- 1Muséum National d'Histoire Naturelle, Sorbonne Université, CNRS UMR 7590, Institut de Minéralogie, de Physique des Matériaux et de Cosmochimie (IMPMC), Paris, France
- 2Université de Paris, Institut de physique du globe de Paris, CNRS, F-75005 Paris, France
- 3Department of Environmental Systems Science, Institute of Biogeochemistry and Pollutant Dynamics, Eidgenössische Technische Hochschule Zurich, Zurich, Switzerland
- 4Institut Universitaire de France, Paris, France
Pyrite, or iron disulfide, is the most common sulfide mineral on the Earth’s surface and is widespread through the geological record. Because sulfides are mainly produced by sulfate-reducing bacteria (SRB) in modern sedimentary environments, microorganisms are assumed to drive the formation of iron sulfides, in particular, pyrite. However, the exact role played by microorganisms in pyrite formation remains unclear and, to date, the precipitation of pyrite in microbial cultures has only rarely been achieved. The present work relies on chemical monitoring, electron microscopy, X-ray diffraction, and synchrotron-based spectroscopy to evaluate the formation of iron sulfides by the sulfate-reducing bacteria Desulfovibrio desulfuricans as a function of the source of iron, either provided as dissolved
Introduction
Iron is the fourth most abundant element on the Earth’s surface, but due to modern oxic conditions, dissolved
Pyrite (
Laboratory experiments are essential to understand the role of SRM in pyrite formation. Several studies of sulfate-reducing bacteria enrichments reported the formation of mackinawite (FeS) and greigite (
In the present contribution, the sulfate-reducing bacterium Desulfovibrio desulfuricans was cultured with either dissolved
Materials and Methods
Culture and Biomineralization Conditions
All solution-preparation methods and manipulations were performed in a Jacomex® glove box under Ar (Alphagaz 1, Air Liquide) free of
The sulfate-reducing bacterium Desulfovibrio desulfuricans DSM642 (DSMZ, Germany) (Beijerinck, 1895) was pre-cultured in an anoxic iron-free medium composed of 2.84 g
Once cells achieved the logarithmic phase of growth (around 1 week), they were centrifuged at 7,000 g for 10 min, rinsed three times in Milli-Q water, and transferred into a biomineralization medium at 50 % (v/v), corresponding to a final cell concentration of approximately 5 × 107 cells mL-1. The biomineralization medium was prepared in the same way as pre-culture medium but contained only
Chemical Monitoring
Biomineralization experiments and abiotic controls were sampled periodically to monitor the chemical evolution of the liquid medium during biomineralization. Aliquots of 3 mL were collected from the 75-mL serum vials with syringes and needles in the glove box. Samples for total and dissolved (0.2 µm-filtered; Merck Millipore) iron were fixed with HCl to a final concentration of 0.5 M. Samples for total and dissolved sulfide were fixed with zinc acetate to a 0.5 M concentration. A separate filtered aliquot was stored in the dark at 4 °C for dissolved phosphate and/or organic acid analyses.
Ferrous iron was analyzed spectrophotometrically with the ferrozine method (Stookey, 1970). Total iron was quantified by both a modified ferrozine method (Viollier et al., 2000) and ICP-AES (Perkin Elmer Optima 3,000). Sulfide concentrations were determined spectrophotometrically using the methylene blue method (Cline, 1969). Sulfate analysis was performed by ion chromatography (Dionex DX-600 IC System). Dissolved phosphate was measured spectrophotometrically using Biomol® Green Reagent (Enzo Life Sciences). Organic acids were detected by high-performance liquid chromatography (HPLC) on a U3000 Thermo Scientific series using a Rezex organic acid column (250 × 4.6 mm, 8 μm) with 5 mM
Mineralogical Characterization
At selected time points, around 10 mL of suspended matter was collected by centrifugation at 7,000 g for 10 min and rinsed three times with deoxygenated Milli-Q water, and subsamples were deposited on a Si(111) wafer for X-ray diffraction (XRD), on a 200-mesh Formvar carbon copper grid (Agar Scientific, United Kingdom) for transmission electron microscopy (TEM), and on a silicon nitride window (Norcada, Canada) for scanning transmission X-ray microscopy (STXM). Scanning electron microscopy (SEM) samples were prepared by filtering 10–100 μL of the culture through a polycarbonate GTTP 0.2 μm filter (Merck Millipore, Darmstadt, Germany) and then rinsing with 10 mL of deoxygenated Milli-Q water. All samples were dried in the glove box and stored under anoxic conditions until analysis.
X-Ray Diffraction
Silicon wafers were mounted in an airtight cell equipped with a Kapton window designed for X-ray diffraction analyses under anoxic conditions. Diffraction patterns were acquired from 5° to 95° with a 2θ step of 0.033°, at 40 kV and 40 mA with Co Kα radiation on a PANalytical X’Pert Pro MPD diffractometer equipped with an X’celerator® detector mounted in Bragg-Brentano configuration.
Electron Microscopy
For scanning electron microscopy analyses, filters were mounted onto pin stubs with an adhesive carbon tape and then carbon coated before analysis in a Zeiss Ultra 55 scanning electron microscope equipped with a field emission gun (FEG) and a Brucker EDS Quantax detector (Brucker Corporation, Houston, TX, USA). Images were preferentially acquired under low-voltage conditions at 3 kV and 3 mm working distance with either a high-performance annular detector (secondary electrons) or an annular detector with filter grids (backscattered electrons). Higher-voltage images were acquired at 15 kV and 7.5 mm working distance with an Everhart Thornley detector (secondary electrons) or an angle selective backscattered detector. Elemental characterizations were performed by energy dispersive X-ray spectrometry (EDS) at 15 kV and 7.5 mm working distance.
For transmission electron microscopy analyses, copper grids were observed under a JEOL 2100F transmission electron microscope equipped with a field emission gun (FEG) operating at 200 kV. Selected-area electron diffraction (SAED) patterns were acquired on areas of interest. Scanning transmission electron microscopy (STEM) was performed in the high-angle annular dark field (HAADF) mode and coupled to energy dispersive X-ray spectrometry (EDS) mapping.
Scanning Transmission X-Ray Microscopy
Scanning transmission X-ray microscopy analyses at the C K-edge and Fe
Results
Chemical Evolution
Desulfovibrio desulfuricans was cultivated in two different biomineralization media containing 20 mM sulfate and 20 mM lactate, with initial iron provided either as 20 mM dissolved
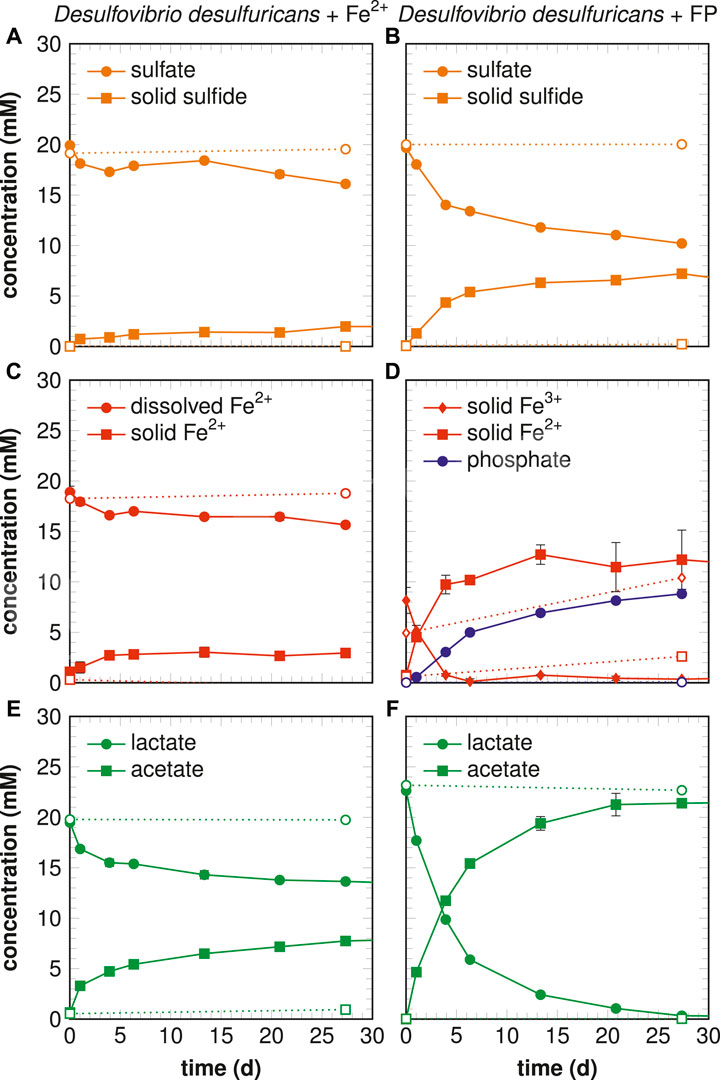
FIGURE 1. Chemical compound concentrations in biotic (filled solid) and abiotic (open dotted) experiments for dissolved
Mineralogical Characterization at the Bulk Scale
Solid phases were analyzed by X-ray diffraction (Figure 2). In the Fe-diss experiment, precipitates formed after 1 week of incubation were mostly amorphous with only a tiny peak at 20° 2θ angle (Co Kα), characteristic of mackinawite (FeS). After 1 month, mackinawite increased in crystallinity, as illustrated by several well-resolved peaks (Fe-diss-1m in Figure 2). In the FP-nano experiment, initial nanoparticulate FeIII-phosphate was amorphous. After 1 week, vivianite (
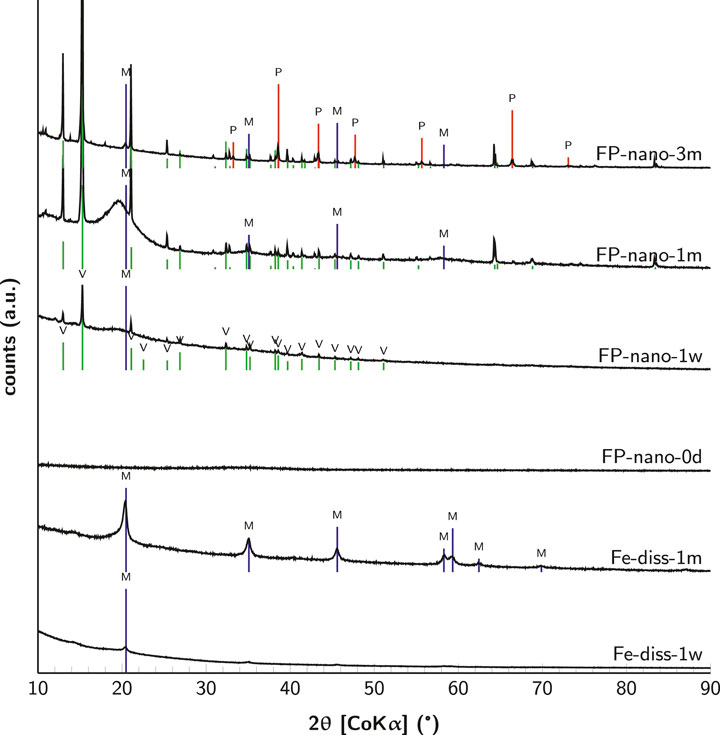
FIGURE 2. X-ray diffractograms of solid phases in cultures after 1 week, 1 month, and 3 months as well as of initial nanoparticulate FeIII-phosphate. Characteristic peaks of reference minerals are indexed for mackinawite (blue), vivianite (green), and pyrite (red).
Microscopy Analyses of Mineral-Organic Assemblages
Fe-Diss Condition
Scanning electron microscopy and transmission electron microscopy observations of the products formed in the Fe-diss experiment revealed the presence of micrometer-scale aggregates of flake-like nanoparticles of iron sulfide at both 1 week and 1 month (Figures 3C,F,I, 4A,D,E,H). After 1 week, the presence of mackinawite was confirmed by the polycrystalline diffraction pattern (Figure 4B) and the high-resolution images obtained by transmission electron microscopy, showing the 5 Å d-spacing characteristic of mackinawite (Figure 4C). Moreover, bacteria could be observed by scanning electron microscopy and transmission electron microscopy in association with mackinawite (Figures 3C, 4A,D). After 1 month, the increase in mackinawite crystallinity was confirmed by single-crystal diffraction patterns (Figure 4F). In these 1-month old samples, the size and shape of mackinawite aggregates suggested the presence of encrusted cells (Figure 4E).
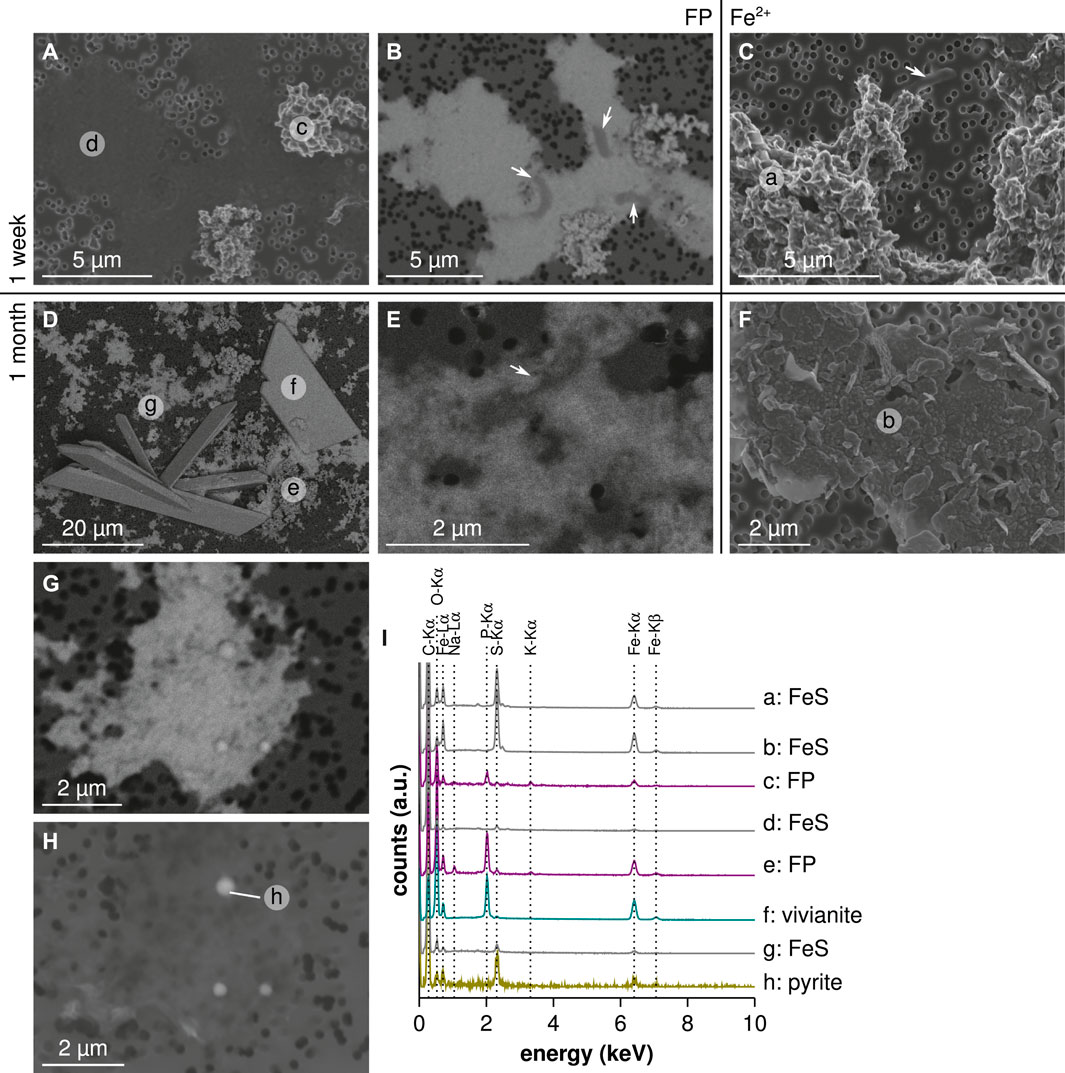
FIGURE 3. SEM observations of precipitates obtained in both conditions, after 1 week and 1 month. (A,B) Comparison of secondary and backscattered electron images showing amorphous iron sulfide association with bacteria for FP-nano condition at 1 week. (C) Secondary electron images of Fe-diss condition at 1 week. (D) Large backscattered electron image at 1 month in FP-nano condition. (E) Backscattered electron image showing a bacterium within iron sulfide film at 1 month in FP-nano condition. (F) Secondary electron images of Fe-diss condition at 1 month. (G,H) Comparison of backscattered and secondary electron images showing pyrite spherules nucleating within the iron sulfide film at 1 month in FP-nano condition. (I) Energy dispersive X-ray spectrometry spectra pinned from previous SEM images. Letters in energy dispersive X-ray spectrometry maps correspond to energy dispersive X-ray spectrometry spectra provided in (I). Except for (H), all images were acquired in low-voltage conditions.
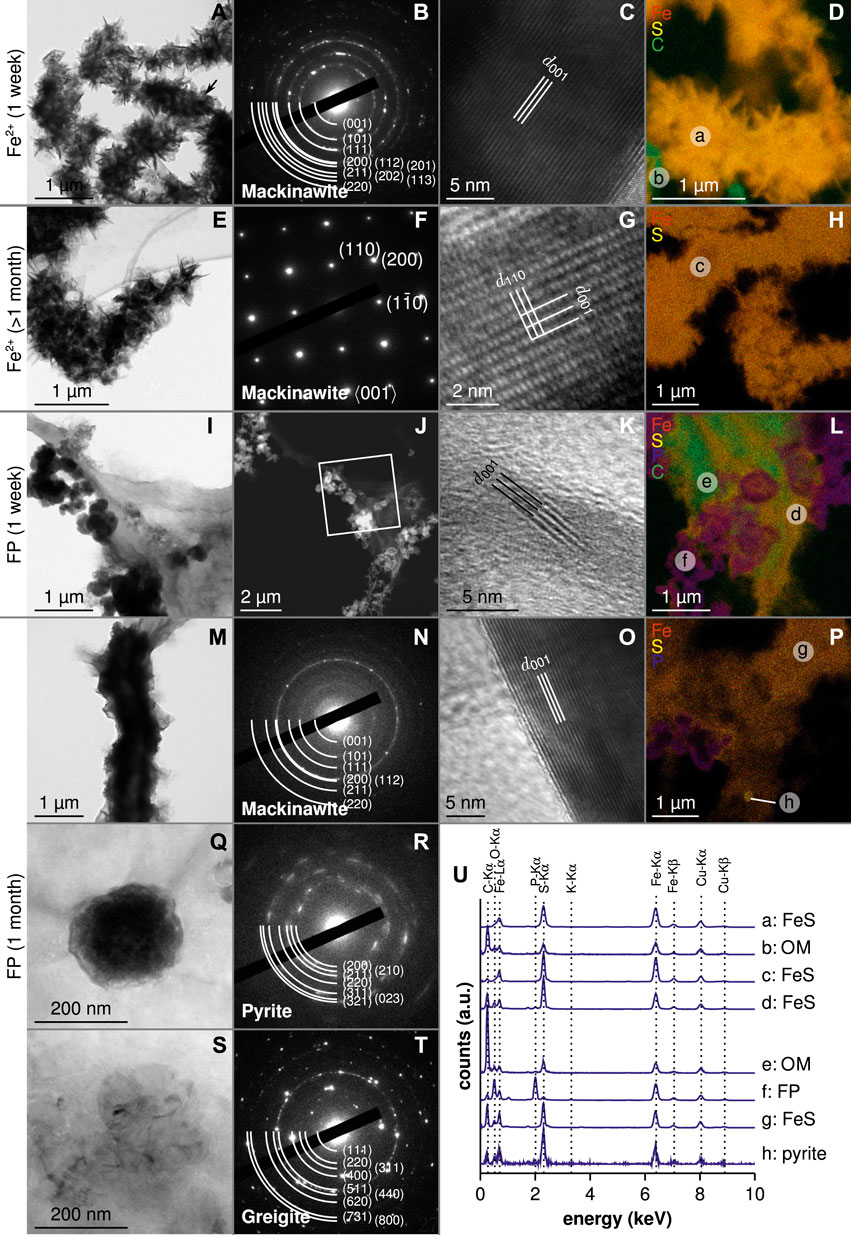
FIGURE 4. Transmission electron microscopy observation of precipitates for both conditions, after 1 week and 1 month. Transmission electron microscopy, selected-area electron diffraction (or scanning transmission electron microscopy for J panel), high-resolution images and energy dispersive X-ray spectrometry map for: (A–D) Fe-diss condition at 1 week, (E–H) Fe-diss condition at 8 months, (I–L) FP-nano condition at 1 week, and (M–T) FP-nano condition at 1 month. (U) Energy dispersive X-ray spectrometry spectra pinned from previous energy dispersive X-ray spectrometry maps. Letters in energy dispersive X-ray spectrometry maps correspond to energy dispersive X-ray spectrometry spectra provided in (U).
FP-Nano Condition
Iron sulfides formed in the FP-nano experiment exhibited a morphology different from that obtained in the Fe-diss condition, specifically forming a thin film spreading over large areas (Figures 3A,B,D,I, 4I,J,L,M,P,U). After 1 week, this iron sulfide film was mostly amorphous, comprising only nano-domains of crystallized mackinawite (Figure 4K). Residual amorphous FP-nano was still present at both 1 week and 1 month (Figures 3A,B,D,I, 4I,J,L,P,U, 5A,G), but additional wide well-crystallized vivianite sheets (Figures 3D,I) were noticeable after 1 month. Due to mineral encrustation, bacteria could not be observed by scanning electron microscopy with secondary electrons (Figure 3A). However, scanning electron microscopy with backscattered electrons (Figure 3B), transmission electron microscopy (Figure 4L), and scanning transmission X-ray microscopy C K-edge analyses (Figure 5B) revealed that cells were intimately associated with the iron sulfide film. Scanning transmission X-ray microscopy analyses showed that this iron sulfide film had a structure close to that of mackinawite (Figures 5C,G). Mackinawite crystallinity increased from mostly amorphous after 1 week to large crystals (Figure 4O) attested by a polycrystalline mackinawite pattern (Figure 4N) after 1 month, similar to that observed in the Fe-diss condition after 1 week. Greigite domains of several hundreds of nanometers were also observed in the mackinawite film (Figures 4S,T). After 1 month, bacteria were still embedded within the mackinawite film (Figures 3E, 4M,P, 5D,E,F,G) and iron sulfide spherules of several hundreds of nanometers in size were observed within the iron sulfide film (Figures 3G,H, 4Q). These spherules had a higher S/Fe ratio than in mackinawite (Figure 3I, spot h). Transmission electron microscopy selected-area electron diffraction patterns indicated that they comprised pyrite particles nucleating within the FeS film (Figure 4R). The S/(Fe + S) ratio of these spherules was estimated to 66 % which is consistent with that of
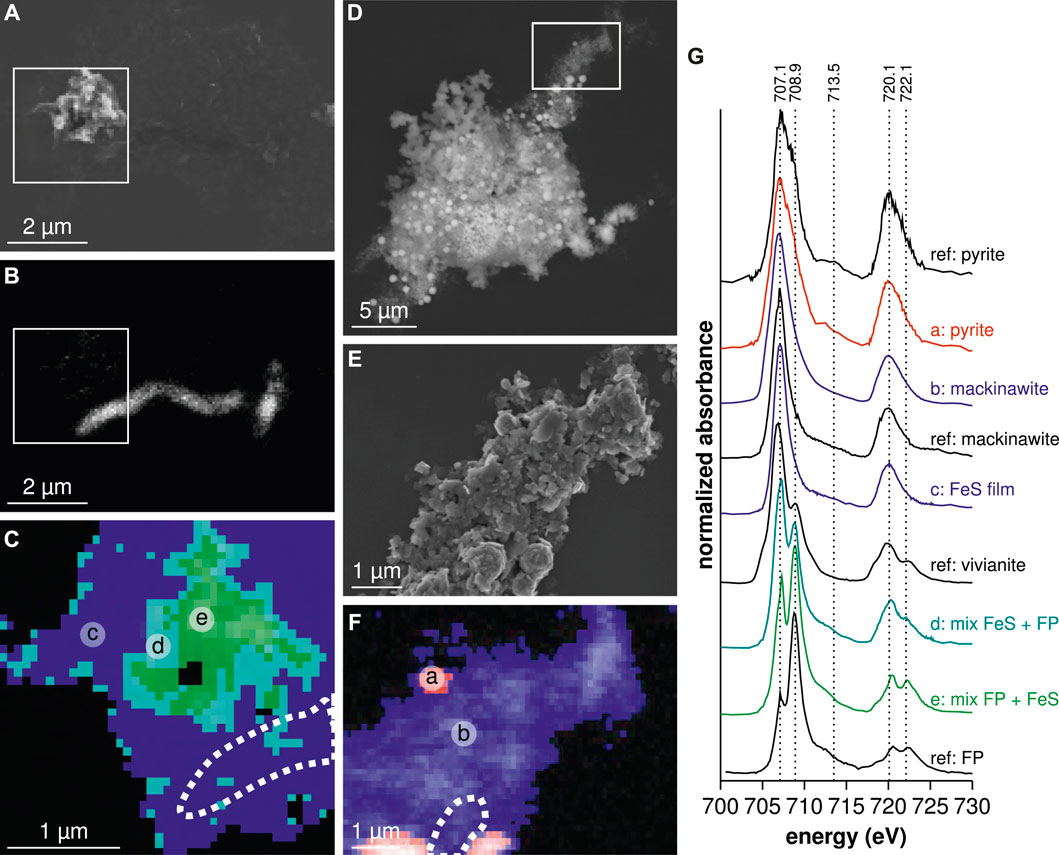
FIGURE 5. SEM and STXM observations in FP-nano condition after 1 week and 6 months. (A) Secondary electron image at 1 week of bacteria embedded in the iron sulfide film close to an FeIII-phosphate particle. (B) STXM C K-edge map of proteins obtained by subtracting the image at 270 eV from the image at 287 eV revealing bacteria from (A). (C) STXM Fe
Discussion
First Stages of Iron Sulfide Formation (1 Week)
Although it is the most stable sulfide phase in sediments, pyrite is unlikely formed by the simple precipitation of
This reaction is kinetically fast (Rickard, 1995), explaining the presence of amorphous iron sulfide (or disordered mackinawite) as the first product of abiotic pyrite synthesis in both ferrous and ferric-sulfide systems (Schoonen and Barnes, 1991; Wei and Osseo-Asare, 1997). Similarly, laboratory sulfate-reducing bacteria pure cultures have mainly yielded the formation of amorphous FeS (Fortin et al., 1994; Williams et al., 2005; Ntarlagiannis et al., 2005; Peltier et al., 2011; Stanley and Southam, 2018) or of well-crystallized mackinawite (Rickard, 1969b; Ivarson and Hallberg, 1976; Zhou et al., 2014; Ikogou et al., 2017; Picard et al., 2018). Some of these studies also detected greigite in long-term experiments (Rickard, 1969b; Picard et al., 2018) with excess of electron donor (Zhou et al., 2014).
In the two types of cultures performed in the present study, bacteria converted lactate and sulfate into acetate and sulfide, respectively, through sulfate respiration. Chemical monitoring (Figures 1E,F) showed a lactate/sulfate consumption ratio of
Hydrogen sulfide quickly reacted with the iron source to form and precipitate black iron sulfide preventing dissolved sulfide accumulation. After 1 week, iron sulfides were characterized as poorly crystallized mackinawite in both conditions (Figure 2). However, significant differences prevailed between Fe-diss and FP-nano experiments.
In Fe-diss condition, the flake-like morphology of iron sulfide aggregates (Figures 3C, 4A) was consistent with that in previous observations of iron sulfides formed in sulfate-reducing bacteria enrichments (Herbert et al., 1998; Watson et al., 2000; Sitte et al., 2013; Berg et al., 2019) or in pure cultures of sulfate-reducing bacteria (Picard et al., 2018; Stanley and Southam, 2018) in the presence of dissolved ferrous iron. In the present study, microbial activity has likely enhanced mackinawite crystallinity produced in one week as shown by the selected-area electron diffraction polycrystalline pattern (Figure 4B) and by high-resolution transmission electron microscopy that revealed large well-crystallized particles with typical (001) lattice fringes of mackinawite (Figure 4C). In contrast, abiotic precipitates were usually reported as amorphous FeS (Ohfuji and Rickard, 2006; Csákberényi-Malasics et al., 2012; Picard et al., 2018). Transmission electron microscopy observations revealed that
While the mineralogy results of Fe-diss experiment after 1 week support previous findings reported in sulfate-reducing bacteria laboratory cultures, the biomineral products obtained in the FP-nano experiment after 1 week exhibit specific characteristics which were never described in previous studies. First of all, an iron sulfide thin film containing significant amounts of organic matter (Figure 4L) and hosting bacterial cells (Figures 3B, 5B) could be interpreted as a mineralized biofilm. Iron sulfide precipitation occurred in this thin layer rather than at the surface of iron phosphate nanoparticles, which were the source of this iron. This implies that HS produced by sulfate-reducing bacteria first reduced FeIII-phosphate following the reaction
Coupling microbial sulfate-reduction (Eq. 2) and FeIII-phosphate reduction (Eq. 3) allows one to explain that the 10 mM of FeIII provided initially in the system was fully reduced by the consumption of 10 mM of lactate which is consistent with the results obtained on day 4 (Figure 1B,F). Dissolved
The difference in mackinawite crystallinity in the two different biomineralization conditions could be explained by the local concentration of iron bound at the cell surface. In the Fe-diss condition, high amounts of
Long-Term Iron Sulfide Evolution ( 1 Month)
Aging of disordered mackinawite leads to its conversion into more stable iron sulfide phases such as crystalline mackinawite, greigite, or pyrite depending on environmental conditions. The increase in mackinawite crystallinity with aging has been interpreted as the expulsion of water molecules entrapped between lattice sheets during rapid mackinawite precipitation (Wolthers et al., 2003). In abiotic experiments, ordering of mackinawite is quite slow and could take several months to a year (Rickard, 1969a). In the present work, the high degree of mackinawite crystallinity in the Fe-diss condition is evidenced after only 1 month by X-ray diffraction (Figure 2), single-crystalline selected-area electron diffraction patterns obtained by transmission electron microscopy (Figure 4F), and high-resolution images (Figure 4G). Mackinawite increase in crystallinity was not as pronounced in the FP-nano experiment, but it showed relatively well-crystallized polycrystalline selected-area electron diffraction patterns after 1 month (Figure 4N), and the small nanometric domains present after 1 week (Figure 4K) evolved into domains of hundreds of nanometers long (Figure 4O). Interestingly, the morphology of mackinawite after 1 month in the FP-nano condition was similar to the one observed in the Fe-diss condition after 1 week.
In the absence of oxidants, the lack of other stable iron sulfides than well-crystallized mackinawite in the Fe-diss experiment could be explained by the low level of sulfide produced, around 2 mM, compared to the high dissolved
The rate of Eq. 5 has been well constrained (Rickard, 1997), and in Fe-diss condition, the formation of 1 mM pyrite after 1 month would require more than 1 mM of aqueous
In the FP-nano experiment, the pyrite formation pathway assumed in the Fe-diss condition (Eq. 5) was shifted to a more efficient pathway involving zero-valent sulfur formed through the reduction of ferric phosphate (Eq. 3). Indeed, pyrite formation might be strongly accelerated if other oxidants than protons are available for oxidation of FeS into
The occurrence of greigite within the iron sulfide film (Figures 4S,T) is consistent with the possible solid-state conversion of mackinawite into greigite (Lennie et al., 1997; Pósfai, 1998). Greigite may originate from the reaction of FeS with zero-valent sulfur:
Greigite possibly represents an intermediate phase in the global mechanism of pyrite formation, but it did not accumulate to sufficient levels to be detectable by X-ray diffraction analyses in the FP-nano condition. Pyrite formation through a greigite pathway has been suggested based on the occurrence of sedimentary magnetic pyrites and has been well studied (Wilkin and Barnes, 1996; Benning et al., 2000; Hunger and Benning, 2007; Lan and Butler, 2014). Although structurally complex (Rickard and Luther, 2007), the conversion of greigite to pyrite through a solid-state reaction may still be possible, as shown by hydrothermal experiments (Hunger and Benning, 2007) and, more recently, by the co-occurrence of greigite and nanocrystalline pyrite domains in micrometer-large pyrite spheres formed in an sulfate-reducing bacteria enrichment culture (Berg et al., 2020). Pyrite could thus have been produced by the reaction of greigite with zero-valent sulfur as follows:
Since FeS and S0 (in the form of cyclo-octasulfur S8) are both solid phases, a direct reaction between them in solution is improbable. Instead, S0 may occur as polysulfides which have been shown to result from the reaction of HS− with ferric phases, especially FeIII-(hydr)oxides such as goethite or lepidocrocite (Hellige et al., 2012; Wan et al., 2014). In addition, polysulfides can be produced by the reaction of
Then, polysulfides may react with FeS to form pyrite as follows (Rickard, 1975):
Interestingly, experiments with millimolar concentrations of dissolved
Environmental Implications
Pyrite and vivianite are barely found together in natural environments and instead are usually reported to form separately (Manning et al., 1999). While pyrite is very abundant in anoxic sediments, in particular, in marine ones, due to the high level of sulfate in modern ocean, vivianite mainly forms in anoxic non-euxinic environments, such as some ferruginous meromictic lakes (Fagel et al., 2005; Rothe et al., 2014; Cosmidis et al., 2014). Indeed, highly reactive sulfide competes with phosphate for reaction with
Despite a low sulfate concentration (<20 µM), Lake Pavin hosts a plethora of sulfate-reducing bacteria in its water column (Lehours et al., 2005; Berg et al., 2019; Berg et al., 2020) and pyrite was found abundantly in the first 12 cm of its sediments, in association with abundant vivianite (Viollier et al., 1997; Busigny et al., 2014; Busigny et al., 2016). Although pyrite was only detected in the sediments and not in the water column, sulfate-rich enrichment cultures from this lake led to rapid pyrite formation after 21 days of culture (Berg et al., 2020). Here, our results demonstrate that the sole activity of Desulfovibrio (representing 80 % of the microbial consortium in the enrichment (Berg et al., 2020)) was able to promote pyrite formation. However, while, in the present study, pyrites occurred as infra micrometric spherules undetectable by X-ray diffraction after one month, pyrites produced within the Lake Pavin consortium were visible on diffractograms after only 21 days and occurred as beads of 1 µm in diameter (Berg et al., 2020). The additional presence of sulfide-oxidizing bacteria (e.g., Sulfuricurvum and Arcobacter) might have strongly enhanced the rate of pyrite formation in the enrichment through an increased delivery of polysulfides (Berg et al., 2020). Hence, kinetics of pyrite formation induced by sulfate-reducing bacteria activity, such as described in our present study, would be accelerated under environmental conditions due to the concomitant activity of sulfide-oxidizing bacteria. In the future, it would be interesting to measure and compare the levels of polysulfides produced in pure sulfate-reducing bacteria vs mixed enrichment cultures in order to evaluate how S0 impacts the formation of pyrite in both systems.
Sedimentary pyrites are commonly found as framboids of several micrometers in size (Wilkin et al., 1996; Busigny et al., 2016). Framboids are sparsely reported in anoxic water column and mainly in euxinic basins (Skei, 1988; Muramoto et al., 1991; Perry and Pedersen, 1993). Such framboids strongly deviate from the biogenic submicrometric pyrite spherules formed in the present FP-nano condition as well as from pyrites obtained in sulfate-reducing bacteria enrichments in previous studies (Donald and Southam, 1999; Thiel et al., 2019; Berg et al., 2020). This dichotomy suggests that framboids in natural environments originate from diagenetic processes rather than from purely biogenic pathways. We suggest that submicrometric pyrite spherules such as those obtained in our pure sulfate-reducing bacteria cultures (FP-nano condition) might be precursors for larger pyrite framboids. Framboidal evolution from biogenic precursors might explain the intra-grain δS34 variability in pyrite framboids (Bryant et al., 2020). In anoxic stratified waters, submicrometric pyrite spherules could form in the water column, below the oxycline, promoted by sulfate-reducing bacteria and other sulfur- and iron-cycling bacteria activities. Due to their small size and their dilution within the water column and the sediments, they may have been overlooked so far in euxinic and non-sulfidic water bodies. Interestingly, X-ray absorption spectroscopy analyses of Lake Pavin samples revealed that including pyrite significantly improved the fits of X-ray absorption spectroscopy spectra of samples collected just below the sulfate-reduction zone in this lake, while pyrites were not detected by X-ray diffraction (Cosmidis et al., 2014). Then, it is possible that pyrite precursors produced by sulfate-reducing bacteria in the water column would evolve into framboidal pyrites deeper in the sediments following diagenetic processes. This is consistent with the occurrence of pyrite microcrystals at the top of modern sediments which give way to pyrite framboids deeper in the sediment (Raven et al., 2016).
To conclude, our results attest that vivianite and pyrite formation can result from the single activity of sulfate-reducing bacteria in the presence of ferric phosphate nanoparticles. Early stages lead to the formation of submicrometric spherules nucleated within an FeS-rich film. Under environmental conditions, this reaction may be accelerated by the contribution of polysulfide-producing microorganisms. Evolution into micrometric framboidal pyrites may require longer timescales, hence occurring preferentially in the sediments upon diagenesis. Further investigations are needed to explore the effects of diagenesis on these pyrite spherules and understand the origin of pyrite framboids.
Data Availability Statement
The raw data supporting the conclusions of this article will be made available by the authors, without undue reservation.
Author Contributions
AD performed the laboratory experiments, AD, JSB, and JM achieved the chemical and mineralogical analyses. AD and FG realized the thermodynamic modeling. All the authors wrote and reviewed the manuscript.
Conflict of Interest
The authors declare that the research was conducted in the absence of any commercial or financial relationships that could be construed as a potential conflict of interest.
Acknowledgments
This work was funded by the ANR SRB project, under grant ANR-14-CE33-0003-01, of the French Agence Nationale de la Recherche to JM. The authors would like to thank the staff of the IMPMC (Sorbonne Universités) for their support and training on instruments: Benoît Baptiste and Ludovic Delbès for the X-ray diffraction facility, Jean-Michel Guigner for the transmission electron microscopy facility, Imène Estéve, Béatrice Doisneau, and Stéphanie Delbrel for the scanning electron microscopy facility, and Fériel Skouri-Panet and Mélanie Poinsot for the Biology Lab. The scanning electron microscopy facility at the IMPMC is funded by Région Ile de France, under grant SESAME 2006 N° I-07-593/R, INSU/CNRS, UPMC-Paris 6, and by the Agence Nationale de la Recherche (grant N° ANR-07-BLAN-0124-01). The transmission electron microscopy facility at IMPMC is supported by Région Ile de France, under grant SESAME 2000 E 1435. Parts of this work were supported by IPGP multidisciplinary program PARI and by Paris-IdF region SESAME, under Grant no. 12015908. The authors thank Laure Cordier (LGE and IPGP) for ICP-AES and iron-chromatography analyses. The authors also thank Séverine Zirah (MCAM and MNHN) for providing access to high-performance liquid chromatography. The authors acknowledge SOLEIL (HERMES beamline, Saint-Aubin, France) for having provided beamtime for scanning transmission X-ray microscopy experiments. The authors also especially thank Sufal Swaraj, Stefan Stanescu, and Rachid Belkhou (SOLEIL) for their support at the beamlines.
Supplementary Material
The Supplementary Material for this article can be found online at: https://www.frontiersin.org/articles/10.3389/feart.2020.588310/full#supplementary-material.
References
Al-Borno, A., and Tomson, M. B. (1994). The temperature dependence of the solubility product constant of vivianite. Geochem. Cosmochim. Acta. 58 (24), 5373–5378. doi:10.1016/0016-7037(94)90236-4
Beijerinck, M. (1895). Über Spirillum desulfuricans als ursache von sulfatreduktion. Zentralbl. Bakteriol. 1, 1–9.
Belkhou, R., Stanescu, S., Swaraj, S., Besson, A., Ledoux, M., Hajlaoui, M., et al. (2015). HERMES: a soft X-ray beamline dedicated to X-ray microscopy. J. Synchrotron Radiat. 22 (4), 968–979. doi:10.1107/s1600577515007778
Benning, L. G., Wilkin, R. T., and Barnes, H. L. (2000). Reaction pathways in the Fe–S system below 100°C. Chem. Geol. 167 (1-2), 25–51. doi:10.1016/s0009-2541(99)00198-9
Berg, J. S., Duverger, A., Cordier, L., Laberty-Robert, C., Guyot, F., and Miot, J. (2020). Rapid pyritization in the presence of a sulfur/sulfate-reducing bacterial consortium. Sci. Rep. 10 (1), 8264. doi:10.1038/s41598-020-64990-6
Berg, J. S., Jézéquel, D., Duverger, A., Lamy, D., Laberty-Robert, C., and Miot, J. (2019). Microbial diversity involved in iron and cryptic sulfur cycling in the ferruginous, low-sulfate waters of Lake Pavin. PLoS One. 14 (2), e0212787. doi:10.1371/journal.pone.0212787
Berg, J. S., Michellod, D., Pjevac, P., Martinez-Perez, C., Buckner, C. R. T., Hach, P. F., et al. (2016). Intensive cryptic microbial iron cycling in the low iron water column of the meromictic Lake Cadagno. Environ. Microbiol. 18 (12), 5288–5302. doi:10.1111/1462-2920.13587
Berner, R. A. (1970). Sedimentary pyrite formation. Am. J. Sci. 268 (1), 1–23. doi:10.2475/ajs.268.1.1
Berner, R. A. (1962). Tetragonal iron sulfide. Science 137 (3531), 669-669. doi:10.1126/science.137.3531.669-a
Berner, R. A. (1969). The synthesis of framboidal pyrite. Econ. Geol. 64 (4), 383–384. doi:10.2113/gsecongeo.64.4.383
Beveridge, T. J. (1989). Role of cellular design in bacterial metal accumulation and mineralization. Annu. Rev. Microbiol. 43 (1), 147–171. doi:10.1146/annurev.mi.43.100189.001051
Borch, T., Masue, Y., Kukkadapu, R. K., and Fendorf, S. (2007). Phosphate imposed limitations on biological reduction and alteration of ferrihydrite. Environ. Sci. Technol. 41 (1), 166–172. doi:10.1021/es060695p
Bourdelle, F., Benzerara, K., Beyssac, O., Cosmidis, J., Neuville, D. R., Brown, G. E., et al. (2013). Quantification of the ferric/ferrous iron ratio in silicates by scanning transmission X-ray microscopy at the Fe L2,3 edges. Contrib. Mineral. Petrol. 166 (2), 423–434. doi:10.1007/s00410-013-0883-4
Bryant, R. N., Jones, C., Raven, M. R., Owens, J. D., and Fike, D. A. (2020). Shifting modes of iron sulfidization at the onset of OAE-2 drive regional shifts in pyrite δ34S records. Chem. Geol. 553, 119808. doi:10.1016/j.chemgeo.2020.119808
Busigny, V., Jézéquel, D., Cosmidis, J., Viollier, E., Benzerara, K., Planavsky, N. J., et al. (2016). “The iron wheel in lac Pavin: interaction with phosphorus cycle,” in Lake Pavin. Editors T. Sime-Ngando, P. Boivin, E. Chapron, D. Jezequel, and M. Meybeck (Cham: Springer International Publishing), 205–220.
Busigny, V., Planavsky, N. J., Jézéquel, D., Crowe, S., Louvat, P., Moureau, J., et al. (2014). Iron isotopes in an Archean ocean analogue. Geochem. Cosmochim. Acta. 133, 443–462. doi:10.1016/j.gca.2014.03.004
Butler, I. B., and Rickard, D. (2000). Framboidal pyrite formation via the oxidation of iron (II) monosulfide by hydrogen sulphide. Geochem. Cosmochim. Acta. 64 (15), 2665–2672. doi:10.1016/s0016-7037(00)00387-2
Cline, J. D. (1969). Spectrophotometric determination of hydrogen sulfide in natural waters1. Limnol. Oceanogr. 14 (3), 454–458. doi:10.4319/lo.1969.14.3.0454
Cornell, R. M., and Schwertmann, U. (2003). The iron oxides: structure, properties, reactions, occurrences, and uses. Weinheim: Wiley VCH. 2nd, completely rev. and extended ed edition.
Cosmidis, J., Benzerara, K., Morin, G., Busigny, V., Lebeau, O., Jézéquel, D., et al. (2014). Biomineralization of iron-phosphates in the water column of Lake Pavin (massif central, France). Geochem. Cosmochim. Acta. 126, 78–96. doi:10.1016/j.gca.2013.10.037
Csákberényi-Malasics, D., Rodriguez-Blanco, J. D., Kis, V. K., Rečnik, A., Benning, L. G., and Pósfai, M. (2012). Structural properties and transformations of precipitated FeS. Chem. Geol. 294-295, 249–258. doi:10.1016/j.chemgeo.2011.12.009
Dijkstra, N., Kraal, P., Kuypers, M. M. M., Schnetger, B., and Slomp, C. P. (2014). Are iron-phosphate minerals a sink for phosphorus in anoxic Black Sea sediments? PLoS One. 9 (7), e101139. doi:10.1371/journal.pone.0101139
Donald, R., and Southam, G. (1999). Low temperature anaerobic bacterial diagenesis of ferrous monosulfide to pyrite. Geochem. Cosmochim. Acta. 63 (13-14), 2019–2023. doi:10.1016/s0016-7037(99)00140-4
Fagel, N., Alleman, L., Granina, L., Hatert, F., Thamo-Bozso, E., Cloots, R., et al. (2005). Vivianite formation and distribution in Lake Baikal sediments. Global Planet. Change. 46 (1-4), 315–336. doi:10.1016/j.gloplacha.2004.09.022
Farrand, M. (1970). Framboidal sulphides precipitated synthetically. Miner. Deposita. 5 (3), 237–247. doi:10.1007/bf00201990
Ferris, F., Fyfe, W., and Beveridge, T. (1987). Bacteria as nucleation sites for authigenic minerals in a metal-contaminated lake sediment. Chem. Geol. 63 (3-4), 225–232. doi:10.1016/0009-2541(87)90165-3
Folk, R. L. (2005). Nannobacteria and the formation of framboidal pyrite: textural evidence. J. Earth Syst. Sci. 114 (3), 369–374. doi:10.1007/bf02702955
Fortin, D., Southam, G., and Beveridge, T. J. (1994). Nickel sulfide, iron-nickel sulfide and iron sulfide precipitation by a newly isolated Desulfotomaculum species and its relation to nickel resistance. FEMS (Fed. Eur. Microbiol. Soc.) Microbiol. Ecol. 14 (2), 121–132. doi:10.1111/j.1574-6941.1994.tb00099.x
Gramp, J. P., Wang, H., Bigham, J. M., Jones, F. S., and Tuovinen, O. H. (2009). Biogenic synthesis and reduction of Fe(III)-hydroxysulfates. Geomicrobiol. J. 26 (4), 275–280. doi:10.1080/01490450902892597
Hellige, K., Pollok, K., Larese-Casanova, P., Behrends, T., and Peiffer, S. (2012). Pathways of ferrous iron mineral formation upon sulfidation of lepidocrocite surfaces. Geochem. Cosmochim. Acta. 81, 69–81. doi:10.1016/j.gca.2011.12.014
Herbert, R. B., Benner, S. G., Pratt, A. R., and Blowes, D. W. (1998). Surface chemistry and morphology of poorly crystalline iron sulfides precipitated in media containing sulfate-reducing bacteria. Chem. Geol. 144 (1-2), 87–97. doi:10.1016/s0009-2541(97)00122-8
Hunger, S., and Benning, L. G. (2007). Greigite: a true intermediate on the polysulfide pathway to pyrite. Geochem. Trans. 8, 1. doi:10.1186/1467-4866-8-1
Ikogou, M., Ona-Nguema, G., Juillot, F., Le Pape, P., Menguy, N., Richeux, N., et al. (2017). Long-term sequestration of nickel in mackinawite formed by Desulfovibrio capillatus upon Fe(III)-citrate reduction in the presence of thiosulfate. Appl. Geochem. 80, 143–154. doi:10.1016/j.apgeochem.2017.02.019
Ivarson, K. C., and Hallberg, R. O. (1976). Formation of mackinawite by the microbial reduction of jarosite and its application to tidal sediments. Geoderma 16 (1), 1–7. doi:10.1016/0016-7061(76)90089-6
Jilbert, T., and Slomp, C. P. (2013). Iron and manganese shuttles control the formation of authigenic phosphorus minerals in the euxinic basins of the Baltic Sea. Geochem. Cosmochim. Acta. 107, 155–169. doi:10.1016/j.gca.2013.01.005
Kamyshny, A., Goifman, A., Gun, J., Rizkov, D., and Lev, O. (2004). Equilibrium distribution of polysulfide ions in aqueous solutions at 25 °C: a new approach for the study of polysulfides’ equilibria. Environ. Sci. Technol. 38 (24), 6633–6644. doi:10.1021/es049514e
Lan, Y., and Butler, E. C. (2014). Monitoring the transformation of mackinawite to greigite and pyrite on polymer supports. Appl. Geochem. 50, 1–6. doi:10.1016/j.apgeochem.2014.07.020
Lehours, A.-C., Bardot, C., Thenot, A., Debroas, D., and Fonty, G. (2005). Anaerobic microbial communities in Lake Pavin, a unique Meromictic Lake in France. Aemilianense 71 (11), 7389–7400. doi:10.1128/aem.71.11.7389-7400.2005
Lehours, A.-C., Batisson, I., Guedon, A., Mailhot, G., and Fonty, G. (2009). Diversity of culturable bacteria, from the anaerobic zone of the Meromictic Lake Pavin, able to perform dissimilatory-iron reduction in different in Vitro conditions. Geomicrobiol. J. 26 (3), 212–223. doi:10.1080/01490450902744012
Lennie, A. R., Redfern, S. A. T., Champness, P. E., Stoddart, C. P., Schofield, P. F., and Vaughan, D. J. (1997). Transformation of mackinawite to greigite; an in situ X-ray powder diffraction and transmission electron microscope study. Am. Mineral. 82 (3-4), 302–309. doi:10.2138/am-1997-3-408
Li, G., Zhang, B., Yu, F., Novakova, A. A., Krivenkov, M. S., Kiseleva, T. Y., et al. (2014). High-purity Fe3S4 greigite microcrystals for magnetic and electrochemical performance. Chem. Mater. 26 (20), 5821–5829. doi:10.1021/cm501493m
Llirós, M., García–Armisen, T., Darchambeau, F., Morana, C., Triadó–Margarit, X., Inceoğlu, O., et al. (2015). Pelagic photoferrotrophy and iron cycling in a modern ferruginous basin. Sci. Rep. 5 (1), 13803. doi:10.1038/srep13803
Love, L. G. (1957). Micro-organisms and the presence of syngenetic pyrite. Quart. J. Geolog. Soc. 113 (1-4), 429–440. doi:10.1144/gsl.jgs.1957.113.01-04.18
Maclean, L. C. W., Tyliszczak, T., Gilbert, P. U. P. A., Zhou, D., Pray, T. J., Onstott, T. C., et al. (2008). A high-resolution chemical and structural study of framboidal pyrite formed within a low-temperature bacterial biofilm. Geobiology 6 (5), 471–480. doi:10.1111/j.1472-4669.2008.00174.x
Manning, P. G., Prepas, E. E., and Serediak, M. S. (1999). Pyrite and vivianite intervals in the bottom sediments of eutrophic Baptiste Lake, Alberta, Canada. Can. Mineral. 37 (3), 593–601.
Miot, J., Benzerara, K., Morin, G., Bernard, S., Beyssac, O., Larquet, E., et al. (2009a). Transformation of vivianite by anaerobic nitrate-reducing iron-oxidizing bacteria. Geobiology 7 (3), 373–384. doi:10.1111/j.1472-4669.2009.00203.x
Miot, J., Benzerara, K., Morin, G., Kappler, A., Bernard, S., Obst, M., et al. (2009b). Iron biomineralization by anaerobic neutrophilic iron-oxidizing bacteria. Geochem. Cosmochim. Acta. 73 (3), 696–711. doi:10.1016/j.gca.2008.10.033
Miot, J., Benzerara, K., Obst, M., Kappler, A., Hegler, F., Schädler, S., et al. (2009c). Extracellular iron biomineralization by photoautotrophic iron-oxidizing bacteria. Appl. Environ. Microbiol. 75 (17), 5586–5591. doi:10.1128/aem.00490-09
Miot, J., and Etique, M. (2016). “Formation and transformation of iron-bearing minerals by iron(II)-oxidizing and iron(III)-reducing bacteria,” in Iron oxides. Editor D. Faivre, (Weinheim, Germany: Wiley-VCH Verlag GmbH & Co. KGaA), 53–98.
Miot, J., Jézéquel, D., Benzerara, K., Cordier, L., Rivas-Lamelo, S., Skouri-Panet, F., et al. (2016). Mineralogical diversity in Lake Pavin: connections with water column chemistry and biomineralization processes. Minerals, 6 (2), 24. doi:10.3390/min6020024
Miot, J., Remusat, L., Duprat, E., Gonzalez, A., Pont, S., and Poinsot, M. (2015). Fe biomineralization mirrors individual metabolic activity in a nitrate-dependent Fe(II)-oxidizer. Front. Microbiol. 6, 879. doi:10.3389/fmicb.2015.00879
Mirvaux, B., Recham, N., Miot, J., Courty, M., Bernard, S., Beyssac, O., et al. (2016). Iron phosphate/bacteria composites as precursors for textured electrode materials with enhanced electrochemical properties. J. Electrochem. Soc. 163 (10), A2139–A2148. doi:10.1149/2.0101610jes
Muramoto, J. A., Honjo, S., Fry, B., Hay, B. J., Howarth, R. W., and Cisne, J. L. (1991). Sulfur, iron and organic carbon fluxes in the Black Sea: sulfur isotopic evidence for origin of sulfur fluxes. Deep Sea Res. A. 38, S1151–S1187. doi:10.1016/s0198-0149(10)80029-9
Muyzer, G., and Stams, A. J. M. (2008). The ecology and biotechnology of sulphate-reducing bacteria. Nat. Rev. Microbiol. 6, 441–454. doi:10.1038/nrmicro1892
Neal, A. L., Techkarnjanaruk, S., Dohnalkova, A., McCready, D., Peyton, B. M., and Geesey, G. G. (2001). Iron sulfides and sulfur species produced at hematite surfaces in the presence of sulfate-reducing bacteria. Geochem. Cosmochim. Acta. 65 (2), 223–235. doi:10.1016/s0016-7037(00)00537-8
Nriagu, J. O. (1972). Stability of vivianite and ion-pair formation in the system fe3(PO4)2-H3PO4H3PO4-H2o. Geochem. Cosmochim. Acta. 36 (4), 459–470. doi:10.1016/0016-7037(72)90035-x
Ntarlagiannis, D., Williams, K. H., Slater, L., and Hubbard, S. (2005). Low-frequency electrical response to microbial induced sulfide precipitation: electrical response to biominerals. J. Geophys. Res. 110 (G2). doi:10.1029/2005jg000024
Ohfuji, H., and Rickard, D. (2005). Experimental syntheses of framboids–a review. Earth Sci. Rev. 71 (3-4), 147–170. doi:10.1016/j.earscirev.2005.02.001
Ohfuji, H., and Rickard, D. (2006). High resolution transmission electron microscopic study of synthetic nanocrystalline mackinawite. Earth Planet Sci. Lett. 241 (1-2), 227–233. doi:10.1016/j.epsl.2005.10.006
Peltier, E., Ilipilla, P., and Fowle, D. (2011). Structure and reactivity of zinc sulfide precipitates formed in the presence of sulfate-reducing bacteria. Appl. Geochem. 26 (9-10), 1673–1680. doi:10.1016/j.apgeochem.2011.04.024
Perry, K. A., and Pedersen, T. F. (1993). Sulphur speciation and pyrite formation in meromictic ex-fjords. Geochem. Cosmochim. Acta. 57 (18), 4405–4418. doi:10.1016/0016-7037(93)90491-e
Picard, A., Gartman, A., Clarke, D. R., and Girguis, P. R. (2018). Sulfate-reducing bacteria influence the nucleation and growth of mackinawite and greigite. Geochem. Cosmochim. Acta. 220, 367–384. doi:10.1016/j.gca.2017.10.006
Pósfai, M. (1998). Reaction sequence of iron sulfide minerals in bacteria and their use as biomarkers. Science 280 (5365), 880–883.
Pye, K. (1981). Marshrock formed by iron sulphide and siderite cementation in saltmarsh sediments. Nature 294 (5842), 650–652. doi:10.1038/294650a0
Raven, M. R., Sessions, A. L., Fischer, W. W., and Adkins, J. F. (2016). Sedimentary pyrite δ34S differs from porewater sulfide in Santa Barbara Basin: proposed role of organic sulfur. Geochem. Cosmochim. Acta. 186, 120–134. doi:10.1016/j.gca.2016.04.037
Rickard, D. (1969a). The chemistry of iron sulphide formation at low temperatures. Stock. Contrib. Geol. 20, 67–95.
Rickard, D. (1969b). The microbiological formation of iron sulphides. Stock. Contrib. Geol., 20:49–66.
Rickard, D. T. (1975). Kinetics and mechanism of pyrite formation at low temperatures. Am. J. Sci. 275 (6), 636–652. doi:10.2475/ajs.275.6.636
Rickard, D. (1995). Kinetics of FeS precipitation: Part 1. Competing reaction mechanisms. Geochem. Cosmochim. Acta. 59 (21), 4367–4379. doi:10.1016/0016-7037(95)00251-t
Rickard, D. (1997). Kinetics of pyrite formation by the H2S oxidation of iron (II) monosulfide in aqueous solutions between 25 and 125°C: the rate equation. Geochem. Cosmochim. Acta. 61 (1), 115–134. doi:10.1016/s0016-7037(96)00321-3
Rickard, D., and Luther, G. W. (1997). Kinetics of pyrite formation by the H2S oxidation of iron (II) monosulfide in aqueous solutions between 25 and 125°C: the mechanism. Geochem. Cosmochim. Acta. 61 (1), 135–147. doi:10.1016/s0016-7037(96)00322-5
Rickard, D., and Luther, G. W. (2007). Chemistry of iron sulfides. Chem. Rev. 107 (2), 514–562. doi:10.1021/cr0503658
Rickard, D., Mussmann, M., and Steadman, J. A. (2017). Sedimentary sulfides. Elements 13 (2), 117–122. doi:10.2113/gselements.13.2.117
Rothe, M., Frederichs, T., Eder, M., Kleeberg, A., and Hupfer, M. (2014). Evidence for vivianite formation and its contribution to long-term phosphorus retention in a recent lake sediment: a novel analytical approach. Biogeosciences 11 (18), 5169–5180. doi:10.5194/bg-11-5169-2014
Rothe, M., Kleeberg, A., Grüneberg, B., Friese, K., Pérez-Mayo, M., and Hupfer, M. (2015). Sedimentary sulphur:iron ratio indicates vivianite occurrence: a study from two contrasting freshwater systems. PLoS One. 10 (11), e0143737. doi:10.1371/journal.pone.0143737
Rothe, M., Kleeberg, A., and Hupfer, M. (2016). The occurrence, identification and environmental relevance of vivianite in waterlogged soils and aquatic sediments. Earth Sci. Rev. 158, 51–64. doi:10.1016/j.earscirev.2016.04.008
Rust, G. W. (1935). Colloidal primary copper ores at cornwall mines, southeastern Missouri. J. Geol. 43 (4), 398–426. doi:10.1086/624318
Schoepfer, V. A., Burton, E. D., and Johnston, S. G. (2019). Contrasting effects of phosphate on the rapid transformation of schwertmannite to Fe(III) (oxy)hydroxides at near-neutral pH. Geoderma 340, 115–123. doi:10.1016/j.geoderma.2018.12.051
Schoonen, M. A. A., and Barnes, H. L. (1991). Reactions forming pyrite and marcasite from solution: I. Nucleation of FeS2 below 100°C. Geochem. Cosmochim. Acta. 55 (6), 1495–1504. doi:10.1016/0016-7037(91)90122-l
Schoonen, M. A. (2004). “Mechanisms of sedimentary pyrite formation,” in Sulfur biogeochemistry - past and present Editors J. P. Amend, K. J. Edwards, and T. W. Lyons. Geological Society of America, 117–134. doi:10.1130/0-8137-2379-5.117
Shen, Y., and Buick, R. (2004). The antiquity of microbial sulfate reduction. Earth Sci. Rev. 64 (3-4), 243–272. doi:10.1016/s0012-8252(03)00054-0
Sitte, J., Pollok, K., Langenhorst, F., and Küsel, K. (2013). Nanocrystalline nickel and cobalt sulfides formed by a heavy metal-tolerant, sulfate-reducing enrichment culture. Geomicrobiol. J. 30 (1), 36–47. doi:10.1080/01490451.2011.653082
Skei, J. (1988). Formation of framboidal iron sulfide in the water of a permanently anoxic fjord-framvaren, South Norway. Mar. Chem. 23 (3-4), 345–352. doi:10.1016/0304-4203(88)90103-x
Stanley, W., and Southam, G. (2018). The effect of gram-positive (Desulfosporosinus orientis) and gram-negative (Desulfovibrio desulfuricans) sulfate-reducing bacteria on iron sulfide mineral precipitation. Can. J. Microbiol. 64 (9), 629–637. doi:10.1139/cjm-2017-0545
Stookey, L. L. (1970). Ferrozine—a new spectrophotometric reagent for iron. Anal. Chem. 42 (7), 779–781. doi:10.1021/ac60289a016
Swaraj, S., Belkhou, R., Stanescu, S., Rioult, M., Besson, A., and Hitchcock, A. P. (2017). Performance of the HERMES beamline at the carbon K-edge. J. Phys. Conf. 849, 012046. doi:10.1088/1742-6596/849/1/012046
Templeton, A. S. (2011). Geomicrobiology of iron in extreme environments. Elements 7 (2), 95–100. doi:10.2113/gselements.7.2.95
Thiel, J., Byrne, J. M., Kappler, A., Schink, B., and Pester, M. (2019). Pyrite formation from FeS and H2S is mediated through microbial redox activity. Proc. Natl. Acad. Sci. U.S.A. 116 (14), 6897–6902. doi:10.1073/pnas.1814412116
Thode, H. G., Macnamara, J., and Fleming, W. H. (1953). Sulphur isotope fractionation in nature and geological and biological time scales. Geochem. Cosmochim. Acta. 3 (5), 235–243. doi:10.1016/0016-7037(53)90042-8
Tonolla, M., Peduzzi, S., Demarta, A., Peduzzi, R., and Hahn, D. (2004). Phototropic sulfur and sulfate-reducing bacteria in the chemocline of meromictic Lake Cadagno, Switzerland. J. Limnol. 63 (2), 161–170. doi:10.4081/jlimnol.2004.161
Tschech, A., and Pfennig, N. (1984). Growth yield increase linked to caffeate reduction in Acetobacterium woodii. Arch. Microbiol. 137 (2), 163–167. doi:10.1007/bf00414460
Vetriani, C., Tran, H. V., and Kerkhof, L. J. (2003). Fingerprinting microbial assemblages from the oxic/anoxic chemocline of the Black Sea. Appl. Environ. Microbiol. 69 (11), 6481–6488. doi:10.1128/aem.69.11.6481-6488.2003
Viollier, E., Michard, G., Jézéquel, D., Pèpe, M., and Sarazin, G. (1997). Geochemical study of a crater lake: lake Pavin, Puy de Dôme, France. Constraints afforded by the particulate matter distribution in the element cycling within the lake. Chem. Geol., 142(3-4):225–241. doi:10.1016/s0009-2541(97)00093-4
Viollier, E., Inglett, P. W., Hunter, K., Roychoudhury, A. N., and Van Cappellen, P. (2000). The ferrozine method revisited: Fe(II)/Fe(III) determination in natural waters. Appl. Geochem. 15 (6), 785–790. doi:10.1016/s0883-2927(99)00097-9
Voegelin, A., Senn, A.-C., Kaegi, R., Hug, S. J., and Mangold, S. (2013). Dynamic Fe-precipitate formation induced by Fe(II) oxidation in aerated phosphate-containing water. Geochem. Cosmochim. Acta. 117, 216–231. doi:10.1016/j.gca.2013.04.022
Wacey, D., Kilburn, M. R., Saunders, M., Cliff, J. B., Kong, C., Liu, A. G., et al. (2015). Uncovering framboidal pyrite biogenicity using nano-scale CNorg mapping. Geology 43 (1), 27–30. doi:10.1130/g36048.1
Wan, M., Shchukarev, A., Lohmayer, R., Planer-Friedrich, B., and Peiffer, S. (2014). Occurrence of surface polysulfides during the interaction between ferric (Hydr)Oxides and aqueous sulfide. Environ. Sci. Technol. 48 (9), 5076–5084. doi:10.1021/es405612f
Wang, J., Morin, C., Li, L., Hitchcock, A., Scholl, A., and Doran, A. (2009). Radiation damage in soft X-ray microscopy. J. Electron. Spectrosc. Relat. Phenom. 170 (1-3), 25–36. doi:10.1016/j.elspec.2008.01.002
Watson, J., Cressey, B., Roberts, A., Ellwood, D., Charnock, J., and Soper, A. (2000). Structural and magnetic studies on heavy-metal-adsorbing iron sulphide nanoparticles produced by sulphate-reducing bacteria. J. Magn. Magn Mater. 214 (1-2), 13–30. doi:10.1016/s0304-8853(00)00025-1
Wei, D., and Osseo-Asare, K. (1995). formation of iron monosulfide: a spectrophotometric study of the reaction between ferrous and sulfide ions in aqueous solutions. J. Colloid Interface Sci., 174(2):273–282. doi:10.1006/jcis.1995.1392
Wei, D., and Osseo-Asare, K. (1997). Aqueous synthesis of finely divided pyrite particles. Colloid. Surface. Physicochem. Eng. Aspect. 121 (1), 27–36. doi:10.1016/s0927-7757(95)03502-8
Widdel, F., Kohring, G.-W., and Mayer, F. (1983). Tudies on dissimilatory sulfate-reducing bacteria that decompose fatty acids: III. Characterization of the filamentous gliding Desulfonema limicola gen. nov. sp. nov., and Desulfonema magnum sp. nov. Arch. Microbiol. 134 (4), 286–294. doi:10.1007/bf00407804
Wilkin, R. T., Barnes, H. L., and Brantley, S. L. (1996). The size distribution of framboidal pyrite in modern sediments: an indicator of redox conditions. Geochem. Cosmochim. Acta. 60 (20), 3897–3912. doi:10.1016/0016-7037(96)00209-8
Wilkin, R. T., and Barnes, H. L. (1996). Pyrite formation by reactions of iron monosulfides with dissolved inorganic and organic sulfur species. Geochem. Cosmochim. Acta. 60 (21), 4167–4179. doi:10.1016/s0016-7037(97)81466-4
Williams, K. H., Ntarlagiannis, D., Slater, L. D., Dohnalkova, A., Hubbard, S. S., and Banfield, J. F. (2005). Geophysical imaging of stimulated microbial biomineralization. Environ. Sci. Technol. 39 (19), 7592–7600. doi:10.1021/es0504035
Wolin, E. A., Wolin, M. J., and Wolfe, R. S. (1963). Formation of methane by bacterial extracts. J. Biol. Chem. 238 (8), 2882–2886.
Wolthers, M., Van der Gaast, S. J., and Rickard, D. (2003). The structure of disordered mackinawite. Am. Mineral. 88 (11-12), 2007–2015. doi:10.2138/am-2003-11-1245
Xiong, Y., Guilbaud, R., Peacock, C. L., Cox, R. P., Canfield, D. E., Krom, M. D., et al. (2019). Phosphorus cycling in Lake Cadagno, Switzerland: a low sulfate euxinic ocean analogue. Geochem. Cosmochim. Acta. 251, 116–135. doi:10.1016/j.gca.2019.02.011
Zeng, Y., Wang, H., Guo, C., Wan, J., Fan, C., Reinfelder, J. R., et al. (2018). Schwertmannite transformation via direct or indirect electron transfer by a sulfate reducing enrichment culture. Environ. Pollut. 242, 738–748. doi:10.1016/j.envpol.2018.07.024
Keywords: sulfate-reducing bacteria, biomineralization, iron sulfide (FeS), pyrite (FeS2), vivianite, electron micoscopy, scanning transmission X-ray microscopy
Citation: Duverger A, Berg JS, Busigny V, Guyot F, Bernard S and Miot J (2020) Mechanisms of Pyrite Formation Promoted by Sulfate-Reducing Bacteria in Pure Culture. Front. Earth Sci. 8:588310. doi:10.3389/feart.2020.588310
Received: 28 July 2020; Accepted: 17 September 2020;
Published: 05 November 2020.
Edited by:
Guillaume Paris, Centre de Recherches Pétrographiques et Géochimiques (CRPG), FranceReviewed by:
Alexey Kamyshny, Ben-Gurion University of the Negev, IsraelMorgan Reed Raven, University of California, Santa Barbara, United States
Copyright © 2020 Duverger, Berg, Busigny, Guyot, Bernard and Miot . This is an open-access article distributed under the terms of the Creative Commons Attribution License (CC BY). The use, distribution or reproduction in other forums is permitted, provided the original author(s) and the copyright owner(s) are credited and that the original publication in this journal is cited, in accordance with accepted academic practice. No use, distribution or reproduction is permitted which does not comply with these terms.
*Correspondence:Arnaud Duverger, YXJuYXVkLmR1dmVyZ2VyQG5vcm1hbGVzdXAub3Jn