- 1Alfred‐Wegener‐Institut Helmholtz‐Zentrum für Polar- und Meeresforschung, Bremerhaven, Germany
- 2Department of Polar Science, SOKENDAI (The Graduate University for Advanced Studies), Tachikawa, Japan
- 3National Institute of Polar Research, Tachikawa, Japan
- 4Department of Geosciences, Eberhard Karls University, Tübingen, Germany
Insoluble and soluble impurities, enclosed in polar ice sheets, have a major impact on the deformation behaviour of the ice. Macro- and Micro-scale deformation observed in ice sheets and ice cores has been retraced to chemical loads in the ice, even though the absolute concentration is negligible. And therefore the exact location of the impurities matters: Allocating impurities to specific locations inside the ice microstructure inherently determines the physical explanation of the observed interaction between chemical load and the deformational behaviour. Both, soluble and non-soluble impurities were located in grain boundaries, triple junctions or in the grain interior, using different methods, samples and theoretical approaches. While each of the observations is adding to the growing understanding of the effect of impurities in polar ice, the growing number of ambiguous results calls for a dedicated and holistic approach in assessing the findings. Thus, we here aim to give a state of the art overview of the development in microstructural impurity research over the last 20 years. We evaluate the used methods, discuss proposed deformation mechanisms and identify two main reasons for the observed ambiguity: 1) limitations and biases of measurement techniques and 2) the physical state of the analysed impurity. To overcome these obstacles we suggest possible approaches, such as the continuous analysis of impurities in deep ice cores with complementary methods, the implementation of these analyses into established in-situ ice core processing routines, a more holistic analysis of the microstructural location of impurities, and an enhanced knowledge-transfer via an open access data base.
1 Introduction
1.1 Impurities in Polar Snow, Firn and Ice
Ice from polar regions is an extraordinary climate archive of information of the past atmosphere of our planet. Therefore, ice from the ice sheets of Greenland and Antarctica is of interest for a vast amount of different studies regarding our climate, the environment and the dynamics of large bodies of ice.
Snow, accumulated at the surface, is compacted by overlaying layers and eventually transformed into ice. Glacier ice consists of a large number of grains with different sizes, shapes and lattice orientations. Adjacent grains are separated by interfaces called grain boundaries, planar lattice defects which can change dynamically and through various processes. A portion of the gas and impurity signature from the initial accumulated snow is preserved and, with time, descends deeper into the ice column. The impurities found in deep ice originate from atmospheric aerosols of different species with unique transport histories and sources (Legrand and Mayewski, 1997).
There are two kinds of impurities in polar ice: insoluble and soluble impurities (Legrand and Mayewski, 1997; Weiss et al., 2002; Lomonaco et al., 2011). Insoluble impurities are particles of terrestrial origin, often referred to as dust, which consist of lattice-incoherent phases (e.g., of extrinsic origin) (Ashby, 1969; Alley et al., 1986a, Alley et al., 1986b; Steffensen, 1997). These phases are rejected from the ice lattice, because, contrary to liquid water, solid water is not a good solvent (Petrenko and Whitworth, 1999). The typical size of mineral-dust particles ranges from hundreds of nanometres to a few micrometres (Wegner et al., 2015). Polar ice sometimes contains larger dust particles (e.g., in the RECAP ice core) (Simonsen et al., 2019) and tephra particles (Bourne et al., 2015). Insoluble micrometre-sized impurities are called “micro-inclusions”. Layers with high micro-inclusion concentrations are called “cloudy bands” (Gow and Williamson, 1971; Svensson et al., 2005; Faria et al., 2010). These bands are often visible with the naked eye and illustrate the variable distribution of insoluble and soluble impurities inside the ice (Figure 1) (Svensson et al., 2005). Insoluble compounds are e.g.,
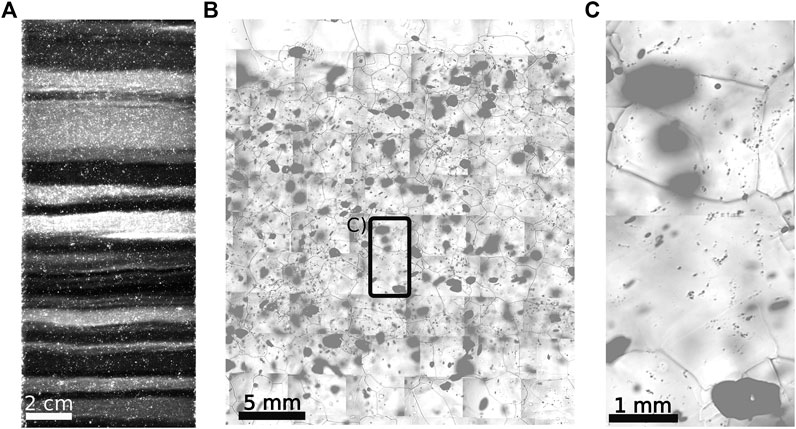
FIGURE 1. Cloudy bands - layers with high impurity concentrations and small grain size (Stoll and Weikusat, 2020). (A) Visual stratigraphy image from the EGRIP ice core (depth: 1,917 m), dark-field microscopy reveals bright layers with high impurity concentrations. (B) Detailed view of the thickest cloudy band from (A). Small dark dots are micro-inclusions, large black objects are bubbles, dark lines are grain boundary contours on the surface of the sample and bright objects are preserved hydrates. (C) Detailed view of the area indicated in (B). Combined from Figure 3 from Svensson et al., (2005), Figures 2 and 4 from Kipfstuhl et al., (2006), Figures 1 and 2 from Faria et al., (2010), and Figure 3b from Eichler et al., (2017).
To reconstruct the climate of the past, the analyses of stable water isotopes and impurities in deep ice cores have been established as standard techniques. Continuous high-resolution methods are, e.g., Ion Chromatography (IC) (Cole-Dai et al., 2006; Severi et al., 2014) and Continuous-Flow Analysis (CFA), which have been improved constantly (Röthlisberger et al., 2000; McConnell et al., 2002; Kaufmann et al., 2008). Thus, it is now possible to reconstruct the age of ice layers and the climate of the past with a high degree of certainty (Rasmussen et al., 2006; Steffensen et al., 2008; Wolff et al., 2010). This progress emphasised the importance of undisturbed climate signals and the need to understand the deformation of polar ice to reconstruct disturbed layers.
This was displayed for the Eemian interglacial in the North Greenland Eemian Ice Drilling (NEEM) ice core (Dahl-Jensen et al., 2013) and the lowest part of the European Project for Ice Coring in Antarctic Dome Concordia (EPICA Dome C) ice core (Tison et al., 2015). These examples show that a better understanding of the relationship between deformation and impurities is necessary. The physical properties of snow, firn and polycrystalline ice interact with impurities, from shallow depths to the deep parts of polar ice sheets (Dahl-Jensen and Gundestrup, 1987; Paterson, 1991; Weiss et al., 2002; Hörhold et al., 2012; Freitag et al., 2013; Fujita et al., 2014; Moser et al., 2020). While the detailed investigation of the crystallographic fabric of polar snow has only recently begun (e.g., Montagnat et al., 2020), it has been shown that porous snow offers a suitable environment (e.g., high temperature gradient and a high surface area) for many chemical species to react resulting in changes in snow structure and physical properties (Domine et al., 2008; Bartels-Rausch et al., 2014). The microstructural evolution of fine-grained firn layers is also impacted by impurities (Lomonaco et al., 2011). Hörhold et al., (2012) found a correlation between high-resolution density and
The importance of the exact location of impurities and the resulting impacts on the physical properties of ice has been debated for more than a century (e.g., Buchanan, 1887; Petit et al., 1987; Weiss et al., 2002; Shigeyama et al., 2019). CFA and IC deliver high-resolution data of the bulk content of dissolved or suspended particles in the melted sample, but composition, location, form and distribution of impurities are different in liquid water (i.e., CFA and IC samples) than in ice. Thus, information about the microstructural location of impurities is lost when melting samples. The mobility of climate proxies (e.g., impurities and water isotopes) depends on the microstructural distribution of impurities (Rempel et al., 2001). Furthermore, the location of impurities is important for physical aspects, such as grain growth, permittivity (Wilhelms et al., 1998), and electrical conductivity (Alley and Woods, 1996; Wolff et al., 1997). Different methods have been used to analyse the microstructural location of impurities in polar ice and a brief historical overview of the conducted work is presented here.
1.2 Pioneering Work on the Location of Impurities in Polar Ice
Pioneering work on impurities in sea ice was conducted by Buchanan (1887) during the Antarctic cruise of the Challenger and in the laboratory. He described the now commonly known relationship between Cl in water and the temperature of freezing. This resulted in discussions about the formation of sea ice and the processes behind the inclusion of salts, chemical compounds of an ionic assembly of anions and cations, in water and ice.
Measuring electrical conductivity, Harrison and Raymond (1976) concluded that impurities in ice from Blue Glacier, Washington State, are mainly concentrated in liquid veins at the intersection of three grains (triple junctions). Varying concentrations were found in grain boundaries and grain interiors. Impurities are more common in the grain interior of coarse grains than in finer grains. Hammer (1977) found acidic layers in Greenlandic ice from Dye 3, Crête and Hans Tausen, which was an important step towards a better understanding of the formation of distinct impurity layers and their origins. Gross et al. (1978) observed that dielectric conductivity depends on impurity concentration while static conductivity is controlled by extrinsic protons. They concluded that static conductivity increases in acids or salts, in which anions are preferentially incorporated.
Wolff et al. (1988) used Scanning electron microscope (SEM) and energy dispersive X-ray spectroscopy (EDS) microanalysis, further explained in Section 2.2, on one sample from the Antarctic Peninsula and found relatively high concentrations of S mainly in triple junctions. Mulvaney et al. (1988) found S at triple junctions in ice from Dolleman Island, Antarctica. Investigating triple junctions, Nye (1991) proposed that soluble impurities are concentrated in liquid veins between ice crystals. Mader (1992a) supported this finding by observing a variable water-vein geometry in laboratory grown ice. An accompanying optical microscopy study by Mader (1992b) showed that these acid-concentrating veins transport impurities to, and from, the sample surface. The author proposed that dissolved impurities lower the freezing point and increase the vein-size.
Raman spectroscopy, further explained in Section 2.3, was introduced to glaciology by Scherer and Snyder (1977). They measured Raman intensities of a single ice Ih crystal, laying the foundation for future Raman spectroscopy studies on ice. Fukazawa et al. (1997) conducted Raman spectroscopy on Mizuho and Nansen ice (Antarctica) and on Dye-3 ice (Greenland), showing that polar ice and pure ice show the same Raman spectrum. Fukazawa et al. (1998) used the same technique to examine two samples from Antarctic ice cores, which showed that aqueous solutions of acids can occur at temperatures found in the Antarctic ice sheet. They reported evidence of liquid
1.3 Overview of Laboratory Deformation Studies on Ice
To compare theoretical ideas and observations, laboratory deformation and grain growth experiments were conducted by e.g., Glen, (1955); Jones and Glen, (1969); Jacka (1984); Miyamoto et al., (1999); Goldsby and Kohlstedt (1997); Trickett et al., (2000); Goldsby and Kohlstedt, (2001); Iliescu et al., (2003); Song et al., (2005, 2006, 2008); Hammonds and Baker, (2016); Saruya et al., (2019). Laboratory results are only partly transferable to natural polar ice due to significant differences in boundary conditions, such as duration of experiments (months vs. thousands of years or longer and thus different strain-rates), sample size, applied (simplified) stress-fields, and concentration and species of impurities. A fully detailed section on deformation experiments goes beyond the scope of this study and only a number of key studies are briefly summarised.
Jones and Glen (1969) were among the first to dope ice with impurities to examine the change in mechanical properties. They showed that small amounts of HF soften the ice, while
Iliescu and Baker (2008) examined the effects of soluble impurities on recrystallisation and their redistribution during this process. They observed large concentrations of impurities located around migrated grain boundaries of newly formed grains, often as filaments. Grain boundary mobility decreased by one order of magnitude when ice was doped with low concentrations of
Song et al., (2005, 2006, 2008) conducted deformation experiments to compare the creep behaviour of artificial pure ice and particle-containing ice, the latter showed higher creep and nucleation rates (Song et al., 2008). Particles enhance dynamic recrystallisation due to the nucleation of new grains, which decreases the average grain size. Mechanical experiments on laboratory-grown ice doped (artificially added) with different amounts of
This brief overview shows that laboratory results are as ambiguous as observational studies. The effect of impurities on deformation is highly complicated and different hypotheses exist, which are briefly summarised below.
1.4 Early Theories on the Interplay Between Impurities and Deformation
Plastic deformation of ice crystals is primarily realized through dislocation creep, i.e., the movement of dislocations in the basal plane resulting in basal slip (Glen and Jones, 1967; Weertman, 1973; Hobbs, 1974; Petrenko and Whitworth, 1999). This slip motion is often hampered by adjacent grains with different orientations. Dislocations in deforming grains need to climb or activate non-basal slip systems in its neighbours to maintain deformation. Glen and Jones (1967) suggested the importance of impurities in ice regarding these processes and the mechanical properties of ice in general. Glen and Jones (1967); Glen (1968) and Jones and Glen (1969) reported of higher dislocation velocities and densities, directly caused by impurities and thus, an enhanced creep of ice.
There are two possible effects of impurities on ice: 1) multiplication of dislocations or the increase of their mobility (“direct control”) and 2) stress accommodating mechanisms and recrystallisation (“indirect control”). Direct control occurs if obstacles in the ice matrix, e.g., micro-inclusions, produce strain localisation, which can result in new dislocation lines (Weertman and Weertman, 1992). Glen (1968) suggested that dissolved impurities can increase dislocation mobilities by occupying lattice sites in the ice matrix, i.e. introducing protonic defects. Indirect control occurs when impurities impact the microstructure by controlling grain boundary mobility, length and energy. Accordingly, soluble and insoluble impurities are attracted by grain boundaries and the following related processes can occur (Figure 2):
(1) Drag of grain boundaries: Soluble impurities are dragged along with migrating grain boundaries, restricting grain growth as displayed in Figure 2A (Alley et al., 1986a; Alley and Woods, 1996).
(2) Zener pining: Grain boundaries are pinned to insoluble impurities (e.g., dust), which exert an extra force countering grain mobility and thus restricting grain growth (Figure 2B) (Smith, 1948; Humphreys and Hatherly, 2004).
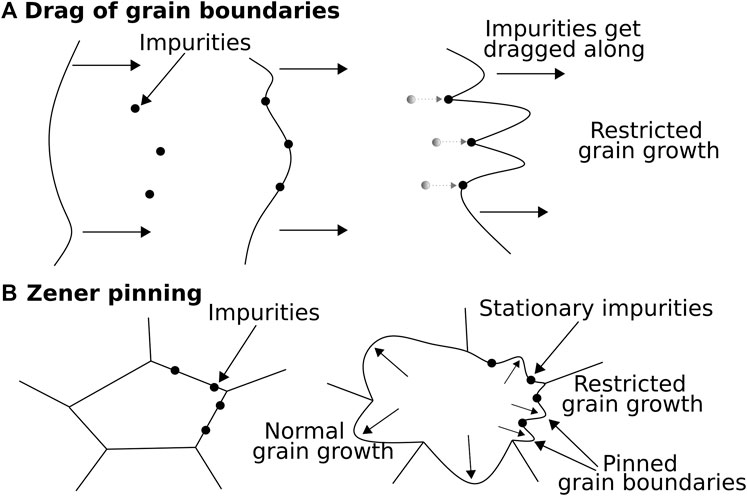
FIGURE 2. Drag of grain boundaries by impurities and Zener pinning. (A) Grain boundaries migrate towards impurities and drag them along. The drag force of the impurities slows down grain growth. (B) A grain expands as the grain boundaries migrate and impurities at grain boundaries stay fixed in place, limiting grain growth of pinned grain boundaries. Simplified and combined from Figure 3 from Alley et al. (1986a) and Figure 3 from Kelka et al. (2015).
Phenomena related to recrystallisation and grain size have been detected on the centimetre-scale. Fisher and Koerner (1986) observed that layers with high impurity concentrations were found in fine-grained crystals. Alley et al. (1986a, 1986b) explained this finding with the impact of extrinsic particles on normal grain growth (Figure 2). Harrison and Raymond (1976), Duval and Lorius (1980), Alley et al. (1986a), and Fisher and Koerner (1987) observed that regions with high bore-hole closure rates are characterised by high micro-particle concentrations and small grains sizes. Fisher and Koerner (1987) furthermore suggested that with time, recrystallisation results in the accumulation of acid impurities in quasi-liquid layers at grain boundaries. Dahl-Jensen and Gundestrup (1987) and Paterson (1991) concluded that the observed change in the behaviour of ice is related to its impurity content, impacting ice rheology, deformation rates and enhanced localised deformation.
Paterson (1991) combined previous observations with laboratory creep experiments and bore-hole deformation data to ask the apt question “Why ice-age ice is sometimes soft”. He suggested that ice in simple shear deforms on average 2.5 times faster if it originates from glacial periods compared to interglacial ice under the same conditions. Glacial ice is characterised by higher concentrations of soluble and insoluble impurities, stronger fabric and smaller grains, compared to interglacial ice. Paterson (1991) further suggested a connection between grain size, crystal preferred orientation (CPO) and impurity concentration. He concluded that 1) reduced grain sizes are caused by high impurity concentrations, which reduce grain boundary migration and grain growth, 2) the observed strong single maximum CPOs of glacial ice develop by the rotation of fine-grained crystals in simple shear and 3) simple shear parallel to bedrock can lead to easier deformation, i.e., a higher deformation rate, of ice with a distinct vertical CPO. These mechanisms differ from the view proposed by Glen and Jones (1967), Glen (1968), and Jones and Glen (1969): a strong relationship between impurity concentration and dislocation properties and strain rate. Another view was presented by Azuma and Higashi (1985) and van der Veen and Whillans (1994), proposing that strain is the main reason for CPO development and small grain sizes.
Goldsby and Kohlstedt (1997, 2001) and Cuffey et al. (2000) agree that impurities have a grain size reduction effect, but propose a different chain of development: finer grain size results in ice that is more easily deformed. The deformation of ice is thus constrained by mechanisms, which are highly affected by grain size (e.g., diffusion along grain boundaries or grain boundary sliding) (Goldsby and Kohlstedt, 1997; Cuffey et al., 2000; Goldsby and Kohlstedt, 2001). Goldsby and Kohlstedt (2001) suggested that grain boundary sliding could accommodate major parts of the stresses occurring in polar ice sheets. These hypotheses indicate that grain boundaries are the main accommodation areas of contact and stress transfer between neighbouring grains. It is still under debate if much stress accommodation is possible if the ratio of grain boundary per volume unit is high, i.e. in fine-grained ice, but recent research supports this theory (e.g., Kuiper et al., 2020b).
This brief overview illustrates that impurities are a main aspect regarding the material behaviour of polar ice, but a detailed understanding of processes, mechanisms and relevant parameters is lacking. Nevertheless, there is a mutual understanding that it is important to better understand the role of the microstructural location of impurities in ice. This aspect was thoroughly examined in the recent years and we discuss this progress in detail.
1.5 Objectives of This Review
The complex and interdisciplinary topic of the microstructural location of impurities in polar ice has been addressed by several research groups during the last two decades. The applied techniques and sample conditions (e.g., location, depth, size) varied significantly, resulting in contrary and ambiguous results. Additionally, difficulties in the in-situ analysis of impurities hampered the study of this topic. Recent technological developments partly overcame these limitations and challenge traditional views, thus implying the need for a state of the art review.
Therefore, we aim to give an overview of the recent work conducted on the microstructural location of impurities and how they impact the deformation of polar ice. Temperate, basal and bottom ice have highly different impurity-contents and boundary conditions than polar ice and are not considered. We do not discuss paleo-climate reconstructions based on impurities and restrict ourselves to deformation-related, i.e., commonly observed, impurities in polycrystalline polar ice. To this end, our major questions are as follows:
(1) What are the microstructural locations of impurities in polar ice and can we generalise those?
(2) What is the impact of impurities on the deformation of polar ice?
2 Methods to Study the Microstructural Locations of Impurities
Classic glaciochemical methods (e.g., CFA, IC) give information about the bulk chemical content of a sample. To analyse the microstructural localisation of impurities different methods are required. A variety of methods have been used to pinpoint down where, and which, impurities are located in the microstructure of ice (Table 1). These methods constantly improved, especially in the last two decades. Four established techniques, and their advantages and disadvantages, are briefly described. More details on the microstructural characterisation of ice can be found in e.g., Baker (2019).
One aspect, which holds true for all methods, is the difficulty in maintaining suitable conditions to analyse ice samples. This includes prevention of melting, controlling sublimation and surface diffusion and conserving the unaltered microstructure of the sample.
2.1 Optical Microscopy
One of the oldest methods in natural sciences is the optical microscope. Samples of different origins can be studied in detail and in several environments and conditions. The sample is placed on a stage and lenses enlarges the examined analyse object (Figure 3A), which can be viewed through eyepieces or a camera (Bardell, 2004).
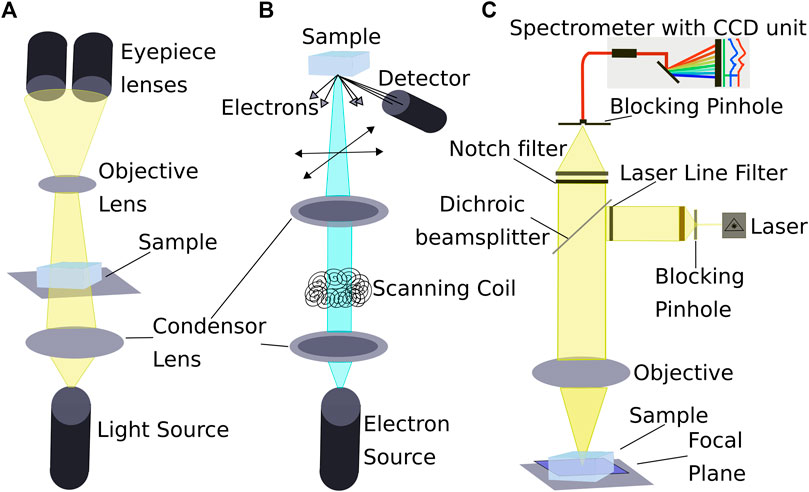
FIGURE 3. Popular methods to locate impurities in the microstructure of ice. Schematic representations of the different setups of (A) Optical microscope, (B) SEM, and (C) Confocal Raman microscope. Necessary cooling systems for cryo-use are not shown. Compiled from Reimer (1998); Dieing et al. (2011); and Markaki and Harz (2017).
Different techniques and modifications were developed, depending on the area of use. Types of illumination are e.g., bright and dark field illumination and cross-polarized light illumination. With additional equipment it is also possible to conduct fluorescence-, ultraviolet- and multiple transmission-microscopy (Wayne, 2014).
The macroscopic counterpart of dark-field microscopy is the simple, but effective ice-core line-scanner (Svensson et al., 2005). Ice cores with dimensions of 1.6 × 0.15 m can be analysed. The high resolution of this method enables the analysis of the optical stratigraphy and gives an overview about microstructural changes and impurity-rich layers (e.g., cloudy bands) as shown in Figure 1 (Faria et al., 2010; Faria et al., 2018; Westhoff et al., 2020).
Kipfstuhl et al., (2006) established a systematic large-scale microstructure mapping approach (Figure 4): a camera coupled to a microscope takes high-resolution images, which are stitched together to create detailed maps of the surface (Figure 4A), and the ice interior (Figure 4B). The sample size is normally limited to several square centimetres. These maps allow the analysis of the microstructure (e.g., grain size, grain-shape, grain boundaries, subgrain boundaries, bubbles) and the allocation of impurities (e.g., micro-inclusions in Figure 4C) for further chemical analysis (Kipfstuhl et al., 2006; Weikusat et al., 2009; Eichler et al., 2017; Eichler et al., 2019).
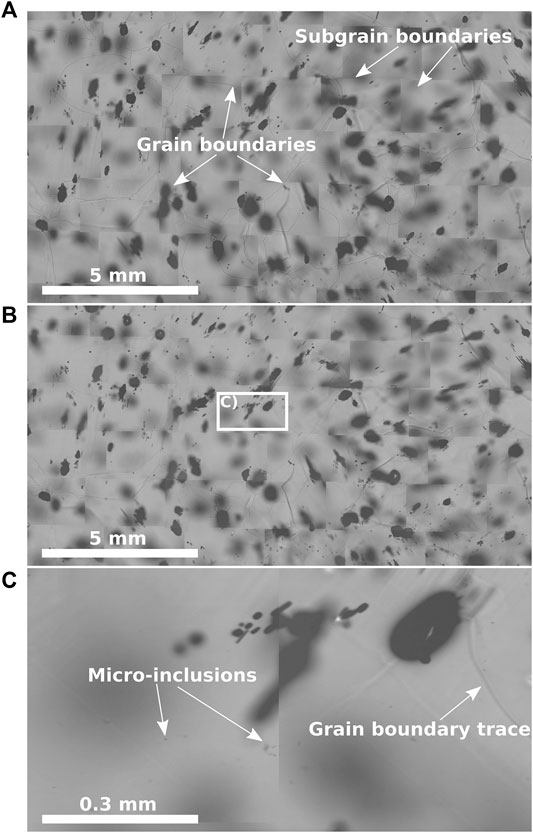
Figure 4. Different steps of microstructure mapping of a sample from a depth of 757.4 m of the EGRIP ice core, Greenland (Stoll and Weikusat, 2020). (A) Microstructure map of the surface, grain- and subgrain boundaries are indicated. (B) The same section as in (A), but with a focus depth of 500 μm to locate impurities inside the ice without the risk of surface contamination. (C) Detailed view of the indicated area in (B), arrows indicate micro-inclusions. The figure concept is based on similar figures, such as Figures 2, 3, and 5 from Kipfstuhl et al., (2006), Figures 1 and 4 from Weikusat et al. (2009), and Figure 1 from Eichler et al. (2017).
2.2 Scanning Electron Microscope and Energy Dispersive X-Ray Spectroscopy
SEMs (Figure 3B) enable the gathering of high-resolution images of the sample’s surface as displayed in Figure 5A (Obbard et al., 2003). A beam of electrons is shot at the surface and results in the emission of secondary electrons, which are detected by a secondary electron detector. SEMs are used on the polished surface of a sample, resulting in an interaction between the electron beam and the surface. Typical samples have dimensions of a few square centimetres. More details regarding the SEM technique can be found in e.g., Seiler (1983).
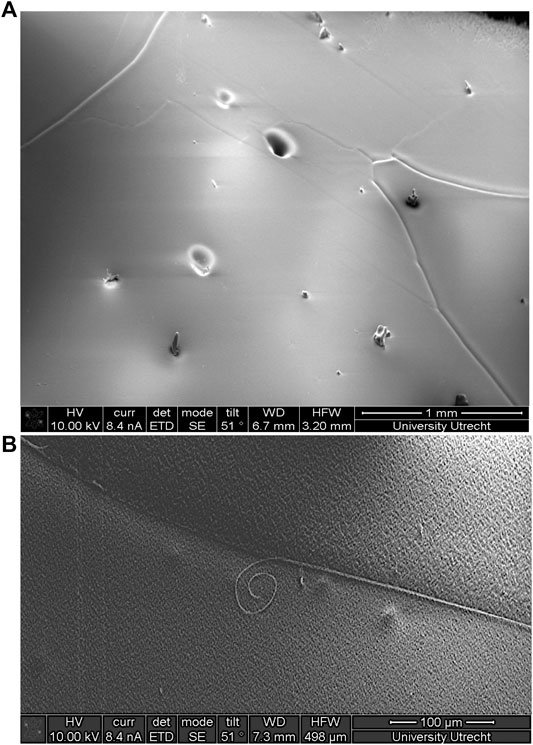
FIGURE 5. SEM-images from a depth of 2035.9 m of the European Project for Ice Coring in Antarctica in Dronning Maud Land (EDML) ice core, Antarctica (Stoll and Weikusat, 2020). (A) Sample surface after polishing and sublimation. Deep grooves are grain boundaries, shallow grooves are subgrain boundaries, roundish dents are bubbles, and objects on the surface are frost particles. (B) Filament at a grain boundary. Summarising SEM-images from, e.g., Figure 4 from Cullen and Baker (2000), Figure 6 from Cullen and Baker (2001), Figure 1 from Barnes et al. (2002a), and Figures 4a and 5a from Baker et al. (2003).
SEMs are applied to analyse impurities in ice due to their high depths of field and high magnification. Drawbacks are the very low temperature needed, the possible absorption of the spectra and the possible, vacuum-related, strong surface diffusion and the risk of a redistribution of impurities along grain boundary ridges or filaments (Figure 5B) as was shown by Barnes et al., (2003b). SEM can be coupled with EDS to analyse chemical elements of impurities (Baker and Cullen, 2003; Obbard et al., 2003) and in principle also with Electron backscatter diffraction (EBSD) (Iliescu et al., 2004; Obbard and Baker, 2007).
2.3 Raman Spectroscopy
The inelastic scattering of a monochromatic light source produces Raman spectra, which was first reported by Raman and Krishnan (1928a, b). Depending on the vibrational or rotational frequency of the scattering center, the scattered radiation is offset from the incident laser frequency and a Raman-spectrum can be obtained. An appropriate microscope is used to focus the laser, enabling the extraction of chemical information on samples at diffraction limited resolution (Barletta, 2012). Analysed samples cover a few square centimetres.
Cryogenic systems for Raman spectroscopy (Figure 3C) became well-established tools to analyse micro-particles non-destructively (Figure 6B). The possibility to focus into a sample enables an analysis free from surface contamination, surface diffusion and sublimation. Thus, the redistribution of impurities is prevented contrary to other techniques, for example the SEM. An advantage is the possibility to examine ice under naturally occurring temperatures, because no vacuum is needed (Obbard et al., 2003). Disadvantages are the superimposition of the ice spectrum itself (Figure 6C) and the Raman-inactivity of some dissociated ions of elements (e.g.,
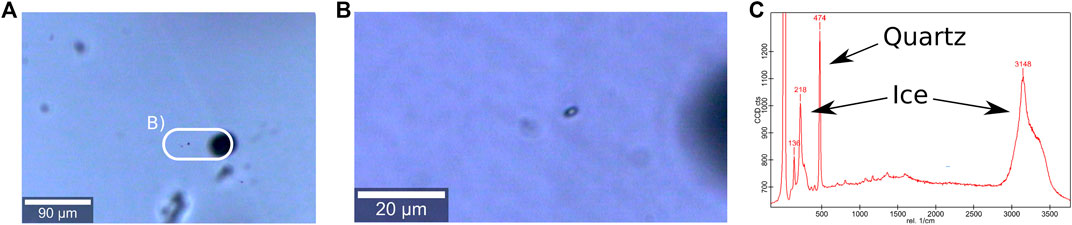
FIGURE 6. Micro-inclusions and derived Raman spectra (Stoll and Weikusat, 2020). (A) Micro-inclusions 500 µm below the sample surface. Large black spheres are bubbles, small dark dots are micro inclusions. The white bordure indicates the area shown in (B). (B) Detailed view of (A), two micro-inclusions in slightly different depths are visible. (C) Raman spectra of the focused micro-inclusion shown in (B). The signals of surrounding ice and inclusion are marked. The high peak on the left represents the used laser. Figure concept compiled after Figure 6 from Scherer and Snyder (1977), Figure 2 from Fukazawa et al. (1997), Figure 2 from Ohno et al. (2005), Figures 1 and 3 from Ohno et al. (2006), Figure 2 from Eichler et al. (2017), and Figure 4 from Eichler et al. (2019).
2.4 Laser Ablation Inductively Coupled Plasma Mass Spectrometry
Laser ablation inductively coupled plasma mass spectrometry (LA-ICP-MS) has been established for the direct analysis of frozen ice by Reinhardt et al., (2001, 2003) and has recently re-emerged for analysing elements in ice cores (Della Lunga et al., 2014; Della Lunga et al., 2017; Spaulding et al., 2017; Bohleber et al., 2020). A laser ablates the uppermost micrometres of the surface of a polished sample. The ablated material is carried into a plasma for ionisation and subsequent analysis in a mass spectrometer (Reinhardt et al., 2001). This allows the structured and continuous detection of elements. It is possible to conduct point or line scans to obtain 2-D grids of impurity locations (Della Lunga et al., 2014; Della Lunga et al., 2017). Resolution is mainly limited by the spot size of the laser, while other factors are acquisition and washout time, dosage and laser repetition rate (Bohleber et al., 2020). Typical samples have dimensions of a few square centimetres.
LA-ICP-MS enables the gathering of high-resolution data without the need to melt the ice, minimising the risk of contamination (Reinhardt et al., 2001). However, disadvantages are interfering species, such as argon from the plasma, contamination from cones and glassware and leaking gases of air.
3 State of the Art
With the new millennium a new era of impurity and microstructural research has begun. Modern techniques have started to overcome technical limitations in the microstructural analysis of impurities. Furthermore, the growing awareness of the importance of a better understanding of polar ice sheets resulted in extended funding and thus, several detailed studies have been conducted. An overview is presented in Table1.
Cullen and Baker (2000) analysed ice from the Greenland Ice Sheet project 2 (GISP2) using SEM and EDS. They examined triple junctions, grain boundaries, grain interiors, filaments and inclusions. Cullen and Baker (2000) found only little
To obtain in-situ information about the location and composition of impurities, Barnes et al. (2002a) and Barnes et al. (2002b) analysed soluble and insoluble impurities in ice from Dronning Maud Land, Dome C and GRIP using SEM and EDS. In Dronning Maud Land ice soluble impurities (mainly Na and Cl) were at grain boundaries and triple junctions. In Dome C and GRIP ice small amounts of Cl were detected at triple junctions or at grain boundaries as filaments (Barnes et al., 2002a). Only impurities in cloudy bands showed a tendency to be located at grain boundaries. Impurity concentrations in these bands might be high enough to explain the observed pinning of grain boundaries Barnes et al. (2002a).
Cullen and Baker (2002) conducted SEM and EDS microanalysis on three grains and one triple junction from large-grained Vostok ice, Antarctica. Impurities in the triple junction consisted mostly of Mg, S and O; Na and Ca were found in smaller numbers. Baker and Cullen (2002) applied SEM, optical microscopy and X-ray spectroscopy and topography on ice from GISP2. Na and Cl were mainly located in grain boundaries and triple junctions, often as filaments. Smaller numbers of Na and Cl were found in the ice lattice, accompanied by S, Mg, K and Ca.
Baker et al. (2003) examined GISP2 and Byrd Station ice, accretion ice from Vostok and river and pond ice using the method introduced by Cullen and Baker (2001). Samples from GISP2 and Byrd Station neither showed depth-related differences in impurity content nor preferred locations of impurities. Mainly Na and Cl were found in triple junctions and at filaments along many grain boundaries of GISP2 ice. In Byrd station ice Baker et al. (2003) mainly observed Mg and S in filaments at grain boundaries and in the grain interior. Mg-sulphate was concentrated at grain boundaries in Byrd ice, while NaCl was located at grain boundaries and triple junctions of GISP2 ice. Baker et al. (2003) analysed one triple junction of Vostok ice and found Mg- and S-rich crystalline structures, possibly Mg-sulphates, and Mg and S in filaments at some grain boundaries. Cl, S, Na, Ca and Mg were common impurity spots. Baker and Cullen (2003) proposed that observations by Cullen and Baker (2000, 2001, 2002); Baker and Cullen (2002); Baker et al., (2003) were artefacts caused by the sublimation of surrounding ice during SEM measurements. This would indicate that impurities are concentrated at the grain boundary plane. Baker and Cullen (2003) further confirmed the existence of impurities in the grain interior. They suggested that some impurities were already present and others moved there by localised surface diffusion.
Obbard et al. (2003) used SEM and EDS to analyse ice samples from various depths of GISP2, Byrd Station, Vostok accretion ice and pond and river ice. They observed impurities in the grain interior and at triple junctions, grain boundaries and grain boundary filaments. S was found in grain interiors, grain boundaries and triple junctions. GISP2 filaments contained mainly Na and Cl; Byrd Station and Vostok filaments mainly contained Mg and S.
More than 100 samples from different regions were analysed with SEM and EDS by Barnes et al. (2003b) to generalise patterns in the microstructural location of impurities. Cl was the most abundant element, often located in the ice lattice. Other elements, such as Na and S, were located along grain boundaries, in isolated inclusions, at the rim of air bubbles and inside grains. Barnes et al. (2003b) located Na and Cl in filaments at some grain boundaries. Only triple junctions with filaments contained impurities, except if a high bulk concentration of S was measured. This might be the result of the saturation of grain boundary layers by an impurity monolayer and the following filling of triple junctions (Barnes et al., 2003b). The authors suggest that impurities are located on grain boundaries first, and can be found at triple junctions after saturation. A follow-up study by Barnes et al. (2003a) examined the post-depositional processes of chemical layers in the upper 350 m of the Dome C ice core, Antarctica. They observed diffusive processes transporting
Baker et al. (2005) used scanning confocal optical microscopy and Raman spectroscopy on GISP2 ice. This combination of methods resulted in the discovery of
Raman spectroscopy was used by Ohno et al. (2005) to examine the chemical form and location of water-soluble impurities in ice from Dome Fuji, Antarctica. The majority of soluble impurities is confined as salts inside grains. Mainly Ca-, Na- and Mg-sulphates were found. Ohno et al. (2005) observed that micro-inclusions are often located away from grain boundaries (Figure 6A) while Ohno et al. (2006) characterised salt inclusions in detail. No depth-related difference in the spatial distribution and number and size of micro-inclusions was found. Inclusions were often distributed in groups within grains and were probably not relocated. Shallow ice was dominated by Na- and Mg-sulphate while Ca-sulphate was dominant in Last Glacial Maximum (LGM) ice.
Durand et al. (2006) combined observational grain size data from the EPICA Dome C ice core with a numerical grain growth model based on observations by Alley et al. (1986a) and Humphrey and Hatherly (1996). Their model includes recrystallisation processes (grain boundary energy driven normal grain growth, rotation recrystallisation) and a grain boundary pinning effect by bubbles, clathrates and impurities, i.e., dust particles. According to their model, grain growth and pinning are the controlling processes for grain size evolution until at a certain depth dislocation densities are locally high enough for rotation recrystallisation, or temperatures are high enough to allow thermally activated unpinning of dust particles (for further explanations of the processes discussed see Alley et al. (1986a) and Faria et al. (2014b). The latter processes can counteract both, normal grain growth and pinning, in intermediate and deep depth regimes. Durand et al. (2006) suggested that soluble impurities do not have to lead to slower grain growth, while insoluble impurities (i.e., dust) show a significant pinning effect on grain boundaries.
A variety of methods (digital image analysis, IC, SEM, EDS and EBSD) were used by Obbard and Baker (2007) to analyse the microstructure of Vostok ice. They described the texture and fabric development and identified impurities composed of Cl, K and Ca on grain boundaries. Furthermore, Obbard and Baker (2007) found Si, Al, Ca, K, Fe and S in the ice lattice. Large impurities (10–50 μm) were evenly distributed and contained Si, Al, K, Na, Ca and rarely Cl, Mg, Fe, S and Ti.
Iliescu and Baker (2008) showed that the concentration of impurities and their microstructural location impacts recrystallisation behaviour, grain growth, diffusion and texture development. Kipfstuhl et al. (2009) suggested that the classical 3-stage model over depth (normal grain growth, rotation recrystallisation and migration recrystallisation sequentially in depth) is oversimplified, refined later towards a relation with strain rate and temperature (Faria et al., 2014a; Faria et al., 2014b). Faria et al. (2010) explained this with the close relationship between impurity content and microstructure. Faria et al. (2010) analysed the microstructure and impurity content of the EPICA-DML ice core using a line-scanner and microstructure mapping. Micro-inclusions were homogeneously distributed and their amount was correlated with grain size. Faria et al. (2010) suggested the possible post-depositional formation of salt inclusions. They found indications for drag and Zener pinning only in warm basal ice. The post-depositional movements of impurities could be caused by reduced grain boundary migration velocity of the coarse-grained ice, which can be an efficient drag mechanism of slow-migrating grain boundaries (Humphreys and Hatherly, 2004).
Sakurai et al. (2009, 2010, 2011) analysed the chemistry of ice from different polar regions using Raman spectroscopy and EDS. In GRIP samples, Sakurai et al. (2009) found different solid soluble compounds.
The interaction between impurities and the nucleation of air hydrates in deep polar ice was examined by Ohno et al. (2010). They used an optical microscope to map the distribution of air bubbles, impurities and air-hydrate crystals and found a correlation between impurities and the concentration of air inclusions. Micro-inclusions were common inside hydrates and in the ice matrix. Thus, Ohno et al. (2010) suggested micro-inclusions as nucleation-aids for clathrate hydrates. Ohno et al. (2014) identified more micro-inclusions from Dome Fuji ice with Raman spectroscopy. They concluded that solid soluble Al-containing particles can be fixed in the ice matrix and that ice depth is an important factor for the formation of Al-bearing sulphate salts.
Della Lunga et al. (2014) applied cryo-cell UV-LA-ICP-MS single point and profile measurements on cloudy bands and clear ice from the NGRIP ice core. Ca was the most abundant element, followed by Na, Al, Fe, Mg, Sr and Pb. Clear ice showed higher elemental concentrations at grain boundaries or triple junctions compared to the grain interior. Contrary to this, impurities in cloudy bands did not show preferred locations. Even in fine-grained samples, impurities were evenly distributed between grain boundaries and the grain interior. Della Lunga et al. (2014) suggested that cloudy bands inhibit larger impurities (e.g., dust). Differences in particle size between cloudy bands and clear ice might cause the observed differences in particle concentration at grain boundaries, resulting in different grain boundary scavenging potential. The study relied on 50 1-D profiles and two 2-D grid measurements on five samples, the authors therefore explained that it is difficult to derive general concepts from their results.
Eichler et al. (2017) combined impurity mapping and microstructural mapping on EDML and NEEM ice, which allowed the detailed analysis of numerous impurity locations. They mapped micro-inclusions to analyse preferred locations and to examine the occurrence of Zener pinning. Impurities were not distributed homogeneously and especially cloudy bands showed high concentrations of, often clustered, micro-inclusions. No relationship between impurity concentration and grain boundaries was found. Antarctic samples contained mainly soluble sulphates while Greenlandic ice contained terrestrial minerals and black carbon. Eichler et al. (2017) discussed slow- and fast-mode pinning, the respectively long- and short-term pinning of grain boundaries by micro-inclusions. No direct evidence was found, but they assumed that fast-mode pinning occurs while the particle concentration in natural polar ice is too low for slow-mode pinning. Eichler et al. (2017) further suggested impurities might have an indirect effect on the development of grain size, by influencing dynamic recrystallisation or enhancing deformation.
A follow-up study by Eichler et al. (2019) combined Raman spectroscopy on micro-inclusions with CFA, IC and dielectric profiling data to analyse EDML ice. Micro-inclusions were often heterogeneously distributed; horizontal bands of higher impurity concentration were sometimes observed. Eichler et al. (2019) did not observe distribution patterns of chemical compounds or a relationship between the spatial distribution of grain boundaries and micro-inclusions. Small clusters and chains of micro-inclusions were found at the sub-millimetre scale, though. A clear differentiation between insoluble and soluble species was observed between Glacial and Interglacial ice. Glacial periods were characterised by up to 100 times more dust particles; two thirds of the analysed micro-inclusions were insoluble dust and one third sulphates. In Interglacial ice, 96% were sulphates while insoluble dust amounted to 4%. Eichler et al. (2019) neither found
Recently, Bohleber et al. (2020) used an improved LA-ICP-MS technique on ice from Talos Dome, Antarctica. They were able to increase the spatial resolution and speed of impurity imaging while minimising image artefacts. Bohleber et al. (2020) found Na, Co, and to a lesser degree Mg and Sr, mainly at grain boundaries. All impurities, especially Mg and Sr, were also found in grain interiors.
4 Discussion
4.1 Methodological Obstacles
The following aspects have to be kept in mind when discussing the presented studies here.
(1) Different environmental conditions prevail in a) Antarctica and Greenland, and b) at each ice core drilling site (e.g., temperature, absolute and relative impurity concentrations, pH, precipitation, deformation and recrystallisation state of grains). Particularly ice core science relies on a small number of deep ice cores, which are single point measurements of vast ice sheets. Difficult logistics and high costs impede a higher spatial resolution - especially regarding the depth-dimension of ice sheets. Most research groups have worked on ice from different geographic locations and on different depth regimes. Combining these different results is challenging, but crucial to understand the underlying processes better.
(2) The variety of methods used to find and analyse impurities hampers the straightforward comparison of results, because different advantages and disadvantages limit each method. No method is able to produce high-resolution data of the location and chemical composition of impurities without being spatially-restricted, time-consuming and independent of the impurity-state (insoluble and soluble).
(3) Limited spatial resolution of measurements and the small size of inclusions complicate the gathering of statistically robust data. In contrast to continuous measurements (e.g., CFA) microstructural impurity studies rely on small sample sizes (mm2–cm2). Derived conclusions are thus limited and difficult to translate to entire ice cores, or polar ice in general.
(4) Sample preparation, finding and analysing impurities with in-situ techniques is time-consuming and challenging. Sublimation, and thus enhanced concentration, and contamination have to be controlled at all stages of the measurements. No automated measuring routines have been developed yet due to the difficulty in detecting impurities in the microstructure of ice (e.g., low-contrast, small size of inclusions).
4.2 Where Are Impurities in the Microstructure of Polar Ice?
One focus of the last decades was the identification of location of insoluble and soluble impurities in polar ice. It has been discussed if they are mainly found throughout the ice (e.g., at dislocations, or substituted in the lattice) or at grain boundaries and triple junctions. A distinct answer to this question was supposed to be the essential step forward in understanding how impurities affect the strength, and thus the deformation, of ice, which in turn determines the disturbance of the paleoclimate record of ice.
Early studies suggested clustering of impurities along grain boundaries, in liquid-like veins at grain boundaries and in triple junctions; and the lacking of impurities in the grain-interior (Harrison and Raymond, 1976; Fisher and Koerner, 1986; Fisher and Koerner, 1987; Mulvaney et al., 1988; Nye, 1991; Mader, 1992a, b). This was challenged by recent studies, which also located impurities in the grain interior and sometimes only there (Barnes et al., 2002a; Baker et al., 2003; Obbard et al., 2003; Ohno et al., 2005; Obbard and Baker, 2007; Sakurai et al., 2009; Sakurai et al., 2011; Della Lunga et al., 2014; Eichler et al., 2017; Eichler et al., 2019). Thus, the assumed preference of impurities to cluster at grain boundaries or triple junctions has been refuted and early hypotheses turned out to be too simplified. Spatial patterns observed by e.g., Fisher and Koerner (1986); Mulvaney et al., (1988); Wolff et al., (1988) and Barnes and Wolff (2004) are valid for their examined samples, but not for polar ice in general. This might be related to the limited number of measurements resulting in simplified conclusions. Some pioneering studies were conducted on a single triple junction while several square centimetres from different depths are analysed nowadays. This progress is exemplified by the development in locating
The state of the art is that different techniques show impurities throughout many locations of ice (Table 1). Impurities can be located on grain boundaries, at triple junctions and in the grain interior (Figure 7). This ambiguous diversity in observations is mainly caused by 1) the limitations of each applied method and 2) the state of the examined impurity. These two factors are briefly discussed by referring to some key studies.
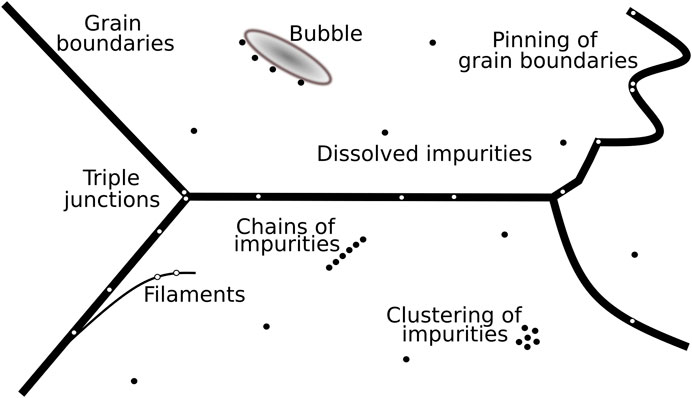
FIGURE 7. Schematic possible microstructural locations of impurities in polar ice. Insoluble impurities are displayed as black and white dots in the grain interior and on grain boundaries, respectively. Modified from Figure 7 from Barnes et al. (2003b).
Microscopes and microstructure mapping were used by e.g., Kipfstuhl et al. (2006); Faria et al. (2010); Ohno et al. (2010); and Eichler et al. (2017, 2019). They agree that more insoluble and soluble particles are found as micro-inclusions inside ice grains than on grain boundaries. Additionally, Ohno et al. (2005); Sakurai et al. (2009, 2011); Ohno et al. (2014); and Eichler et al. (2017, 2019) applied Raman spectroscopy and found water-soluble impurities, confined as micro-inclusions, mostly within grains. LA-ICP-MS measurements by Della Lunga et al., (2014) partly support these findings. In cloudy bands, most impurities were located inside grains while dissolved impurities were concentrated at grain boundaries in clean ice (Della Lunga et al., 2014). Ambiguously, this also partly supports SEM and EDS measurements by e.g., Cullen and Baker (2000, 2001); Barnes et al., (2002a, 2003b) and Barnes and Wolff (2004), which found high concentrations of dissolved impurities mainly at grain boundaries. SEM-results by Obbard and Baker (2007) are similar: soluble impurities are at grain boundaries and micro-particles in the ice lattice. Recent LA-ICP-MS results by Bohleber et al., (2020) indicate that some elements are more localised than others. All these studies show that impurities in polar ice can be located at a variety of locations (Figure 7). It is crucial to be aware of the used technique and the impurity state when interpreting measurements. Almost two decades ago, Baker and Cullen (2002) emphasised that the understanding of different techniques is an important factor in understanding the differences in the observed location of impurities. For example, optical microscopy and Raman spectroscopy are valuable tools to examine impurities in micro-inclusions, but dissolved impurities are almost impossible to find and measure. On the other hand, SEM and EDS analyses of insoluble dust particles are difficult due to their small size, which results in, e.g., limitations in resolving them and exceeding the EDS detection limit (Barnes et al., 2002a; 2003b). Similar limitations apply for each method and drawing conclusions based on results from one single method is thus not representative for polar ice. Furthermore, these findings support the significant impact of the state of impurities (soluble or insoluble) on their observed microstructural locations. This is important, because it supports the hypothesis that a method-related bias in the observed location of impurities exists. This bias depends on both, the applied method and the state of the analysed impurity.
We conclude that the microstructural location of impurities in polar ice cannot be generalised. With modern methods, it is possible to find impurities throughout many microstructural locations of polar ice as displayed in Table 1. The difference in reported observations represents the technical limitations in observing impurities rather than their absence. All in all, there is a small tendency of observed preferred locations depending on the state of impurities: undissolved micro-inclusions are often found in the grain interior and dissolved impurities at grain boundaries and in grains. Consequently, future research should focus on explanations, e.g., why are impurities at their locations, under which conditions do certain spatial patterns occur and which processes are involved? More specific research goals have to be defined while accepting, and aiming to improve, current technical limitations. A holistic methodological analysis approach is needed and a deeper examination of the impact of other conditions, such as geographical location and post-drilling artefacts.
4.3 What Is the Impact of Impurities on the Deformation of Polar Ice?
An important question is how impurities affect the physical and mechanical properties of ice, especially regarding deformation and flow (Paterson, 1991; Cullen and Baker, 2001; Iliescu and Baker, 2008). Early studies show that it is generally easier to deform impurity-loaded ice by simple shear (Fisher and Koerner, 1986; Paterson, 1991; Cuffey et al., 2000). The exact mechanisms and processes behind this observation are still unclear. Yet, the control of impurities on deformation have been formulated in the following hypotheses, which however indicate the causality dilemma associated with the impact of impurities on the deformation of polar ice: 1) impurities lead to small grains and strong CPOs, and thus enhanced deformation (Paterson, 1991), 2) impurities lead to enhanced deformation and thus, to smaller grains and strong CPOs (Glen, 1968; Jones and Glen, 1969), or 3) impurities lead to small grains and enhanced deformation, which eventually results in strong CPOs (Goldsby and Kohlstedt, 1997; Cuffey et al., 2000). The two “quantities” used in the hypotheses are grain size and CPO, because they represent measurable parameters of two major deformation mechanism groups: dislocation creep (dislocation glide + climb) and grain size sensitive creep. Grain size, as the easiest accessible state variable with respect to flow laws (Karato, 2008) has been studied extensively in ice and can have a direct effect on deformation mechanisms in the low-stress regimes of ice sheets, e.g., via activating grain-boundary sliding. CPO being the most directly observable parameter for dislocation creep is shaped dominantly by dislocation glide on the basal slip system. In turn, most of the strain in polar ice (hexagonal ice Ih) is realized trough the basal slip system (“easy glide”), but the rate of deformation is controlled by the processes handling the strain progression and incompatibilities (e.g., avoiding creation of pore space or voids) which arise from the intrinsically, highly mechanical anisotropy of ice Ih (Duval et al., 1983). The rate-control can be by the hard slip systems (“hard glide”, i.e., non-basal slip) or/and by intergranular stress-accommodating mechanisms at grain boundaries. These “accommodating” grain boundary processes (e.g., Goldsby and Kohlstedt, 2001; Schulson and Duval, 2009) are partly deformation mechanisms (e.g., dislocation climb or grain size sensitive creep mechanisms such as grain boundary sliding) and partly recrystallisation processes (e.g., grain boundary migration or formation).
4.3.1 Direct Effects: Deformation Mechanisms
Goldsby and Kohlstedt (1997); Montagnat et al. (2003); Duval and Montagnat (2006); Saruya et al. (2019); and Kuiper et al. (2020a, 2020b) propose that grain size sensitive creep takes place in fine-grained ice at high and medium stresses. Goldsby and Kohlstedt (2001) and Saruya et al. (2019) suggest that grain size has an even larger impact on ice deformation than impurities. These experiments are cost- and time-intensive, difficult to conduct and challenging to upscale to larger ice bodies. Thus, they are able to answer highly specific research questions under specific boundary conditions, e.g. tiny grain size and controlled impurity types and dispersion, however more steps are needed for generalisations. A first step towards a generalisation into natural ice conditions is the finding that grain size sensitive flow, not dislocation creep, is likely producing almost all of the deformation for large parts over the length of the NEEM ice core (Kuiper et al., 2020a, b) predicted by a composite flow law model which considers both deformation mechanisms equally (Goldsby and Kohlstedt, 2001). The grain size sensitive creep considered here is basal dislocation glide rate-limited by grain boundary sliding. Being associated with a lower stress exponent (1–2) compared to dislocation creep (3–4) the question arises, why grain size sensitive creep would make the ice softer in natural conditions. Besides the appropriate full flow parameter set for each individual deformation process (activation energy for the underlying process, material properties in “rate-factor” or “enhancement factor”), one explanation would be the lower stress level needed to initiate and effectivate deformation as shown by various deformation mechanism maps for ice (Shoji and Higashi, 1978; Frost and Ashby, 1982; Goldsby and Kohlstedt, 2001; Goldsby, 2006). Additionally, fine-grained layers in ice from Glacial periods may localise deformation as internal shear zones in the ice sheet deforming by grain size sensitive creep, again before dislocation creep may become dominant and thus effective. Grain size sensitive deformation mechanisms, e.g., grain boundary sliding, are subject of a decade-long dispute concerning the relevance for ice particularly with large grain sizes as found in nature (Goldsby and Kohlstedt, 1997; Goldsby and Kohlstedt, 2001; Duval and Montagnat, 2002; Goldsby and Kohlstedt, 2002).
The frequent observation of strong CPO developments in natural ice does, of course, proof dislocation activity, in the just described scenario, partly caused by basal dislocation glide “only” being rate-limited by grain boundary sliding. The impact of dislocations on the ice lattice of individual grains in polar ice cannot be observed directly. However, it can alter the deformation activity, which is displayed in the CPO of the bulk ice. So far, evidences of direct influence on deformation by dislocations (see Hypothesis 2 above) in natural ice are very difficult. Still, a few indications may still be worth investigating. For example, ice with a high concentration of dust, e.g., from the LGM, shows distinct changes in the deformation activity, indicated by discernible changes in the CPO orientation tensor eigenvalues (Wallbrecher, 1986). This pattern has been observed in deep ice cores, such as EPICA Dome C (Figure 5 in Durand et al., 2009), West Antarctic Ice Sheet (WAIS) (Figure 2 in Fitzpatrick et al., 2014), EDML (Figure 4 in Weikusat et al., 2017), or NEEM (Figures 2, 4, and 5 in Montagnat et al., 2014). Thus, large-scale changes in the CPO associated with climatic transitions and thus changes in impurity load and type might indicate changes in dislocation processes on the small-scale of the ice lattice caused by the interaction of impurities and dislocations. The interaction to (e.g.) soften ice with impurities can work via the amount or the velocity or mobility of dislocations. A possible mechanism group could provide e.g., multiplication of dislocations. It is a crucial requirement for the plastic deformation of polar ice. Frank and Read (1950) suggested such a mechanism and Ahmad et al. (1986) observed it in ice. The Frank-Read deformation sources occur if a segment of dislocation lying in its glide plane terminates at a step or at a node. Taking the segment out of the plane during forward motion can cause the dislocation line to spiral around this point resulting in the sweeping of dislocations across the plane (Ahmad et al., 1986). This glide needs a certain mobility of the dislocation, which depends on its type (edge, screw, mixed) (Shearwood and Whitworth, 1991; Hondoh, 2000), but also can be influenced by large amounts of lattice-solved impurities (Hu et al., 1995), because ice is proton-disordered and thus dislocations need electrical point defects to move (Glen, 1968; Glen, 1969). The characteristics of dislocation motion such as the behaviour as avalanches (Weiss, 2003; Louchet, 2004) in ice in general must have an influence, however is not transferred to polycrystalline, natural ice yet, and may be influenced by the presence of dislocations. A further process on the mobility of dislocations to mention is that impurities can act as obstacles in the slip process impeding the movement of dislocations. A strong enough external stress is needed for the dislocation to stretch and tear off from the obstacle, i.e., an impurity. The stretched dislocation might hit the next impurity creating a stretching regime (Foreman and Makin, 1966). Impurity-ice interfaces may also act as sinks for dislocations. These processes are well described for many materials (Hirth and Lothe, 1982; Weertman and Weertman, 1992), however their relevance for natural ice is difficult to proof and thus remain so far hypothetical. Indirect effects, as acting on dislocation densities rather than individual dislocations, are much better described and discussed.
4.3.2 Recrystallisation
Indirect effects of grain size on the rheology of ice can be explained considering grain boundary migration. Grain boundary migration eliminates strain-hardening features, if it is surface energy driven, but even more effective and targeting if it is strain-induced boundary migration. Strain-induced boundary migration is driven by internal strain energies resulting from the elastic lattice distortion caused by dislocations (Humphreys and Hatherly, 2004). Internal strain energy depends on the dislocation density, which in turn, if very high, leads to “entanglement” of dislocations, thus lock-down of dislocation mobility resulting in hardening of the material (references within Hirth and Lothe, 1982; Weertman and Weertman, 1992; Faria et al., 2014b). Active strain-induced boundary migration results in the focused and directed migration of grain boundaries towards highly strained areas in neighbouring grains leaving behind dislocation-free regions, which homogenised leads to recovery of the materials properties towards the state without deformation experienced by the material, and thus softening of the ice (Schulson and Duval, 2009). Strain-induced boundary migration leaves traces in the microstructure and thus can be recognised easily by bulges towards high-energy regions and irregular grain boundaries (Means, 1981; Duval and Castelnau, 1995; Weikusat et al., 2009).
The mechanisms cited for smaller grain sizes in impurity-rich ice is a reduced grain boundary migration due to drag of grain boundaries and Zener pinning. Zener pinning depends mainly on two factors: 1) the ratio of the mean distance between particles to the average grain size and 2) microstructural location of the particles (Ralph, 1990; Humphrey and Hatherly, 1996). Humphrey and Hatherly (1996) and Weygand et al. (1998) report that grain growth is not affected if inter-particle distances are large compared to small grain sizes. In the case of small inter-particle distances, the growth process can be stopped due to the pinning of grain boundaries. Fisher and Koerner (1986), Paterson (1991), Durand et al. (2006) suggested that the interaction between grain boundaries and impurities is responsible for the occurrence of impurity-rich layers of fine-grained ice with Zener pinning as a key mechanism (see also Weiss et al., 2002). Grain boundary pinning models simulating reduced grain growth by dust particles, bubbles and clathrates support this interpretation (Durand et al., 2006), even though this is only valid for certain temperature and strain rate conditions, because these models only involve static grain boundary migration driven by grain boundary surface energy and grain splitting by rotation recrystallisation. To date no dynamic grain growth law is available yet, partly due to still unconsidered processes (Steinbach et al., 2017). Being strongly temperature dependent via the grain boundary mobility, unpinning is observed in the deep parts of some ice cores (Durand et al., 2006). Grain boundaries unpinned from dust particles might explain why Eichler et al. (2019) did not find any indications of Zener pinning in the EDML ice core (below 2,000 m).
An alternative explanation for Zener pinning is the reduction of grain growth kinetics by a high soluble impurity content which can reduce the grain boundary mobility significantly, which, however, could not yet be confirmed (Weiss et al., 2002). Contrary to micro-particles, dissolved impurities are mobile enough to stick to moving grain boundaries, similar to air bubbles during normal grain growth (Azuma et al., 2012). Thus, their concentration in the grain boundary could incline with time while decreasing the grain boundary mobility. The interface energy and molecular structure of grain boundaries could also be affected by dissolved impurities, which would result in changes in the driving force for recrystallisation processes which involve grain boundary migration.
Changes in grain size often correlate with climatic transitions and therefore changes in parameters, such as conductivity, different concentrations of impurities and isotopic record. This interplay of several parameters makes it challenging to obtain key mechanisms controlling grain size change (Weiss et al., 2002). Some studies apply that a certain impurity concentration threshold is needed to impact grain size (Alley et al., 1986b; Fisher and Koerner, 1986; Jun et al., 1998; Iliescu and Baker, 2008). The presented results indicate that drag and Zener pinning can impact grain growth in ice, but generalisations are not possible, neither for certain depth regimes nor for entire ice cores. It could also be possible that impurities directly impact the strength of deformation, and small grain sizes and distinct CPOs are merely an indirect second order consequence.
The impact of dislocations on the ice lattice of individual grains in polar ice can not be observed directly. However, it can alter the deformation activity, which is displayed in the CPO of the bulk ice. Ice with a high concentration of dust, e.g., at climatic terminations, shows distinct changes in the deformation activity, indicated by strong changes in the eigenvalues. This pattern has been observed in deep ice cores, such as EPICA Dome C (Durand et al., 2009), West Antarctic Ice Sheet (WAIS) (Fitzpatrick et al., 2014), EDML (Weikusat et al., 2017), or NEEM (Montagnat et al., 2014). Thus, large-scale changes in the CPO indicate dislocations changes on the small-scale of the ice lattice.
4.3.3 Implications for Post-depositional Alteration of Impurity Records
An almost unexplored topic is the interplay between chemical reactions in ice and deformation. Bartels-Rausch et al. (2014) showed that snow is a good environment for chemical reactions to occur. Chemical reactions in ice seemed to be limited to diffusion, but mixing of impurities could also be enhanced by the flow of ice. Steffensen et al. (1997); Masson-Delmotte et al. (2010); de Angelis et al. (2013); Baccolo et al. (2018); and Eichler et al. (2019) suggest that, depending on the temporal and spatial scale, ice could not only passively store a climate signal, but act as an effective reactor resulting in post-depositional changes of the composition of impurities. Possible reactions between soluble impurities and dust particles was confirmed by Barnes and Wolff (2004). Barnes et al. (2003b) suggested post depositional movements of sulphate and chloride ions, which was later supported by Masson-Delmotte et al., (2010) and de Angelis et al., (2013). Additionally, Baccolo et al. (2018) showed that some chemical compounds are affected more by post-depositional processes than others and Eichler et al. (2019) proposed a possible break-up of Mg-sulphates during deposition or reactions inside the ice. Recent progress eventually showed that chemical reactions and alterations and spatial redistribution of impurities occur in deep ice and affect the deformation of ice (e.g., Ohno et al., 2006; Faria et al., 2010; de Angelis et al., 2013; Eichler et al., 2019). Data is scarce, but Eichler et al. (2019) suggested that dynamic recrystallisation and grain growth could result in the drag and accumulation of dissolved impurities by migrating grain boundaries. During this process dragged impurities could react and precipitate as formed salt particles. Another possibility suggested by Eichler et al. (2019) is the mixing of impurities in localised shear bands with high strain and strain rate as shown by Llorens et al. (2016); Steinbach et al. (2016) and de Riese et al. (2019). These possibilities agree with observations by e.g., Faria et al. (2010) regarding the formation of new solid inclusions with depth. However, these recent developments show that the role of chemical processes inside polar ice is not understood yet and should be taken into consideration in future studies.
4.4 Summary and Outlook
The state of the art of microstructural locations of impurities in polar ice and the effect of impurities on the deformation of ice was compiled in this review. Over the recent years, impurities of varying chemistry, were located in several different microstructural locations, ranging from grain boundaries to the grain interior. The state of the impurity plays an important role, often dissolved impurities seem to be located at grain boundaries while undissolved impurities are in the grain interior. However, the large variability in 1) analysed ice samples and 2) measurement techniques limit conclusions and rule generalisations of microstructural locations and distinct related deformation processes for polar ice in general out. Direct (e.g., multiplication of dislocations) and indirect (e.g., strain-induced boundary migration) effects of impurities on the deformation of ice have been discussed, including possible post-depositional alterations of impurities (e.g., chemical reactions and movement of ions) inside the ice. Many questions remain unanswered, but the last two decades show a large potential for future developments.
One crucial aspect is the lack of continuous measurements on the same ice core. Very few studies examined a large number of samples and, if so, samples were taken from many different locations (Barnes et al., 2003b). No study has analysed the microstructural distribution of impurities in detail over a comprehensive range of depths at one site. While CFA is often applied continuously on deep ice cores, detailed microstructural studies still only cover a few samples, which is not representative for entire ice cores. Examining several depth intervals with different methods, as done by Obbard and Baker (2007) on the Vostok ice core, could deliver a more profound understanding of relevant processes. Especially the use of complimentary methods on the same sample should be included in future studies. For example, combining microstructure-mapping, Raman spectroscopy and LA-ICP-MS or SEM + EDS analysis would allow to locate dissolved and particulate impurities on the surface and in the sample, to measure a wide range of chemical elements and compounds and to erase existing methodological uncertainties (Bohleber et al., 2020). Such a holistic analysis approach is crucial to derive more robust data, which is needed to fill the existing knowledge gaps. This is especially important to identify the locations of dissolved and undissolved impurities with more certainty. Such an approach would test, and most certainly improve, the hypothesis regarding the connection between preferred locations of impurities and their state.
Another missing link is the enhanced combination of in-situ impurity and deformation analysis. By now, deformation modes can be derived by analysing the c-axes of ice crystals in thin sections with autonomous fabric analysers (Wilson et al., 2003; Peternell et al., 2011). This method has been established as a standard procedure in deep ice core science (Wang et al., 2002; Montagnat et al., 2014; Weikusat et al., 2017). The resolution of in-situ microstructure and fabric measurements has recently increased to continuous measurements of several decimetres every 5–15 m. Thus, it would be beneficial to include consistent impurity studies in established ice core processing routines, combining it with microstructure and fabric research on the same site. Current projects, such as the East Greenland Ice Core Project (EGRIP), and future projects (e.g., Beyond EPICA-Oldest Ice) are opportunities to apply these approaches and to gather more in-situ data, which would help to prevent post-drilling and storage artefacts as presented by e.g., Eichler et al. (2017).
Furthermore, no clear distribution patterns of impurities have been observed in cloudy bands. During seasonal event, such as atmospheric storms, the increased transport and deposition of impurities results in the formation of these bands (Svensson et al., 2005). Only a few studies examined cloudy bands in detail. Within cloudy bands Della Lunga et al. (2014) found no concentration of impurities at grain boundaries. This also applies to cloudy bands in the upper 2,300 m of the EDML core while the deepest 400 m show the accumulation of micro-inclusions at grain boundaries (Faria et al., 2010). Cloudy bands are highly abundant in many ice cores and thus could have large impacts on internal deformation. Due to the lack of data it is not possible to estimate their influence in detail and to define related microstructural processes. However, cloudy bands are numerous and easy to locate (e.g., by Visual Stratigraphy as done by Westhoff et al., 2020), a well-structured analysis focusing on them would thus be feasible. Retrieving this data would imply a reasonable amount of effort and a high-potential to close some major knowledge gaps.
In addition, there are numerous parameters (e.g., changes in pH), which have been widely neglected regarding their impact on impurities and the deformation of polar ice. Bartels-Rausch et al. (2014) showed the importance of such parameters regarding chemical reactions in snow and Taylor and Alley (2004) and Fudge et al. (2016) used acidity profiles to analyse flow discontinuities and impurity translocations in Antarctic ice. A broad investigation of the material ice is a challenging task, but it becomes more and more apparent that detailed interdisciplinary analyses are needed for a better understanding of chemical and deformational processes in ice. One step is to enhance the transfer of knowledge by extending the area of research to less traditional sites. We focused on polar ice in this work, but there is also some research on different types of ice. Obbard et al. (2011) used SEM, EDS and EBSD to analyse ice samples from the Himalaya and found particles only in the grain interior. Barletta (2012) applied Raman spectroscopy to Antarctic blue ice to identify source regions of ash. Recently, Baccolo et al. (2018) successfully used synchrotron light on ice core samples to examine post-depositional processes of mineral dust. These approaches could be further expanded to the analysis of the microstructural location of impurities, enabling to gain more information of different ice types and to establish the use of new, promising methods on polar ice.
Besides possible enhancements in measurement approaches, substantial technical progress offers new possibilities to improve the measurement process itself. Recently, Baker (2019) suggested possible techniques, such as synchrotron-based diffraction contrast tomography or the electron channelling contrast imaging method, for future use to understand the microstructure of ice better. Technically it is still challenging to analyse impurities in the ice microstructure without destroying the sample, and with-it spatial information. The spatial distribution of impurities is therefore still difficult to obtain, to link it to processes regarding deformation is even more challenging. Exemplary, hunting for single micro-inclusions in ice is tedious work and requires manpower, time and a certain amount of luck. The recent advances in computer power, machine learning and image analyses might offer the chance to automate the processes of detecting and analysing particles. Consequently, a higher number of samples could be analysed and statistical uncertainties would decrease.
This approach will go hand in hand with the establishment of a open access data base. In such a data base, all conducted research on impurities in polar ice will be collected, and structured in an efficient way as displayed in Table 2. Some criteria, which would enable a structured documentation of findings, are: applied techniques, microstructural locations and chemical composition of impurities, overall impurity content, location, depth and age of samples, main c-axis pattern, grain size, boundary conditions and experienced limitations. A data base will help significantly to compare results and to find reoccurring spatial patterns, which could result in first limited generalisations, e.g., for single ice cores, microstructural phenomena, such as cloudy bands, or specific periods of time.
Summarising, former studies revealed ambiguous results on the location and impact of impurities in polar ice, with improved technologies this discrepancy grew stronger. We identify the major reason in the very variable ice samples analysed and the measurement techniques. To fill the discussed knowledge gaps regarding the microspatial distribution of impurities and their impact on polar ice, a more holistic approach is needed. Location of impurities in deep ice cores should be analysed in higher resolution and with complementary methods to minimise method-related observational biases. Additionally, a more structured organization of the available data is needed, such as a freely-available data base. Improving interdisciplinary cooperation and more efficient know-how transfer is crucial for a better understanding of polar ice.
Author Contributions
Initial concept by NS. JE, MH, WS, and IW contributed to the discussion and interpretation of the results. The manuscript was written by NS with contributions of all co-authors.
Funding
This work was carried out as part of the Helmholtz Junior Research group “The effect of deformation mechanisms for ice sheet dynamics” (VH-NG-802).
Conflict of Interest
The authors declare that the research was conducted in the absence of any commercial or financial relationships that could be construed as a potential conflict of interest.
Acknowledgments
We thank E. Smith, S. Wahl and J. Westhoff for proof reading and fruitful discussions. Furthermore, we thank M. Drury and G. Pennock for providing the infrastructure for Cryo-SEM work, which was conducted at EM square, the electron microscopy facility of Utrecht University.
References
Ahmad, S., Ohtomo, M., and Whitworth, R. W. (1986). Observation of a dislocation source in ice by synchrotron radiation topography. Nature 319, 659–660. doi:10.1038/319659a0
Alley, R. B., Blankenship, D. D., Bentley, C. R., and Rooney, S. T. (1986b). Deformation of till beneath ice stream B, West Antarctica. Nature 322, 57–59. doi:10.1038/322057a0
Alley, R. B., and Woods, G. A. (1996). Impurity influence on normal grain growth in the GISP2 ice core, Greenland. J. Glaciol. 42, 255–260.
Alley, R., Perepezko, J., and Bentley, C. R. (1986a). Grain growth in polar ice: I. Theory. J. Glaciol. 32, 415–424. doi:10.3189/S0022143000012132
Ashby, M. F. (1969). Boundary defects and the mechanism of particle movement through crystals. Scripta Metall. 3, 843–848 doi:10.1016/0036-9748(69)90192-6
Azuma, N., and Higashi, A. (1985). Formation processes of ice fabric pattern in ice sheets. Ann. Glaciol. 6, 130–134. doi:10.1145/3209219.3209221
Azuma, N., Miyakoshi, T., Yokoyama, S., and Takata, M. (2012). Impeding effect of air bubbles on normal grain growth of ice. J. Struct. Geol. 42, 184–193. doi:10.1016/j.jsg.2012.05.005
Baccolo, G., Cibin, G., Delmonte, B., Hampai, D., Marcelli, A., Stefano, E. D., et al. (2018). The contribution of synchrotron light for the characterization of atmospheric mineral dust in deep ice cores : preliminary results from the Talos Dome ice core (East Antarctica). Condensed Matter. 3. doi:10.3390/condmat3030025
Baker, I., Cullen, D., and Iliescu, D. (2003). The microstructural location of impurities in ice. Can. J. Phys. 81, 1–9. doi:10.1139/p03-030
Baker, I., and Cullen, D. (2003). SEM/EDS observations of impurities in polar ice: artifacts or not?. J. Glaciol. 49, 184–190.
Baker, I., and Cullen, D. (2002). The structure and chemistry of 94 m Greenland Ice Sheet Project 2 ice. Ann. Glaciol. 35, 224–230. doi:10.3189/172756402781816627
Baker, I., Iliescu, D., Obbard, R., Chang, H., Bostick, B., and Daghlian, C. P. (2005). Microstructural characterization of ice cores. Ann. Glaciol. 42, 441–444. doi:10.3189/172756405781812853
Baker, I. (2019). Microstructural characterization of snow, firn and ice. Phil. Trans. Math. Phys. Eng. Sci. 377, 20180162. doi:10.1098/rsta.2018.0162
Bardell, D. (2004). The invention of the microscope. Bios. 75, 78–84. doi:10.1893/0005-3155(2004)7578:TIOTM2.0.CO;2
Barletta, R. E. (2012). Raman analysis of blue ice tephra : an approach to tephrachronological dating of ice cores Raman analysis of blue ice tephra : an approach to tephrachronological dating of ice cores. Antarct. Sci. 24, 202–208. doi:10.1017/S0954102011000885
Barnes, P. R., Mulvaney, R., Wolff, E. W., and Robinson, K. (2002b). A technique for the examination of polar ice using the scanning electron microscope. J. Microsc. 205, 118–124. doi:10.1046/j.0022-2720.2001.00981.x
Barnes, P. R., Wolff, E. W., Mallard, D. C., and Mader, H. M. (2003b). SEM studies of the morphology and chemistry of polar ice. Microsc. Res. Tech. 62, 62–69. doi:10.1002/jemt.10385
Barnes, P. R. F., Mulvaney, R., Robinson, K., and Wolff, E. W. (2002a). Observations of polar ice from the Holocene and the glacial period using the scanning electron microscope. Ann. Glaciol. 35, 559–566. doi:10.3189/172756402781816735
Barnes, P. R. F., and Wolff, E. W. (2004). Distribution of soluble impurities in cold glacial ice. J. Glaciol. 50, 311–324. doi:10.3189/172756504781829918
Barnes, P. R. F., Wolff, E. W., Mader, H. M., Udisti, R., Castellano, E., and Röthlisberger, R. (2003a). Evolution of chemical peak shapes in the Dome C, Antarctica, ice core. J. Geophys. Res. 108, 4126. doi:10.1029/2002JD002538
Bartels-Rausch, T., Jacobi, H.-W., Kahan, T. F., Thomas, J. L., Thomson, E. S., Abbatt, J. P. D., et al. (2014). A review of air–ice chemical and physical interactions (AICI): liquids, quasi-liquids, and solids in snow. Atmos. Chem. Phys. 14, 1587–1633. doi:10.5194/acp-14-1587-2014
Bohleber, P., Roman, M., Šala, M., and Barbante, C. (2020). Imaging the impurity distribution in glacier ice cores with LA-ICP-MS. J. Anal. At. Spectrom. 35 (10), 2204–2212. doi:10.1039/d0ja00170h
Bourne, A., Cook, E., Abbott, P., Seierstad, I., Steffensen, J., Svensson, A., et al. (2015). A tephra lattice for Greenland and a reconstruction of volcanic events spanning 25–45 ka b2k. Quat. Sci. Rev. 118, 122–141. doi:10.1016/j.quascirev.2014.07.017
Cole-Dai, J., Budner, D. M., and Ferris, D. G. (2006). High speed, high resolution, and continuous chemical analysis of ice cores using a melter and ion chromatography. Environ. Sci. Technol. 40, 6764–6769. doi:10.1021/es061188a
Cuffey, K. M., Conway, H., Gades, A., Hallet, B., Raymond, C. F., and Whitlow, S. (2000). Deformation properties of subfreezing glacier ice : role of crystal size, chemical impurities, and rock particles inferred from in situ measurements. J. Geophys. Res. 105, 27895–27915. doi:10.1029/2000JB900271
Cullen, D., and Baker, I. (2001). Observation of impurities in ice. Microsc. Res. Tech. 55, 198–207. doi:10.1002/jemt.10000
Cullen, D., and Baker, I. (2002). Observation of sulphate crystallites in Vostok accretion ice. Mater. Char. 48, 263–269. doi:10.1016/S1044-5803(02)00273-5
Cullen, D., and Baker, I. (2000). The chemistry of grain boundaries in Greenland ice. J. Glaciol. 46, 703–706. doi:10.3189/S0022143000212756
Dahl-Jensen, D., Albert, M. R., Aldahan, A., Azuma, N., Balslev-Clausen, D., Baumgartner, M., et al. (2013). Eemian interglacial reconstructed from a Greenland folded ice core. Nature 493, 489–494. doi:10.1038/nature11789
Dahl-Jensen, D., and Gundestrup, N. S. (1987). Constitutive properties of ice at Dye 3. Oxfordshire, United Kingdom: Greenland. International Association of Hydrological Sciences Publication, 31–43.
de Angelis, M., Tison, J. L., Morel-Fourcade, M. C., and Susini, J. (2013). Micro-investigation of EPICA Dome C bottom ice: evidence of long term in situ processes involving acid-salt interactions, mineral dust, and organic matter. Quat. Sci. Rev. 78, 248–265. doi:10.1016/j.quascirev.2013.08.012
de Riese, T., Evans, L., Gomez-Rivas, E., Griera, A., Lebensohn, R. A., Llorens, M.-G., et al. (2019). Shear localisation in anisotropic, non-linear viscous materials that develop a CPO: a numerical study. J. Struct. Geol. 124, 81–90. doi:10.1016/j.jsg.2019.03.006
T. Dieing, O. Hollricher, and J. Toporski (2011). “Confocal Raman microscopy,” in Springer series in optical sciences. (Berlin, Heidelberg: Springer), Vol. 158. doi:10.1007/978-3-642-12522-5
Della Lunga, D., Müller, W., Rasmussen, S. O., and Svensson, A. (2014). Location of cation impurities in NGRIP deep ice revealed by cryo-cell UV-laser-ablation ICPMS. J. Glaciol. 60, 970–988. doi:10.3189/2014JoG13J199
Della Lunga, D., Müller, W., Rasmussen, S. O., Svensson, A., and Vallelonga, P. (2017). Calibrated cryo-cell UV-LA-ICPMS elemental concentrations from the NGRIP ice core reveal abrupt, sub-annual variability in dust across the GI-21.2 interstadial period. Cryosphere 11, 1297–1309. doi:10.5194/tc-11-1297-2017
Domine, F., Albert, M., Huthwelker, T., Jacobi, H., Kokhanovsky, A. A., Lehning, M., et al. (2008). Snow physics as relevant to snow photochemistry. Atmos. Chem. Phys. 8, 171–208. doi:10.5194/acp-8-171-2008
Durand, G., Gagliardini, O., Thorsteinsson, T., Svensson, A., Kipfstuhl, S., and Dahl-Jensen, D. (2006). Ice microstructure and fabric: an up-to-date approach for measuring textures. J. Glaciol. 52, 619–630. doi:10.3189/172756506781828377
Durand, G., Svensson, A., Persson, A., Gagliardini, O., Gillet-Chaulet, F., Sjolte, J., et al. (2009). Evolution of the texture along the EPICA Dome C ice core. Low Temp. Sci. 68, 91–105.
Duval, P., Ashby, M. F., and Anderman, I. (1983). Rate-controlling processes in the creep of polycrystalline ice. J. Phys. Chem. 87, 4066–4074. doi:10.1021/j100244a014
Duval, P., and Castelnau, O. (1995). Dynamic recrystallisation of ice in polar ice sheets. J. Phys. IV. 05, 193–197. doi:10.1051/jp4:1995317
Duval, P., and Lorius, C. (1980). Crystal size and climatic record down to the last ice age from Antarctic ice. Earth Planet Sci. Lett. 48, 59–64. doi:10.1016/0012-821X(80)90170-3
Duval, P., and Montagnat, M. (2002). Comment on “Superplastic deformation of ice: experimental observations” by D. L. Goldsby and D. L. Kohlstedt. J. Geophys. Res.: Solid Earth. 107, 4–1. doi:10.1029/2001JB000946
Duval, P., and Montagnat, M. (2006). “Physical deformation modes of ice in glaciers and ice sheets,” in Glacier science and environmental change. Malden, MA, United States: Blackwell Publishing, Wiley Online Books, 303–308. doi:10.1002/9780470750636.ch59
Eichler, J., Kleitz, I., Bayer-Giraldi, M., Jansen, D., Kipfstuhl, S., Shigeyama, W., et al. (2017). Location and distribution of micro-inclusions in the EDML and NEEM ice cores using optical microscopy and in situ Raman spectroscopy. Cryosphere 11, 1075–1090. doi:10.5194/tc-11-1075-2017
Eichler, J., Weikusat, C., Wegner, A., Twarloh, B., Behrens, M., Fischer, H., et al. (2019). Impurity Analysis and Microstructure Along the Climatic Transition From MIS 6 Into 5e in the EDML Ice Core Using Cryo-Raman Microscopy. Front. Earth Sci. 7, 1–16. doi:10.3389/feart.2019.00020
Faria, S. H., Freitag, J., and Kipfstuhl, S. (2010). Polar ice structure and the integrity of ice-core paleoclimate records. Quat. Sci. Rev. 29, 338–351. doi:10.1016/j.quascirev.2009.10.016
Faria, S. H., Kipfstuhl, S., and Lambrecht, A. (2018). The EPICA-DML deep ice core: a visual record. Berlin, Germany: Springer.
Faria, S. H., Weikusat, I., and Azuma, N. (2014a). The microstructure of polar ice. Part I: highlights from ice core research. J. Struct. Geol. 61, 2–20. doi:10.1016/j.jsg.2013.09.010
Faria, S. H., Weikusat, I., and Azuma, N. (2014b). The microstructure of polar ice. Part II: state of the art. J. Struct. Geol. 61, 21–49. doi:10.1016/j.jsg.2013.11.003
Fisher, B. D. A., and Koerner, R. M. (1986). On the special rheological properties of ancient microparticles-laden northern hemisphere ice as derived from borehole and core measurements. J. Glaciol. 32, 501–510. doi:10.3189/S0022143000012211
Fisher, D. A., and Koerner, R. M. (1987). Enhanced flow of Wisconsin ice related to solid conductivity through strain history and recrystallisation. Int. Assoc. Hydrol. Sci. 170, 45–51.
Fitzpatrick, J. J., Voigt, D. E., Fegyveresi, J. M., Stevens, N. T., Spencer, M. K., Cole-Dai, J., et al. (2014). Physical properties of the WAIS Divide ice core. J. Glaciol. 60, 1181–1198. doi:10.3189/2014JoG14J100
Foreman, A. J. E., and Makin, M. J. (1966). Dislocation movement through random arrays of obstacles. Phil. Mag. 14, 911–924. doi:10.1080/14786436608244762
Frank, F. C., and Read, W. T. (1950). Multiplication processes for slow moving dislocations. Phys. Rev. 79, 722–723. doi:10.1103/PhysRev.79.722
Freitag, J., Kipfstuhl, S., Laepple, T., and Wilhelms, F. (2013). Impurity-controlled densification: a new model for stratified polar firn. J. Glaciol. 59, 1163–1169. doi:10.3189/2013JoG13J042
Frost, H. J., and Ashby, M. F. (1982). Deformation mechanism maps—the plasticity and creep of metals and ceramics. Oxfordshire, NY, United States: Pergamon Press.
Fudge, T. J., Taylor, K. C., Waddington, E. D., Fitzpatrick, J. J., and Conway, H. (2016). Electrical stratigraphy of the WAIS Divide ice core: identification of centimeter-scale irregular layering. J. Geophys. Res.: Earth Surface. 121, 1218–1229. doi:10.1002/2016JF003845
Fujita, S., Hirabayashi, M., Goto-Azuma, K., Dallmayr, R., Satow, K., Zheng, J., et al. (2014). Densification of layered firn of the ice sheet at NEEM, Greenland. J. Glaciol. 60, 905–921. doi:10.3189/2014JoG14J006
Fukazawa, H., Sugiyama, K., Shinji, M., Narita, H., and Hondoh, T. (1998). Acid ions at triple junction of Antarctic ice observed by Raman scattering. Geophys. Res. Lett. 25, 2845–2848. doi:10.1029/98GL02178
Fukazawa, H., Suzuki, D., Ikeda, T., Mae, S., and Hondoh, T. (1997). Raman spectra of translational lattice vibrations in polar ice. J. Phys. Chem. B. 101, 6184–6187. doi:10.1021/jp963161r
Glen, J. W., and Jones, S. J. (1967). The deformation of ice single crystals at low temperatures. Phys. Snow Ice: Proc. 1, 267–275.
Glen, J. W. (1969). Structure and point defects of ice: their effect on the electrical and mechanical properties. Sci. Prog. 57, 1–21.
Glen, J. W. (1955). The creep of polycrystalline ice. Proc. Roy. Soc. Lond. Math. Phys. Sci. 288, 519–538. doi:10.1002/2015JB012542
Glen, J. W. (1968). The effect of hydrogen disorder on dislocation movement and plastic deformation of ice. Phys. Kondensierten Mater. 7, 43–51.
Goldsby, D. L., and Kohlstedt, D. L. (1997). Grain boundary sliding in fine-grained ice I. Scripta Mater. 37, 1399–1406. doi:10.1016/S1359-6462(97)00246-7
Goldsby, D. L., and Kohlstedt, D. L. (2002). Reply to comment by P. Duval and M. Montagnat on “Superplastic deformation of ice: experimental observations”. J. Geophys. Res. Solid Earth. 107, 17–21. doi:10.1029/2002JB001842
Goldsby, D. L., and Kohlstedt, D. L. (2001). Superplastic deformation of ice: experimental observations. J. Geophys. Res.: Solid Earth. 106, 11017–11030. doi:10.1029/2000jb900336
Goldsby, D. L. (2006). “Superplastic flow of ice relevant to glacier and ice-sheet mechanics,” in Glacier science and environmental change. Editor P. G. Knight (Malden, MA, United States: Blackwell Publishing), 308–314. doi:10.1002/9780470750636.ch60
Gow, A. J., and Williamson, T. (1971). Volcanic ash in the Antarctic Ice Sheet and its possible climatic implications. Earth Planet Sci. Lett. 13, 210–218. doi:10.1016/0012-821X(71)90126-9
Gross, G. W., Hayslip, I. C., and Hoy, R. N. (1978). Electrical donductivity and relaxation in ice crystals with known Impurity content. J. Glaciol. 21.
Hammer, C. U. (1977). Past volcanism revealed by Greenland ice sheet impurities. Nature. 270, 482–486. doi:10.1038/270482a0
Hammonds, K., and Baker, I. (2016). The effects of Ca++ on the strength of polycrystalline ice. J. Glaciol. 62, 954–962. doi:10.1017/jog.2016.84
Hammonds, K., and Baker, I. (2018). The effects of H2SO4 on the mechanical behavior and microstructural evolution of polycrystalline ice. J. Geophys. Res.: Earth Surface. 123, 535–556. doi:10.1002/2017JF004335
Harrison, B. W. D., and Raymond, C. F. (1976). Impurities and their distribution in temperate glacier ice. J. Glaciol. 16, 173–181. doi:10.3189/S0022143000031518
Hirth, J. P., and Lothe, J. (1982). Theory of dislocations. 2nd edn. Malabar, Florida: Krieger Publishing Company.
Hörhold, M. W., Laepple, T., Freitag, J., Bigler, M., Fischer, H., and Kipfstuhl, S. (2012). On the impact of impurities on the densification of polar firn. Earth Planet Sci. Lett. 325-326, 93–99. doi:10.1016/j.epsl.2011.12.022
Hu, X., Jia, K., Liu, F., Baker, I., and Black, D. (1995). Dislocation mobility in HCL-doped ice. Proc. Mater. Res. Soc. 375, 287–292. doi:10.1557/PROC-375-287
Humphrey, F. J., and Hatherly, M. (1996). recrystallisation and related annealing phenomena. Oxford, NY, United States: Pergamon Press.
Humphreys, F., and Hatherly, M. (2004). recrystallisation and related annealing phenomena. Amsterdam, Netherlands: Elsevier. doi:10.1016/B978-0-08-044164-1.X5000-2
Iliescu, D., Baker, I., and Chang, H. (2004). Determining the orientations of ice crystals using electron backscatter patterns. Microsc. Res. Tech. 63, 183–187. doi:10.1002/jemt.20029
Iliescu, D., and Baker, I. (2008). Effects of impurities and their redistribution during recrystallisation of ice crystals. J. Glaciol. 54, 362–370. doi:10.3189/002214308784886216
Iliescu, D., Baker, I., and Li, X. (2003). The effects of sulfuric acid on the creep, recrystallisation, and electrical properties of ice. Can. J. Phys. 81, 395–400. doi:10.1139/p02-130
Jacka, T. H. (1984). The time and strain required for development of minimum strain rates in ice. Cold Reg. Sci. Technol. 8, 261–268. doi:10.1016/0165-232X(84)90057-0
Jones, S. J., and Glen, J. W. (1969). The effect of dissolved impurities on the mechanical properties of ice crystals. Phil. Mag. 19, 13–24. doi:10.1080/14786436908217758
Jun, L., Jacka, T. H., and Morgan, V. (1998). Crystal-size and microparticle record in the ice core from Dome summit South, law Dome, East Antarctica. Ann. Glaciol. 27, 343–348. doi:10.3189/1998AoG27-1-343-348
Karato, S.-i. (2008). Deformation of Earth materials. Cambridge, England: Cambridge University Press. doi:10.1017/CBO9780511804892
Kaufmann, P. R., Federer, U., Hutterli, M. A., Bigler, M., Schüpbach, S., Ruth, U., et al. (2008). An improved continuous flow analysis system for high-resolution field measurements on ice cores. Environ. Sci. Technol. 42, 8044–8050. doi:10.1021/es8007722
Kelka, U., Koehn, D., and Beaudoin, N. (2015). Zebra pattern in rocks as a function of grain growth affected by second-phase particles. Frontiers in Physics. 3, 74. doi:10.3389/fphy.2015.00074
Kipfstuhl, Sepp., Hamann, Ilka., Lambrecht, Anja., et al. (2006). Microstructure mapping: a new method for imaging deformation-induced microstructural features of ice on the grain scale. J. Glaciol. 52, 398–406. doi:10.3189/172756506781828647
Kipfstuhl, S., Faria, S. H., Azuma, N., Freitag, J., Hamann, I., Kaufmann, P., et al. (2009). Evidence of dynamic recrystallisation in polar firn. J. Geophys. Res.: Solid Earth. 114, 1–10. doi:10.1029/2008JB005583
Kuiper, E.-J. N., de Bresser, J. H. P., Drury, M. R., Eichler, J., Pennock, G. M., and Weikusat, I. (2020a). Using a composite flow law to model deformation in the NEEM deep ice core, Greenland – Part 2: the role of grain size and premelting on ice deformation at high homologous temperature. Cryosphere 14, 2449–2467. doi:10.5194/tc-14-2449-2020
Kuiper, E.-J. N., Weikusat, I., de Bresser, J. H. P., Jansen, D., Pennock, G. M., and Drury, M. R. (2020b). Using a composite flow law to model deformation in the NEEM deep ice core, Greenland – Part 1: the role of grain size and grain size distribution on deformation of the upper 2207 m. Cryosphere 14, 2429–2448. doi:10.5194/tc-14-2429-2020
Legrand, M., and Mayewski, P. (1997). Glaciochemistry of polar ice cores: a review. Rev. Geophys. 35, 219–243. doi:10.1029/96RG03527
Legrand, M. R., and Delmas, R. J. (1988). Soluble impurities in four antarctic ice cores over the last 30 000 years. Ann. Glaciol. 10, 116–120. doi:10.3189/s0260305500004274
Llorens, M. G., Griera, A., Steinbach, F., Bons, P. D., Gomez-Rivas, E., Jansen, D., et al. (2016). Dynamic recrystallisation during deformation of polycrystalline ice: insights from numerical simulations. Philos. Trans. A Math. Phys. Eng. Sci. 375, 20150346. doi:10.1098/rsta.2015.0346
Lomonaco, R., Albert, M., and Baker, I. (2011). Microstructural evolution of fine-grained layers through the firn column at Summit, Greenland. J. Glaciol. 57, 755–762. doi:10.3189/002214311797409730
Louchet, F. (2004). Dislocations and plasticity in ice. Compt. Rendus Phys. 5, 687–698. doi:10.1016/j.crhy.2004.09.001
Mader, H. M. (1992a). Observations of the water-vein system in polycrystalline ice. J. Glaciol. 38, 333–347. doi:10.1017/S0022143000002227
Mader, H. M. (1992b). The thermal behaviour of the water-vein system in polyrystalline ice. J. Glaciol. 38, 359–374. doi:10.3189/S0022143000002240
Y. Markaki, and H. Harz (2017). “Methods in molecular biology,” in Light microscopy. New York, NY, United States: Springer, Vol. 1563. doi:10.1007/978-1-4939-6810-7
Masson-Delmotte, V., Stenni, B., Pol, K., Braconnot, P., Cattani, O., Falourd, S., et al. (2010). EPICA Dome C record of glacial and interglacial intensities. Quat. Sci. Rev. 29, 113–128. doi:10.1016/j.quascirev.2009.09.030
McConnell, J. R., Lamorey, G. W., Lambert, S. W., and Taylor, K. C. (2002). Continuous ice-core chemical analyses using inductively coupled plasma mass spectrometry. Environ. Sci. Technol. 36, 7–11. doi:10.1021/es011088z
Means, W. (1981). The concept of steady-state foliation. Tectonophysics. 78, 179–199. doi:10.1016/0040-1951(81)90013-5
Miyamoto, A., Narita, H., Hondoh, T., Shoji, H., Kawada, K., Watanabe, O., et al. (1999). Ice-sheet flow conditions deduced from mechanical tests of ice core. Ann. Glaciol. 29, 179–183. doi:10.3189/172756499781820950
Montagnat, M., Azuma, N., Dahl-Jensen, D., Eichler, J., Fujita, S., Gillet-Chaulet, F., et al. (2014). Fabric along the NEEM ice core, Greenland, and its comparison with GRIP and NGRIP ice cores. Cryosphere. 8, 1129–1138. doi:10.5194/tc-8-1129-2014
Montagnat, M., Duval, P., Bastie, P., Hamelin, B., and Lipenkov, V. Y. (2003). Lattice distortion in ice crystals from the Vostok core (Antarctica) revealed by hard X-ray diffraction; implication in the deformation of ice at low stresses. Earth Planet Sci. Lett. 214, 369–378. doi:10.1016/S0012-821X(03)00364-9
Montagnat, M., Löwe, H., Calonne, N., Schneebeli, M., Matzl, M., and Jaggi, M. (2020). On the birth of structural and crystallographic fabric signals in polar snow: a case study from the EastGRIP snowpack. Front. Earth Sci. 8, 1–23. doi:10.3389/feart.2020.00365
Moser, D. E., Hörhold, M., Kipfstuhl, S., and Freitag, J. (2020). Microstructure of snow and its link to trace elements and isotopic composition at Kohnen station, dronning Maud Land, Antarctica. Front. Earth Sci. 8, 23. doi:10.3389/feart.2020.00023
Mulvaney, R., Wolff, E. W., and Oatest, K. (1988). Sulphuric acid at grain boundaries in Antarctic ice. Nature (Lond.). 331, 247–249.
Nye, J. F. (1998). Diffusion of isotopes in the annual layers of ice sheets. J. Glaciol. 44, 467–468.
Obbard, R., Iliescu, D., Cullen, D., Chang, J., and Baker, I. (2003). SEM/EDS comparison of polar and seasonal temperate ice. Microsc. Res. Tech. 62, 49–61. doi:10.1002/jemt.10381
Obbard, R., and Baker, I. (2007). The microstructure of meteoric ice from Vostok, Antarctica. J. Glaciol. 53, 41–62. doi:10.3189/172756507781833901
Obbard, R. W., Baker, I., and Prior, D. J. (2011). Instruments and methods a scanning electron microscope technique for identifying the mineralogy of dust in ice cores. J. Glaciol. 57, 511–514. doi:10.3189/002214311796905622
Ohno, H., Igarashi, M., and Hondoh, T. (2006). Characteristics of salt inclusions in polar ice from Dome Fuji, East Antarctica. Geophys. Res. Lett. 33, L08501. doi:10.1029/2006GL025774
Ohno, H., Igarashi, M., and Hondoh, T. (2005). Salt inclusions in polar ice core: location and chemical form of water-soluble impurities. Earth Planet Sci. Lett. 232, 171–178. doi:10.1016/j.epsl.2005.01.001
Ohno, H., Iizuka, Y., Horikawa, S., Sakurai, T., Hondoh, T., and Motoyama, H. (2014). Potassium alum and aluminum sulphate micro-inclusions in polar ice from Dome Fuji, East Antarctica. Polar Science. 8, 1–9. doi:10.1016/j.polar.2013.11.003
Ohno, H., Lipenkov, V. Y., and Hondoh, T. (2010). Formation of air clathrate hydrates in polar ice sheets : heterogeneous nucleation induced by micro-inclusions. J. Glaciol. 56, 917–921. doi:10.3189/002214310794457317
Paterson, W. S. B. (1991). Why ice-age ice is sometimes soft, Cold Reg. Sci. Technol. 20, 75–98. doi:10.1016/0165-232X(91)90058-O
Peternell, M., Russell-Head, D. S., and Wilson, C. J. (2011). A technique for recording polycrystalline structure and orientation during in situ deformation cycles of rock analogues using an automated fabric analyser. J. Microsc. 242, 181–188. doi:10.1111/j.1365-2818.2010.03456.x
Petit, J. R., Duval, P., and Lorius, C. (1987). Long-term climatic changes indicated by crystal growth in polar ice. Nature. 326, 62–64. doi:10.1038/326062a0
Petrenko, V. F., and Whitworth, R. W. (1999). Physics of ice. London, United Kingdom: Clarendon Press.
Raman, C. V., and Krishnan, K. S. (1928a). A new type of secondary radiation. Nature. 121, 501–502. doi:10.1038/121501c0
Raman, C. V., and Krishnan, K. S. (1928b). The optical analogue of the compton effect. Nature. 121, 711. doi:10.15421/141708
Rasmussen, S. O., Andersen, K. K., Svensson, A. M., Steffensen, J. P., Vinther, B. M., Clausen, H. B., et al. (2006). A new Greenland ice core chronology for the last glacial termination. J. Geophys. Res. 111, D06102. doi:10.1029/2005JD006079
L. Reimer (1998). “Scanning electron microscopy,” in Springer series in optical sciences. (Berlin, Heidelberg: Springer), Vol. 45, 2. doi:10.1007/978-3-540-38967-5
Reinhardt, H., Kriews, M., Miller, H., Lüdke, C., Hoffmann, E., and Skole, J. (2003). Application of LA-ICP-MS in polar ice core studies. Anal. Bioanal. Chem. 375, 1265–1275. doi:10.1007/s00216-003-1793-5
Reinhardt, H., Kriews, M., Miller, H., Schrems, O., Lüdke, C., Hoffmann, E., et al. (2001). Laser ablation inductively coupled plasma mass spectrometry: a new tool for trace element analysis in ice cores. Fresenius’ J. Anal. Chem. 370, 629–636. doi:10.1007/s002160100853
Rempel, A. W., Waddington, E. D., Wettlaufer, J. S., and Worster, M. G. (2001). Possible displacement of the climate signal in ancient ice by premelting and anomalous diffusion. Nature. 411, 568–571. doi:10.1038/35079043
Röthlisberger, R., Bigler, M., Hutterli, M., Sommer, S., Stauffer, B., Junghans, H. G., et al. (2000). Technique for continuous high-resolution analysis of trace substances in firn and ice cores. Environ. Sci. Technol. 34, 338–342. doi:10.1021/es9907055
Sakurai, T., Ilzuka, Y., Horlkawa, S., Johnsen, S., Dahl-Jensen, D., Steffensen, J. P., et al. (2009). Direct observation of salts as micro-inclusions in the Greenland GRIP ice core. J. Glaciol. 55, 777–783. doi:10.3189/002214309790152483
Sakurai, T., Ohno, H., Genceli, F. E., Horikawa, S., Iizuka, Y., Uchida, T., et al. (2010). Magnesium methanesulfonate salt found in the Dome Fuji (Antarctica) ice core. J. Glaciol. 56, 837–842. doi:10.3189/002214310794457335
Sakurai, T., Ohno, H., Horikawa, S., Iizuka, Y., Uchida, T., Hirakawa, K., et al. (2011). The chemical forms of water-soluble microparticles preserved in the Antarctic ice sheet during Termination I. J. Glaciol. 57, 1027–1032. doi:10.3189/002214311798843403
Saruya, T., Nakajima, K., Takata, M., Homma, T., Azuma, N., and Goto-Azuma, K. (2019). Effects of microparticles on deformation and microstructural evolution of fine-grained ice. J. Glaciol. 65, 531–541. doi:10.1017/jog.2019.29
Scherer, J. R., and Snyder, R. G. (1977). Raman intensities of single crystal ice Ih. J. Chem. Phys. 67, 4794–4811. doi:10.1063/1.434683
Schulson, E. M., and Duval, P. (2009). Creep and fracture of ice. Cambridge, England: Cambridge University Press. doi:10.1017/CBO9780511581397
Seiler, H. (1983). Secondary electron emission in the scanning electron microscope. J. Appl. Phys. 54. doi:10.1063/1.332840
Severi, M., Becagli, S., Frosini, D., Marconi, M., Traversi, R., and Udisti, R. (2014). A novel fast ion chromatographic method for the analysis of fluoride in antarctic snow and ice. Environ. Sci. Technol. 48, 1795–1802. doi:10.1021/es404126z
Shearwood, C., and Whitworth, R. W. (1991). The velocity of dislocations in ice. Philos. Mag. A. 64, 289–302. doi:10.1080/01418619108221186
Shigeyama, W., Nagatsuka, N., Homma, T., Takata, M., Goto-Azuma, K., Weikusat, I., et al. (2019). Microstructural analysis of Greenland ice using a cryogenic scanning electron microscope equipped with an electron backscatter diffraction detector. Bull. Glaciol. Res. 37, 31–45. doi:10.5331/BGR.19R01
Shoji, H., and Higashi, A. (1978). A deformation mechanism map of ice. J. Glaciol. 21, 419–427. doi:10.3189/s002214300003358x
Simonsen, M. F., Baccolo, G., Blunier, T., Borunda, A., Delmonte, B., Frei, R., et al. (2019). East Greenland ice core dust record reveals timing of Greenland ice sheet advance and retreat. Nat. Commun. 10. doi:10.1038/s41467-019-12546-2
Smith, C. S. (1948). Grains, phases, and interfaces: an introduction of microstructure. Trans. AIME. 175, 15–51.
Song, M., Baker, I., and Cole, D. M. (2008). The effect of particles on creep rate and microstructures of granular ice. J. Glaciol. 54, 533–537. doi:10.3189/002214308785836959
Song, M., Cole, D. M., and Baker, I. (2006). An investigation of the effects of particles on creep of polycrystalline ice. Scripta Mater. 55, 91–94. doi:10.1016/j.scriptamat.2006.03.029
Song, M., Cole, D. M., and Baker, I. (2005). Creep of granular ice with and without dispersed particles. J. Glaciol. 51, 210–218. doi:10.3189/172756505781829377
Spaulding, N. E., Sneed, S. B., Handley, M. J., Bohleber, P., Kurbatov, A. V., Pearce, N. J., et al. (2017). A new multielement method for LA-ICP-MS data acquisition from glacier ice cores. Environ. Sci. Technol. 51, 13282–13287. doi:10.1021/acs.est.7b03950
Steffensen, J. P., Andersen, K. K., Bigler, M., Clausen, H. B., Dahl-Jensen, D., Fischer, H., et al. (2008). High-resolution Greenland ice core data show abrupt climate change happens in few years. Science 321, 680–684. doi:10.1126/science.1157707
Steffensen, J. P., Clausen, H. B., Hammer, C. U., Legrand, M., and De Angelis, M. (1997). The chemical composition of cold events within the Eemian section of the Greenland Ice Core Project ice core from Summit, Greenland. J. Geophys. Res.: Oceans. 102, 26747–26754. doi:10.1029/97JC01491
Steffensen, J. P. (1997). The size distribution of microparticles from selected segments of the Greenland Ice Core Project representing different climatic periods. J. Geophys. Res. 102, 755–762. doi:10.1029/97JC01490
Steinbach, F., Bons, P., Griera, A., Jansen, D., Llorens, M.-G., Roessiger, J., et al. (2016). Strain localisation and dynamic recrystallisation in the ice-air aggregate: a numerical study. Cryosphere 10, 3071–3089. doi:10.5194/tc-10-3071-2016
Steinbach, F., Kuiper, E.-J. N., Eichler, J., Bons, P. D., Drury, M. R., Griera, A., et al. (2017). The relevance of grain dissection for grain size reduction in polar ice: insights from numerical models and ice core microstructure analysis. Front. Earth Sci. 5. doi:10.3389/feart.2017.00066
Stoll, N., and Weikusat, I. (2020). Microstructure mapping, Raman, Visual Stratigraphy and SEM measurements of the EGRIP and EDML ice core. PANGAEA. doi:10.1594/PANGAEA.924849
Svensson, A., Nielsen, S. W., Kipfstuhl, S., Johnsen, S. J., Steffensen, J. P., Bigler, M., et al. (2005). Visual stratigraphy of the North Greenland Ice Core Project (NorthGRIP) ice core during the last glacial period. J. Geophys. Res. 110, 1–11. doi:10.1029/2004JD005134
Taylor, K. C., and Alley, R. B. (2004). Two-dimensional electrical stratigraphy of the Siple Dome (Antarctica) ice core. J. Glaciol. 50, 231–235. doi:10.3189/172756504781830033
Tison, J. L., De Angelis, M., Littot, G., Wolff, E., Fischer, H., Hansson, M., et al. (2015). Retrieving the paleoclimatic signal from the deeper part of the EPICA Dome C ice core. Cryosphere 9, 1633–1648. doi:10.5194/tc-9-1633-2015
Trickett, Y. L., Baker, I., and Pradhan, P. M. S. (2000). The effects of sulfuric acid on the mechanical properties of ice single crystals. J. Glaciol. 46, 239–243. doi:10.3189/172756500781832819
van der Veen, C. J., and Whillans, I. M. (1994). Development of fabric in ice. Cold Reg. Sci. Technol. 22, 171–195. doi:10.1016/0165-232X(94)90027-2
Wallbrecher, E. (1986). Tektonische und gefügeanalytische Arbeitsweisen: graphische, rechnerische und statistische Verfahren. Stuttgart, Germany: Enke.
Wang, Y., Thorsteinsson, T., Kipfstuhl, J., Miller, H., Dahl-Jensen, D., and Shoji, H. (2002). A vertical girdle fabric in the NorthGRIP deep ice core, North Greenland. Ann. Glaciol. 35, 515–520. doi:10.3189/172756402781817301
Wayne, R. O. (2014). Light and video microscopy. Amsterdam, Netherland: Elsevier. doi:10.1016/C2012-0-07122-0
Weertman, J. (1973). “Creep of ice,” in Physics and chemistry of ice. Editors E. Whalley, S. W. Jones, and L. W. Gold (Ottawa: Royal Society of Canada), 320.
Weertman, J., and Weertman, J. R. (1992). Elementary dislocation theory. Oxford, NY, United States: Oxford University Press.
Wegner, A., Fischer, H., Delmonte, B., Petit, J.-R., Erhardt, T., Ruth, U., et al. (2015). The role of seasonality of mineral dust concentration and size on glacial/interglacial dust changes in the EPICA Dronning Maud Land ice core. J. Geophys. Res.: Atmosphere 120, 9916–9931. doi:10.1002/2015JD023608
Weikusat, I., Kipfstuhl, S., Faria, S. H., Azuma, N., and Miyamoto, A. (2009). Subgrain boundaries and related microstructural features in EDML (Antarctica) deep ice core. J. Glaciol. 55, 461–472. doi:10.3189/002214309788816614
Weikusat, I., Kuiper, E. J. N., Pennock, G. M., Kipfstuhl, S., and Drury, M. R. (2017). EBSD analysis of subgrain boundaries and dislocation slip systems in Antarctic and Greenland ice. Solid Earth. 8, 883–898. doi:10.1159/000320310
Weiss, J., and Marsan, D. (2003). Three-Dimensional mapping of dislocation avalanches: clustering and space/time coupling. Science 299, 89–92. doi:10.1126/science.1079312
Weiss, J., Vidot, J., Gay, M., Arnaud, L., Duval, P., and Petit, J. R. (2002). Dome Concordia ice microstructure: impurities effect on grain growth. Ann. Glaciol. 35, 552–558. doi:10.3189/172756402781816573
Westhoff, J., Stoll, N., Franke, S., Weikusat, I., Bons, P., Kerch, J., et al. (2020). A stratigraphy-based method for reconstructing ice core orientation. Ann. Glaciol. 1, 12. doi:10.1017/aog.2020.76
Weygand, D., Bréchet, Y., and Lépinoux, J. (1998). A vertex dynamics simulation of grain growth in two dimensions. Phil. Mag. B. 78, 329–352. doi:10.1080/13642819808206731
Wilhelms, F., Kipfstuhl, J., Miller, H., Heinloth, K., and Firestone, J. (1998). Precise dielectric profiling of ice cores: a new device with improved guarding and its theory. J. Glaciol. 44, 171–174. doi:10.3189/S002214300000246X
Wilson, C. J., Russell-Head, D. S., and Sim, H. M. (2003). The application of an automated fabric analyser system to the textural evolution of folded ice layers in shear zones. Ann. Glaciol. 37, 7–17. doi:10.3189/172756403781815401
Wolff, E. W., Chappellaz, J., Blunier, T., Rasmussen, S. O., and Svensson, A. (2010). Millennial-scale variability during the last glacial: the ice core record. Quat. Sci. Rev. 29, 2828–2838. doi:10.1016/j.quascirev.2009.10.013
Wolff, E. W., Miners, W. D., Moore, J. C., and Paren, J. G. (1997). Factors controlling the electrical conductivity of ice from the polar regions. A summary. J. Phys. Chem. B. 101, 6090–6094. doi:10.1021/jp9631543
Keywords: location of impurities, microstructure, flow and deformation of ice, polar ice sheets, micro-particles, raman spectroscopy, grain growth, recrystallisation
Citation: Stoll N, Eichler J, Hörhold M, Shigeyama W and Weikusat I (2021) A Review of the Microstructural Location of Impurities in Polar Ice and Their Impacts on Deformation. Front. Earth Sci. 8:615613. doi: 10.3389/feart.2020.615613
Received: 09 October 2020; Accepted: 30 November 2020;
Published: 14 January 2021.
Edited by:
Markus Michael Frey, British Antarctic Survey (BAS), United KingdomReviewed by:
Anders Svensson, University of Copenhagen, DenmarkIan Baker, Dartmouth College, United States
Copyright © 2021 Stoll, Eichler, Hörhold, Shigeyama and Weikusat. This is an open-access article distributed under the terms of the Creative Commons Attribution License (CC BY). The use, distribution or reproduction in other forums is permitted, provided the original author(s) and the copyright owner(s) are credited and that the original publication in this journal is cited, in accordance with accepted academic practice. No use, distribution or reproduction is permitted which does not comply with these terms.
*Correspondence: Nicolas Stoll, bmljb2xhcy5zdG9sbEBhd2kuZGU=