- 1School of Earth and Environment, University of Canterbury, Christchurch, New Zealand
- 2School of Earth and Atmospheric Sciences, Georgia Institute of Technology, Atlanta, GA, United States
- 3School of Environment, University of Auckland, Auckland, New Zealand
Pyroclastic density currents (PDCs) are a destructive volcanic hazard. Quantifying the types, frequency and magnitudes of PDC events is essential for effective risk management, but since historical records at best extend a few hundred years this usually relies on identifying deposits in the geological record. However, small volume unconsolidated PDC deposits have low preservation potential and can be difficult to distinguish from other volcaniclastic units, especially in proximal locations. Consequently many small or poorly exposed deposits can be overlooked. Here, we introduce a structured field method for assessing volcaniclastic deposits of unknown origin with a particular focus on identifying deposits from concentrated PDCs (pyroclastic flows). The method differs from traditional identification schemes in that it does not uniquely attribute a deposit to a single depositional process, but instead assesses how confidently different volcaniclastic processes could explain the observed deposit features. Therefore, the underlying uncertainties in the assessment are explicitly addressed. The method allows consistent, rapid assessment of candidate pyroclastic flow deposits in the field, and the concept could easily be adapted for assessing other types of volcaniclastic deposit. The introduction of confidence levels in deposit interpretations should be useful for carrying though uncertainties into probabilistic assessments of volcanic hazards.
Introduction
A fundamental principle in volcanology is that a volcano’s past behavior provides insight into the kind of activity that can be expected in the future. Therefore, quantifying the distributions, types, frequencies and magnitudes of previous hazards is an important component of volcanic hazard management (Nakada, 2000; Leonard et al., 2014). Since historical records only date back at most a few hundred years, this normally relies on identifying deposits in the geological record. However, it can be difficult to identify formative processes from small or poorly preserved deposits that lack distinguishing features, and absence of evidence is not always evidence of absence (White and Valentine, 2016). Therefore, properly assessing even small or poorly preserved deposits, and acknowledging any interpretative uncertainty, is essential for thoroughly understanding a volcano’s past activity.
Volcanic cones are constructed by eruption of lavas and explosively erupted material from one or more vents. During and between eruptive episodes, non-volcanic erosion and transport processes rework these materials downslope to form a wide variety of fragmental deposits composed almost entirely of volcanic material. Thus, most volcanic surfaces present a diverse mix of primary and non-primary fragmental deposits (“volcaniclastic deposits;” e.g., McPhie et al., 1993) that can be difficult to distinguish in the field. In the case of historical deposits, identification is usually straightforward since the causative process is either observed directly, or significant amounts of the deposit remain (e.g., Cronin et al., 2013). Hence, modern deposits are well suited to traditional field assessment methods that emphasize broad initial observations of deposit geometry, followed by closer observations of outcrop-scale textures and components (Lube et al., 2007; Charbonnier et al., 2013). In contrast, because fragmental deposits are easily reworked on steep proximal volcanic slopes, many small or ancient deposits are commonly only preserved in a few poorly-exposed locations (e.g., Donoghue et al., 1999; Le Pennec et al., 2016). It is therefore more difficult to confidently assess small and ancient deposits in the absence of the broader-scale context that aids interpretation.
The motivation for this study was the observation of numerous unidentified volcaniclastic deposits at Mount Ruapehu (Cowlyn, 2016), an active andesitic-dacitic stratovolcano at the southern end of New Zealand’s Taupo Volcanic Zone. Many of the deposits contained clasts with abundant juvenile/primary textures, and initial suspicions were that these were deposits from relatively young PDCs. However PDC deposits have significant textural variability (Branney and Kokelaar, 2002; Brown and Andrews, 2015), and often require detailed fieldwork over different outcrops to make confident interpretations. The poorly exposed, small and patchy deposits at Ruapehu were difficult to confidently attribute to PDCs and could just as easily be attributed to other volcaniclastic processes that reworked erupted material. While historical phreatic and phreatomagmatic eruptions at Ruapehu have frequently produced dilute PDCs (Nairn et al., 1979; Kilgour et al., 2010), hazardous longer-runout concentrated PDCs (“pyroclastic flows”) have not been historically described and few examples are known in Ruapehu’s deposit record (Donoghue et al., 1995). Therefore, identifying young pyroclastic flow deposits at Ruapehu is essential to properly characterize the hazard.
Terminology
A wide range of terms have been applied to primary and reworked volcaniclastic deposits and their components (see White and Houghton, 2006), including pyroclastic deposits containing explosively fragmented material (Wentworth and Williams, 1932; Fisher, 1966; Fisher and Schmincke, 1984), autoclastic deposits containing material fragmented at the surface of moving lava (McPhie et al., 1993; White and Houghton, 2006), and epiclastic deposits containing material fragmented by (McPhie et al., 1993) and/or transported and deposited by non-volcanic surface processes (Cas and Wright, 1987). On volcanic edifices, these deposits usually contain either juvenile material derived directly from erupting magma, or lithic material derived by fragmentation of pre-existing material. Combining several earlier classifications, White and Houghton (2006) introduced the term “primary volcaniclastic” to describe deposits where particles are mobilized directly by explosive or effusive volcanism and not stored prior to arriving at the deposition site. However, even this broad classification introduces difficulties when interpreting ancient deposits. For example, following a large eruption much of a volcanic edifice may be covered in juvenile-textured volcanic material that can then be reworked for years after the eruption has ended (e.g., Pinatubo; Gran and Montgomery, 2005). The resulting deposits would not be primary volcaniclastic; however in proximal-medial locations (i.e., within the main volcanic edifice) where short transport distances provide little opportunity for clast modification or sorting, these deposits can be difficult or impossible to distinguish from primary deposits that were emplaced at the time of eruption. Hence almost all of the current classification schemes require at least limited interpretation of the source and mode of particle fragmentation, as well as the transport and depositional processes, and the timing of the events that formed the deposits. In proximal-medial volcanic locations where almost all material begins with an eruptive origin, such knowledge is often impossible, and the same outcrop may be interpreted in different ways by different observers. Therefore for deposit identification purposes, it is preferable to avoid any initial interpretation altogether and only assign genetic names after the most likely depositional process has been assessed. Hence, in the assessment method introduced here, we start by treating all fragmental deposits on a volcanic edifice as only “volcaniclastic deposits” until their possible origin has been evaluated.
Pyroclastic Density Currents and Their Deposits
Pyroclastic density currents are dangerous mass flows of erupted materials and gas that caused 49% (44,928) of all recorded volcanic deaths in the 20th century (Witham, 2005). They can be generated by any process that results in gravitational collapse of erupting volcanic material; including collapsing eruption columns and “boiling over” style eruptions, gravitational or explosive collapse of lava domes (Boudon et al., 1993; Calder et al., 1999; Saucedo et al., 2004; Cronin et al., 2013), collapsing lava flows (Saucedo et al., 2002; Belousov et al., 2011), or co-eruptive remobilization of erupted material falling on steep slopes (Yamamoto et al., 2005). In general, PDCs contain particles that are hotter than the ambient environment, though “cold” PDCs generated by phreatic eruptions can contain material well below magmatic temperatures (Lube et al., 2014).
PDCs form a continuous spectrum from highly mobile, dilute currents dominated by fluid turbulence (traditionally termed pyroclastic surges), to concentrated currents (pyroclastic flows) that have higher basal particle concentrations and are dominated by particle collisions near the lower flow boundary (Branney and Kokelaar, 2002; Sulpizio et al., 2014). In practice the same PDC can exhibit both flow-like and surge-like characteristics at different points in space and time, and as such the cover-all term pyroclastic density current is generally used. However, the concentrated and dilute end-members typically produce very different deposits, and the distinction is also significant from a hazards perspective due to differences in their particle concentrations and mobility (Nakada, 2000; Valentine and Fisher, 2000). Small-volume dilute PDCs are frequently generated by phreatic or phreatomagmatic eruptions (e.g., Kilgour et al., 2010), as well as being generated by separating from moving pyroclastic flows. These energetic currents can overtop local topography, but the area impacted by them is often limited to only a few kilometers from their source (vent or basal avalanche) due to their lower momentum and density (Nakada, 2000). Dilute PDCs typically produce thin, fine-grained deposits that may have limited preservation potential in the long-term volcanic record (Kilgour et al., 2019). In contrast, concentrated PDCs are usually valley-confined and can have runout distances that extend well beyond the main edifice (Crandell, 1987). They are typically accompanied by an overriding dilute component that can separate from the main flow, further increasing the hazard (e.g., Yamamoto et al., 1993; Cole et al., 1998; Cronin et al., 2013). They therefore represent a major threat to life and infrastructure. Concentrated PDCs typically produce massive, poorly sorted deposits that can be difficult to distinguish from deposits from other volcanic mass flows. Hence for the deposit assessment purposes outlined here, the distinction between flow-like and surge-like PDCs is important and we use the legacy terms “pyroclastic flow” and “pyroclastic surge” to describe the dominant process contributing to the observed deposits. Because of the potentially greater extents and hazards associated with pyroclastic flows, and their apparent under-representation in previous studies at Ruapehu, it is these deposits that are the focus of the assessment scheme presented here.
Deposit Characteristics
Pyroclastic flow deposits span an extensive array of textures (Wilson and Walker, 1982; Branney and Kokelaar, 2002; Brown and Andrews, 2015) depending on factors including the generation mechanism and nature of the source material (e.g., Saucedo et al., 2010), transport parameters (particle concentrations, channelization and avulsion (e.g., Cronin et al., 2013), interaction with topography (Sulpizio et al., 2008), particle entrainment (Roche et al., 2013), abrasion (Dufek and Manga, 2008) and communition (Manga et al., 2010)), and depositional variables (en-mass vs. progressive deposition (Branney and Kokelaar, 1992) diachronous deposition (Brown and Branney, 2004), deposit temperature, welding and outgassing (Wright and Cashman, 2014)). Furthermore, post-deposition alteration, and erosion and winnowing of fine material can continue to affect the preserved deposit textures even if parts of the deposit remain in-situ, while more extensive reworking can produce new volcaniclastic deposits containing similar components to the original PDC (e.g., Donoghue et al., 1999; Sarocchi et al., 2011). In general, these processes all combine to produce poorly sorted accumulations of material that contain abundant clasts attributable to a single eruption. However, in proximal locations where all material has a volcanic origin, other volcanic mass flows, glacial processes, mass wasting, and even vent-proximal tephras can all produce texturally similar deposits.
The difficulties in distinguishing proximal-medial (i.e., within the main edifice) pyroclastic flow deposits from other volcaniclastic deposits have been frequently described. Several authors have commented on the similarities between pyroclastic flow and debris flow deposits (e.g., Fisher and Schmincke, 1984; Cas and Wright, 1987; Lerner et al., 2018), and these can be challenging to differentiate in proximal locations. Small-volume block and ash flow deposits (e.g., Saucedo et al., 2004) and debris flow deposits are both typically distributed along drainages, and unconsolidated PDC deposits are then readily remobilized as debris flows (e.g., Davila et al., 2007) containing similar components. Likewise, pumice-rich debris flows (e.g., Bond and Sparks, 1976) and proximal fallout containing ballistically-emplaced clasts can both produce deposits that are texturally similar to pumice-rich pyroclastic flow deposits. Even glacial moraines, which are extensive features on many tall stratovolcanoes, are difficult to distinguish at an outcrop scale from some pyroclastic flow and debris flow deposits (e.g., Hackett, 1985; Cowlyn, 2016).
Evidence of high temperatures (e.g., presence of charcoal, aligned clast magnetic fabric, intact but friable thermally jointed clasts, welding) at the time of deposition is one of the more reliable ways to distinguish pyroclastic flow deposits from other volcaniclastic deposits (Crandell, 1971; Uehara et al., 2015; Lerner et al., 2018; Lerner et al., 2019), especially if the deposit geometry (e.g., channelized deposit) or distance from source rule out hot vent-proximal tephras. Platz et al. (2007, 2012) used aligned magnetic fabric to help identify pyroclastic flow deposits at Taranaki volcano (NZ), but this can be difficult at volcanoes with lower concentrations of magnetic minerals like Ruapehu (Cowlyn, 2016). Furthermore, some pyroclastic flows are relatively cool at deposition and do not preserve high-temperature textures, while co-eruptive debris flows have also been known to contain hot pyroclasts (Mullineaux and Crandell, 1962; Arguden and Rodolfo, 1990). Debris flow deposits can even contain charcoal (Crandell 1987), especially where the debris flows remobilize unconsolidated deposits of earlier pyroclastic flows. There is also evidence that chemically acquired remnant magnetisation can in some instances mislead field magnetometer results (Donoghue et al., 1999), so in the absence of unequivocal high temperature evidence (e.g., welding) it is helpful to document as many different lines of high-temperature evidence as are observed.
In light of the difficulties outlined above, distinguishing pyroclastic flow deposits from other volcaniclastic deposits usually relies on the judgment and experience of the observer to weigh the field evidence and make an interpretation. This is an essential component in any geological fieldwork, but individual expert opinions do no necessarily provide consistency (e.g., Aspinall, 2006; Aspinall, 2010). Several authors (Fisher and Schmincke, 1984; Crandell, 1987) have produced tables of key deposit characteristics that are helpful for guiding field observations and making informed interpretations. However these identification tables usually present considerable overlap in deposit characteristics across different volcaniclastic deposit types, making confident deposit identification difficult (Figure 1). This is especially the case for small or poorly exposed deposits that may not contain definitive identifying textures. A further drawback of traditional identification tables is that they indirectly lead the user toward making a single definitive interpretation that does not then acknowledge the underlying uncertainties and confidence in the interpretation. A consequence of these uncertainties is that many small or poorly exposed volcaniclastic deposits may simply be overlooked or ignored in favor of better preserved deposits. However, when investigating the frequencies and characteristics of volcanic hazards, it is important to identify as many deposits as possible irrespective of deposit size or preservation state.
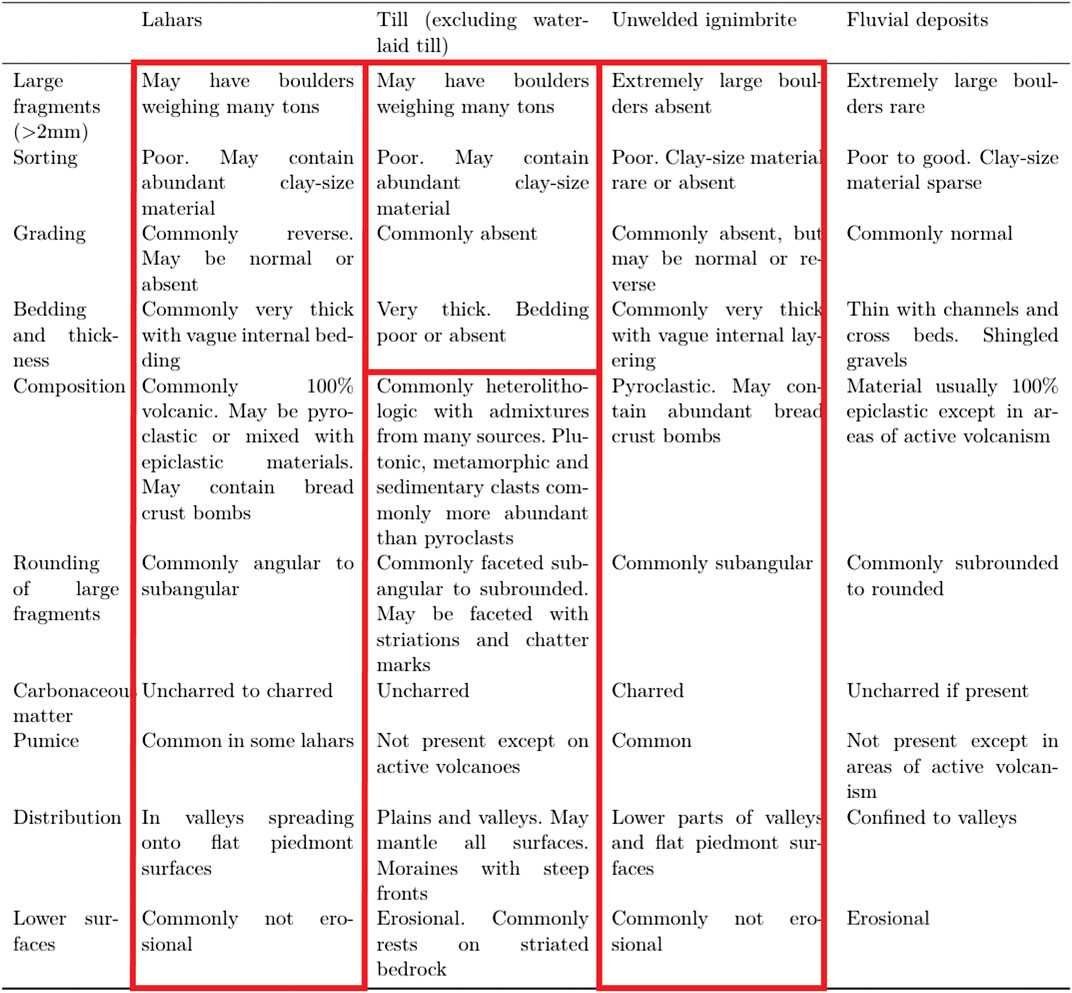
FIGURE 1. Example of an identification table for different volcaniclastic deposits adapted from Fisher and Schmincke (1984). The overlain boxes highlight the similarities between the identifying criteria, making it difficult to confidently distinguish between the different deposits. Adapted by permission from Springer Nature: Springer, Berlin, Heidelberg, Pyroclastic Rocks by Fisher, R.V. and Schmincke, H.‐U., COPYRIGHT 1984.
Methods
In order to investigate pyroclastic flow deposits at Ruapehu, we developed a novel system for quickly and consistently assessing the possible origins of the different volcaniclastic deposits observed. The aim of this system was to distill the reasoning of an experienced field geologist into a more structured and repeatable method for assessing candidate pyroclastic flow deposits, and at the same time explicitly address any interpretative uncertainty. This follows four principles:
(1) To identify deposit features that directly support or oppose an interpretation that the deposit was emplaced by a pyroclastic flow.
(2) To identify deposit features that might instead suggest the deposit was emplaced by other volcaniclastic processes, and hence challenge a pyroclastic flow interpretation.
(3) To use (1) and (2) to assess the confidence by which the deposit can be interpreted to have been emplaced by a pyroclastic flow, and to compare that to the confidence by which it may be interpreted to have been emplaced by other processes.
(4) Where the deposit features can be explained by more than one volcaniclastic process, to recognize there is uncertainty in the interpretation and therefore to identify the process(es) that may best explain the observed features.
In practical terms, certain criteria are more useful than others when initially assessing a deposit’s origin. For example, a deposit containing abundant vesicular clasts could have been emplaced by any volcaniclastic process since there is often abundant vesicular material available for reworking on a volcanic edifice. Hence the presence of vesicular clasts, by itself, would not significantly help identify a deposit’s origin. In contrast, a welded volcaniclastic deposit could only have been emplaced by “hot” volcaniclastic processes such as proximal spatter/tephra or pyroclastic flows. The presence of welding alone would therefore rule out “cold” volcaniclastic processes irrespective of any deposit textures that may otherwise support them, and hence would greatly focus the interpretation. An experienced field geologist intuitively uses the most indicative features to focus their initial assessment, and this is something we aim to replicate in the assessment scheme. Therefore to identify the most “useful” criteria for assessing pyroclastic flows and other common volcaniclastic deposits, we first compiled a list of characteristic outcrop-scale deposit features (Figure 2 and digital appendix). We then focused on those criteria that:
(1) Identify deposits that are more likely to have been emplaced directly during an eruption (i.e., similar to White and Houghton’s (2006) “Primary Volcaniclastic”)
(2) Are likely to be observable in most outcrops irrespective of outcrop size or preservation state.
(3) Are particularly useful as standalone criteria to identify specific volcaniclastic processes, especially where the feature helps support or oppose an interpretation of deposition from a pyroclastic flow.
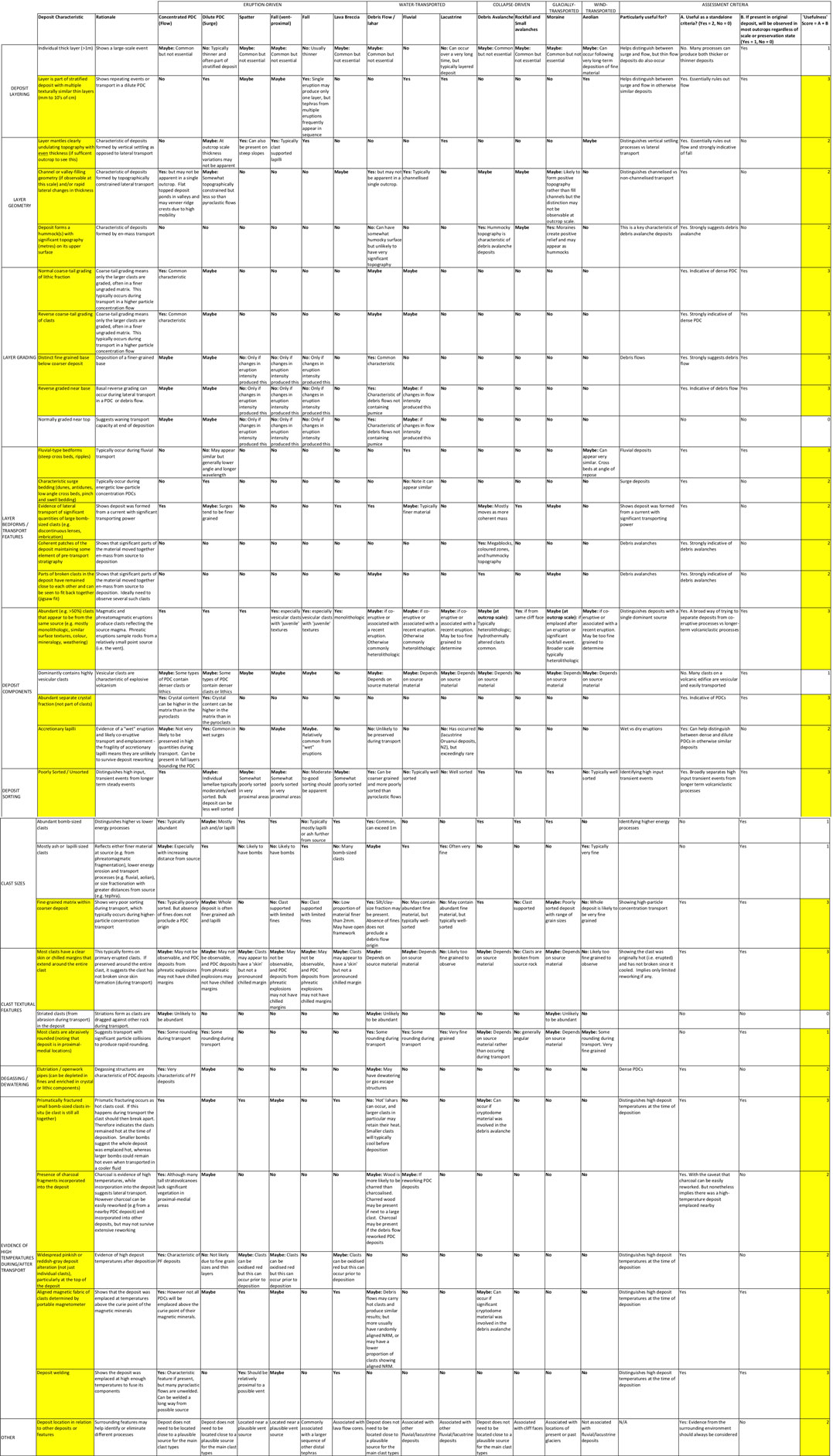
FIGURE 2. Key deposit characteristics resulting from most common volcaniclastic processes. Criteria are assessed on their usefulness as standalone criteria for identifying particular volcaniclastic processes (scored from 0 to 2), and on their ability to be observed in any deposit (scored from 0 to 1). Highlighted criteria, including all criteria with a total ‘usefulness’ score
After focusing and combining similar criteria from Figure 2, a shortlist of 15 criteria were identified for use in the final assessment scheme.
A Confidence-Based Method for Assessing Candidate Pyroclastic Flow Deposits
The new system for assessing candidate pyroclastic flow deposits is presented in Figure 3, and also as an excel worksheet in the digital appendix. A glossary for the assessment criteria is provided in Appendix 1. It is important to note that the assessment scheme’s observational criteria are tailored specifically for assessing whether the deposit features are consistent with deposition by a pyroclastic flow, and are not intended as an in-depth assessment of other volcaniclastic deposits. It is assumed that the deposit is located on/near a volcanic source and within the reasonable range of PDCs.
The assessment begins with two observations that can be determined in every deposit regardless of size or state of preservation. The first, “does the deposit contain >50% compositionally and texturally similar volcanic clasts,” identifies deposits that are mostly homogenous, and hence reflects a dominant particle source. This is consistent with (but not limited to) deposits emplaced during or shortly after an eruption. It is therefore similar to White and Houghton’s (2006) “Primary Volcaniclastic” classification, but recognizes that erupted material does not always have juvenile clast textures, and that primary deposits can be difficult to distinguish from secondary reworking of freshly erupted material.
The second observation considers whether the deposit has poor vs. moderate/good sorting. This distinguishes between deposits from transient, high energy and high particle concentration events like pyroclastic flows, and those from steadier and typically lower energy and lower particle concentration processes whose clasts become better sorted during transport. Together, the first two observations focus the assessment into three categories that broadly distinguish the most likely volcaniclastic processes to have formed the deposit in each case. These are:
(1) Heterolithologic deposits: Heterolithologic deposits cannot confidently be associated with an eruption, so are considered “unlikely” to have been emplaced by a pyroclastic flow. The most likely types of heterolithologic deposits are fluvial, lacustrine and eolian deposits, glacial moraines, debris flow and debris avalanche deposits, and phreatic tephras (including phreatic surges).
(2) Homogenous, poorly sorted deposits: Homogenous deposits might be associated with an eruption and so it is possible they were emplaced by a pyroclastic flow. The most likely types of poorly sorted homogenous deposits are pyroclastic flow deposits and the more poorly sorted surge deposits, co/post-eruptive debris flow deposits, proximal fall deposits, lava breccias, rockfall deposits, and post-eruptive moraines.
(3) Homogenous, moderately/well sorted deposits: Homogenous deposits might be associated with an eruption and so it is possible they were emplaced by a pyroclastic flow. However, pyroclastic flow deposits are typically poorly sorted. The most likely types of moderately/well sorted homogenous deposits are fall deposits, better sorted pyroclastic surge deposits, and post-eruptive fluvial, lacustrine and aeolian deposits.
While deposits from different volcaniclastic processes will not always fit neatly into these categories, the categories are sufficiently characteristic that their presence or absence strongly influences the confidence in a given interpretation. For example, it would be difficult to confidently attribute a poorly sorted deposit to low-energy fluvial processes, or to interpret a heterolithologic deposit as a lava breccia. Therefore, the three categories sufficiently focus the range of possible interpretations that the remaining assessment criteria can then function as independent (standalone) items which by themselves either support emplacement from a particular volcaniclastic process, or oppose it and eliminate that process from further consideration. However, unlike the first two criteria which can be assessed for any deposit irrespective of size or preservation state, the remaining 13 criteria are process-specific and hence only some of these apply for any given deposit. As such, only those that are actually observed in the deposit are used for the final assessment.
Assigning Confidence Scores
In order to assess which volcaniclastic process(es) are most likely to have formed a given deposit, the user first determines which of the 13 standalone criteria are present in the deposit, placing a tick next to each that applies. These 13 criteria are associated with a series of pre-assigned values, assigned by the authors, that show how indicative each criteria is of deposits from the different volcaniclastic processes (Boxes A and B, Figure 3). Values 1−4 mean the criteria sometimes occurs in deposits from the relevant process, with 1 meaning it is not strongly indicative (i.e., the feature’s presence does not strongly support that volcaniclastic process) and 4 meaning it is very strongly indicative (i.e., the feature’s presence strongly supports that process). A value of 5 means that the criteria opposes emplacement by that process, eliminating it from further consideration. So, for example, the first three indicative values for the criteria “layer is part of a stratified deposit with multiple thin layers” (Figure 3) are:
This in essence states that thinly stratified layering is unlikely to occur in deposits from pyroclastic flows (5), strongly indicative of deposits from surges (3), and can occur in spatter deposits but is not as indicative (2). Because several of the 13 standalone criteria may be present in any given deposit, their respective indicative values then need to be combined to determine the deposit’s overall “score.” Here, the highest indicative value for each volcaniclastic process is the one used. Hence, if a deposit is both “stratified” and has “evidence of high temperature emplacement” (i.e., 2 of the 13 standalone criteria) then their first three indicative values and final assessment scores are:
Carrying forward the highest values reflects the standalone nature of each of the 13 criteria - that is to say that for each of the criteria observed in the deposit, it is the criteria that most strongly points to emplacement by a particular process that supersedes other criteria that are less strongly indicative. This is also why the highest indicative value (5) is assigned when a criteria opposes emplacement from a particular process, since any observation that eliminates a process must supersede any other criteria that might support it. The resulting final assessment scores therefore reflect how indicative all of the observed criteria, taken together, are of emplacement by each of the different volcaniclastic processes. As such, they indicate the level of confidence by which the deposit’s features can be explained by each volcaniclastic process. A final score of 1–4 indicates the deposit features are consistent with emplacement from that process, at increasing levels of confidence (low, moderate, high, very high). A final score of 5 means the deposit features are unlikely to have been formed by that process. Hence in the example above, the deposit features are consistent with surge (3 = high confidence) or spatter deposits (3 = also high confidence), but are unlikely to have formed from a pyroclastic flow (5 = unlikely).
While the assessment scheme provides a rapid, standardized mechanism for assessing candidate pyroclastic flow deposits, it cannot cover every possible observational criteria and is not intended to supersede additional observations that assist the final interpretation. Therefore, more experienced users may manually adjust scores or introduce additional observational criteria, if present, that change the confidence ratings. Any such adjustments should be documented, and if additional criteria are introduced then appropriate user-defined indicative values must be allocated in Boxes A and B. The paper chart includes a blank row for this purpose. The excel worksheet in the digital appendix automates the scoring process outlined above, and may be used in the field using freely available spreadsheet software for smartphones or tablet computers.
Application of the Method to a Volcaniclastic Deposit at Ruapehu Volcano
Fieldwork by Cowlyn (2016) identified an extensive pumiceous volcaniclastic deposit on the northern edge of Ruapehu’s Mangatoetoenui valley (Figure 4). Chapman (1996) had previously interpreted this deposit as a tephra-covered moraine, but acknowledged that its thick tephra cover had prevented observation of the internal fabric. Chapman’s (1996) interpretation was likely guided by the overall morphology of the remaining deposit, which at its western edge superficially resembles a lateral moraine ridge and glacial close-out loop. Cowlyn (2016) later found several incised gulleys where small patches of the in-situ deposit were exposed. These showed that the deposit was dominantly composed of at least two poorly sorted volcaniclastic units containing a mix of large pumices and lithic clasts in a finer grained matrix, and separated in at least one location by a layered ash. Field observations of the lowermost unit revealed:
• The layer contains approximately 50% pumice and 50% lithic clasts for much of its thickness, but this increases to ∼95% pumice at the top. The pumices are texturally and compositionally similar.
• The layer is poorly sorted, containing lapilli and bomb-sized clasts and a significant amount of ash matrix.
• Although not observable at a single-outcrop scale, the larger deposit sequence (all layers) completely fills a small palaeovalley.
• At the top of the lowermost layer the number of very large pumices increases (i.e., reverse coarse-tail grading of pumices).
• Several of the pumices appear to have been abrasively rounded.
• The deposit is ∼5 km from Ruapehu’s summit and not near any known vents.
• The layer is at least 10 m thick
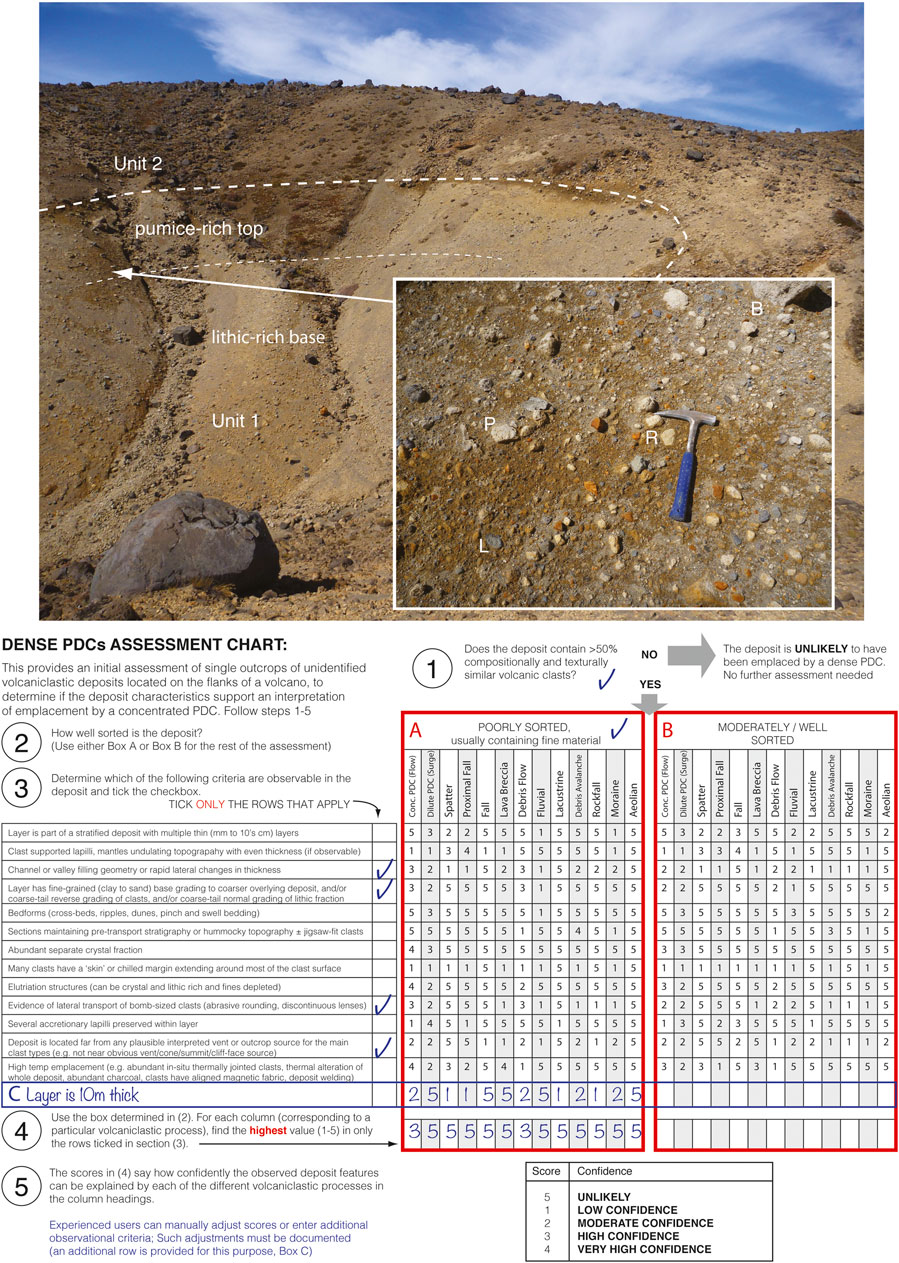
FIGURE 4. Example volcaniclastic deposit from Ruapehu volcano. This poorly sorted deposit contains pumice (P) and lithic (L) clasts in a fine-grained matrix. Many of the pumices are bomb-sized (B) and show signs of abrasive rounding during transport (R). The final confidence scores (Step 4) are the highest numbers from the columns above, following the process detailed in Section 5.1 of the main text. Here, the scores suggest the observed textures are best attributed to either a debris flow or a pyroclastic flow, both at “high confidence.” Note the manual addition of an additional assessment criteria (deposit thickness) and associated user-generated indicative scores in Box C. This demonstrates how more experienced users can modify the scheme in light of their own observations; in this case helping to eliminate surge and fluvial deposits from the interpretation.
An initial field assessment (without using the assessment scheme) was that the deposit resembled a debris flow deposit that had reworked freshly erupted pumices and entrained significant amounts of lithic material. This would be a reasonable experience-based assessment, particularly in light of the high proportion of lithic material. Working through the new assessment and manually including the additional observation of the deposit’s 10 m thickness, together with user-defined indicative scores for this extra criteria (Figure 4, Box C), the scheme agrees that the observed deposit features are consistent with emplacement from a debris flow at “high confidence.” However, the assessment also suggests that the deposit features can be explained by emplacement from a pyroclastic flow, again with “high confidence” (Figure 4). The assessment result therefore explicitly encourages consideration of both interpretations. In cases where it is important to definitively identify a deposit, the results of this initial assessment can inform any follow-up field or laboratory investigations (e.g., component or grain size analyses) that may then refine the interpretation. For the Ruapehu deposit, investigations with a portable fluxgate magnetometer (e.g., Platz et al., 2012) showed that many of the bomb-sized clasts had aligned magnetism consistent with hot emplacement (Cowlyn, 2016), most strongly supporting emplacement by a pyroclastic flow with a new score of 4 (“very high confidence”). At a volcano with few documented pyroclastic flows, this is an important finding, and highlights the importance of recognizing and addressing interpretative uncertainty.
Discussion
The assessment scheme presented here is a rapid, repeatable method for assessing volcaniclastic deposits of unknown origin to determine if they may have been emplaced by a pyroclastic flow. Where possible, it uses criteria that are easily observed at single outcrops of any scale, while rarer criteria are only used to distinguish between similar deposits or increase interpretative confidence. Hence, this system can be used at any outcrop irrespective of size or preservation state, and irrespective of how many (or how few) deposit characteristics can be observed. This is advantageous, as it encourages assessment of even poorly-exposed deposits that may otherwise be overlooked. The system differs from identification tables that typically list “ideal” deposit features, as these do not address the significant variability of natural systems or acknowledge the resulting interpretative uncertainty. The new system explicity addresses such uncertainty by identifying processes that could have produced the observed deposit and quantifying how confidently each of those processes explain the deposit features. In this way, the most likely process(es) to have formed the assessed deposit features can be identified and any final interpretation fully justified.
Instead of only looking for criteria that support emplacement by a pyroclastic flow, the assessment system actively looks for features that distinguish between these and other potentially similar deposits. In other words, the system actively considers and tries to eliminate other possible interpretations in order to increase the interpretative confidence. This, together with transparently reporting any remaining uncertainty, is a best-practice approach applicable to research investigations of all kinds. As such, the assessment scheme is useful as a teaching and discussion tool that guides students toward developing a scaffolding framework that encourages expert-like habits (Petcovic and Libarkin, 2007). It is important to remember, however, that the assessment scheme’s observational criteria are tailored specifically for assessing whether the deposit features are consistent with deposition by a pyroclastic flow. As such, the assessment is not suitable for fully identifying deposits from other volcaniclastic processes. To do so would require observations of additional assessment criteria tailored specifically to those deposits. For example, the presence of “bedforms” is here sufficient to challenge an interpretation that the deposit was emplaced by a pyroclastic flow, but does not provide the necessary detail (e.g., bedform type, angle, wavelength) to correctly distinguish between fluvial or surge deposits. Therefore, when different volcaniclastic processes return higher confidence scores, this serves to show that the observed deposit features may not be best explained by deposition from a pyroclastic flow, but does not provide an in-depth assessment of the other volcaniclastic processes. Nevertheless, the basic principles governing this confidence-based approach can easily be adapted to any geological deposit assessment (not just volcaniclastic deposits) by modifying the specific assessment criteria for the required purpose.
The biggest limitation of the scheme is that 15 criteria cannot cover every possible deposit feature useful to an interpretation, and hence it cannot fully substitute for professional experience. An experienced field investigator integrates multiple lines of evidence to quickly access and assess prior knowledge (Petcovic and Libarkin, 2007) in a way that is not possible within the rigid framework of a chart or table. However the assessment scheme partially addresses this limitation by explicitly allowing the user to manually adjust the confidence ratings in light of additional observational evidence and personal experience (see example in Figure 4). This is not a “fudge” factor, as it still requires the user to justify and record any changes and report the adjusted confidence scores across the full range of volcaniclastic processes. Hence the user must still justify their final interpretations and acknowledge remaining uncertainty. As such, an experienced volcanologist can modify the scheme as needed while still maintaining its core principle of interpretive transparency.
Torres-Orozco et al. (2018) showed that grouping different lithofacies from multiple outcrops into broader lithofacies associations is a powerful approach to interpreting eruptive processes, and highlights the benefits of detailed fieldwork across multiple sites. The assessment scheme presented here cannot replace this kind of detail. Instead, it is hoped that the scheme’s simplicity encourages initial investigation and discussion of multiple deposits that may then form a starting point for further studies.
Conclusions
The assessment scheme developed here provides a new method for rapidly and consistently assessing volcaniclastic deposits to determine if they could have been emplaced by a pyroclastic flow. The scheme differs from previous identification systems as it explicitly acknowledges that different volcaniclastic processes can produce texturally similar deposits and hence result in interpretative uncertainty. Therefore, the final interpretation is presented in terms of which process(es) most confidently explain the observed features, while still recognizing other processes that could have formed the deposit. This approach encourages assessment of all deposits regardless of size or preservation state. Recognition of pyroclastic flow deposits is important for quantifying the frequency and magnitudes of the hazard (Le Pennec et al., 2016), so rapid deposit assessments such as this can provide a valuable input for future probabilistic hazard assessments (e.g., Marzocchi et al., 2004) at high-risk volcanoes like Ruapehu.
Data Availability Statement
The datasets presented in this study can be found in online repositories. The names of the repository/repositories and accession number(s) can be found in the article/Supplementary Material.
Author Contributions
JC developed the deposit assessment scheme and wrote the manuscript; BK, DG, and SC provided feedback for the assessment scheme and manuscript.
Funding
Funding for JC’s PhD research was provided by a University of Canterbury Doctoral Scholarship, the University of Canterbury Mason Trust Fund, and a Project Tongariro Memorial Award. JC’s Postdoc at the Georgia Institute of Technology was supported by NSF Project 3506H14. BK and SC acknowledge NSC Resilience to natures challenges: Āhea riri ai ngā maunga puia? When will our volcanoes become angry? Ministry of Business, Innovation & Employment.
Conflict of Interest
The authors declare that the research was conducted in the absence of any commercial or financial relationships that could be construed as a potential conflict of interest.
Acknowledgments
JC acknowledges helpful feedback from Josef Dufek, and feedback for the relevant PhD chapter from Richard Brown and Geoff Kilgour. Andrew Cullen investigated possible implementation of the scheme as a smartphone app. Harry Keys, the NZ Department of Conservation, Ngāti Tūwharetoa and Ngāti Rangi facilitated research at Ruapehu. Versions of the scheme were trialed by students at the Georgia Institute of Technology (United States) and the “Frontiers Abroad” study abroad program in New Zealand.
Supplementary Material
The Supplementary Material for this article can be found online at: https://www.frontiersin.org/articles/10.3389/feart.2020.581195/full#supplementary-material
Appendix: Glossary of Assessment Criteria
>50% Compositionally and Texturally Similar Volcanic Clasts.
A broad assessment of whether the majority of volcanic clasts in the deposit are sufficiently similar that they might have come from the same source and hence may have been produced during the same volcanic eruption. The clasts may appear to have similar colors, surface textures, vesicularity, mineralogy, and similar weathering or alteration patterns.
Sorting
Poorly sorted deposits have components spanning a wide range of grain sizes. The presence of very large clasts is common but not a prerequisite for poor sorting; deposits containing significant amounts of both ash (<2 mm) and lapilli (2–64 mm) would still be considered poorly sorted.
Moderate/well sorted deposits contain clasts whose sizes are broadly similar. In fine-grained deposits (i.e., grain sizes <2 mm) larger clasts should be absent or very rare. In deposits containing mostly larger grain sizes (>2 mm), a small amount of finer material may be stuck to the surfaces of the larger clasts, but there should not be significant amounts of fines.
Layer is Part of a Stratified Deposit With Multiple Thin (mm to 10’s cm) Layers
The deposit contains multiple layers whose textures are sufficiently similar that it is clear to the observer that they were all formed by the same volcaniclastic process. This assessment criteria is sufficiently broad that it applies to both individual events (e.g., a layered sequence of surge deposits originating from a single eruption) and longer term processes (e.g., a sequence of fall deposits from several different eruptions but all sharing textural similarities).
Clast Supported Lapilli, Mantles Undulating Topography With Even Thickness (if Observable)
This is particularly for deposits containing dominantly lapilli-sized clasts. The clasts should be in contact with each other, without significant interstitial matrix. The deposit should also maintain an even thickness over any underlying topography, though this may not be observable at all outcrops.
Channel or Valley Filling Geometry or Rapid Lateral Changes in Thickness
The deposit is laterally constrained by a channel or by surrounding topography (e.g., valley sides). This can be at any scale (centimetres to kilometers), so where the deposit extends beyond a single outcrop this should be considered when possible. Generally, any rapid change in deposit thickness suggests lateral emplacement mechanisms. Care should be taken to determine that thickness changes are original and not a result of later erosion.
Layer has Fine-Grained (Clay to Sand) Base Grading to Coarser Overlying Deposit, and/or Coarse-Tail Reverse Grading of Clasts, and/or Coarse-Tail Normal Grading of Lithic Fraction
Any fine-grained base should be part of the unit as a whole and typically grade into the overlying deposit. Coarse-tail grading refers to systematic vertical changes in grain size for only the coarser clasts. This typically occurs in deposits that have a finer matrix, where, for example the lapilli or bomb-sized clasts get larger (coarse-tail reverse grading) or smaller (coarse-tail normal grading) vertically up-sequence, but the finer matrix remains the same.
Bedforms (Cross-Beds, Ripples, Dunes, Pinch and Swell Bedding)
The assessment scheme makes no differentiation between bedforms produced by pyroclastic surges (typically shallower and longer wavelength) vs. fluvial/aeolian processes (typically steeper and shorter wavelength), but instead uses the presence of bedforms to differentiate these processes from other volcaniclastic processes that typically do not produce bedforms.
Sections Maintaining Pre-Transport Stratigraphy or Hummocky Topography ± Jigsaw-Fit Clasts
These features are typical of debris avalanche deposits. Hummocks should be clearly defined, indicating significant topography to the deposit’s upper surface, and preferably with several hummocks observed. These may be metre-scale to many tens of meters in size. Since large sections of material move together en-mass in a debris avalanche, the deposit may contain hints of the source stratigraphy. The key here is identifying zones within an otherwise fragmental deposit that suggest sections moved together en-mass despite their fragmental nature. Examples include coherent patches of alteration/color or zones containing concentrations of distinct clasts. Similarly, jigsaw-fit clasts are larger clasts that have broken during transport and whose fragments have moved slightly apart, but have nonetheless remained sufficiently close to each other to still be identified as belonging to the same original clast.
Abundant Separate Crystal Fraction
Abundant free crystals in the ash-sized fraction of a deposit. The crystals should be the same compositions as the phenocryst phases in the dominant larger clasts.
Many Clasts Have a “Skin” or Chilled Margin Extending Around Most of the Clast Surface
A thin fine-grained or glassy rind that forms due to rapid cooling at the surface of a hot pyroclast. Breaking a clast open can show if the outer surface is texturally different to the very immediate (i.e., within < millimeter) interior, and can evidence a very thin “skin” typical of many pyroclasts even if a more pronounced chilled margin is not present.
If the “skin” or chilled margin is present around the entire clast, this shows the clast was formed when hot (i.e., juvenile/primary) and subsequently remained intact; and hence was not fragmented or abraded by the longer-term erosive system.
Elutriation Structures (can be Crystal and Lithic Rich and Fines Depleted)
Vertical pipe-like structures within the deposit evidencing vertical movement of gases or water that may have carried away finer or less dense material.
Evidence of Lateral Transport of Bomb-Sized Clasts (Abrasive Rounding, Discontinuous Lenses)
Any evidence that large clasts in the deposit have been laterally transported across the volcanic surface prior to deposition. Clasts may be noticeably rounded, imbricated, or show evidence of lateral interaction with surrounding clasts in the deposit (e.g., groups of larger clasts forming discontinuous lenses).
Several Accretionary Lapilli Preserved Within Layer
Accretionary lapilli are spherical aggregates of fine ash, typically with concentrically layered interiors. They evidence co-eruptive interaction with water, and are often associated with pyroclastic surge and phreatomagmatic fall deposits. Due to their fragility, they are unlikely to survive significant transport in higher particle concentration flows or significant reworking.
Deposit is Located Far From Any Plausible Interpreted Vent or Outcrop Source for the Main Clast Types (e.g., Not Near Obvious Vent/Cone/Summit)
This criteria relies on user judgment, but is designed to identify material that is likely to have been transported a substantial distance before deposition. This criteria may be difficult to assess for older deposits.
High Temp Emplacement (e.g., Abundant In-Situ Thermally Jointed Clasts, Thermal Alteration of Whole Deposit, Abundant Charcoal, Clasts Have Aligned Magnetic Fabric, Deposit Welding)
This criteria looks for evidence that the deposit was hot at the time of emplacement. Thermally jointed clasts have prismatic interior cooling fractures radiating perpendicularly inwards from their surfaces that cause them to disintegrate when removed from the deposit. The clasts’ fragility suggests that the fractures only developed after deposition, meaning they were still hot during transport. Deposit thermal alteration, extending across clasts and matrix, is often evidenced by pink or reddish alteration toward the top of the deposit. Magnetic field orientations of larger clasts can be determined using a magnetometer; consistent (aligned) field directions suggest the clasts only cooled through the Curie temperature after deposition. Deposit welding, where clasts have fused with surrounding clasts, is very clear evidence of high temperature deposition.
Charcoal fragments within a deposit suggest the interaction of high temperature material with vegetation and is a strong indicator of pyroclastic flows. However, unconsolidated pyroclastic flow deposits are easily reworked, so charcoal alone is a slightly less reliable indicator of hot emplacement. Therefore, any evidence that the deposit has not been reworked should be documented.
References
Aramaki, S., and Akimoto, S. (1957). Temperature estimation of pyroclastic deposits by natural remanent magnetism. Am. J. Sci. 255, 619–627. doi:10.2475/ajs.255.9.619
Arguden, A. T., and Rodolfo, K. S. (1990). Sedimentologic and dynamic differences between hot and cold laharic debris flows of Mayon Volcano, Philippines. Geol. Soc. Am. Bull. 102, 865–876. doi:10.1130/0016-7606(1990)102〈0865:SADDBH〉2.3.CO;2
Aspinall, W. (2010). A route to more tractable expert advice. Nature 463, 294–295. doi:10.1038/463294a
Aspinall, W. P. (2006). “Structured elicitation of expert judgement for probabilistic hazard and risk assessment in volcanic eruptions,” in Statistics in volcanology. Editors H. M. Mader, S. Coles, C. Connor, and L. Connor (London, UK: Special Publications of IAVCEI No. 1, The Geological Society for IAVCEI), 15–30. doi:10.1144/iavcei001.2
Belousov, A., Behncke, B., and Belousova, M. (2011). Generation of pyroclastic flows by explosive interaction of lava flows with ice/water-saturated substrate. J. Volcanol. Geoth. Res. 202, 60–72. doi:10.1016/j.jvolgeores.2011.01.004
Bernard, B., Van Wyk de Vries, B., and Leyrit, H. (2009). Distinguishing volcanic debris avalanche deposits from their reworked products: the perrier sequence (French Massif Central). Bull. Volcanol. 71, 1041–1056. doi:10.1007/s00445-009-0285-7
Bond, A., and Sparks, R. S. J. (1976). The minoan eruption of Santorini, Greece. J. Geol. Soc. 132, 1–16. doi:10.1144/gsjgs.132.1.0001
Boudon, G., Camus, G., Gourgaud, A., and Lajoie, J. (1993). The 1984 nuée-ardente deposits of Merapi volcano, Central Java, Indonesia: stratigraphy, textural characteristics, and transport mechanisms. Bull. Volcanol. 55, 327–342. doi:10.1007/BF00301144
Branney, M. J., and Kokelaar, P. (1992). A reappraisal of ignimbrite emplacement: progressive aggradation and changes from particulate to non-particulate flow during emplacement of high-grade ignimbrite. Bull. Volcanol. 54, 504–520. doi:10.1007/BF00301396
Branney, M. J., and Kokelaar, P. (2002). Pyroclastic density currents and the sedimentation of ignimbrites. Bath, UK: Geological Society Publishing House, 143.
Brown, R. J., and Andrews, G. D. (2015). “Deposits of pyroclastic density currents,” in The encyclopedia of volcanoes. 2nd Edn. Editors H. Sigurdsson, B. Houghton, S. McNutt, H. Rymer, and J. Stix (Cambridge, MA: Elsevier Academic Press), Chap. 36, 631–648. doi:10.1016/B978-0-12-385938-9.00036-5
Brown, R. J., and Branney, M. J. (2004). Bypassing and diachronous deposition from density currents: evidence from a giant regressive bed form in the Poris ignimbrite, Tenerife, Canary Islands. Geology 32, 445–448. doi:10.1130/G20188.1
Calder, E. S., Cole, P. D., Dade, W. B., Druitt, T. H., Hoblitt, R. P., Huppert, H. E., et al. (1999). Mobility of pyroclastic flows and surges at the Soufriere Hills Volcano, Montserrat. Geophys. Res. Lett. 26, 537–540. doi:10.1029/1999GL900051
Capra, L., and Macı́as, J. L. (2002). The cohesive Naranjo debris-flow deposit (10 km3): A dam breakout flow derived from the Pleistocene debris-avalanche deposit of Nevado de Colima Volcano (México). J. Volcanol. Geoth. Res. 117, 213–235. doi:10.1016/S0377-0273(02)00245-7
Cas, R., and Wright, J. (1987). Volcanic successions: modern and ancient. London, UK: Allen & Unwin, 528.
Chapman, J. (1996). Geology and geochemistry of andesitic rocks of the upper Mangatoetoenui Valley, Mount Ruapehu, North Island, New Zealand. Bsc honours thesis. Bundoora (New Zealand): La Trobe University.
Charbonnier, S. J., Germa, A., Connor, C. B., Gertisser, R., Preece, K., Komorowski, J.-C., et al. (2013). Evaluation of the impact of the 2010 pyroclastic density currents at Merapi volcano from high-resolution satellite imagery, field investigations and numerical simulations. J. Volcanol. Geoth. Res. 261, 295–315. doi:10.1016/j.jvolgeores.2012.12.021
Cole, P. D., Calder, E. S., Druitt, T. H., Hoblitt, R., Robertson, R., Sparks, R. S. J., et al. (1998). Pyroclastic flows generated by gravitational instability of the 1996–97 lava dome of Soufriere Hills Volcano, Montserrat. Geophys. Res. Lett. 25, 3425–3428. doi:10.1029/98GL01510
Cowlyn, J. D. (2016). Pyroclastic density currents at Ruapehu volcano; New Zealand. PhD thesis. Christchurch (New Zealand): University of Canterbury.
Crandell, D. (1971). Postglacial lahars from Mount Rainier Volcano, Washington. Washington, D.C.: U. S. Geological Survey Professional Paper, 75.
Crandell, D. (1987). Deposits of pre-1980 pyroclastic flows and lahars from Mount St. Helens Volcano, Washington. U.S. Denver, CO: Geological Survey Professional Paper, 93.
Cronin, S. J., Lube, G., Dayudi, D. S., Sumarti, S., Subrandiyo, S., and Surono, (2013). Insights into the October-November 2010 Gunung Merapi eruption (Central Java, Indonesia) from the stratigraphy, volume and characteristics of its pyroclastic deposits. J. Volcanol. Geoth. Res. 261, 244–259. doi:10.1016/j.jvolgeores.2013.01.005
Davila, N., Capra, L., Gavilanes-Ruiz, J. C., Varley, N., Norini, G., and Vazquez, A. G. (2007). Recent lahars at Volcán de Colima (Mexico): drainage variation and spectral classification. J. Volcanol. Geoth. Res. 165, 127–141. doi:10.1016/j.jvolgeores.2007.05.016
Donoghue, S. L., Gamble, J. A., Palmer, A. S., and Stewart, R. B. (1995). Magma mingling in an andesite pyroclastic flow of the Pourahu Member, Ruapehu volcano, New Zealand. J. Volcanol. Geoth. Res. 68, 177–191. doi:10.1016/0377-0273(95)00012-J
Donoghue, S. L., Palmer, A. S., McClelland, E., Hobson, K., Stewart, R. B., Neall, V. E., et al. (1999). The Taurewa Eruptive Episode: evidence for climactic eruptions at Ruapehu volcano, New Zealand. Bull. Volcanol. 61, 223–240. doi:10.1007/s004450050273
Druitt, T. H. (1998). Pyroclastic density currents. Geol. Soc. Lond. Spec. Public. 145, 145–182. doi:10.1144/GSL.SP.1996.145.01.08
Dufek, J., and Manga, M. (2008). In situ production of ash in pyroclastic flows. J. Geophys. Res. 113, 1–17. doi:10.1029/2007JB005555
Fisher, R. V. (1966). Rocks composed of volcanic fragments and their classification. Earth Sci. Rev. 1, 287–298. doi:10.1016/0012-8252(66)90010-9
Fisher, R. V., and Schmincke, H.-U. (1984). Pyroclastic rocks. (Berlin, Heidelberg, New York, Tokyo: Springer-Verlag), 472.
Freundt, A., Wilson, C., and Carey, S. (2000). “Ignimbrites and block-and-ash flow deposits,” in Encyclopedia of volcanoes. Editors H. Sigurdsson, B. Houghton, S. McNutt, H. Rymer, and J. Stix (San Diego, CA: Academic Press), 581–599.
Gran, K. B., and Montgomery, D. R. (2005). Spatial and temporal patterns in fluvial recovery following volcanic eruptions: channel response to basin-wide sediment loading at Mount Pinatubo, Philippines. Geol. Soc. Am. Bull. 117, 195–211. doi:10.1130/B25528.1
Hackett, W. (1985). Geology and petrology of Ruapehu volcano and related vents. PhD thesis. Wellington (New Zealand): Victoria University
Hoblitt, R. P., and Kellogg, K. S. (1979). Emplacement temperatures of unsorted and unstratified deposits of volcanic rock debris as determined by paleomagnetic techniques. Geol. Soc. Am. Bull. 90, 633. doi:10.1130/0016-7606(1979)90〈633:ETOUAU〉2.0.CO;2
Johnson, A. M. (1970). Physical processes in geology, a method for interpretation of natural phenomena, intrusions in igneous rocks, fractures, and folds; flow of debris and ice. San Francisco, CA: Freeman Cooper, 577
Kilgour, G., Gates, S., Kennedy, B., Farquhar, A., McSporran, A., and Asher, C. (2019). Phreatic eruption dynamics derived from deposit analysis: a case study from a small, phreatic eruption from Whakāri/White Island, New Zealand. Earth Planets Space. 71, 36. doi:10.1186/s40623-019-1008-8
Kilgour, G., Manville, V. V., Pasqua, F. D., Graettinger, A. A., Hodgson, K. A., and Jolly, G. E. (2010). The 25 September 2007 eruption of Mount Ruapehu, New Zealand: directed ballistics, surtseyan jets, and ice-slurry lahars. J. Volcanol. Geoth. Res. 191, 1–14. doi:10.1016/j.jvolgeores.2009.10.015
Leonard, G. S., Stewart, C., Wilson, T. M., Procter, J. N., Scott, B. J., Keys, H. J., et al. (2014). Integrating multidisciplinary science, modelling and impact data into evolving, syn-event volcanic hazard mapping and communication: a case study from the 2012 Tongariro eruption crisis, New Zealand. J. Volcanol. Geoth. Res. 286, 208–232. doi:10.1016/j.jvolgeores.2014.08.018
Le Pennec, J.-L., Ramón, P., Robin, C., and Almeida, E. (2016). Combining historical and 14C data to assess pyroclastic density current hazards in Baños city near Tungurahua volcano (Ecuador). Quat. Int. 394, 98–114. doi:10.1016/j.quaint.2015.06.052
Lerner, G. A., Cronin, S., and Turner, G. (2018). Evaluating emplacement temperature of a 1000-year sequence of mass flows using paleomagnetism of their deposits at Mt. Taranaki, New Zealand. Volcanica 2, 11–24. doi:10.30909/vol.02.01.1124
Lerner, G. A., Cronin, S. J., Turner, G. M., and Piispa, E. J. (2019). Recognizing long-runout pyroclastic flow deposits using paleomagnetism of ash. Bull. Geol. Soc. Am. 131, 1783–1793. doi:10.1130/B35029.1
Lube, G., Cronin, S. J., Platz, T., Freundt, A., Procter, J. N., Henderson, C., et al. (2007). Flow and deposition of pyroclastic granular flows: a type example from the 1975 Ngauruhoe eruption, New Zealand. J. Volcanol. Geoth. Res. 161, 165–186. doi:10.1016/j.jvolgeores.2006.12.003
Lube, G., Breard, E. C. P., Cronin, S. J., Procter, J. N., Brenna, M., Moebis, A., et al. (2014). Dynamics of surges generated by hydrothermal blasts during the 6 August 2012 Te Maari eruption, Mt. Tongariro, New Zealand. J. Volcanol. Geoth. Res. 286, 348–366. doi:10.1016/j.jvolgeores.2014.05.010
Manga, M., Patel, A., and Dufek, J. (2010). Rounding of pumice clasts during transport: field measurements and laboratory studies. Bull. Volcanol. 73, 321–333. doi:10.1007/s00445-010-0411-6
Marzocchi, W., Sandri, L., Gasparini, P., Newhall, C., and Boschi, E. (2004). Quantifying probabilities of volcanic events: the example of volcanic hazard at Mount Vesuvius. J. Geophys. Res. 109, B11201. doi:10.1029/2004jb003155
McPhie, J., Doyle, M., and Allen, R. L. (1993). Volcanic textures: a guide to the interpretation of textures in volcanic rocks. Tasmania, Australia: University of Tasmania, 198.
Mullineaux, D. R., and Crandell, D. R. (1962). Recent lahars from Mount St. Helens, Washington. Geol. Soc. Am. Bull. 73, 855. doi:10.1130/0016-7606(1962)73[855:RLFMSH]2.0.CO;2
Nairn, I. A., and Self, S. (1978). Explosive eruptions and pyroclastic avalanches from Ngauruhoe in February 1975. J. Volcanol. Geoth. Res. 3, 39–60. doi:10.1016/0377-0273(78)90003-3
Nairn, I. A., Wood, C. P., and Hewson, C. A. Y. (1979). Phreatic eruptions of Ruapehu: April 1975. N. Z. J. Geol. Geophys. 22, 155–170. doi:10.1080/00288306.1979.10424215
Nakada, S. (2000). “Hazards from pyroclastic flows and surges,” in Encyclopedia of volcanoes. Editors H. Sigurdsson, B. Houghton, S. McNutt, H. Rymer, and J. Stix (San Diego, CA: Academic Press), 945–995.
Pacheco-Hoyos, J. G., Aguirre-Díaz, G. J., and Dávila-Harris, P. (2018). Boiling-over dense pyroclastic density currents during the formation of the ∼ 100 km3 Huichapan ignimbrite in Central Mexico: stratigraphic and lithofacies analysis. J. Volcanol. Geoth. Res. 349, 268–282. doi:10.1016/j.jvolgeores.2017.11.007
Parsons, W. H. (1969). Criteria for the recognition of volcanic breccias: review. Mem. Geol. Soc. Am. 115, 263–304. doi:10.1130/MEM115-p263
Petcovic, H. L., and Libarkin, J. C. (2007). Research in science education: the expert-novice continuum. J. Geosci. Educ. 55, 333–339. doi:10.1080/10899995.2007.12028060
Platz, T., Cronin, S. J., Cashman, K. V., Stewart, R. B., and Smith, I. E. M. (2007). Transition from effusive to explosive phases in andesite eruptions - a case-study from the AD1655 eruption of Mt. Taranaki, New Zealand. J. Volcanol. Geoth. Res. 161, 15–34. doi:10.1016/j.jvolgeores.2006.11.005
Platz, T., Cronin, S. J., Procter, J. N., Neall, V. E., and Foley, S. F. (2012). Non-explosive, dome-forming eruptions at Mt. Taranaki, New Zealand. Geomorphology 136, 15–30. doi:10.1016/j.geomorph.2011.06.016
Roche, O., Niño, Y., Mangeney, A., Brand, B., Pollock, N., and Valentine, G. A. (2013). Dynamic pore-pressure variations induce substrate erosion by pyroclastic flows. Geology 41, 1107–1110. doi:10.1130/G34668.1
Rosi, M., Paladio-Melosantos, M., Di Muro, A., Leoni, R., and Bacolcol, T. (2001). Fall vs flow activity during the 1991 climactic eruption of Pinatubo Volcano (Philippines). Bull. Volcanol. 62, 549–566. doi:10.1007/s004450000118
Sarocchi, D., Sulpizio, R., Macías, J. L., and Saucedo, R. (2011). The 17 July 1999 block-and-ash flow (BAF) at Colima Volcano: new insights on volcanic granular flows from textural analysis. J. Volcanol. Geoth. Res. 204, 40–56. doi:10.1016/j.jvolgeores.2011.04.013
Saucedo, R., Macías, J. L., Gavilanes, J. C., Arce, J. L., Komorowski, J. C., Gardner, J. E., et al. (2010). Eyewitness, stratigraphy, chemistry, and eruptive dynamics of the 1913 Plinian eruption of Volcán de Colima, México. J. Volcanol. Geoth. Res. 191, 149–166. doi:10.1016/j.jvolgeores.2010.01.011
Saucedo, R., Macías, J. L., Gavilanes-Ruiz, J. C., Bursik, M. I., and Vargas-Gutiérrez, V. (2019). “Pyroclastic density currents at Volcán de Colima,” in Volcán de Colima. Editors N. Varley, C. B. Connor, and J.-C. Komorowski Active volcanoes of the world (Berlin, Heidelberg: Springer Berlin Heidelberg), 111–139. doi:10.1007/978-3-642-25911-1
Saucedo, R., Macías, J. L., and Bursik, M. (2004). Pyroclastic flow deposits of the 1991 eruption of Volcán de Colima, Mexico. Bull. Volcanol. 66, 291–306. doi:10.1007/s00445-003-0311-0
Saucedo, R., Macı́as, J. L., Bursik, M. I., Mora, J. C., Gavilanes, J. C., and Cortes, A. (2002). Emplacement of pyroclastic flows during the 1998–1999 eruption of Volcán de Colima, México. J. Volcanol. Geoth. Res. 117, 129–153. doi:10.1016/S0377-0273(02)00241-X
Sparks, R. S. J., and Walker, G. P. L. (1977). The significance of vitric-enriched air-fall ashes associated with crystal-enriched ignimbrites. J. Volcanol. Geoth. Res. 2, 329–341. doi:10.1016/0377-0273(77)90019-1
Sulpizio, R., De Rosa, R., and Donato, P. (2008). The influence of variable topography on the depositional behaviour of pyroclastic density currents: the examples of the upper Pollara eruption (Salina Island, southern Italy). J. Volcanol. Geoth. Res. 175, 367–385. doi:10.1016/j.jvolgeores.2008.03.018
Sulpizio, R., Dellino, P., Doronzo, D. M., and Sarocchi, D. (2014). Pyroclastic density currents: state of the art and perspectives. J. Volcanol. Geoth. Res. 283, 36–65. doi:10.1016/j.jvolgeores.2014.06.014
Torres-Orozco, R., Cronin, S. J., Pardo, N., and Palmer, A. S. (2018). Volcanic hazard scenarios for multiphase andesitic Plinian eruptions from lithostratigraphy: insights into pyroclastic density current diversity at Mount Taranaki, New Zealand. Bull. Geol. Soc. Am. 130, 1645–1663. doi:10.1130/B31850.1
Uehara, D., Cas, R. A. F., Folkes, C., Takarada, S., Oda, H., and Porreca, M. (2015). Using thermal remanent magnetisation (TRM) to distinguish block and ash flow and debris flow deposits, and to estimate their emplacement temperature: 1991–1995 lava dome eruption at Mt. Unzen Volcano, Japan. J. Volcanol. Geoth. Res. 303, 92–111. doi:10.1016/j.jvolgeores.2015.07.019
Valentine, G. A., and Fisher, R. V. (2000). “Pyroclastic surges and blasts,” in Encyclopedia of volcanoes. Editors H. Sigurdsson, B. Houghton, S. McNutt, H. Rymer, and J. Stix (San Diego, CA: Academic Press), 581–589.
Walker, G. P. L. (1971). Grain-size characteristics of pyroclastic deposits. J. Geol. 79, 696–714. doi:10.1086/627699
Wentworth, C., and Williams, H. (1932). The classification and terminology of the pyroclastic rocks. Bull. Natl. Res. Counc. 89, 19–53.
White, J. D. L., and Houghton, B. F. (2006). Primary volcaniclastic rocks. Geology 34, 677–680. doi:10.1130/G22346.1
White, J. D. L., and Valentine, G. A. (2016). Magmatic versus phreatomagmatic fragmentation: absence of evidence is not evidence of absence. Geosphere 12, 1478–1488. doi:10.1130/GES01337.1
Wilson, C. J. N. (1980). The role of fluidization in the emplacement of pyroclastic flows: an experimental approach. J. Volcanol. Geoth. Res. 8, 231–249. doi:10.1016/0377-0273(80)90106-7
Wilson, C. J. N., and Walker, G. P. L. (1982). Ignimbrite depositional facies: the anatomy of a pyroclastic flow. J. Geol. Soc. 139, 581–592. doi:10.1144/gsjgs.139.5.0581
Witham, C. S. (2005). Volcanic disasters and incidents: a new database. J. Volcanol. Geoth. Res. 148, 191–233. doi:10.1016/j.jvolgeores.2005.04.017
Wright, H. M., and Cashman, K. V. (2014). Compaction and gas loss in welded pyroclastic deposits as revealed by porosity, permeability, and electrical conductivity measurements of the Shevlin Park Tuff. Geol. Soc. Am. Bull. 126, 234–247. doi:10.1130/B30668.1
Yamamoto, T., Takada, A., Ishizuka, Y., Miyaji, N., and Tajima, Y. (2005). Basaltic pyroclastic flows of Fuji volcano, Japan: characteristics of the deposits and their origin. Bull. Volcanol. 67, 622–633. doi:10.1007/s00445-004-0398-y
Keywords: pyroclastic density current, pyroclastic flow, volcaniclastic, deposit, identification, classification, assessment
Citation: Cowlyn J, Kennedy BM, Gravley DM and Cronin SJ (2020) A Confidence-Based Assessment Method for Distinguishing Pyroclastic Density Current Deposits From Other Volcaniclastic Units. Front. Earth Sci. 8:581195. doi: 10.3389/feart.2020.581195
Received: 08 July 2020; Accepted: 22 September 2020;
Published: 30 November 2020.
Edited by:
Roberto Sulpizio, University of Bari Aldo Moro, ItalyReviewed by:
Jose Luis Macias, National Autonomous University of Mexico, MexicoLucia Capra, National Autonomous University of Mexico, Mexico
Copyright © 2020 Cowlyn, Kennedy, Gravley and Cronin. This is an open-access article distributed under the terms of the Creative Commons Attribution License (CC BY). The use, distribution or reproduction in other forums is permitted, provided the original author(s) and the copyright owner(s) are credited and that the original publication in this journal is cited, in accordance with accepted academic practice. No use, distribution or reproduction is permitted which does not comply with these terms.
*Correspondence: James Cowlyn, ai5jb3dseW5AZ21haWwuY29t, Darren Gravley, ZGFycmVuLmdyYXZsZXlAY2FudGVyYnVyeS5hYy5ueg==