/1827-806X.48.3.2255
McKay, D. S., Gibson, E. K., Thomas-Keprta, K. L., Vali, H., Romanek, C. S., Clemett, S. J., et al. (1996). Search for past life on Mars: possible relic biogenic activity in martian meteorite ALH84001. Science 273, 924–930. doi:10.1126/science.273.5277.924
McMahon, S., Bosak, T., Grotzinger, J. P., Milliken, R. E., Summons, R. E., Daye, M., et al. (2018). A field guide to finding fossils on Mars. J. Geophys. Res. Planets 123, 1012–1040. doi:10.1029/2017JE005478
Miller, A., Gonzalez Pimentel, J., Maurer, M., Stahl, S., Castro-Wallace, S., Bessone, L., et al. (2020). “Geomicrobiological field researching a subsurface analogue environment for future planetary caves mission,” in 3rd international planetary caves conference, San Antonio, Texas, February 18–21, 2020.
Miller, A., Gonzalez Pimentel, J., Stahl, S., Castro-Wallace, S., Sauro, F., Pozzobon, R., et al. (2018). “Exploring possible Mars-like microbial life in a lava tube from Lanzarote: preliminary results of in-situ DNA-based analysis as part of the PANGAEA-X test campaign,” in General assemblies of the European geosciences union (EGU) (Vienna, Austria: European Geosciences Union (Geophysical Research Abstracts).
Miller, A. Z., Jurado, V., Pereira, M. F. C., Fernández, O., Calaforra, J. M., Dionísio, A., et al. (2015). Cave speleothems as repositories of microbial biosignatures. EGU General Assembly Conference Abstracts. (AA(IRNAS-CSIC, Seville, Spain), AB(IRNAS-CSIC, Seville, Spain), AC(CERENA, Instituto Superior Técnico, Universidade de Lisboa, Lisbon, Portugal), AD(Grupo de Espeleología Tebexcorade-La Palma, La Palma, Spain). AE(Department of Biology and Geology, Univer), 14276, 2015. Available at: https://ui.adsabs.harvard.edu/abs/2015EGUGA.1714276M.
Miot, J., Benzerara, K., and Kappler, A. (2014). Investigating microbe-mineral interactions: recent advances in X-ray and electron microscopy and redox-sensitive methods. Annu. Rev. Earth Planet Sci. 42, 271–289. doi:10.1146/annurev-earth-050212-124110
Mizrahi-Man, O., Davenport, E. R., and Gilad, Y. (2013). Taxonomic classification of bacterial 16S rRNA genes using short sequencing reads: evaluation of effective study designs. PloS One 8, e53608. doi:10.1371/journal.pone.0053608
Naylor, L. A. (2005). “The contributions of biogeomorphology to the emerging field of geobiology,” in Geobiology: objectives, concepts, perspectives (Amsterdam, Netherlands: Elsevier), 35–51. doi:10.1016/B978-0-444-52019-7.50006-1
Noffke, N. (2009). The criteria for the biogeneicity of microbial induced sedimentary structures (MISS) in Archean and younger, sandy deposits. Earth Sci. Rev. 96, 173–180. doi:10.1016/j.earscirev.2008.08.002
Northup, D. E., Barns, S. M., Yu, L. E., Spilde, M. N., Schelble, R. T., Dano, K. E., et al. (2003). Diverse microbial communities inhabiting ferromanganese deposits in Lechuguilla and Spider caves. Environ. Microbiol. 5, 1071–1086. doi:10.1046/j.1462-2920.2003.00500.x
Northup, D. E., and Lavoie, K. H. (2001). Geomicrobiology of caves: a review. Geomicrobiol. J. 18, 199–222. doi:10.1080/01490450152467750
Northup, D. E., Melim, L. A., Spilde, M. N., Hathaway, J. J., Garcia, M. G., Moya, M., et al. (2011). Lava cave microbial communities within mats and secondary mineral deposits: implications for life detection on other planets. Astrobiology 11, 601–618. doi:10.1089/ast.2010.0562
Northup, D. E., Snider, J. R., Spilde, M. N., Porter, M. L., van de Kamp, J. L., Boston, P. J., et al. (2010). Diversity of rock varnish bacterial communities from Black Canyon, New Mexico. J. Geophys. Res. Biogeosciences 115. doi:10.1029/2009JG001107
Poindexter, J. S. (2006). “Dimorphic prosthecate bacteria: the genera Caulobacter, asticcacaulis, Hyphomicrobium, Pedomicrobium, hyphomonas and thiodendron,” in The prokaryotes. New York, NY: Springer, 72–90. doi:10.1007/0-387-30745-1_4
Šebela, S., Miler, M., Skobe, S., Torkar, S., and Zupančič, N. (2015). Characterization of black deposits in karst caves, examples from Slovenia. Facies 61, 6. doi:10.1007/s10347-015-0430-z
Sjöberg, S., Callac, N., Allard, B., Smittenberg, R. H., and Dupraz, C. (2018). Microbial communities inhabiting a rare Earth element enriched birnessite-type manganese deposit in the Ytterby mine, Sweden. Geomicrobiol. J. 35, 657–674. doi:10.1080/01490451.2018.1444690
Spilde, M. N., Northup, D. E., and Boston, P. J. (2006). “Ferromanganese deposits in the caves of the guadalupe mountains,” in Caves and karst of southeastern New Mexico. Editors L. Land, V. W. Lueth, W. Raatz, P. Boston, and D. L. Love (Socorro, New Mexico: New Mexico Geological Society), 161–166.
Spilde, M. N., Northup, D. E., Boston, P. J., Schelble, R. T., Dano, K. E., Crossey, L. J., et al. (2005). Geomicrobiology of cave ferromanganese deposits: a field and laboratory investigation. Geomicrobiol. J. 22, 99–116. doi:10.1080/01490450590945889
Spiro, T. G., Bargar, J. R., Sposito, G., and Tebo, B. M. (2010). Bacteriogenic manganese oxides. Acc. Chem. Res. 43, 2–9. doi:10.1021/ar800232a
Tisato, N., Torriani, S. F. F., Monteux, S., Sauro, F., De Waele, J., Tavagna, M. L., et al. (2015). Microbial mediation of complex subterranean mineral structures. Sci. Rep. 5, 15525. doi:10.1038/srep15525
Tomczyk-Żak, K., and Zielenkiewicz, U. (2016). Microbial diversity in caves. Geomicrobiol. J. 33, 20–38. doi:10.1080/01490451.2014.1003341
Uckert, K., Chanover, N. J., Getty, S., Voelz, D. G., Brinckerhoff, W. B., McMillan, N., et al. (2017). The characterization of biosignatures in caves using an instrument suite. Astrobiology 17, 1203–1218. doi:10.1089/ast.2016.1568
Vago, J. L., Westall, F., L. S., , Coates, A. J., Jaumann, R., Korablev, O., et al. Pasteur Instrument Teams (2017). Habitability on early Mars and the search for biosignatures with the ExoMars rover. Astrobiology 17, 471–510. doi:10.1089/ast.2016.1533
Westall, F., Foucher, F., Bost, N., Bertrand, M., Loizeau, D., Vago, J. L., et al. (2015). Biosignatures on Mars: what, where, and how? Implications for the search for martian life. Astrobiology 15, 998–1029. doi:10.1089/ast.2015.1374
Keywords: black deposits, Grotta Grande dei Cervi cave, Mn-bacteria, deep biosphere biosignatures, SEM-EDS analysis, 3D modeling, Next Generation Sequencing
Citation: Vaccarelli I, Matteucci F, Pellegrini M, Bellatreccia F and Del Gallo M (2021) Exploring Microbial Biosignatures in Mn-Deposits of Deep Biosphere: A Preliminary Cross-Disciplinary Approach to Investigate Geomicrobiological Interactions in a Cave in Central Italy. Front. Earth Sci. 9:590257. doi: 10.3389/feart.2021.590257
Received: 31 July 2020; Accepted: 11 January 2021;
Published: 05 March 2021.
Edited by:
Geoffrey Battle Smith, New Mexico State University, United StatesReviewed by:
Huan Cui, Université de Paris, FranceCopyright © 2021 Vaccarelli, Matteucci, Pellegrini, Bellatreccia and Del Gallo. This is an open-access article distributed under the terms of the Creative Commons Attribution License (CC BY). The use, distribution or reproduction in other forums is permitted, provided the original author(s) and the copyright owner(s) are credited and that the original publication in this journal is cited, in accordance with accepted academic practice. No use, distribution or reproduction is permitted which does not comply with these terms.
*Correspondence: Marika Pellegrini, bWFyaWthLnBlbGxlZ3JpbmlAdW5pdmFxLml0
Disclaimer: All claims expressed in this article are solely those of the authors and do not necessarily represent those of their affiliated organizations, or those of the publisher, the editors and the reviewers. Any product that may be evaluated in this article or claim that may be made by its manufacturer is not guaranteed or endorsed by the publisher.
Research integrity at Frontiers
Learn more about the work of our research integrity team to safeguard the quality of each article we publish.