- 1Biogeochemistry Research Center, Japan Agency for Marine-Earth Science and Technology, Yokosuka, Japan
- 2Kochi Institute for Core Sample Research, Japan Agency for Marine-Earth Science and Technology, Nankoku, Japan
- 3Atmosphere and Ocean Research Institute, The University of Tokyo, Kashiwa, Japan
- 4Geological Survey of Japan, National Institute of Advanced Industrial Science and Technology, Tsukuba, Japan
Variations in the stable isotopic composition of seawater Sr (δ88Sr) is a new tool for estimating the rates of global carbonate sedimentation over geologic time, yet the isotope compositions of the major sources and sinks of Sr to the world oceans are still in need of further constraint. We report δ88Sr values of vent fluids from arc/back-arc seafloor hydrothermal systems in the western Pacific. In the sediment-starved hydrothermal fields of the Manus Basin, Izu-Bonin Arc, and Mariana Trough, the δ88Sr values of end-member fluids for each site showed little variation (0.29–0.30‰) and were close to the average value of oceanic volcanic rocks, reflecting dissolved Sr sourced from host rocks. Chlorine-depleted fluids from phase-separated hydrothermal systems in the North Fiji Basin had the end-member δ88Sr values of 0.26, 0.28, and 0.29‰. Thus, both sediment-starved and phase-separated vent fluids had the end-member δ88Sr values indistinguishable from or very close to the range of oceanic volcanic rocks. Therefore, the δ88Sr compositions in these hydrothermal sites are controlled predominantly by Sr sourced from host rock with a small influence from secondary mineral precipitation/re-dissolution. Fluids from the sediment-hosted hydrothermal fields of the Okinawa Trough, however, were characterized by low δ88Sr values of approximately 0.22‰ and high 87Sr/86Sr ratios, indicating interactions with sedimentary carbonates. As for the modern oceanic δ88Sr budget, the sediment-hosted sites lower the global hydrothermal δ88Sr. Since both sediment-starved and -hosted hydrothermal systems provide a long-term control on the global Sr cycle, the end-member δ88Sr value is an important constraint on the evolution of Sr cycling in past oceans.
Introduction
Seafloor hydrothermal systems play an important role in chemical and thermal fluxes to the ocean, which impact the evolution of ocean chemistry, climate, and life throughout geologic time (Elderfield and Schultz, 1996; Hardie, 1996). The concentration of Sr2+ in seawater is 89 μM, the fifth most abundant cation in seawater, with a mean residence time of about 2.5 million years (Hodell et al., 1990). Strontium inputs from hydrothermal systems have played an important role in past changes of seawater 87Sr/86Sr values, accounting for approximately 5% of total input fluxes with end-member 87Sr/86Sr values of 0.703–0.704 (Pearce et al., 2015a). These changes in the 87Sr/86Sr value of seawater have been used to elucidate the relative intensities of continental weathering and hydrothermal alteration of oceanic crust over time (DePaolo and Ingram, 1985; Hodell et al., 1990; Raymo and Ruddiman, 1992; Veizer et al., 1999; McArthur et al., 2001).
The combined use of radiogenic (87Sr/86Sr) and stable isotopic ratios (88Sr/86Sr, denoted by δ88Sr) is a novel approach that is useful for identifying differences in Sr sources and processes involving chemical reactions in the Earth’s surface (Figure 1; Ohno and Hirata, 2007; Halicz et al., 2008; Ohno et al., 2008; Wei et al., 2013; Neymark et al., 2014; Chao et al., 2015; Andrews et al., 2016; Stevenson et al., 2016; Liu et al., 2017; Shalev et al., 2017). Fractionation of 88Sr/86Sr, but not of 87Sr/86Sr, accompanies mineral precipitation and biological uptake (Böhm et al., 2012; Stevenson et al., 2014), and is of importance in understanding isotopic mass balance in Earth’s Sr cycle. The utility of the isotopic mass balance of δ88Sr and 87Sr/86Sr for the marine Sr budget has been favorably assessed in studies of both modern and past oceans (Krabbenhöft et al., 2010; Pearce et al., 2015a). Modern seawater is isotopically quite homogeneous in terms of both δ88Sr and 87Sr/86Sr, irrespective of area or depth (0.39‰ and 0.70917, Wakaki et al., 2017), and seawater Sr concentrations reflect the balance between contributions from hydrothermal systems, marine diagenesis, chemical weathering of continental rocks to seawater, and carbonate burial. The evolution of the seawater 87Sr/86Sr value is determined by the balance of inputs from rivers (approximately 0.713) and hydrothermal systems (0.704), whereas the δ88Sr record reflects primarily changes in the balance between riverine (0.32‰) and hydrothermal (0.24–0.27‰) inputs (Figure 1). The removal of marine biogenic carbonates 0.18–0.24‰ depleted in 88Sr leaves the ocean isotopically heavier than δ88Sr values of the input fluxes (Krabbenhöft et al., 2010; Pearce et al., 2015a). As a result, δ88Sr of past seawater varied from 0.25 to 0.60‰ in the Phanerozoic era (Vollstaedt et al., 2014). Strontium inputs from hydrothermal systems play a major role in the 87Sr/86Sr flux to the ocean (Elderfield and Schultz, 1996), but the magnitude of Sr isotope fractionation and the compositional variation of the end-member solutions in hydrothermal water-rock reactions must be known for accurate estimation of the global marine Sr input and output fluxes (Krabbenhöft et al., 2010). Strontium uptake and release during oceanic hydrothermal circulation may result in its enrichment in certain isotopes from the recharging seawater; the resolution of this key issue requires further investigation in different types of hydrothermal settings.
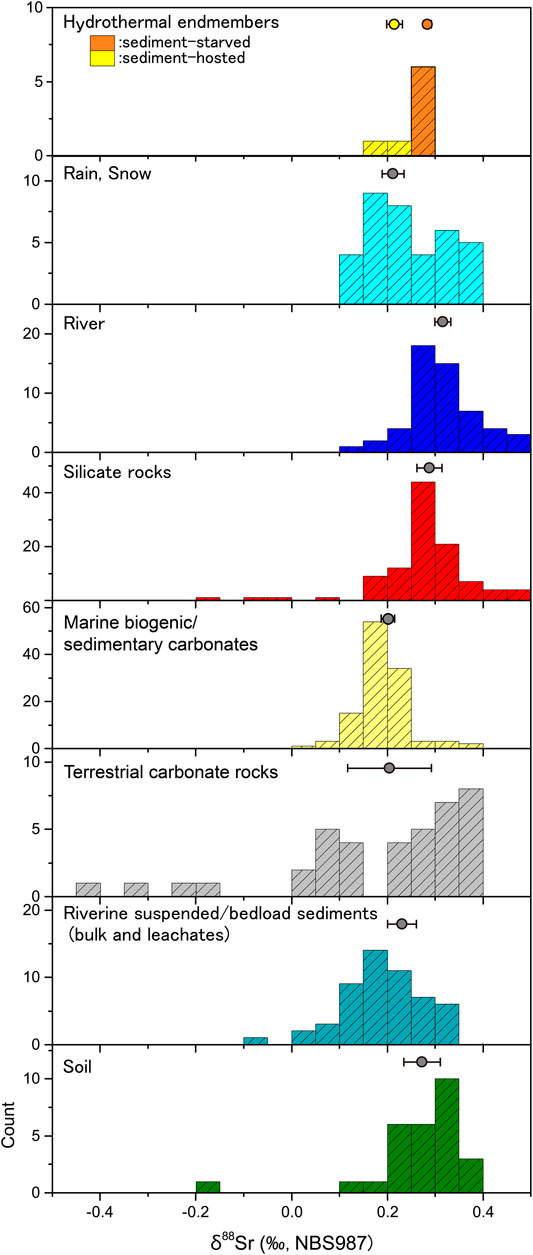
FIGURE 1. Compilation of δ88Sr values of terrestrial and marine: rain and snow, river water, groundwater, silicate rocks, carbonate rocks, and suspended mixed riverine sediments from glacial catchments. Data are from this study and other published δ88Sr analyses (Ohno and Hirata, 2007; Halicz et al., 2008; Ohno et al., 2008; Rüggeberg et al., 2008; Krabbenhöft et al., 2010; Moynier et al., 2010; Böhm et al., 2012; Charlier et al., 2012; Raddatz et al., 2013; Wei et al., 2013; Neymark et al., 2014; Stevenson et al., 2014; Chao et al., 2015; Pearce et al., 2015a, Pearce et al., 2015b; Voigt et al., 2015; Andrews et al., 2016; Fruchter et al., 2016; Stevenson et al., 2016; Liu et al., 2017; Shalev et al., 2017; Amsellem et al., 2018). The circles represent the averages of each material with 95% confidence interval.
Most data reported from Mid-Atlantic Ridge (MAR) hydrothermal vent fluids (Krabbenhöft et al., 2010; Pearce et al., 2015a) lie on the mixing line between seawater and an end-member component with estimated δ88Sr and 87Sr/86Sr values of 0.27‰ and 0.7045, respectively (Krabbenhöft et al., 2010), or 0.24‰ and 0.7037 (Pearce et al., 2015a), respectively. These end-member values are consistent with the reference values of oceanic basalt (about 0.25‰, Halicz et al., 2008; Ohno et al., 2008; Pearce et al., 2015a), although the 87Sr/86Sr values are slightly higher than those of fresh mid-ocean ridge basalt (MORB). More recently, the δ88Sr value of MORB has been suggested to be 0.31 ± 0.05‰ (Amsellem et al., 2018). In some MAR hydrothermal fluids, however, δ88Sr values are higher than expected from the mixing trend (Figure 2), and these higher values are interpreted as a result of isotopic fractionation during hydrothermal mineral precipitation or dissolution (Pearce et al., 2015a). A recent experimental study conducted under high-temperature and high-pressure conditions has shown that in fluid-basalt interactions, 88Sr is preferentially taken up into anhydrite (i.e., greater anhydrite precipitation and re-dissolution result in lower and higher δ88Sr in the fluid, respectively) and 86Sr is preferentially released as a result of the incongruent dissolution of crystalline basalt (Voigt et al., 2018). It is still uncertain to what degree the variation of δ88Sr values in hydrothermal systems can be attributed to solute sources or secondary mineral (e.g., anhydrite and calcite veins) formation.
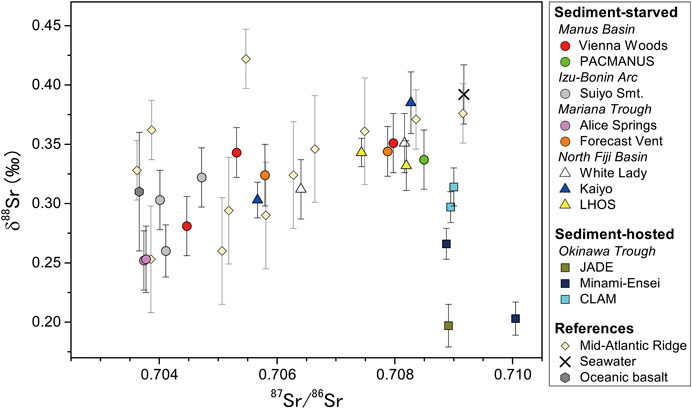
FIGURE 2. δ88Sr vs. 87Sr/86Sr in hydrothermal waters from western Pacific sites, along with data for the Mid-Atlantic Ridge reported by Krabbenhöft et al. (2010) and Pearce et al. (2015a). Bars represent 2SD errors. Because the internal errors of δ88Sr measurements are generally significantly small compared with long-term external reproducibility, reference data are plotted with the long-term reproducibility of each laboratory: 0.025‰ (n = 33) from Pearce et al. (2015a) and 0.044‰ (n = 90) from Krabbenhöft et al. (2009).
In arc and back-arc basins, the chemical compositions of vent fluids vary more widely than those of vent fluids in sediment-starved hydrothermal sites because of the compositional variation of the host rocks as some are buried by sediments (Volpe et al., 1987). Given the tectonic differences between back-arc rifting and mid-ocean ridge spreading zones, it is essential to fully assess δ88Sr systematics in arc/back-arc settings. In addition, although phase separation and segregation of vapor and fluid phases greatly affect hydrothermal fluid chemistry in both mid-ocean ridge and arc/back arc settings (Von Damm and Bischoff, 1987), the impact of separation and segregation processes on the δ88Sr values of hydrothermal fluids has not yet been examined. Finally, as an extreme case, hydrothermal fields of back-arc setting include heavily sediment-covered rift that lacks typical oceanic crust, as represented by the Okinawa Trough (Figure 3). Terrigenous materials contained in the overlying sediments have considerably influenced the 87Sr/86Sr signatures of the hydrothermal fluids in this region (Chiba et al., 1993; Noguchi et al., 2009; Araoka et al., 2016), and δ88Sr can be utilized to precisely apportion Sr sources in these sediment-hosted fields, that also impact the oceanic δ88Sr inventory.
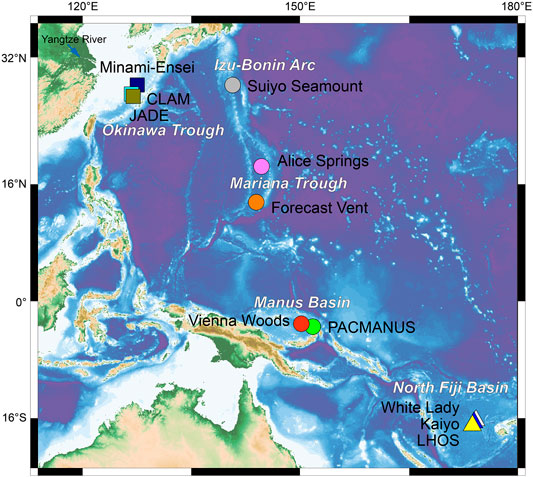
FIGURE 3. Map showing the sampling site locations. Circles, triangles, and squares represent sediment-starved, phase-separated, and sediment-hosted hydrothermal vents, respectively.
The characteristics of hydrothermal systems in arc/back arc settings are diverse with respect to the presence or absence of marine sediments, host-rock type, phase separation, and temperature of the vent fluids. However, how these factors influence 88Sr/86Sr fractionation and variations in the δ88Sr values of the fluids remains unknown. In this study, we investigated the 87Sr/86Sr and δ88Sr systematics of hydrothermal fluids collected from 11 vents in five arc and back-arc hydrothermal systems in the western Pacific. The studied sites, which include both sediment-starved and sediment-hosted sites and cover the large variation in hydrothermal settings described above, provide essential information for comprehensive understanding of Sr geochemistry in hydrothermal systems.
Materials and Methods
Geological Settings of Hydrothermal Vents
Hydrothermal vent fluids previously collected from eight sediment-starved sites in the Manus Basin (n = 5), Izu-Bonin Arc (n = 3), Mariana Trough (n = 4), and North Fiji Basin (n = 6), and from three sediment-hosted sites in the Okinawa Trough (n = 7) are used for this study (Table 1; Figure 3) (Sakai et al., 1990a; Gamo et al., 1991; Grimaud et al., 1991; Chiba et al., 1993; Ishibashi, 1994; Ishibashi et al., 1994a; Gamo et al., 1997). The chemical variability of the vent fluids as well as hydrothermal mineral deposits of these arc/back-arc hydrothermal systems reflect diversity in geological structures (axial graben, crest of a ridge, seamount, frank of an axial volcano, volcanic depression, and sediment-rich depression) and lithologic types ranging from mafic to felsic rocks (Ishibashi and Urabe, 1995; Table 2). The sediment-hosted Okinawa Trough sites are all developed on continental lithosphere, not oceanic lithosphere. According to the estimate of hydrothermal fluid fluxes from the major types of hydrothermal systems (Nakamura and Takai, 2015), the sediment-associated hydrothermal systems yield approximately 10% of the total hydrothermal flux to seawater; the sediment-starved mid-ocean ridge and arc/back-arc hydrothermal systems are estimated to deliver 80% and 10%, respectively. Chemical properties of sediment-starved hydrothermal system are controlled essentially by chemical equilibria between fluids and host rocks, while the hydrothermal fluids in the Okinawa Trough have been shown to interact with terrigenous and biogenic materials (Sakai et al., 1990a; Gamo et al., 1991).
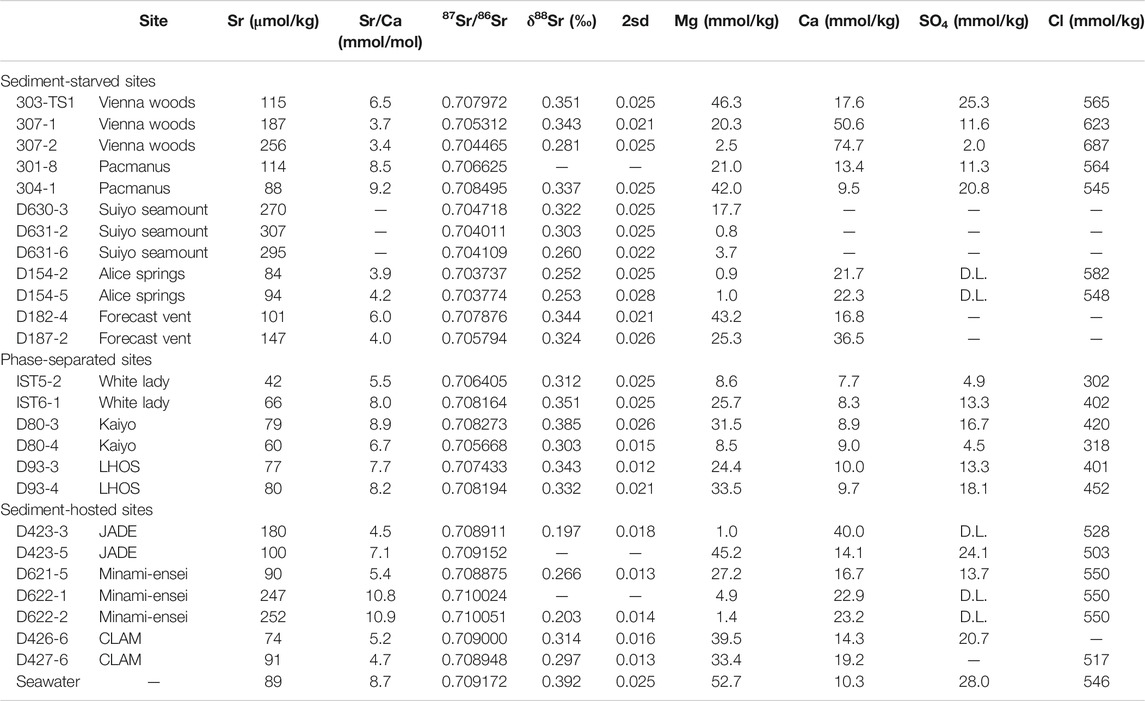
TABLE 1. Sr isotopic ratios and other chemical characteristics of hydrothermal fluid and seawater samples.
Sediment-Starved Sites (Manus Basin, Izu-Bonin Arc, Mariana Trough, North Fiji Basin)
The Vienna Woods field is located on the central spreading axis in the Manus Basin. This site is hosted in basalt and the chemical characteristics of the vent fluids have been previously reported to be comparable to those of mid-ocean ridge hydrothermal fluids (Lisitsyn et al., 1993; Gamo et al., 1997). The vent fluids of the Pacmanus field, located in the Eastern Manus Volcanic Zone, are characterized by higher K and B concentrations and a lower Ca concentration compared with the Vienna Woods fluids, reflecting their interaction with dacitic host rocks (Yamaoka et al., 2015).
The Suiyo Seamount is a dacitic submarine volcano along the volcanic front of the Izu-Bonin Arc. Its fluids are enriched in Ca and Sr, as well as in gaseous CO2, compared with the fluids from the mid-ocean ridge site (Ishibashi, 1994; Tsunogai et al., 1994).
The Mariana Trough is an actively spreading back-arc basin behind the Mariana Arc. The Alice Springs field is located on the flank of an axial basaltic andesite volcano in the mid-Mariana Trough. Clear, low-temperature (202°C) vent fluids were collected from an anhydrite chimney in the Forecast Vent field at the summit of a seamount in the southern Mariana Trough (Gamo et al., 1994).
The White Lady, Kaiyo, and LHOS sites in the North Fiji Basin have low chlorinity, suggesting segregation of vapor-rich phases (Grimaud et al., 1991). The petrological characteristics of the basalt in the North Fiji Basin are similar to those of normal MORB (N-MORB) but include a regional influence of coexisting oceanic island basalt components (Eissen et al., 1991; Nohara et al., 1994). Fluid samples from the White Lady field were collected from an anhydrite chimney at a topographic high point in the axial graben of the northern-central segment near the triple junction. Two chimneys, Kaiyo and LHOS, are also located in the same segment, 150 m southwest of the White Lady field. The chlorinity of vent fluids in the North Fiji Basin is estimated to be 47–49% that of seawater, suggesting that phase separation occurs beneath the seafloor and that a vapor-rich phase has condensed to form the hydrothermal fluid (Grimaud et al., 1991; Ishibashi et al., 1994b).
Sediment-Hosted Sites (Okinawa Trough)
The Okinawa Trough is a back-arc basin located at the eastern margin of the Eurasian Continent. It is considered to be initiated as continental rifting which commenced since approximately 2 Ma (Sibuet al., 1995). Hydrothermal sites in the Okinawa Trough are hosted in basalt for JADE and rhyolite-andesite for CLAM sites, and accompanied by a thick sediment layer consisting mainly of terrigenous clay derived from the Eurasian Continent (e.g., the Yangtze and Yellow Rivers) and biogenic carbonates (Halbach et al., 2017). For hydrothermal sites in the mid-ocean ridges, the primary fluid source is overlying seawater recharged through basaltic outcrops. In contrast, the large reservoir of sedimentary pore water in the thick trough-fill sediments recharges hydrothermal fluids in the sediment-hosted hydrothermal system of the mid-Okinawa Trough (Figure 4; Tsuji et al., 2012). Fluid migration driven by thermal advection of the silicic arc volcanism is considered to flow laterally and updip along permeable volcanoclastic sedimentary layers and along basement faults within the crust (Tsuji et al., 2012). These sediment-hosted hydrothermal systems are highly alkaline and enriched in methane derived from thermally altered sedimentary organic matter (Von Damm et al., 1985; Gamo et al., 1991; Kawagucci et al., 2013). The hydrothermal fluids in this area have high alkalinity and are enriched in NH4+, CH4, and CO2 as a result of interactions with sediments (Sakai et al., 1990a; Sakai et al., 1990b; Gamo et al., 1991; Chiba et al., 1993; Kawagucci et al., 2013).
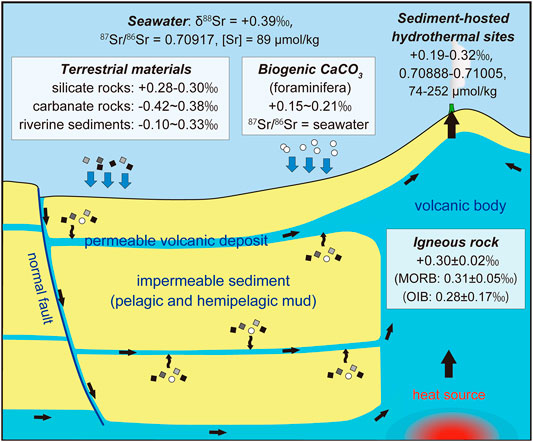
FIGURE 4. Schematic fluid flow of the hydrothermal system in the sediment-hosted continental back-arc basin of the mid-Okinawa Trough (modified after Tsuji et al., 2012). The primary fluid recharge and migration paths are normal faults and permeable volcanic deposits, and migrate through volcanic body to hydrothermal discharge zones (Tsuji et al., 2012). δ88Sr, 87Sr/86Sr, and [Sr2+] values of terrestrial and marine materials are referenced in Figure 1.
The JADE site is a high-temperature (320°C) hydrothermal vent field in the middle Okinawa Trough, and the fluids are emitted from sulfide-sulfate chimneys. The Minami-Ensei knoll is located in a rifting center in the northern Okinawa Trough (Chiba et al., 1993). This knoll is a volcanic complex characterized by a complicated topography of small hills and depressions within a circular rim of 2 km radius. The samples were collected from fragile anhydrite chimneys. The CLAM hydrothermal field is located in a small depression along the eastern Iheya Ridge and is associated with basalt breccia (Ishibashi et al., 1995). Mineralized chimney precipitates of the CLAM field consist mainly of manganoan calcite, rhodochrosite, anhydrite, and amorphous silica (Izawa et al., 1991). At the CLAM site, the end-member fluids contain Mg2+ (approximately 20 mM), reflecting a low maximum temperature of 220 °C and suggesting an incomplete water-rock reaction (Sakai et al., 1990b).
Analytical Methods
Hydrothermal fluid samples were filtered, acidified with HNO3, and stored in polypropylene bottles. Major and trace element concentrations and δ7Li, δ11B, δ26Mg, 87Sr/86Sr values of the fluid samples have been reported previously (Yamaoka et al., 2015; Araoka et al., 2016; Eom et al., 2020).
δ88Sr and 87Sr/86Sr values of the samples were determined simultaneously using the double-spike technique (Wakaki et al., 2017) in a TRITON thermal ionization mass spectrometer (Thermo Scientific, Germany) at Kochi Core Center, Japan. Two aliquots of each fluid sample, with and without the addition of the 84Sr-86Sr spike, were prepared for Sr isotopic analysis. After chemical separation of Sr by a Sr-Spec resin (Eichrom resin), the Sr sample with a Ta activator was loaded onto a single W filament. The 88Sr+ beam intensities were approximately 11 and 4 V for the unspiked and spiked runs, respectively. Peaks for 88Sr, 87Sr, 86Sr, 84Sr, and 85Rb were monitored. The 87Sr/86Sr ratio was measured in the unspiked sample by the conventional internal normalization technique using 86Sr/88Sr = 0.1194 and the exponential law. Stable 88Sr/86Sr ratios were calculated by using the data from the unspiked and spiked runs, and expressed as permil (‰) deviations relative to the NBS-987 standard as:
Repeated analyses of the in-house Sr isotopic reference reagent Wako-9999 (Wakaki et al., 2017) gave δ88Sr = 0.322 ± 0.025‰ (2 SD, n = 45). The external error for vent fluid samples was estimated as the 2SD repeatability of multiple SRM-987 analyses during each analytical session (Wakaki et al., 2017) and ranged from ±0.013‰ to ±0.028‰ (Table 1). To check the accuracy of the analysis, we analyzed the IAPSO seawater (0.392 ± 0.015‰, 2 SE, n = 3) as reference material, and consistent with published values for seawater samples from the Atlantic, Pacific, Indian, and Southern Oceans (Krabbenhöft et al., 2009; Neymark et al., 2014; Stevenson et al., 2014; Vollstaedt et al., 2014; Pearce et al., 2015a). The conventional 87Sr/86Sr ratios obtained by unspiked runs were corrected for a small systematic mass bias to give 87Sr/86SrSRM-987 = 0.710248. Typical repeatability of the 87Sr/86Sr ratios was ±0.000005 (2SD). The 87Sr/86Sr values determined in this study were essentially consistent with those previously reported by Araoka et al. (2016). For consistency with the δ88Sr values, we used the newly determined 87Sr/86Sr values in the present analysis.
Results
We examined the relationship between δ88Sr and 87Sr/86Sr in the hydrothermal fluids and compared the result with data for MAR hydrothermal fluids (Krabbenhöft et al., 2010; Pearce et al., 2015a) and MORB (Amsellem et al., 2018) (Figure 2; Table 1). The samples from sediment-starved (Manus Basin, Izu-Bonin Arc, and Mariana Basin) and phase-separated (North Fiji Basin) sites showed a clear positive correlation between δ88Sr and 87Sr/86Sr (Figure 2) and plot right along with the data reported previously by Krabbenhöft et al. (2010) for MAR. All 87Sr/86Sr values of the fluids from the sediment-hosted sites (Okinawa Trough) were distinctly higher than those from the other sites, and the δ88Sr values were distinctly lower. The fluids from sites in the Okinawa Trough had the following characteristics: 1) their 87Sr/86Sr values are similar to or even higher than the seawater value; 2) on the 87Sr/86Sr–δ88Sr scatter plot, they are outliers compared with the trend for sediment-starved sites; and 3) δ88Sr values of fluids from the JADE and Minami-Ensei sites were approximately 0.20‰ or less (Figure 2; Table 1).
Because hydrothermal fluid samples represent a mixture of seawater and the end-member hydrothermal fluid, previous studies calculated δ88Sr end-member values by extrapolating Mg/Sr to zero (Supplementary Figure S1). This approach is based on the hypothesis that Sr and Mg concentrations are closely coupled through water-rock reactions. However, hydrothermal fluids can experience either a net gain or loss of Sr, compared with the initial seawater concentration, during hydrothermal fluid circulation (Kawagucci et al., 2013), irrespective of whether the Mg concentration is close to zero in the end-member fluid. In this study, the Sr concentrations of the end-member fluid at each site were estimated by extrapolating the observed Sr-Mg linear regression line fitted to the data of two or three samples from each site to Mg = 0 (Figures 5A–E). For the CLAM site only, the regression line was extrapolated to an estimated end-member Mg content of 20 mmol/kg (Araoka et al., 2016) based on the observed relatively low maximum temperature of approximately 220 °C (Sakai et al., 1990a; Gamo et al., 1991). At most sites, both 87Sr/86Sr (Figures 5F–J) and δ88Sr (Figures 5K–O) values were linearly related to 1/Sr; therefore, the end-member Sr isotopic compositions were calculated using the end-member Sr concentrations. All linear regression lines were forced through the seawater end point. Significant correlations were not observed between δ88Sr and 1/Sr in samples from the Pacmanus, Alice Springs, and CLAM sites because these samples had a Sr concentration similar to that of seawater. The estimated end-member compositions are summarized in Table 2 along with the type of hydrothermal system, host rock, observed maximum temperature, and pH (Table 2).
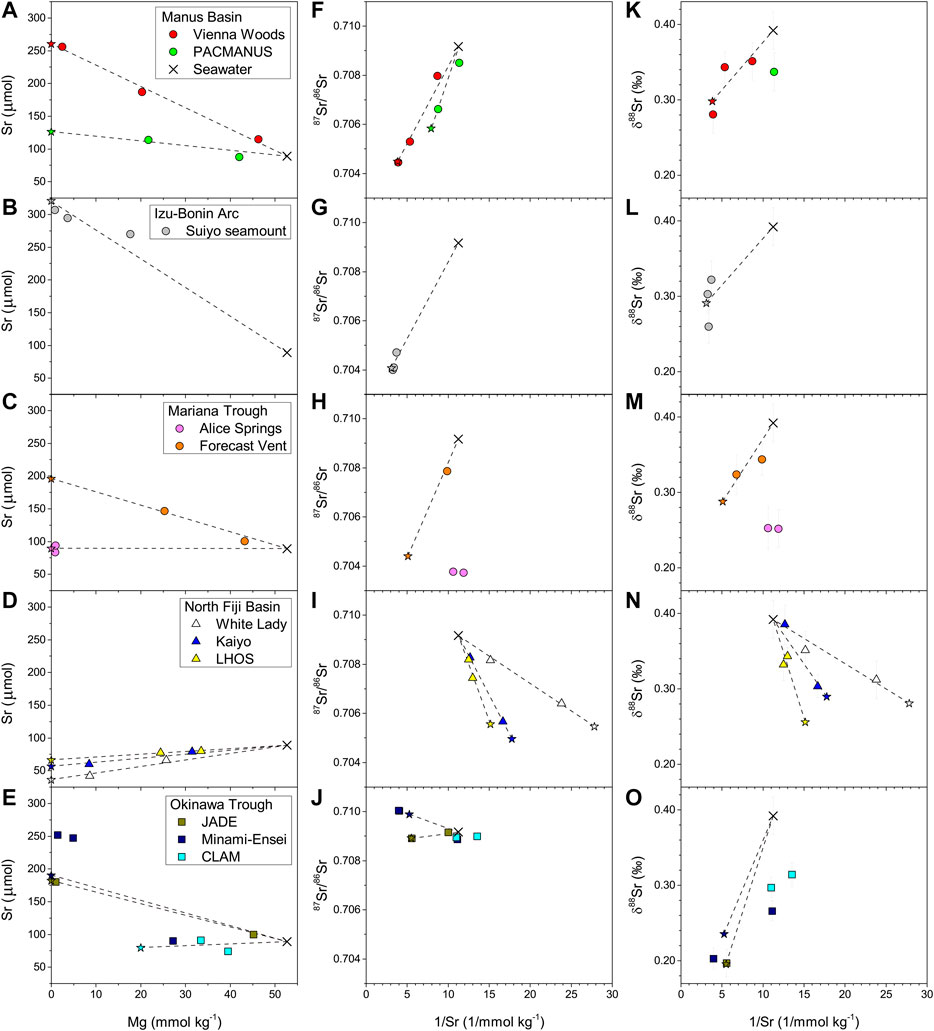
FIGURE 5. Plots of (A–E) [Sr2+] vs. [Mg2+], and (F–J)87Sr/86Sr and (K–O)δ88Sr vs. 1/[Sr2+] in hydrothermal fluid samples. End-member elemental and isotopic compositions (stars) for individual vents were estimated by linear regression forced through seawater values (crosses) and by extrapolating the observed Sr-Mg linear regression line to Mg = 0, except at the CLAM site, where it was extrapolated to Mg = 20 mM (Gamo et al., 1991).
Compared to seawater values (Sr = 89 μmol/kg, δ88Sr = 0.392‰, and 87Sr/86Sr = 0.709, 172), the end-member fluids of the sediment-starved sites were characterized by higher Sr concentrations of up to 320 μmol/kg and lower δ88Sr and 87Sr/86Sr values of 0.29–0.30‰ and 0.7041–0.7058, respectively. Slight differences from the 87Sr/86Sr values reported by Araoka et al. (2016) are because the regression line was forced through the seawater end point. Most Sr isotopic end-member values for samples from the phase-separated sites were similar to those of samples from the sediment-starved sites, but the Sr concentrations were notably lower. The δ88Sr end-member of most sediment-starved sites is in good agreement with MORB composition. The global marine δ88Sr and 87Sr/86Sr fluxes are assessed by an intercept point between 1) a fractionation line between seawater-carbonate and 2) a binary mixing line of hydrothermal-river endmembers (Figure 6; Krabbenhöft et al., 2010; Pearce et al., 2015a). The LHOS δ88Sr value (0.26‰) was lower than the values at the sediment-starved and other phase-separated sites. The sediment-hosted sites in the Okinawa Trough exhibited markedly high 87Sr/86Sr (0.7089–0.7099) and low δ88Sr (0.20–0.24‰) values, and Sr concentrations varied greatly among these sites (Table 2). The end-member values for the sediment-hosted sites are close to the value of marine biogenic carbonates (δ88Sr = 0.150‰, 87Sr/86Sr = 0.70917) rather than that of igneous rocks (Figure 6).
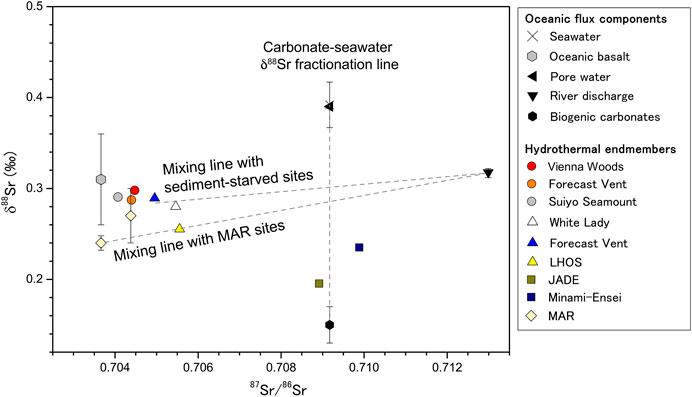
FIGURE 6. δ88Sr plotted against 87Sr/86Sr for hydrothermal end members of western Pacific hydrothermal vents and for the Mid-Atlantic Ridge (Krabbenhöft et al., 2010; Pearce et al., 2015a) along with major oceanic flux components reported by Pearce et al. (2015a).
Discussion
Host Rock and Fractionation Controls on δ88Sr in Sediment-Starved Systems
The data obtained in this study provide insights into the control of δ88Sr in end-member fluids associated with hydrothermal systems with varied host rock compositions and with or without phase separation. The Vienna Woods field in the Manus Basin is a basalt-hosted hydrothermal system in MORB-like basaltic rocks (Dril et al., 1997; Gamo et al., 1997). In contrast, the host rocks of the Pacmanus field in the Manus Basin and the Suiyo Seamount site in the Izu-Bonin Arc are arc-type dacitic rocks. The geochemical characteristics of the Mariana Trough basalts reflect a mixing of melts in the source region prior to eruption (Volpe et al., 1987) with abundant CO2 derived from subducting slab material (Gamo et al., 1997). Among these sites, the dacite-hosted Pacmanus site alone has a high 87Sr/86Sr value of 0.7058. The δ88Sr end-member values at the five sediment-starved sites in these hydrothermal fields are similar at approximately 0.29‰ (Table 2). The estimated δ88Sr end-member values are 0.02–0.04‰ higher than those of the MAR reported by Krabbenhöft et al. (2010) and Pearce et al. (2015a). Amsellem et al. (2018) recently demonstrated that igneous processes have a limited effect on 88Sr/86Sr value of MORB and also on ocean island basalts over a wide range of MgO contents. The δ88Sr value of MORB is 0.31 ± 0.05‰, which is close to those of the bulk silicate earth (0.30 ± 0.02‰) and carbonaceous chondrites (0.29 ± 0.06‰) (Amsellem et al., 2018). Regarding different host-rock types, basalts, granites, and andesites have similar average values (Moynier et al., 2010; Charlier et al., 2012; Amsellem et al., 2018). The calculated end-member fluids of the sediment-starved sites (0.284 ± 0.030‰) suggest that δ88Sr is essentially independent of rock type (basalt, dacite) and hydrothermal setting (volcanic front, back-arc basin). Therefore, the δ88Sr values of end-member fluids for each site mainly reflect dissolved Sr sourced from host rocks.
We observed little systematic offset at two phase-separated sites, White Lady and Kaiyo, relative to the sediment-starved sites, which suggests that the phase separation and segregation has minimal impact on hydrothermal δ88Sr values. The three North Fiji Basin sites are all hosted by MORB-like basalt (Ishibashi et al., 1994b; Yamaoka et al., 2015) and have similar physicochemical conditions (Table 2) with their δ88Sr values being in the range of oceanic basalt (Amsellem et al., 2018).
The LHOS field in the North Fiji Basin had a slightly lower δ88Sr end-member value (0.26‰) compared with those of the other phase-separated sites. We wish to address the possibility that it is lower due to the impact of anhydrite precipitation/re-dissolution, as well as hydrothermal calcite veins. Voigt et al. (2018) conducted an experiment, demonstrating that the formation of hydrothermal anhydrite and the preferential dissolution of 86Sr-enriched minerals occurs during seawater-basalt reactions at 250°C and 290°C; together, these processes account for 0.07–0.18‰ decrease in the δ88Sr values of fluids after a few months of reaction time between seawater and basaltic materials. Because Sr2+ co-precipitation in anhydrite serves as the main Sr sink in hydrothermal reactions, we used Ca2+ and SO42– concentrations to infer the contributions of rock dissolution and anhydrite precipitation (Figure 7A) to the end-member δ88Sr values. The vectors in Figure 7A show the directions associated with anhydrite precipitation (a decrease in the 1:1 M ratio) and rock dissolution (predominantly the release of Ca2+) on the scatter diagram of Ca2+ and SO42. The overall magnitude of the rock dissolution effect relative to the anhydrite precipitation effect was less at phase-separated sites than those of the other sediment-starved sites (Figure 7A). An impact of anhydrite precipitation on the lower δ88Sr values of LHOS site was also evaluated using a simple closed/semi-closed type fractionation (Supplementary Figure S2). The anhydrite-fluid fractionation factor εanhydrite-fluid of 0.034 ± 0.019‰ (Voigt et al., 2018) was used for calculation. We considered two extreme cases: 1) anhydrite precipitation from recharged seawater (0.392‰), and 2) anhydrite precipitation from end-member fluids of other sediment-starved sites in the North Fiji Basin (0.285‰). In the former case, 98% removal of Sr by anhydrite is necessary to obtain the LHOS end-member of 0.256‰; in the latter case, 58% is necessary. However, Sr concentrations of the LHOS end-member (66 μmol/kg) is about half of that of seawater (89 μmol/kg), and higher than other end-members in the North Fiji Basin (36 μmol/kg for White Lady, 56 μmol/kg for Kaiyo, Table 2). Because of the similarity of the end-member δ88Sr values among the sediment-starved sites, Sr co-precipitation with anhydrite cannot by itself account for the lower δ88Sr end-member value at the LHOS site. Therefore, the variation in δ88Sr value of oceanic basalt (0.31 ± 0.05‰, Amsellem et al., 2018) would be an explanation for the lower δ88Sr value of LHOS site. Similar to anhydrite, the impact of Sr co-precipitation within hydrothermal calcite veins is also considered to be negligible, as calculated from the magnitude of Ca depletion observed in the hydrothermal fluids and the partitioning coefficient for Sr to Ca ratios (Rimstidt et al., 1998).
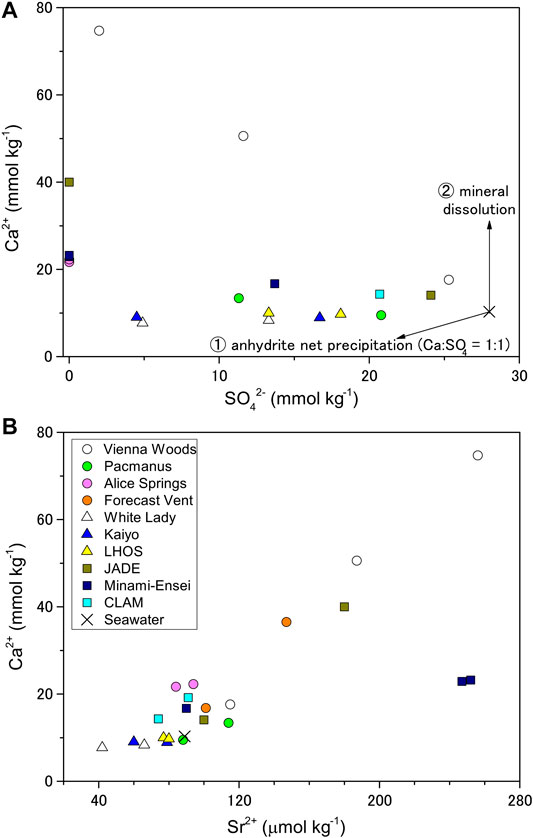
FIGURE 7. Plots of [Ca2+] vs. (A) [SO42–] and (B) [Sr2+] for the western Pacific hydrothermal vents. Two vectors in the Ca2+-SO42– plane explain the expected concentration changes due to anhydrite precipitation and mineral dissolution of host rocks and sediments.
The difference between the flux-weighted marine δ88Sr-87Sr/86Sr input and the intercept value has been attributed to an elevated supply of riverine Sr to the ocean due to post-glacial weathering (Figure 6; Krabbenhöft et al., 2010; Pearce et al., 2015a). Our data imply that the mean marine δ88Sr composition of hydrothermal input may be closer to an average oceanic crustal value of approximately 0.30‰ (Amsellem et al., 2018), as it gives a higher intercept δ88Sr value (Figure 6).
Influence of Host Sediments on Okinawa Trough Hydrothermal Fluids
The Sr isotopes in the end-member hydrothermal fluids from the sediment-hosted vents in the Okinawa Trough have 87Sr/86Sr values distinctly higher than the other vent (approximately 0.710–0.709 vs. < 0.705, Noguchi et al., 2009) as well as distinctly lower δ88Sr values (approximately 0.22 vs. 0.28‰). The higher 87Sr/86Sr values most likely reflect hydrothermal interaction with the clastic fraction of the host sediments. Unlike the thick sedimentary sequences that overlie basaltic crust on the flanks of mid-ocean ridges, the chemical properties of fluids from sediment-hosted sites strongly reflect contributions of sediment-derived components; e.g., high CH4 and NH4 in the fluids (Sakai et al., 1990a; Sakai et al., 1990b; Gamo et al., 1991; Chiba et al., 1993; Kawagucci et al., 2013). These Okinawa Trough samples also have extremely high B and Cs concentrations, derived predominantly from clay minerals, and relatively low δ11B values (Yamaoka et al., 2015). δ11B are characterized by a strong correlation with δ88Sr (R2 = 0.82, Figure 8 is readily leached out from the solid phase by fluids at high temperatures (You et al., 1994; Yamaoka et al., 2015), suggesting extensive water-sediment interaction in the Okinawa Trough. The hydrothermal system in the Okinawa Trough is sourced from complex migration networks for recharge and discharge via normal faults that extend from the seafloor to crustal basement and pore fluids in sediments of a thickness of about a few km (Figure 4; Tsuji et al., 2012).
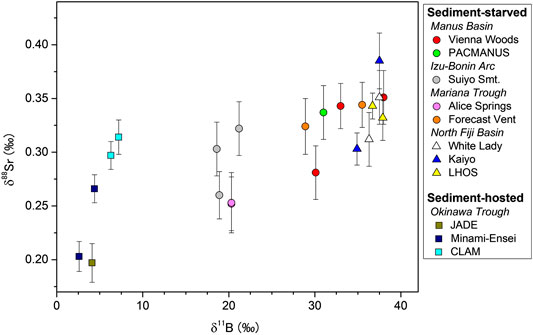
FIGURE 8. δ88Sr vs. δ11B in hydrothermal waters from western Pacific sites. The boron data were previously reported by Yamaoka et al. (2015).
The δ88Sr of a wide range of silicate rocks show nearly identical values around 0.28–0.30‰ (Moynier et al., 2010; Amsellem et al., 2018). The only solid-phase reservoir with distinctly lower δ88Sr values are carbonates, suggesting the lower δ88Sr in the Okinawa hydrothermal fluids likely reflects Sr inputs from carbonates in the host sediments (Figures 1,6). Some continental carbonate rocks are more depleted in 88Sr down to δ88Sr of –0.4‰ (Figure 1; Ohno et al., 2008). Potential sources of Sr that can account for the decrease in the δ88Sr are marine biogenic carbonate (0.15–0.23‰, Krabbenhöft et al., 2010; Böhm et al., 2012; Stevenson et al., 2014; Pearce et al., 2015a; Voigt et al., 2015, 87Sr/86Sr = 0.7089–0.7092 for Late Miocene to Holocene biogenic CaCO3, McArthur et al., 2001) in trough-filling hemipelagic sediments. In turn, this suggests that the actual 87Sr/86Sr value of the detrital component in sediment is higher than the measured >0.709 originated from catchments of the Yangtze and Yellow Rivers. The sediment-hosted vent fluids are affected by variable contributions from sedimentary silicate and carbonate sources. The combined use of 87Sr/86Sr and δ88Sr demonstrated that the main Sr sources in overlying sediments are carbonates.
Despite the similar sedimentary origins of D621-5, D622-1 and D622-2, two vents at the Minami-Ensei site, the chemical data differ considerably between them (Table 1). The chemical characteristics of the D622-2 vent fluid, including the end member Sr concentration of 252 μM, are comparable to those of the vent fluids of Minami-Ensei Knoll reported by Kawaguchi et al. (2013). Although D621-5 is chemically similar to the other sediment-hosted samples, D622-2 exhibited higher Sr/Ca ratios and 87Sr/86Sr values that were trend outliers. Previous studies have reported that the fluid/sediment mass ratio during interactions of circulating hydrothermal fluids with terrigenous sediments determines the leaching of fixed and adsorbed trace elements from sediments (Yamaoka et al., 2015; Araoka et al., 2016). Therefore, inter-vent variability is also related to heterogeneity of the sediments (such as relative abundance of hemipelagic sediments to volcanic deposits) and the scales of subseafloor hydrothermal fluid flow structures at sediment-hosted sites.
Conclusion
We investigated δ88Sr in submarine vent fluids from arc and back-arc hydrothermal systems in the western Pacific. The important observation of this study is that the end-member δ88Sr values (0.256–0.298‰, calculated by extrapolating the linear regression line fitted to data from two or three samples from each site to a Mg concentration of zero) are similar to the average value of oceanic volcanic rocks, about 0.28–0.31‰ (Amsellem et al., 2018). This result confirms the utility of the δ88Sr mass balance for past changes in the marine carbonate system (Krabbenhöft et al., 2010; Vollstaedt et al., 2014; Pearce et al., 2015a), and supports the view that hydrothermal end-member in sediment-starved hydrothermal systems is probably a unique δ88Sr value through geologic time because of the similarity and homogeneity of stable Sr isotopes in between igneous rocks as well as carbonaceous chondrites (Amsellem et al., 2018). The decreased δ88Sr values, as low as 0.20‰, accompanied by higher 87Sr/86Sr, in vent fluids of the sediment-hosted sites are linked to the release of Sr from sedimentary materials, that we find to be sensitive to the extent of mixing between the different sources and sinks of sedimentary Sr phases. A global average of hydrothermal δ88Sr value would be reassessed with taking into account these data and other representative hydrothermal system, such as the East Pacific Rise.
Data Availability Statement
The original contributions presented in the study are included in the article/Supplementary Material, further inquiries can be directed to the corresponding author.
Author Contributions
TY, SW, and HK contributed to conception of the study. Samples were collected by TG. TY, SW, and TI performed the isotopic analysis. DA performed the trace metal analysis. TY wrote the first draft of the manuscript with input from NO, TI, and SW. SW wrote the analytical sections of the manuscript. All authors contributed to manuscript revision, read, and approved the submitted version.
Funding
This work was undertaken with the support of Japan Society for the Promotion of Science (JSPS) to TY (no. 16H05883, 19K21908), to SW (no. 18K03814) and to TG (no. 16H02701).
Conflict of Interest
The authors declare that the research was conducted in the absence of any commercial or financial relationships that could be construed as a potential conflict of interest.
Acknowledgments
We thank Kazuya Nagaishi and Tatsuya Kawai of Marine Work Japan for their support during the analysis. The suggestions of Thomas M. Blattmann greatly improved the language. The comments of the reviewers are gratefully acknowledged.
Supplementary Material
The Supplementary Material for this article can be found online at: https://www.frontiersin.org/articles/10.3389/feart.2020.591711/full#supplementary-material
References
Amsellem, E., Moynier, F., Day, J. M. D., Moreira, M., Puchtel, I. S., and Teng, F.-Z. (2018). The stable strontium isotopic composition of ocean island basalts, mid-ocean ridge basalts, and komatiites. Chem. Geol. 483, 595–602. doi:10.1016/j.chemgeo.2018.03.030
Andrews, M. G., Jacobson, A. D., Lehn, G. O., Horton, T. W., and Craw, D. (2016). Radiogenic and stable Sr isotope ratios (87 Sr/86 Sr, δ 88/86 Sr) as tracers of riverine cation sources and biogeochemical cycling in the Milford Sound region of Fiordland, New Zealand. Geochem. Cosmochim. Acta. 173, 284–303. doi:10.1016/j.gca.2015.10.005
Araoka, D., Nishio, Y., Gamo, T., Yamaoka, K., and Kawahata, H. (2016). Lithium isotopic systematics of submarine vent fluids from arc and back-arc hydrothermal systems in the western Pacific. Geochem. Geophys. Geosyst. 17 (10), 3835–3853. doi:10.1002/2016gc006355
Böhm, F., Eisenhauer, A., Tang, J., Dietzel, M., Krabbenhöft, A., Kisakürek, B., et al. (2012). Strontium isotope fractionation of planktic foraminifera and inorganic calcite. Geochem. Cosmochim. Acta. 93, 300–314. doi:10.1016/j.gca.2012.04.038
Chao, H.-C., You, C.-F., Liu, H.-C., and Chung, C.-H. (2015). Evidence for stable Sr isotope fractionation by silicate weathering in a small sedimentary watershed in southwestern Taiwan. Geochem. Cosmochim. Acta. 165, 324–341. doi:10.1016/j.gca.2015.06.006
Charlier, B. L. A., Nowell, G. M., Parkinson, I. J., Kelley, S. P., Pearson, D. G., and Burton, K. W. (2012). High temperature strontium stable isotope behaviour in the early solar system and planetary bodies. Earth Planet Sci. Lett. 329-330, 31–40. doi:10.1016/j.epsl.2012.02.008
Chiba, H., Nakashima, K., Gamo, T., Ishibashi, J., Tsunogai, U., and Sakai, H. (1993). Hydrothermal activity at the Minami-Ensei Knoll, Okinawa Trough: chemical characteristics of hydrothermal solutions. Proc. JAMSTEC Symp. Deep Sea Res.
DePaolo, D. J., and Ingram, B. L. (1985). High-resolution stratigraphy with strontium isotopes. Science 227 (4689), 938–941. doi:10.1126/science.227.4689.938
Dril, S. I., Kuzmin, M. I., Tsipukova, S. S., and Zonenshain, L. P. (1997). Geochemistry of basalts from the western Woodlark, Lau and Manus basins: implications for their petrogenesis and source rock compositions. Mar. Geol. 142 (1–4), 57–83. doi:10.1016/s0025-3227(97)00041-8
Eissen, J.-P., Lefevre, C., Maillet, P., Morvan, G., and Nohara, M. (1991). Petrology and geochemistry of the central North Fiji basin spreading centre (southwest pacific) between 16 S and 22 S. Mar. Geol. 98, 2–4. doi:10.1016/0025-3227(91)90104-c
Elderfield, H., and Schultz, A. (1996). Mid-ocean ridge hydrothermal fluxes and the chemical composition of the ocean. Annu. Rev. Earth Planet Sci. 24, 191–224. doi:10.1146/annurev.earth.24.1.191
Eom, J., Yoshimura, T., Araoka, D., Gamo, T., and Kawahata, H. (2020). Magnesium isotopic composition of submarine vent fluids from arc and back-arc hydrothermal systems in the western Pacific. Chem. Geol. 551, 119767. doi:10.1016/j.chemgeo.2020.119767
Fruchter, N., Eisenhauer, A., Dietzel, M., Fietzke, J., Böhm, F., Montagna, P., et al. (2016). 88Sr/86Sr fractionation in inorganic aragonite and in corals. Geochem. Cosmochim. Acta. 178, 268–280. doi:10.1016/j.gca.2016.01.039
Gamo, T., Chiba, H., Fryer, P., Ishibashi, J., Ishii, T., Johnson, L. E., et al. (1994). Mariana 1992 diving surveys by Shinkai 6500 (Y9204 cruise): revisits to the mid-Mariana Trough hydrothermal area and discovery of hydrothermal vents in the southern Mariana region. Proc. JAMSTEC Symp. Deep Sea Res. 10, 153–162.
Gamo, T., Sakai, H., Kim, E.-S., Shitashima, K., and Ishibashi, J.-i. (1991). High alkalinity due to sulfate reduction in the CLAM hydrothermal field, Okinawa Trough. Earth Planet Sci. Lett. 107 (2), 328–338. doi:10.1016/0012-821x(91)90080-2
Gamo, T., Tsunogai, U., Ishibashi, J., Masuda, H., and Chiba, H. (1997). Chemical characteristics of hydrothermal fluids from the Mariana Trough. Jamstec J. Deep Sea Res., 69–74
Grimaud, D., Ishibashi, J.-I., Lagabrielle, Y., Auzende, J.-M., and Urabe, T. (1991). Chemistry of hydrothermal fluids from the 17S active site on the north Fiji basin ridge (SW pacific). Chem. Geol. 93 (3-4), 209–218. doi:10.1016/0009-2541(91)90114-7
Halbach, P., Nakamura, K.-I., Wahsner, M., Lange, J., Sakai, H., Käselitz, L., et al. (1989). Probable modern analogue of Kuroko-type massive sulphide deposits in the Okinawa Trough back-arc basin. Nature 338 (6215), 496–499. doi:10.1038/338496a0
Halicz, L., Segal, I., Fruchter, N., Stein, M., and Lazar, B. (2008). Strontium stable isotopes fractionate in the soil environments?. Earth Planet Sci. Lett. 272 (1-2), 406–411. doi:10.1016/j.epsl.2008.05.005
Hardie, L. A. (1996). Secular variation in seawater chemistry: an explanation for the coupled secular variation in the mineralogies of marine limestones and potash evaporites over the past 600 m.y. Geol. 24 (3), 279–283. doi:10.1130/0091-7613(1996)024<0279:svisca>2.3.co;2
Hodell, D. A., Mead, G. A., and Mueller, P. A. (1990). Variation in the strontium isotopic composition of seawater (8 Ma to present): implications for chemical weathering rates and dissolved fluxes to the oceans. Chem. Geol. Isot. Geosci. 80 (4), 291–307. doi:10.1016/0168-9622(90)90011-z
Ishibashi, J. (1994). Chemical composition of hydrothermal fluids from the Suiyo and the mokuyo seamounts, Izu-Bonin Arc. JAMSTEC Deep Sea Res. 10, 89–97.
Ishibashi, J.-I., Grimaud, D., Nojiri, Y., Auzende, J.-M., and Urabe, T. (1994a). Fluctuation of chemical compositions of the phase-separated hydrothermal fluid from the North Fiji Basin Ridge. Mar. Geol. 116 (1-2), 215–226. doi:10.1016/0025-3227(94)90177-5
Ishibashi, J.-I., and Urabe, T. (1995). Hydrothermal activity related to arc-backarc magmatism in the western pacific, in Backarc basins. Editor B., Taylor (Boston, MA: Springer), 451–495. doi:10.1007/978-1-4615-1843-3_13
Ishibashi, J.-I., Wakita, H., Nojiri, Y., Grimaud, D., Jean-Baptiste, P., Gamo, T., et al. (1994b). Helium and carbon geochemistry of hydrothermal fluids from the North Fiji Basin spreading ridge (southwest Pacific). Earth Planet Sci. Lett. 128 (3-4), 183–197. doi:10.1016/0012-821x(94)90144-9
Ishibashi, J., Sano, Y., Wakita, H., Gamo, T., Tsutsumi, M., and Sakai, H. (1995). Helium and carbon geochemistry of hydrothermal fluids from the mid-Okinawa Trough back arc basin, southwest of Japan. Chem. Geol. 123 (1–4), 1–15. doi:10.1016/0009-2541(95)00051-m
Izawa, E., Motomura, Y., Tanaka, T., and Kimura, M. (1991). Hydrothermal carbonate chimneys in the Iheya Ridge of the Okinawa Trough. JAMSTEC Deep Sea Res. 7, 185–192.
Kawagucci, S., Ueno, Y., Takai, K., Toki, T., Ito, M., Inoue, K., et al. (2013). Geochemical origin of hydrothermal fluid methane in sediment-associated fields and its relevance to the geographical distribution of whole hydrothermal circulation. Chem. Geol. 339, 213–225. doi:10.1016/j.chemgeo.2012.05.003
Krabbenhöft, A., Eisenhauer, A., Böhm, F., Vollstaedt, H., Fietzke, J., Liebetrau, V., et al. (2010). Constraining the marine strontium budget with natural strontium isotope fractionations (87Sr/86Sr∗, δ88/86Sr) of carbonates, hydrothermal solutions and river waters. Geochem. Cosmochim. Acta., 74 (14), 4097–4109. doi:10.1016/j.gca.2010.04.009
Krabbenhöft, A., Fietzke, J., Eisenhauer, A., Liebetrau, V., Böhm, F., and Vollstaedt, H. (2009). Determination of radiogenic and stable strontium isotope ratios (87Sr/86Sr; δ88/86Sr) by thermal ionization mass spectrometry applying an 87Sr/84Sr double spike. J. Anal. At. Spectrom. 24, 1267–1271. doi:10.1039/b906292k
Lisitsyn, A. P., Crook, K. A. W., Bogdanov, Y. A., Zonenshayn, L. P., Murav’yev, K. G., Tufar, W., et al. (1993). A hydrothermal field in the rift zone of the Manus Basin, Bismarck Sea. Int. Geol. Rev. 35 (2), 105–126. doi:10.1080/00206819309465517
Liu, H.-C., You, C.-F., Zhou, H., Huang, K.-F., Chung, C.-H., Huang, W.-J., et al. (2017). Effect of calcite precipitation on stable strontium isotopic compositions: insights from riverine and pool waters in a karst cave. Chem. Geol. 456, 85–97. doi:10.1016/j.chemgeo.2017.03.008
McArthur, J. M., Howarth, R. J., and Bailey, T. R. (2001). Strontium isotope stratigraphy: LOWESS version 3: best fit to the marine Sr‐isotope curve for 0-509 Ma and accompanying look‐up table for deriving numerical age. J. Geol. 109 (2), 155–170. doi:10.1086/319243
Moynier, F., Agranier, A., Hezel, D. C., and Bouvier, A. (2010). Sr stable isotope composition of Earth, the Moon, Mars, Vesta and meteorites. Earth Planet Sci. Lett. 300 (3–4), 359–366. doi:10.1016/j.epsl.2010.10.017
Nakamura, K., and Takai, K.(2015). Geochemical constraints on potential biomass sustained by subseafloor water-rock interactions, in Subseafloor biosphere linked to hydrothermal systems. Editor M., Sunamura (Tokyo, Japan:Springer), 11–30. doi:10.1007/978-4-431-54865-2_2
Neymark, L. A., Premo, W. R., Mel’nikov, N. N., and Emsbo, P. (2014). Precise determination of δ88Sr in rocks, minerals, and waters by double-spike TIMS: a powerful tool in the study of geological, hydrological and biological processes. J. Anal. At. Spectrom. 29 (1), 65–75. doi:10.1039/c3ja50310k
Noguchi, T., Shinjo, R., Ito, M., Takada, J., and Oomori, T. (2009). Barite geochemistry from hydrothermal chimneys of the Okinawa Trough: insight into chimney formation and fluid/sediment interaction. J. Mineral. Petrol. Sci. 106 (1), 26–35. doi:10.2465/jmps.090825
Nohara, M., Hirose, K., Eissen, J. P., Urabe, T., and Joshima, M. (1994). The North Fiji Basin basalts and their magma sources: Part II. Sr-Nd isotopic and trace element constraints. Mar. Geol. 116 (1–2), 179–195. doi:10.1016/0025-3227(94)90175-9
Ohno, T., and Hirata, T. (2007). Simultaneous determination of mass-dependent isotopic fractionation and radiogenic isotope variation of strontium in geochemical samples by multiple collector-ICP-mass spectrometry. Anal. Sci. 23 (11), 1275–1280. doi:10.2116/analsci.23.1275
Ohno, T., Komiya, T., Ueno, Y., Hirata, T., and Maruyama, S. (2008). Determination of 88Sr/86Sr mass-dependent isotopic fractionation and radiogenic isotope variation of 87Sr/86Sr in the Neoproterozoic Doushantuo Formation. Gondwana Res. 14 (1–2), 126–133. doi:10.1016/j.gr.2007.10.007
Pearce, C. R., Parkinson, I. J., Gaillardet, J., Charlier, B. L. A., Mokadem, F., and Burton, K. W. (2015a). Reassessing the stable (δ88/86Sr) and radiogenic (87Sr/86Sr) strontium isotopic composition of marine inputs. Geochem. Cosmochim. Acta. 157, 125–146. doi:10.1016/j.gca.2015.02.029
Pearce, C. R., Parkinson, I. J., Gaillardet, J., Chetelat, B., and Burton, K. W. (2015b). Characterising the stable (δ88/86Sr) and radiogenic (87Sr/86Sr) isotopic composition of strontium in rainwater. Chem. Geol. 409, 54–60. doi:10.1016/j.chemgeo.2015.05.010
Raddatz, J., Liebetrau, V., Rüggeberg, A., Hathorne, E., Krabbenhöft, A., Eisenhauer, A., et al. (2013). Stable Sr-isotope, Sr/Ca, Mg/Ca, Li/Ca and Mg/Li ratios in the scleractinian cold-water coral Lophelia pertusa. Chem. Geol. 352, 143–152. doi:10.1016/j.chemgeo.2013.06.013
Raymo, M. E., and Ruddiman, W. F. (1992). Tectonic forcing of late Cenozoic climate. Nature 359 (6391), 117. doi:10.1038/359117a0
Rimstidt, J. D., Balog, A., and Webb, J. (1998). Distribution of trace elements between carbonate minerals and aqueous solutions. Geochem. Cosmochim. Acta. 62 (11), 1851–1863. doi:10.1016/s0016-7037(98)00125-2
Rüggeberg, A., Fietzke, J., Liebetrau, V., Eisenhauer, A., Dullo, W. C., and Freiwald, A. (2008). Stable strontium isotopes (δ88/86Sr) in cold-water corals−a new proxy for reconstruction of intermediate ocean water temperatures. Earth Planet Sci. Lett. 269 (3–4), 570–575. doi:10.1016/j.epsl.2008.03.002
Sakai, H., Gamo, T., Kim, E.-S., Shitashima, K., Yanagisawa, F., Tsutsumi, M., et al. (1990). Unique chemistry of the hydrothermal solution in the mid-Okinawa Trough Backarc Basin. Geophys. Res. Lett. 17 (12), 2133–2136. doi:10.1029/gl017i012p02133
Naganuma, H., Gamo, T., Kim, E.-S., Tsutsumi, M., Tanaka, T., Ishibashi, J., et al. (1990b). Venting of carbon dioxide-rich fluid and hydrate formation in mid-Okinawa trough backarc basin. Science 248 (4959), 1093–1096. doi:10.1126/science.248.4959.1093
Shalev, N., Gavrieli, I., Halicz, L., Sandler, A., Stein, M., and Lazar, B. (2017). Enrichment of 88 Sr in continental waters due to calcium carbonate precipitation. Earth Planet Sci. Lett. 459, 381–393. doi:10.1016/j.epsl.2016.11.042
Sibuet, J.-C., Hsu, S.-K., Shyu, C.-T., and Liu, C.-S. (1995). Structural and kinematic evolutions of the Okinawa Trough backarc basin, in Backarc basins, Editor B., Taylor (Boston, MA: Springer), 343–379. doi:10.1007/978-1-4615-1843-3_9
Stevenson, E. I., Aciego, S. M., Chutcharavan, P., Parkinson, I. J., Burton, K. W., Blakowski, M. A., et al. (2016). Insights into combined radiogenic and stable strontium isotopes as tracers for weathering processes in subglacial environments. Chem. Geol. 429, 33–43. doi:10.1016/j.chemgeo.2016.03.008
Stevenson, E. I., Hermoso, M., Rickaby, R. E. M., Tyler, J. J., Minoletti, F., Parkinson, I. J., et al. (2014). Controls on stable strontium isotope fractionation in coccolithophores with implications for the marine Sr cycle. Geochem. Cosmochim. Acta. 128, 225–235. doi:10.1016/j.gca.2013.11.043
Tsuji, T., Takai, K., Oiwane, H., Nakamura, Y., Masaki, Y., Kumagai, H., et al. (2012). Hydrothermal fluid flow system around the Iheya North Knoll in the mid-Okinawa trough based on seismic reflection data. J. Volcanol. Geoth. Res. 213–214, 41–50. doi:10.1016/j.jvolgeores.2011.11.007
Tsunogai, U., Ishibashi, J., Wakita, H., Gamo, T., Watanabe, K., Kajimura, T., et al. (1994). Peculiar features of Suiyo Seamount hydrothermal fluids, Izu-Bonin Arc: differences from subaerial volcanism. Earth Planet Sci. Lett. 126 (4), 289–301. doi:10.1016/0012-821x(94)90113-9
Veizer, J., Ala, D., Azmy, K., Bruckschen, P., Buhl, D., Bruhn, F., et al. (1999). 87Sr/86Sr, δ13C and δ18O evolution of Phanerozoic seawater. Chem. Geol. 161 (1–3), 59–88. doi:10.1016/s0009-2541(99)00081-9
Voigt, J., Hathorne, E. C., Frank, M., Vollstaedt, H., and Eisenhauer, A. (2015). Variability of carbonate diagenesis in equatorial Pacific sediments deduced from radiogenic and stable Sr isotopes. Geochem. Cosmochim. Acta. 148, 360–377. doi:10.1016/j.gca.2014.10.001
Voigt, M., Pearce, C. R., Baldermann, A., and Oelkers, E. H. (2018). Stable and radiogenic strontium isotope fractionation during hydrothermal seawater-basalt interaction. Geochem. Cosmochim. Acta. 240, 131–151. doi:10.1016/j.gca.2018.08.018
Vollstaedt, H., Eisenhauer, A., Wallmann, K., Böhm, F., Fietzke, J., Liebetrau, V., et al. (2014). The Phanerozoic δ88/86Sr record of seawater: new constraints on past changes in oceanic carbonate fluxes. Geochem. Cosmochim. Acta, 128, 249–265. doi:10.1016/j.gca.2013.10.006
Volpe, A. M., Macdougall, J. D., and Hawkins, J. W. (1987). Mariana trough basalts (MTB): trace element and SrNd isotopic evidence for mixing between MORB-like and Arc-like melts. Earth Planet Sci. Lett. 82 (3-4), 241–254. doi:10.1016/0012-821x(87)90199-3
Von Damm, K. L., and Bischoff, J. L. (1987). Chemistry of hydrothermal solutions from the southern Juan de Fuca Ridge. J. Geophys. Res. 92 (B11), 11334–11346. doi:10.1029/jb092ib11p11334
Von Damm, K. L., Edmond, J. M., Measures, C. I., and Grant, B. (1985). Chemistry of submarine hydrothermal solutions at guaymas basin, gulf of California. Geochem. Cosmochim. Acta. 49 (11), 2221–2237. doi:10.1016/0016-7037(85)90223-6
Wakaki, S., Obata, H., Tazoe, H., and Ishikawa, T. (2017). Precise and accurate analysis of deep and surface seawater Sr stable isotopic composition by double-spike thermal ionization mass spectrometry. Geochem. J. 51 (3), 227–239. doi:10.2343/geochemj.2.0461
Wei, G., Ma, J., Liu, Y., Xie, L., Lu, W., Deng, W., et al. (2013). Seasonal changes in the radiogenic and stable strontium isotopic composition of Xijiang River water: implications for chemical weathering. Chem. Geol. 343, 67–75. doi:10.1016/j.chemgeo.2013.02.004
Yamaoka, K., Hong, E., Ishikawa, T., Gamo, T., and Kawahata, H. (2015). Boron isotope geochemistry of vent fluids from arc/back-arc seafloor hydrothermal systems in the western Pacific. Chem. Geol. 392, 9–18. doi:10.1016/j.chemgeo.2014.11.009
You, C. F., Butterfield, D. A., Spivack, A. J., Gieskes, J. M., Gamo, T., and Campbell, A. J. (1994). Boron and halide systematics in submarine hydrothermal systems: effects of phase separation and sedimentary contributions. Earth Planet Sci. Lett. 123 (1–3), 227–238. doi:10.1016/0012-821x(94)90270-4
Keywords: strontium, isotopes, arc, back-arc, convergent margin, hydrothermal vent fluid
Citation: Yoshimura T, Wakaki S, Ishikawa T, Gamo T, Araoka D, Ohkouchi N and Kawahata H (2020) A Systematic Assessment of Stable Sr Isotopic Compositions of Vent Fluids in Arc/Back-Arc Hydrothermal Systems: Effects of Host Rock Type, Phase Separation, and Overlying Sediment. Front. Earth Sci. 8:591711. doi: 10.3389/feart.2020.591711
Received: 05 August 2020; Accepted: 09 November 2020;
Published: 03 December 2020.
Edited by:
Julia Ribeiro, Chinese Academy of Sciences, ChinaReviewed by:
Aiguo Dong, China University of Geosciences, ChinaXianrong Zhang, Qingdao Institute of Marine Geology (QIMG), China
Copyright © 2020 Yoshimura, Wakaki, Ishikawa, Gamo, Araoka, Ohkouchi and Kawahata. This is an open-access article distributed under the terms of the Creative Commons Attribution License (CC BY). The use, distribution or reproduction in other forums is permitted, provided the original author(s) and the copyright owner(s) are credited and that the original publication in this journal is cited, in accordance with accepted academic practice. No use, distribution or reproduction is permitted which does not comply with these terms.
*Correspondence: Toshihiro Yoshimura, eW9zaGltdXJhdEBqYW1zdGVjLmdvLmpw