- 1Key Laboratory of Geographic Information Science (Ministry of Education of China), East China Normal University, Shanghai, China
- 2School of Geographical Sciences, East China Normal University, Shanghai, China
- 3State Key Laboratory of Frozen Soil Engineering, Northwest Institute of Eco-Environment and Resources, Chinese Academy of Sciences, Lanzhou, China
- 4State Key Laboratory of Soil and Sustainable Agriculture, Institute of Soil Science, Chinese Academy of Sciences, Nanjing, China
- 5State Key Laboratory of Cryospheric Science, Northwest Institute of Eco-Environment and Resources, Chinese Academy of Sciences, Lanzhou, China
- 6China-Pakistan Joint Research Center on Earth Science, CAS-HEC, Islamabad, Pakistan
- 7Department of Physical Geography and Ecosystem Science, Lund University, Lund, Sweden
Permafrost extends 40% of the Qinghai-Tibet Plateau (QTP), a region which contains the headwaters of numerous major rivers in Asia. As an aquiclude, permafrost substantially controls surface runoff and its hydraulic connection with groundwater. The freeze–thaw cycle in the active layer significantly impacts soil water movement direction, velocity, storage capacity, and hydraulic conductivity. Under the accelerating warming on the QTP, permafrost degradation is drastically altering regional and even continental hydrological regimes, attracting the attention of hydrologists, climatologists, ecologists, engineers, and decision-makers. A systematic review of permafrost hydrological processes and modeling on the QTP is still lacking, however, leaving a number of knowledge gaps. In this review, we summarize the current understanding of permafrost hydrological processes and applications of some permafrost hydrological models of varying complexity at different scales on the QTP. We then discuss the current challenges and future opportunities, including observations and data, the understanding of processes, and model realism. The goal of this review is to provide a clear picture of where we are now and to describe future challenges and opportunities. We concluded that more efforts are needed to conduct long-term field measurements, employ more advanced observation technologies, and develop flexible and modular models to deepen our understanding of permafrost hydrological processes and to improve our ability to predict the future responses of permafrost hydrology to climate changes.
Introduction
Permafrost, which is defined as ground that remains at or below 0°C for two or more consecutive years, covers 1.06 × 106 km2, or approximately 40%, of the Qinghai-Tibet Plateau (QTP) (Figure 1; Zou et al., 2017; Zhao et al., 2020). As the source of most major Asian rivers (e.g., the Yangtze, Yellow, Indus, and Mekong; see Figure 1), the QTP encompasses the largest area of high-altitude permafrost and serves as the so-called water tower for nearly 1.4 billion people (Immerzeel et al., 2010). The freeze–thaw cycles of the active layer along with the aquiclude effect of the permafrost layer control the land–surface hydrology on the QTP by altering seasonal soil moisture, water storage, evaporation, and water movement through soil and vegetation (Zhang et al., 2003). The thick layer of underground ice lies near the ground surface (at depths of 1–3 m), has a thickness that ranges from 0.3 to 0.6 m (Cheng, 1983), usually occurs as lenses, and has a total volume of 1.27 × 1013 m3 (Zhao et al., 2019). Both the vertical and lateral soil water fluxes on the QTP are strongly dependent on the soil freezing or thawing depths of the active layer, as well as the extremely low permeability of ground ice.
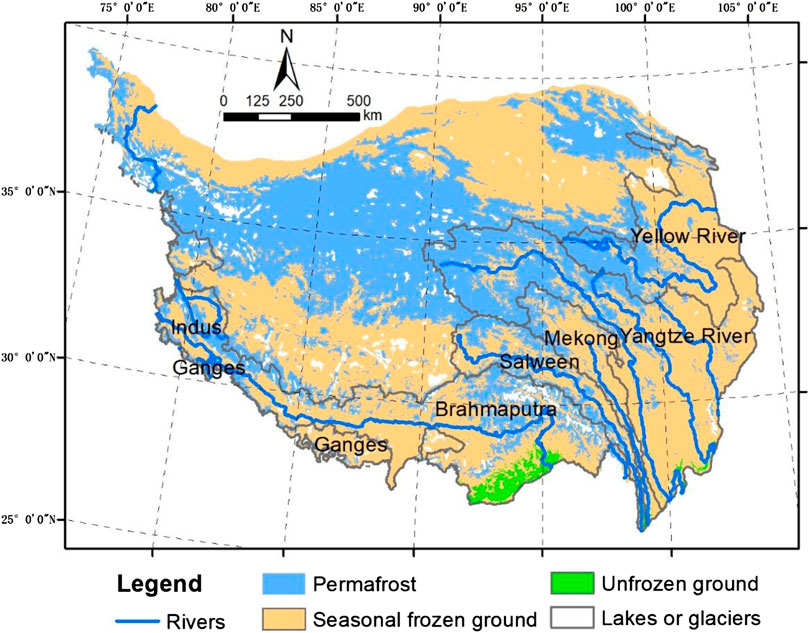
FIGURE 1. Permafrost distribution map of the Qinghai-Tibet Plateau. The permafrost map was adapted from Zou et al. (2017), and the rivers and river basin boundary maps were obtained from the Global Runoff Data Centre (GRDC; data source: https://www.bafg.de/GRDC/EN/Home/homepage_node.html).
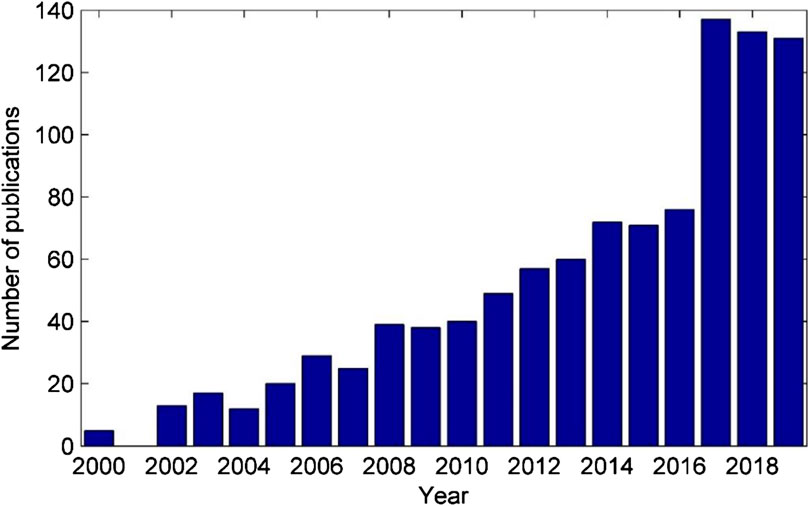
FIGURE 2. Number of publications (2000–2019) listed in the Web of Science database for the terms “permafrost hydrology” or “frozen soil hydrology” or “permafrost water” or “frozen soil water” at “Xizang” or “Tibet” or “Qinghai” or “Heihe” or “Hei River.”
As the Earth’s “third pole,” the QTP has been getting warmer and wetter in recent decades (Chen et al., 2015; Kuang and Jiao, 2016). Air temperatures on the QTP have increased 0.3°C–0.4°C per decade over the past 5 decades, which is twice the global average warming rate (Chen et al., 2015). The most significant warming trends have occurred in the northern QTP, where continuous permafrost is present (Figure 1; Yang et al., 2014). Warming, associated with variations in precipitation and snow cover reduction, has resulted in the thickening of the active layer and permafrost degradation (Cheng and Wu, 2007; Zhao et al., 2019). Determining the contribution of underground ice melting to streamflow is one of the major challenges when evaluating the hydrology regime shift on the QTP under climate change (Zhao et al., 2019). Given permafrost’s role as a major component of the cryosphere, its degradation is significantly altering the cold region hydrology regime (Cheng and Wu, 2007), which could result in a cascade effect on downstream water resources, a phenomenon that has already been reported in Arctic regions (Lafrenière and Lamoureux, 2019).
The interplay of the freeze–thaw process with cold region climatology, ecology, the carbon cycle, biogeochemistry, and infrastructure stability makes permafrost hydrology one of the hot topics of interdisciplinary studies, being of great interest to climatologists, ecologists, engineers, and decision-makers (Cheng et al., 2019). For example, the QTP, as the world’s highest plateau and one that covers a vast area, exerts significant effects on regional-scale water fluxes and energy balance (Ma et al., 2018), and even continental-scale climate (Lu et al., 2018). The spring freeze–thaw on the QTP has a close connection with the Asian summer monsoon, as well as summer precipitation in eastern China (Wang et al., 2020). Permafrost degradation on the QTP releases carbon stored in frozen soil, likely triggering large biogeochemical changes and resulting in positive feedback to global warming (Mu et al., 2019; Wang et al., 2020). Ecosystem resilience is also threatened by permafrost degradation, the thickening of the active layer, drying of surface soil moisture, and the resultant alterations of active layer hydrology (Wang et al., 2006). Catastrophic desertification due to surface water loss occurs as a consequence of ecological degradation. In the eastern and western Tibet Plateau, desert areas have been increasing by approximately 1.8% annually (Yang et al., 2010). For permafrost infrastructure (e.g., buildings, railways, and highways), permafrost degradation results in thermokarst collapse and lake expansion, posing threats to infrastructure stability and security (Yu et al., 2008). Hence, permafrost hydrology studies on the QTP have multidisciplinary interests and broad implications for both scientific research and practical applications.
Despite the vast area of permafrost on the QTP (Cheng and Jin, 2013), our understanding of its hydrology has to some extent lagged behind that of other cold region components, that is, glacier and snow hydrology, which is a significant knowledge gap that must be filled in order to project future water resource variations under climate change (Ding et al., 2020). From a global perspective, permafrost hydrology has been intensively studied in the pan-Arctic, for example, North America and Russia, which are characterized by high-latitude climates and generally low-gradient terrain consisting of plains and wetlands (Woo et al., 2008). The QTP has a limited number of permafrost hydrology in situ observation areas, such as Fenghuo Mountain in the upper Yangtze River region (Wang and Zhang, 2016), the Hulugou watershed in the upper Heihe River Basin (Ma et al., 2017; Chen et al., 2018), and the Shuangchagou watershed in the source area of the Yellow River (Yang et al., 2019). The QTP permafrost has unique characteristics compared with the pan-Arctic. For instance, topographical features, including aspect and elevation, are major factors affecting permafrost distribution on the QTP. Complex mountainous terrain, as a result of neotectonic movement, leads to large spatial heterogeneities of energy and water balance. Moreover, snowfall on the QTP is not as heavy as in the pan-Arctic, since most precipitation on the QTP occurs as rainfall during the summer. Thus, although several review articles have reported the latest research progress in permafrost hydrology (Woo et al., 2008; Walvoord and Kurylyk, 2016), it is still worthwhile to review permafrost hydrology on the QTP due to its high levels of spatial heterogeneity and complexity (Cheng and Wu, 2007). From the Web of Science database, we analyzed the number of publications from 2000 to 2019 containing the terms “permafrost hydrology” or “frozen soil hydrology” or “permafrost water” or “frozen soil water” at “Xizang” or “Tibet” or “Qinghai” or “Heihe” or “Hei River” (Figure 1). The significant increase of publications, especially after the year 2016, demonstrates that permafrost hydrology on the QTP is a rapidly evolving discipline receiving increased attention, as documented by over 1,000 peer-reviewed articles in English.
In this study, we first reviewed the current understanding of permafrost hydrology on the QTP, including the impact of the freeze–thaw process on water movement in soil, rainfall–runoff, baseflow recession, and evaporation, as well as the impact of snow and vegetation on the freeze–thaw process. Subsequently, we reviewed permafrost hydrology modeling studies, including freeze–thaw models, catchment-scale permafrost rainfall–runoff models, model validation, and future projections. We then discussed the challenges and opportunities, including data limitation and advanced observation technology, process comprehension, and model realism testing. In the end, a general summary is provided as a conclusion.
Permafrost Hydrology Processes
Soil Water Movement
Whether in temperate or cold regions, water movement in soil is driven by water potential. The total potential at any point is the sum of the gravitational and matric (or pressure) potentials. In temperate regions, two phases of water movement in the soil porous medium need to be considered, that is, liquid water and vapor. Permafrost regions, however, exhibit more complex processes in both shallow and deep layers.
Unlike common vadose-zone soils, the existence of ice in the active layer alters the porous medium and water potential, resulting in the migration of liquid water and water vapor to the freezing front (Yu et al., 2018). At the beginning of the cold season, freezing starts at the surface and gradually moves downward. Interestingly, during this process, the soil moisture close to the freezing front moves upward, while the soil moisture close to the bottom of the supra-permafrost moves downward. An equilibrium interface (with zero-potential) may exist between the permafrost table and the freezing front. At a particular time, the bottom of the supra-permafrost begins to freeze upwards. This bidirectional freezing process and the large thermal conductivity of the frozen soil result in fast freezing (Jiao et al., 2014). In the warming season, the thawing process starts at the surface. During this process, soil moisture in the active layer moves downward, induced mostly by gravity. The thawing process usually takes four months, however, which is much slower than the freezing process (approximately 25 days) on the QTP. This is likely due to the lower amount of thermal conductivity of liquid water in topsoil, which prevents energy transfer to deep soil, thereby slowing the thawing process (Jiao et al., 2014).
In deep layers, the groundwater is controlled by the permafrost, and can be classified as three different types: supra-permafrost water, intra-permafrost water, and sub-permafrost water (Cheng and Jin, 2013). The supra-permafrost water in the seasonally thawed layer (the active layer) is sensitive to climate, and is thus significantly impacted by precipitation, temperature, snow, and vegetation (Cheng and Jin, 2013). This type of groundwater contributes most of the subsurface storm flow in warm seasons and to some degree regulates the surface runoff. Permafrost, a layer with extremely low permeability due to its low hydraulic conductivity, reduces percolation from surface water to groundwater. Thus, a shallow layer of unconfined groundwater exists in the bottom of the active layer during the thawing season; however, in the freezing season, the downward freezing acts as a water-resistant roof, resulting in a temporal layer of groundwater within the active layer. In the fast-warming permafrost regions, however, this layer might not disappear in the cold season and becomes inter-permafrost groundwater.
Sub-permafrost groundwater is defined as the water under the base of the permafrost layer (Cheng and Jin, 2013). This type of groundwater can be recharged by supra-permafrost water and surface water via non-permafrost conduits, and contributes baseflow recession or surface springs. Typically, widespread taliks—bodies or layers of unfrozen ground in permafrost regions—could form conduits when underlying permafrost disappears. Consequently, hydrogeological conditions, including groundwater flow directions, velocity, conductivity, and pathways, would be altered substantially. Particularly with climate warming, the expansion of the talik area and depth is likely increasing the hydrological connectivity between surface water (e.g., lakes and rivers) and groundwater, consequently shifting basin-scale hydrological regimes (Yang et al., 2019; O’Neill et al., 2020). Interestingly, after being “completely” frozen, unfrozen water still exists as intra-permafrost (ground ice) water, whose movement is mainly driven by temperature gradients (Cheng, 1983; Chang et al., 2015). There is a huge body of research concerning this unfrozen water because the water movement causes frost heaves, which are a significant challenge for infrastructure stability in permafrost regions (e.g., highways, railways, and pipelines). An understanding of intra-permafrost water in terms of hydrology is still generally lacking, however, indicated by the large uncertainty when estimating the release of permafrost and ground ice water to streamflow. In general, permafrost degradation can strongly influence water movement direction, velocity, storage capacity, and hydraulic conductivity.
Rainfall Runoff
Rainfall runoff is a key issue in hydrology, as it affects most permafrost regions on the QTP (Wang and Zhang, 2016). Permafrost rainfall–runoff is greatly influenced by the freeze–thaw cycle in the active, intra-permafrost, and sub-permafrost layers. The aquiclude effect of frozen soil reduces surface infiltration, increases soil moisture, and enhances surface runoff generation (Woo et al., 2008). It is well documented that permafrost increases peak flow, reduces recharge to groundwater, and amplifies streamflow seasonal variability (Ye et al., 2009).
Runoff generation in the permafrost region is controlled by multiple factors on the QTP, including soil temperature, thaw depth, precipitation frequency and amount, and antecedent soil moisture (Gao T. G. et al., 2018). The impacts of permafrost on runoff exhibit strong seasonality and are closely connected to soil temperature. It was found that the runoff coefficient is larger in spring and autumn and smaller in summer (Wang et al., 2009). This is very likely due to the seasonal variation of the thawed active layer, which has larger storage capacity and less runoff in summer and smaller storage capacity in the spring and autumn. It is interesting that permafrost hydrology only differs from that of temperate regions during the frozen season, when both precipitation and runoff are very limited on the QTP (Wang and Zhang, 2016). During the summer monsoon period, however, when dominant runoff occurs, there is no obvious difference between permafrost rainfall–runoff and that in temperate regions, given the completely thawed active layer (Wang and Zhang, 2016). The Fenghuo Mountain observatory revealed that 55–60 cm is the likely threshold thawing depth. Above this threshold depth, the thawing depth exerts a negligible impact on runoff (Wang and Zhang, 2016).
Baseflow Process
Since most rainfall–runoff processes occur beneath the surface, they generally cannot be directly measured. Hydrography itself provides a valuable and integrated signal for hydrological processes on the catchment scale. An increase of winter flow has been reported in a large number of cold regions (Liu et al., 2007). Recession flow analysis has been widely used to grasp the hydrological impacts of permafrost degradation and to derive the thawing rate of permafrost in cold regions (Lyon et al., 2009; Qin et al., 2020). Ye et al. (2009) proposed the Qmax/Qmin ratio (maximum monthly runoff divided by minimum monthly runoff) as an indicator to quantify the impact of permafrost on hydrography recession. This index has been widely used in QTP permafrost hydrology analysis, including in the headwaters of the Yellow River (Wu P. et al., 2020) and across the QTP (Liu et al., 2020; Song et al., 2020). These researchers found that there was a significant positive relationship between the Qmax/Qmin ratio and basin permafrost coverage. Most studies have found that under the influence of climate change on the QTP, Qmax/Qmin has decreased due to the decreased Qmax during the thawing summer season caused by the increase of infiltration and percolation, while the Qmin in winter has increased due to the increased groundwater recession. In other words, thawing permafrost leads to more water being routed through subsurface pathways, decreased peak flow, and increased groundwater recession. Interestingly, although an obvious basin-scale hydrological regime shift has been detected, there has been no direct evidence at the local scale reporting that the increase of taliks in permafrost have formed and enhanced the recharge to groundwater and baseflow. More intriguingly, a long-term study on the northern QTP revealed that the thawing permafrost has reduced winter runoff, for example, in the Three-River Headwaters Region and the upper Heihe River (Gao et al., 2016). The increase of deep percolation in these discontinuous permafrost regions (e.g., the upper Heihe River) could explain the decreasing trend of winter baseflow (Gao et al., 2016). In summary, hydrography recession provides a powerful indicator for understanding the impacts of permafrost on basin-scale hydrology, although the conflicting observations in different regions and scales still require further investigation.
Evaporation
Evaporation, including canopy interception, soil evaporation, water evaporation, and vegetation transpiration, is an important component in catchment water balance. Despite its importance, evaporation has not been as thoroughly explored as other hydrological processes on the QTP. The lysimeter provides accurate plot-scale evaporation data, while advanced micro-climatology observation, for example, eddy covariance, provides state-of-the-art measurements of evaporation on the field scale, and remote sensing can reveal the spatial patterns of evaporation distribution. In catchment hydrology, however, it is still common for evaporation to be derived simply from the water balance, as the residual of precipitation, runoff, and the delta of storage. The energy balance–based remote sensing approach provides an important alternative for estimating the spatial distribution and temporal variation of evaporation, although still with large uncertainty (Chen et al., 2019).
Generally, the existence of permafrost reduces the long-term average evaporation. Yang et al. (2003) discovered that freezing prevented soil moisture from evaporating on the plot scale. On the catchment scale, it was found that the runoff coefficient in permafrost regions was larger than in other regions due to the limited evaporation and larger amount of precipitation (Kang et al., 2002; Wang et al., 2009). Interestingly, when we investigated evaporation at finer temporal scales (e.g., daily), the impact of permafrost became more complex. It was found that at the beginning of the melting season, the shallow active layer leads to large supra-permafrost soil moisture, a shallow saturation zone, and a rapid increase in evaporation. This evaporation was reduced when the saturated zone moved downward due to the deepening of the active layer. A decrease of evaporation in August and September was found in the continuous permafrost region, with short-term variations coupled to precipitation events (Zhang et al., 2003; Wu et al., 2011; Wang G. X. et al., 2020). For climate change, Wang et al. (2020) proposed a new method to represent energy consumption due to ice phase changes and improved the nonlinear complementary relationship. They discovered that reductions in permafrost under a warming climate likely lead to accelerating evaporation over the QTP. Evaporation studies on the QTP remain one of the bottlenecks in hydrology and land surface research and still require more advanced measuring and modeling efforts.
Impacts of Snow and Vegetation on the Freeze–Thaw Process
Snow conditions have a significant impact on the development of soil frost. Snow cover with high albedo affects the ground thermal regime, reducing both incoming solar radiation and ground temperature. Snow as an isolation layer also prevents heat transfer between the atmosphere and soil. Thus, in winter, thick snow cover increases the soil surface temperature and reduces the depth of frozen soil. Paired-plot experiments have demonstrated that when snow cover is removed, soil freezing is deeper than in the undisturbed snow cover plot (Iwata et al., 2010; Chang et al., 2014). On the QTP, however, when the snow depth is greater than 15 cm, snow-induced thermal insulation is very limited (Zhao et al., 2018). The overall impact of snow cover on permafrost hydrology depends on the timing, duration, accumulation, and melting of snow cover (Zhou et al., 2013). There are more characteristics that influence the snow cover–permafrost relationship, such as topography, vegetation cover, and micrometeorological conditions (Zhang, 2005).
As with snow cover, vegetation helps to preserve the underlying permafrost and impacts the depth of the active layer, thereby affecting hydrology (Wang et al., 2006; Luo et al., 2018). It has been found that in well-vegetated areas, permafrost degradation was less than in locations with little vegetation (Li et al., 2015). Areas with little vegetation cover exhibited greater annual variability of soil temperature and were likely to be more sensitive to changes in air temperature. Low vegetation cover was also linked to higher thermal diffusivity and thermal conductivity in the soil. The maintenance of dense vegetation cover on alpine meadows was found to reduce the impact of seasonal heat cycling on the permafrost, possibly minimize the impact of climate change, and help to preserve the microenvironment of the soil (Wang G. X. et al., 2010). Additionally, the organic layer on top of the soil as well as the peat layer within the soil act as isolation layers that prevent heat transfer (Yi et al., 2007; Zhou et al., 2013).
Permafrost Hydrological Models
There has been a revival in the development of permafrost hydrological models simulating coupled heat and water transfer (Walvoord and Kurylyk, 2016). Permafrost hydrology models are more complicated than models of temperate climate regions in terms of at least three aspects: 1) the very special solid–liquid–vapor relationship, including energy balance and phase transform, which cannot be modeled by traditional hydrology methods; 2) mountainous regions of the QTP have complex terrain and microclimates, causing significant spatial heterogeneity of almost all hydrological factors; and 3) hydrological factors in cold mountains are impacted by energy, with different phases of precipitation, radiation, and terrain shadowing. All of these factors exhibit complex daily and diurnal variations, and require detailed spatial and temporal measurements in order to sufficiently represent/capture this large variability. A key factor for water fluxes and heat transport in permafrost regions is that water undergoes a phase transition between solid ice and liquid water as the subsurface temperature fluctuates around the freezing point seasonally (Ge et al., 2011).
Freeze–Thaw Models
Physically based freeze–thaw models are based on energy and mass balance as well as heat transfer equations. Although most freeze–thaw models have governing equations that are quite similar, they differ in terms of simplification due to assumptions, numerical discretization, and diagnostic variables (Walvoord and Kurylyk, 2016). Numerous models of varying complexity have been developed to simulate freeze–thaw processes on the QTP (Table 1). For example, the Simultaneous Transfer of Energy Momentum and Mass in Unsaturated Soil (STEMMUS) land surface model was coupled with a freeze–thaw process, a combination known as the STEMMUS-FT, by incorporating unfrozen water content, hydraulic conductivity, and heat capacity/conductivity (Yu et al., 2018). This research has revealed that during the freeze–thaw process, not only is liquid water transferred to the freezing front but also vapor fluxes. Xiao et al. (2013) improved the Common Land Model (CoLM) by refining the permafrost algorithm and achieved an improved simulation for reproducing soil liquid water content at the Tanggula station. Yang K. et al. (2013) compared the performances of the simultaneous heat and water (SHAW) model and the coupled heat and mass transfer model (CoupModel) in simulating the transfer processes of energy and water at the Hulugou study site. They found that both models were better at simulating soil temperature than soil water content. It is worth noting that most numerical models are computationally expensive and scale dependent due to the large spatial heterogeneity of the study field.
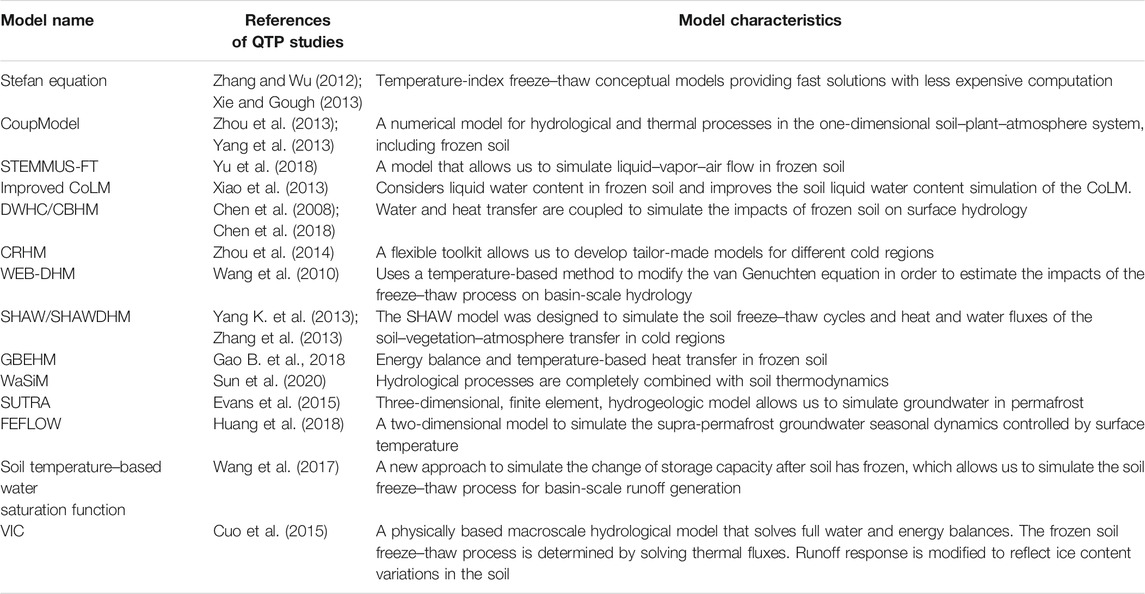
TABLE 1. A selection of hydrological models, relevant references, model characteristics, and key findings in relevant references.
In addition to physically based models, temperature-index freeze–thaw conceptual models provide fast solutions with costing less computation. The Stefan equation (Xie and Gough, 2013), for example, uses the ground surface thawing (freezing) index (°C) to estimate the depth from the ground surface to the thawing (freezing) front. Zhang and Wu (2012) utilized the Stefan equation to project the change of the active layer thickness on the QTP. It is worth noting that both heat transfer models and temperature-index models are mostly 1-D vertical simulations. Meanwhile, the importance of 1-D vertical flux in catchment-scale hydrology is still not clear. Most if not all models are still too simple to describe the complex phase transition of water for the heterogeneous soil, vegetation, roots, and topographical conditions on the QTP. Thus, it is not surprising that it is still highly uncertain if involving the freeze–thaw process will increase model performance at the catchment scale, which will be discussed in Permafrost Surface Runoff Models at the Catchment Scale.
Permafrost Surface Runoff Models at the Catchment Scale
Most permafrost runoff modeling studies on the QTP have been based on existing catchment hydrological models coupled with different freeze–thaw modules. The freeze–thaw modules were integrated into existing physically based hydrological models by adjusting the calculations of three sets of parameters/variables: unfrozen water content, hydraulic conductivity, and heat capacity/conductivity. Chen et al. (2008), for example, designed a distributed water-heat coupled (DWHC) model and employed a simple numerical solution to the continuous water and heat equation. The DWHC model was tested in the upper Heihe River Basin. Based on the DWHC, Chen et al. (2018) further included glaciers, snow cover, and heat transfer of soil, extended their simulation to a distributed cryospheric basin hydrological model (CBHM), and used it to evaluate the effects of cryospheric changes on streamflow in the upper Heihe River Basin. Wang et al. (2010) developed a distributed hydrological model, that is, the energy budget–based distributed hydrological model (WEB-DHM), which incorporated frozen soil processes. Zhang et al. (2013) coupled the one-dimensional SHAW model with a geomorphologically based distributed hydrological model (GBHM) (Yang et al., 1998) to create the SHAWDHM model. Gao et al. (2018) extended the GBHM with cryosphere hydrological processes, developed the distributed geomorphology-based eco-hydrological model (GBEHM), and tested it in the upper Heihe River region. Zhou J. et al. (2014) employed a modular platform—the Cold Regions Hydrological Model (CRHM) (Pomeroy et al., 2007)—as a flexible toolkit to develop tailor-made models for a snowmelt-dominated high alpine basin and a soil freeze–thaw–dominated steppe basin. They found that the uncalibrated CRHM demonstrated encouraging accuracy when reproducing cold region hydrological processes, indicating its potential capability for prediction in ungauged cold basins. Sun et al. (2020) used the water balance simulation model (WaSiM) to simulate the hydrological responses to permafrost degradation in the headwaters of the Yellow River (HWYR) in High Asia and found that permafrost degradation decreased autumn runoff and increased winter/spring runoff. Cuo et al. (2015) employed the variable infiltration capacity (VIC) model with a frozen soil model to simulate soil moisture, soil temperature, soil ice content, evaporation, and runoff at 13 permafrost sites on the QTP. They discovered that precipitation plays a dominant role in surface hydrology enhancement, while frozen soil degradation is the secondary factor.
Only a few studies have been designed to couple the freeze–thaw process with existing conceptual hydrological models. Wang G. X. et al. (2017), for instance, proposed a new approach that introduced a soil temperature-based water saturation function and modified the soil water storage curve with a soil temperature threshold. By changing the water saturation function, the new model allowed us to simulate the storage capacity change after the soil had frozen, as well as the decrease of groundwater discharge caused by active layer freezing.
It is worth noting that most permafrost hydrology modeling studies on the QTP have made the a priori assumption that permafrost exerts a clear and significant effect on runoff. It is still a prevailing practice to harness a ready-to-use model, and most research efforts have been spent on forcing data preparation and parameter calibration. Intriguingly, a strong influence of permafrost on basin-scale hydrology has not always been verified in other cold regions (Shanley and Chalmers, 1999; Lindstrom et al., 2002). Conflicting conclusions obtained by modeling studies suggest that there is a need for more hypothesis-testing modeling investigations in order to accept or reject the influence of permafrost on hydrological processes at diverse scales on the QTP. Moreover, most modeling studies have been focused on 1D vertical fluxes and solving water-energy balance differential equations, while neglecting horizontal landscape heterogeneities and mountainous terrain, both of which are important factors connecting point-scale permafrost observations and basin-scale hydrograph data.
Permafrost Groundwater Models
The effects of the seasonal freeze–thaw and subsurface hydrogeological structures on groundwater recharge and discharge are critical factors in permafrost hydrology. Permafrost groundwater models provide powerful tools to quantitatively describe subsurface hydrological processes and predict future warming based on groundwater processes. Evans et al. (2015) used a three-dimensional, finite element, hydrogeological model (the saturated-unsaturated transport model, i.e., SUTRA) to evaluate the effects of climate change on groundwater movement in permafrost on the QTP. The SUTRA model simulations indicated that groundwater provides significant contributions to streams in the form of baseflow and that the majority of groundwater flow is from the shallow aquifer above the permafrost. Huang et al. (2019) used the FEFLOW hydrogeological software program to simulate supra-permafrost groundwater discharge in different land surface temperature conditions on the central QTP. They found that topography, particularly aspect, plays an essential role in temperature, permeability, and groundwater discharge.
It is worth noting that most modeling studies have still focused on the freeze–thaw process, which has an important impact on plot-scale infiltration and groundwater recharge. Additional processes, however, for example, the thawing of underground ice, open taliks, and hydraulic connectivity, are also closely linked with permafrost runoff, but have not yet been given sufficient attention in modeling. The complex hydrogeological structures in permafrost regions pose great challenges for model development. The complexities go beyond the heterogeneous soil texture, plant roots, animal caves and wormholes, depth to rock, and permeability of bedrock, which widely exist in temperate climate regions. Permafrost hydrogeology has its own specific characteristics, for example, the complex geological characteristics of supra-permafrost, intra-permafrost, sub-permafrost, and ground ice, as well as their complex dynamics and interactions. The complex boundary conditions of the permafrost groundwater system include the water and energy budget, the impacts of snow and vegetation in the upper boundary, and the depth to rock and permeability of bedrock in the lower boundary, all of which make permafrost groundwater modeling substantially more complex than in other regions. Although a few perceptual models have been proposed to describe the permafrost-related groundwater system (Hu et al., 2019; Yang et al., 2019), physically based numerical models that can quantify these complex groundwater systems are still generally lacking.
Model Validation and Future Projection
Multivariable validation is important when testing model realism for permafrost hydrology. Variables, such as hydrograph measurements, frozen soil depth, soil temperature, snow cover, and streamflow, can all be used to validate model performance. Hydrograph information is the most widely used variable to validate catchment-scale hydrology, while soil temperature, soil water content, and frozen soil depth have generally been the variables chosen for plot-scale validation (Chen, 2008; Zhang et al., 2013; Evans et al., 2018; Yu et al., 2018; Sun et al., 2020). Zeng et al. (2016) blended satellite observations, model simulations, and in situ measurements to quantify soil moisture on the QTP. They discovered that there was a need to constrain the model-simulated soil moisture estimates with the in situ measurements prior to their further applications in scaling soil moisture satellite products. Chen et al. (2017) coupled observed snow maps to calibrate and validate a cold region hydrological model. They demonstrated the trade-off effect of snow cover area and snow water equivalent in the upper Brahmaputra River Basin. We believe that model validation with multi-source data at multiple scales is important for testing model realism.
Although it is widely recognized that permafrost degradation will result in hydrological changes on the QTP (Ge et al., 2011; Kong and Wang, 2017; Wang et al., 2018), the future relationships between permafrost degradation and the changes of streams, rivers, and lakes are still being debated. The lack of understanding concerning the mechanism behind permafrost degradation, as well as models to simulate it, remains the biggest obstacle for future projection. Take the estimation of underground ice melting as an example. The melting of ground ice increases recharge from surface water to groundwater, while the melting of ground ice itself is an important source of water that contributes to surface runoff (Yang et al., 2019). The amount of water released from ground ice is still largely uncertain in future projections, however, due to the lack of mechanism comprehension and currently available models.
Challenges and Opportunities
Much fundamental progress has recently been made in the understanding of hydrological processes and the modeling of permafrost hydrology on the QTP (Cheng and Jin, 2013). Critical limitations in hydrologic data coverage, subsurface characterization, process-level understanding, and integrated modeling approaches still exist, however. These challenges also pose great opportunities for further exploration of permafrost hydrological processes.
Data Limitation and Advanced Observation Technology
A lack of data and inferior data quality remain two of the biggest challenges for QTP permafrost hydrology. The QTP covers a vast land area of 2.5 million km2, but is very sparsely populated and has a limited measurement network (Yang et al., 2014). The QTP has an extremely high elevation, features a harsh climate, and lacks adequate oxygen, all of which together result in severe logistical hurdles. The formidable and even dangerous accessibility makes studying permafrost hydrology here more difficult than in other regions, resulting in poor data quality and frequent data gaps (Woo et al., 2008). Although a few local-scale long-term observations provide reliable first-hand datasets, the data issue in the permafrost region cannot be overcome in a short period of time. Tackling the data issue requires constant financial support, the dedication and enthusiasm of scientists, and the continuous investment of time and effort by researchers.
Remote sensing is a promising technique for monitoring large-scale permafrost hydrology and the land surface energy budget at various spatial and temporal resolutions in an economical way. Remote sensing techniques, including optical-thermal wavelength, passive microwave, and active microwave, have been widely implemented to fill in the data gaps on the QTP. Numerous hydrological components can be monitored by satellite remote sensing, including precipitation (Sun et al., 2018), soil moisture (Zheng et al., 2017), vegetation (Liu et al., 2017), topography and snow cover area (Che et al., 2014; Huang et al., 2017; Han et al., 2019; You et al., 2020), glacier mass balance (Brun et al., 2017), river width and water depth (Huang et al., 2018), lake variations (Wu and Zhu, 2008; Zhang et al., 2017), river flow (Huang et al., 2020), and storage variations (Xiang et al., 2016). Microwave remote sensing, for example, synthetic aperture radar, provides information on the timing, duration, and regional progression of the near-surface soil freeze–thaw status (Zhang et al., 2009). Unlike other cryosphere components, however, many permafrost hydrological processes have subsurface features, which cannot be directly detected by remote sensing.
Geophysical detection technology allows us to measure various permafrost features at plot/field scales. For example, liquid water in frozen soil can be measured by time-domain reflectometry (TDR) and ground-penetrating radar can detect the depths of the active layer and permafrost ice layer (Wu et al., 2005; Pan et al., 2017). Gamma-ray attenuation (GRA) provides useful information for determining total water content. Combining TDR and GRA allows for the simultaneous measurement of liquid and total water content in frozen soil, and the subsequent derivation of the ice content (Zhou et al., 2014). Electromagnetic induction, electrical resistivity tomography, and seismic refraction have all shown promise for capturing the active layer thickness with good spatial coverage. These geological detection technologies have the potential to improve our understanding of permafrost degradation in climate change, evaluating the amount of melting water from permafrost, and inferring subsurface hydrological connectivity.
Water isotope and geochemical data provide important integrated and orthogonal information for catchment-scale hydrology (Liu et al., 2013). Isotope fractionation is associated with water phase transition, which provides essential auxiliary data for understanding the origin and fate of the water cycle (Kong et al., 2019). For hydrological processes, water-stable isotope data allow us to estimate the source, storage, pathway, and age of different water bodies. Wan et al. (2019) used water-stable isotope data (2H, 18O) to assess the thermokarst lake water balance in the headwaters of the Yellow River and proposed a perceptual model to illustrate the evolution of thermokarst lakes under permafrost degradation. Water-stable isotope studies have revealed that, as with unfrozen catchments, pre-event “old” water also plays an important role in runoff generation (Zhou et al., 2015; Ma et al., 2017), challenging the long-held belief that permafrost is an aquiclude layer. Yang et al. (2019), however, used isotope data from the headwaters of the Yellow River and drew the conclusion that rainfall (“new” water) was the most important source of streamflow and permafrost melting was another important source of streamflow. In the future, we expect to further explore isotope data in order to quantify additional processes, including evaporation, snow sublimation, snow melting, and mixing among water bodies.
Overall, remote sensing technology has advantages when exploring land surface processes and hydrology at the regional scale. Geophysical investigations definitely have the potential to identify the subsurface hydrological processes and groundwater conditions. Last, isotopic tracers exhibit great strength in illuminating runoff generation, process-based mechanism interpretation, and quantifying the study of hydrograph separation. Combining and integrating the various technologies and methods is suggested in order to further enhance our understanding of permafrost hydrological processes at diverse scales.
Improving Process Understanding and Model Realism
Field studies on the QTP continue to catalog and characterize mounting complexities and heterogeneities of permafrost hydrological processes at various scales. This is highly beneficial for accumulating first-hand data and documenting facts in order to enhance our understanding of small-scale processes. Based on field observations and experiments, many descriptive perceptual models have been proposed to describe these spatial and temporal heterogeneities. Compared with the enormous field heterogeneities, however, most modeling studies on QTP permafrost are still too parsimonious in terms of process representation, although many models utilize complex numerical solutions with expensive computations to calculate heat transfer and water movement. Most of these modeling practices could be categorized as bottom-up frameworks, meaning that the modelers assumed that small-scale physics were also appropriate at the modeling catchment scale (Sivapalan et al., 2003; McDonnell et al., 2007). Top-down modeling practices (Shanley and Chalmers, 1999; Lindstrom et al., 2002), however, found negligible influences of the freeze–thaw process on runoff at the basin scale. These seemingly counterintuitive results illustrate that permafrost likely displays a kind of self-organized pattern at the basin scale, which allows us to create simulations using surprisingly simple models. Alternatively, there are probably more dominant processes that determine permafrost hydrology than the topsoil freeze–thaw process.
Water-stable isotope data have provided valuable insights into how water and solutes are partitioned and stored, as well as their pathways, knowledge that significantly benefits model validation (Lindstrom et al., 2002; Kirchner, 2006). Ala-aho et al. (2017) extended the Spatially distributed Tracer-Aided Rainfall–Runoff (STARR) model to snow-influenced catchments and coupled the isotope fraction of snow sublimation and melting. Smith et al. (2019) adapted a tracer-aided ecohydrological model, the EcH2O-iso, for cold regions with the explicit conceptualization of dynamic soil freeze–thaw processes. These modeling frameworks have the potential to be used in QTP studies. Incorporating these new data requires us to construct more flexible and modular frameworks, which will allow us to develop tailor-made models based on specific basin characteristics, rather than using a single fixed model to fit all basins by simply calibrating parameters (Fenicia et al., 2011; Zhou et al., 2013).
Hydrology modeling is not only a science but also an art that requires modelers’ creativity, inspiration, and ingenuity, as well as their experience and skill (Savenije, 2009). All models are simplifications of the real world. Hence, it is unrealistic and unnecessary to include all heterogeneous processes observed in the field in permafrost hydrology models. The appropriate level of simplification is the key for achieving model realism, which is also scale and objective dependent. In many cases, distinguishing which processes should be involved and which should be disregarded is more important than the precision of solving numerical equations. Stepwise modeling and top-down flexible modeling frameworks allow us to develop models with a particular level of complexity in order to simulate hydrological processes, while simultaneously avoiding over-parameterization (Sivapalan et al., 2003; Gao et al., 2014a; Gao et al., 2014b). The concept of “hydrological connectivity” might be beneficial for linking local-scale observations and catchment-scale modeling, as Woo et al. (2008) suggested, and for implementation in numerous temperate basins (Gao et al., 2019).
Summary
We conclude that 1) permafrost hydrology studies on the QTP are attracting broad interest, including that of hydrologists, ecologists, climatologists, and water resource managers; 2) the number of publications on QTP permafrost hydrology is mounting. Several in situ observation stations have been established to gather long-term measurements. Given the vast area of the QTP, however, permafrost hydrology research in this region remains far from adequate; 3) permafrost comprehensively impacts all hydrological processes on the QTP, including soil water movement, rainfall–runoff, baseflow, and evaporation; 4) permafrost hydrological modeling is a powerful tool for quantifying the spatiotemporal variations of QTP hydrological processes, and for projecting future changes. It still remains worthwhile, however, to test the importance of permafrost on basin-scale hydrology, and to incorporate landscape and topographical data in order to sufficiently represent horizontal heterogeneities; 5) data limitation will continue to exist for a long time, although in situ measurements, remote sensing data, isotope data, and geological detection methods are quickly improving; and 6) determining the balance point between model simplicity and catchment complexity is likely one of the most important scientific challenges yet to be solved in permafrost hydrological modeling on the QTP. Overall, the systematic and quantitative understanding of permafrost hydrology will benefit water resource management, flood mitigation, nature conservation, and adaption to climate change on the QTP and in its vast downstream regions.
Author Contributions
HG, YD, and YY conceived the central idea; HG and YY wrote the initial draft of the manuscript; and all authors edited the manuscript and agreed to its submission.
Funding
This study was supported by the National Natural Science Foundation of China (Grant Nos. 42071081, 41801036, 4181101490, and 41771262).
Conflict of Interest
The authors declare that the research was conducted in the absence of any commercial or financial relationships that could be construed as a potential conflict of interest.
Acknowledgments
We thank Professor Genxu Wang for his constructive comments and discussions during the development of this manuscript. Any errors in this article are the sole responsibility of the authors. A special acknowledgement should be expressed to China-Pakistan Joint Research Center on Earth Sciences that supported the implementation of this study.
References
Ala-Aho, P., Tetzlaff, D., Mcnamara, J. P., Laudon, H., and Soulsby, C. (2017). Using isotopes to constrain waterflux and age estimates in snow-influenced catchments using the STARR (Spatially distributed Tracer-Aided Rainfall-Runoff) model. Hydrol. Earth Syst. Sci. 21, 5089–5110. doi:10.5194/hess-2017-106
Brun, F., Berthier, E., Wagnon, P., Kaab, A., and Treichler, D. (2017). A spatially resolved estimate of High Mountain Asia glacier mass balances from 2000 to 2016. Nat. Geosci. 10, 668–673. doi:10.1038/NGEO2999
Chang, J., Wang, G. X., Gao, Y. H., and Wang, Y. B. (2014). The influence of seasonal snow on soil thermal and water dynamics under different vegetation covers in a permafrost region. J. Mt. Sci. 11, 727–745. doi:10.1007/s11629-013-2893-0
Chang, J., Wang, G. X., Li, C. J., and Mao, T. X. (2015). Seasonal dynamics of suprapermafrost groundwater and its response to the freeing-thawing processes of soil in the permafrost region of Qinghai-Tibet Plateau. Sci. China Earth Sci. 58, 727–738. doi:10.1007/s11430-014-5009-y
Che, T., Li, X., Jin, R., and Huang, C. L. (2014). Assimilating passive microwave remote sensing data into a land surface model to improve the estimation of snow depth. Remote Sens. Environ. 143, 54–63. doi:10.1016/j.rse.2013.12.009
Chen, D. L., Xu, B. Q., Yao, T. D., Guo, Z. T., Cui, P., Chen, F. H., et al. (2015). Assessment of past, present and future environmental changes on the Tibetan Plateau. Chin. Sci. Bull. 60, 3025–3035. doi:10.1360/N972014-01370
Chen, R., Lu, S., Kang, E., Ji, X., Zhang, Z., Yang, Y., et al. (2008). A distributed water-heat coupled model for mountainous watershed of an inland river basin of Northwest China (I) model structure and equations. Env. Geol. 53 (6), 1299–1309.
Chen, R., Wang, G., Yang, Y., Liu, J., Han, C., Song, Y., et al. (2018). Effects of cryospheric change on alpine hydrology: combining a model with observations in the upper reaches of the Hei River, China. J. Geophys. Res. Atmos. 123, 3414–3442. doi:10.1002/2017JD027876
Chen, X., Long, D., Hong, Y., Zeng, C., and Yan, D. H. (2017). Improved modeling of snow and glacier melting by a progressive two-stage calibration strategy with GRACE and multisource data: how snow and glacier meltwater contributes to the runoff of the Upper Brahmaputra River basin? Water Resour. Res. 53, 2431–2466. doi:10.1002/2016WR019656
Chen, X., Massman, W. J., and Su, Z. (2019). A column canopy‐air turbulent diffusion method for different canopy structures. J. Geophys. Res. Atmos. 124, 488–506. doi:10.1029/2018JD028883
Cheng, G., and Wu, T. (2007). Responses of permafrost to climate change and their environmental significance, Qinghai‐Tibet Plateau. J. Geophys. Res. 112, F02S03. doi:10.1029/2006JF000631
Cheng, G. D., Zhao, L., Li, R., Wu, X. D., Sheng, Y., Hu, G., et al. (2019). Characteristic, changes and impacts of permafrost on Qinghai-Tibet Plateau (in Chinese). Chin. Sci. Bull. 64, 2783–2795. doi:10.1360/TB-2019-0191
Cheng, G. D., and Jin, H. J. (2013). Groundwater in the permafrost regions on the Qinghai-Tibet Plateau and it changes. Hydrogeol. Eng. Geol. 40, 1–11.
Cheng, G. D. (1983). The mechanism of repeated-segregation for the formation of thick layered ground ice. Cold Reg. Sci. Technol. 8, 57–66.
Cuo, L., Zhang, Y. X., Bohn, T. J., Zhao, L., Li, J. L., Liu, Q. M., et al. (2015). Frozen soil degradation and its effects on surface hydrology in the northern Tibetan Plateau. J. Geophys. Res. Atmosp. 120, 8276–8298. doi:10.1002/2015JD023193
Ding, Y. J., Zhang, S. Q., and Chen, R. S. (2020). Cryospheric hydrology: decode the largest freshwater reservoir on Earth. Bull. Chin. Acad. Sci. 35, 414–424.
Evans, S. G., Ge, S., Voss, C. I., and Molotch, N. P. (2018). The role of frozen soil in groundwater discharge predictions for warming alpine watersheds. Water Resour. Res. 54, 1599–1615. doi:10.1002/2017WR022098
Evans, S. G., Ge, S. M., and Liang, S. H. (2015). Analysis of groundwater flow in mountainous, headwater catchments with permafrost. Water Resour. Res. 51, 9564–9576. doi:10.1002/2015WR017732
Fenicia, F., Kavetski, D., and Savenije, H. H. G. (2011). Elements of a flexible approach for conceptual hydrological modeling: 1. Motivation and theoretical development. Water Resour. Res. 47. doi:10.1029/2010WR010174
Gao, B., Yang, D. W., Qin, Y., Wang, Y. H., Li, H. Y., Zhang, Y. L., et al. (2018). Change in frozen soils and its effect on regional hydrology, upper Heihe basin, northeastern Qinghai-Tibetan Plateau. Cryosphere. 12, 657–673. doi:10.5194/tc-2016-289
Gao, H., Hrachowitz, M., Fenicia, F., Gharari, S., and Savenije, H. H. G. (2014a). Testing the realism of a topography-driven model (FLEX-Topo) in the nested catchments of the Upper Heihe, China. Hydrol. Earth Syst. Sci. 18, 1895–1915. doi:10.5194/hess-18-1895-2014
Gao, H., Hrachowitz, M., Schymanski, S. J., Fenicia, F., Sriwongsitanon, N., and Savenije, H. H. G. (2014b). Climate controls how ecosystems size the root zone storage capacity at catchment scale. Geophys. Res. Lett. 41, 7916–7923. doi:10.1002/2014GL061668
Gao, H. K., Birkel, C., Hrachowitz, M., Tetzlaff, D., Soulsby, C., and Savenije, H. H. G. (2019). A simple topography-driven and calibration-free runoff generation module. Hydrol. Earth Syst. Sci. 23, 787–809. doi:10.5194/hess-23-787-2019
Gao, T. G., Zhang, T. J., Cao, L., Kang, S. C., and Sillanpaa, M. (2016). Reduced winter runoff in a mountainous permafrost region in the northern Tibetan Plateau. Cold Reg. Sci. Technol. 126, 36–43. doi:10.1016/j.coldregions.2016.03.007
Gao, T. G., , Zhang, T. J., Guo, H., Hu, Y. T., Shang, J. G., and Zhang, Y. L. (2018). Impacts of the active layer on runoff in an upland permafrost basin, northern Tibetan Plateau. PLoS One. 13. doi:10.1371/journal.pone.0192591
Gao, T., Kang, S., Chen, R., Zhang, T., and Zhang, Y. (2019). Riverine dissolved organic carbon and its optical properties in a permafrost region of the upper heihe river basin in the northern Tibetan plateau. Sci. Total Environ. 686, 370–381. doi:10.1016/j.scitotenv.2019.05.478
Ge, S. M., Mckenzie, J., Voss, C., and Wu, Q. B. (2011). Exchange of groundwater and surface-water mediated by permafrost response to seasonal and long term air temperature variation. Geophys. Res. Lett. 38. doi:10.1029/2011GL047911
Han, P. F., Long, D., Han, Z. Y., Du, M. D., Dai, L. Y., and Hao, X. H. (2019). Improved understanding of snowmelt runoff from the headwaters of China’s Yangtze River using remotely sensed snow products and hydrological modeling. Remote Sens. Environ. 224, 44–59. doi:10.1016/j.rse.2019.01.041
Hu, Y. L., Ma, R., Wang, Y. X., Chang, Q. X., Wang, S., Ge, M. Y., et al. (2019). Using hydrogeochemical data to trace groundwater flow paths in a cold alpine catchment. Hydrol. Process. 33, 1942–1960. doi:10.1002/hyp.13440
Huang, K. W., Dai, J. C., Wang, G. X., Chang, J., Lu, Y. Q., Song, C. L., et al. (2019). The impact of land surface temperatures on suprapermafrost groundwater on the central Qinghai-Tibet Plateau. Hydrol. Process. 34, 1475–1488. doi:10.1002/hyp.13677
Huang, Q., Long, D., Du, M., Han, Z., and Pengfei, H. (2020). Daily continuous river discharge estimation for ungauged basins using a hydrologic model calibrated by satellite altimetry: implications for the SWOT mission. Water Resour. Res. 56, e2020WR027309. doi:10.1029/2020WR027309
Huang, Q., Long, D., Du, M. D., Zeng, C., Li, X. D., Hou, A. Z., et al. (2018). An improved approach to monitoring Brahmaputra river water levels using retracked altimetry data. Remote Sens. Environ. 211, 112–128. doi:10.1016/j.rse.2018.04.018
Huang, X. D., Deng, J., Wang, W., Feng, Q. S., and Liang, T. G. (2017). Impact of climate and elevation on snow cover using integrated remote sensing snow products in Tibetan Plateau. Remote Sens. Environ. 190, 274–288. doi:10.1016/j.rse.2016.12.028
Immerzeel, W. W., Van Beek, L. P. H., and Bierkens, M. F. P. (2010). Climate change will affect the asian water towers. Science. 328, 1382–1385. doi:10.1126/science.1183188
Immerzeel, W. W., Wanders, N., Lutz, A. F., Shea, J. M., and Bierkens, M. F. P. (2015). Reconciling high-altitude precipitation in the upper Indus basin with glacier mass balances and runoff. Hydrol. Earth Syst. Sci. 19, 4673–4687. doi:10.5194/hess-19-4673-2015
Iwata, Y., Hayashi, M., Suzuki, S., Hirota, T., and Hasegawa, S. (2010). Effects of snow cover on soil freezing, water movement, and snowmelt infiltration: a paired plot experiment. Water Resour. Res. 46. doi:10.1029/2009WR008070
Jiao, Y. L., Li, R., Zhao, L., Wu, T. H., Xiao, Y., Hu, G. J., et al. (2014). Processes of soil thawing-freezing and features of soil moisture migration in the permafrost active layer. J. Glaciol. Geocryol. 36, 237–247.
Kang, E. S., Cheng, G. D., Lan, Y. C., Chen, R. S., and Zhang, J. S. (2002). Application of a conceptual hydrological model in the runoff forecast of a mountainous watershed. Adv. Earth Sci. 18–26.
Kirchner, J. W. (2006). Getting the right answers for the right reasons: Linking measurements, analyses, and models to advance the science of hydrology. Water Resour. Res. 42, W03S04. doi:10.1029/2005WR004362
Kong, Y., and Wang, C. H. (2017). Responses and changes in the permafrost and snow water equivalent in the Northern Hemisphere under a scenario of 1.5°C warming. Adv. Clim. Change Res. 8, 235–244. doi:10.1016/j.accre.2017.07.002
Kong, Y., Wang, K., Pu, T., and Shi, X. (2019). Nonmonsoon precipitation dominates groundwater recharge beneath a monsoon-affected glacier in Tibetan plateau. J. Geophys. Res. Atmos. 124, 10913–10930. doi:10.1029/2019JD030492
Kuang, X., and Jiao, J. J. (2016). Review on climate change on the Tibetan Plateau during the last half century. J. Geophys. Res. Atmos. 121, 3979–4007. doi:10.1002/2015JD024728
Lafrenière, M. J., and Lamoureux, S. F. (2019). Effects of changing permafrost conditions on hydrological processes and fluvial fluxes. Earth Sci. Rev. 191, 212–223. doi:10.1016/j.earscirev.2019.02.018
Li, Z., Tang, P. P., Zhou, J. M., Tian, B. S., Chen, Q., and Fu, S. T. (2015). Permafrost environment monitoring on the Qinghai-Tibet Plateau using time series ASAR images. Int. J. Digit. Earth. 8, 840–860. doi:10.1080/17538947.2014.923943
Lindstrom, G., Bishop, K., and Lofvenius, M. O. (2002). Soil frost and runoff at Svartberget, northern Sweden - measurements and model analysis. Hydrol. Process. 16, 3379–3392. doi:10.1002/hyp.1106
Liu, F. J., Hunsaker, C., and Bales, R. C. (2013). Controls of streamflow generation in small catchments across the snow-rain transition in the Southern Sierra Nevada, California. Hydrol. Process. 27, 1959–1972. doi:10.1002/hyp.9304
Liu, J. S., Wang, S. Y., and Huang, Y. Y. (2007). Effect of climate change on runoff in a basin with mountain permafrost, northwest China. Permafr. Periglac. Process. 18, 369–377. doi:10.1002/ppp.602
Liu, L., Zhang, W. J., Lu, Q. F., Jiang, H. R., Tang, Y., Xiao, H. M., et al. (2020). Hydrological impacts of near-surface soil warming on the Tibetan Plateau. Permafr. Periglac. Process. 31, 324–336. doi:10.1002/ppp.2049
Liu, S. L., Cheng, F. Y., Dong, S. K., Zhao, H. D., Hou, X. Y., and Wu, X. (2017). Spatiotemporal dynamics of grassland aboveground biomass on the Qinghai-Tibet Plateau based on validated MODIS NDVI. Sci. Rep. 7, 4182. doi:10.1038/s41598-017-04038-4
Lu, M., Yang, S., Li, Z., He, B., He, S., and Wang, Z. (2018). Possible effect of the Tibetan plateau on the “upstream” climate over west Asia, North africa, south europe and the north atlantic. Clim. Dynam. 51, 1485–1498. doi:10.1007/s00382-017-3966-5
Luo, D., Jin, H., Jin, X., He, R., Li, X., Muskett, R. R., et al. (2018). Elevation-dependent thermal regime and dynamics of frozen ground in the Bayan Har Mountains, northeastern Qinghai-Tibet Plateau, southwest China. Permafr. Periglac. Process. 29, 257–270. doi:10.1002/ppp.1988
Lyon, S. W., Destouni, G., Giesler, R., Humborg, C., Morth, M., Seibert, J., et al. (2009). Estimation of permafrost thawing rates in a sub-arctic catchment using recession flow analysis. Hydrol. Earth Syst. Sci. 13, 595–604. doi:10.5194/hessd-6-63-2009
Ma, R., Sun, Z. Y., Hu, Y. L., Chang, Q. X., Wang, S., Xing, W. L., et al. (2017). Hydrological connectivity from glaciers to rivers in the Qinghai-Tibet Plateau: roles of suprapermafrost and subpermafrost groundwater. Hydrol. Earth Syst. Sci. 21, 4803–4823. doi:10.5194/hess-21-4803-2017
Ma, Y. M., Wang, Y. Y., and Han, C. B. (2018). Regionalization of land surface heat fluxes over the heterogeneous landscape: from the Tibetan Plateau to the third pole region. Int. J. Rem. Sens. 39, 5872–5890. doi:10.1080/01431161.2018.1508923
Mcdonnell, J. J., Sivapalan, M., Vache, K., Dunn, S., Grant, G., Haggerty, R., et al. (2007). Moving beyond heterogeneity and process complexity: a new vision for watershed hydrology. Water Resour. Res. 43. doi:10.1029/2006WR005467
Mu, C. C., Zhang, F., Chen, X., Ge, S. M., Mu, M., Jia, L., et al. (2019). Carbon and mercury export from the Arctic rivers and response to permafrost degradation. Water Res. 161, 54–60. doi:10.1016/j.watres.2019.05.082
O’neill, H. B., Roy-Leveillee, P., Lebedeva, L., and Ling, F. (2020). Recent advances (2010–2019) in the study of taliks. Permafr. Periglac. Process. 31, 346–357. doi:10.1002/ppp.2050
Pan, X. C., Yu, Q. H., You, Y. H., Chun, K. P., Shi, X. G., and Li, Y. P. (2017). Contribution of supra-permafrost discharge to thermokarst lake water balances on the northeastern Qinghai-Tibet Plateau. J. Hydrol. 555, 621–630. doi:10.1016/j.jhydrol.2017.10.046
Pomeroy, J. W., Gray, D. M., Brown, T., Hedstrom, N. R., Quinton, W. L., Granger, R. J., et al. (2007). The cold regions hydrological process representation and model: a platform for basing model structure on physical evidence. Hydrol. Process. 21, 2650–2667. doi:10.1002/hyp.6787
Qin, J., Ding, Y. J., and Han, T. D. (2020). Quantitative assessment of winter baseflow variations and their causes in Eurasia over the past 100 years. Cold Reg. Sci. Technol. 172, 102989. doi:10.1016/j.coldregions.2020.102989
Savenije, H. H. G. (2009). HESS Opinions “The art of hydrology”. Hydrol. Earth Syst. Sci. 13, 157–161. doi:10.5194/hessd-5-3157-2008
Shanley, J. B., and Chalmers, A. (1999). The effect of frozen soil on snowmelt runoff at Sleepers River, Vermont. Hydrol. Process. 13, 1843–1857. doi:10.1002/(SICI)1099-1085(199909)13:12/13<1843::AID-HYP879>3.0.CO;2-G
Sivapalan, M., Bloschl, G., Zhang, L., and Vertessy, R. (2003). Downward approach to hydrological prediction. Hydrol. Process. 17, 2101–2111. doi:10.1002/hyp.1425
Smith, A., Tetzlaff, D., Laudon, H., Maneta, M., and Soulsby, C. (2019). Assessing the influence of soil freeze-thaw cycles on catchment water storage-flux-age interactions using a tracer-aided ecohydrological model. Hydrol. Earth Syst. Sci. 23, 3319–3334. doi:10.5194/hess-23-3319-2019
Song, C. L., Wang, G. X., Mao, T. X., Dai, J. C., and Yang, D. Q. (2020). Linkage between permafrost distribution and river runoff changes across the Arctic and the Tibetan Plateau. Sci. China Earth Sci. 63, 292–302. doi:10.1007/s11430-018-9383-6
Sun, A. L., Yu, Z. B., Zhou, J., Acharya, K., Ju, Q., Xing, R. F., et al. (2020). Quantified hydrological responses to permafrost degradation in the headwaters of the Yellow River (HWYR) in High Asia. Sci. Total Environ. 712, 135632. doi:10.1016/j.scitotenv.2019.135632
Sun, Q. H., Miao, C. Y., Duan, Q. Y., Ashouri, H., Sorooshian, S., and Hsu, K. L. (2018). A review of global precipitation data sets: data sources, estimation, and intercomparisons. Rev. Geophys. 56, 79–107. doi:10.1002/2017RG000574
Walvoord, M. A., and Kurylyk, B. L. (2016). Hydrologic impacts of thawing permafrost-A review. Vadose Zone J. 15. doi:10.2136/vzj2016.01.0010
Wan, C. W., Gibson, J. J., Shen, S. C., Yi, Y., Yi, P., and Yu, Z. B. (2019). Using stable isotopes paired with tritium analysis to assess thermokarst lake water balances in the Source Area of the Yellow River, northeastern Qinghai-Tibet Plateau, China. Sci. Total Environ. 689, 1276–1292. doi:10.1016/j.scitotenv.2019.06.427
Wang, G. X., and Zhang, Y. S. (2016). Ecohydrology in cold regions: theory and practice. Beijing: Science Press.
Wang, C., Yang, K., and Zhang, F. (2020). Impacts of soil freeze–thaw process and snow melting over Tibetan plateau on asian summer monsoon system: a review and perspective. Front. Earth Sci. 8:133. doi:10.3389/feart.2020.00133
Wang, G. X., Li, Y. S., Wu, Q. B., and Wang, Y. B. (2006). Impacts of permafrost changes on alpine ecosystem in Qinghai-Tibet Plateau. Sci. China Earth Sci. 49, 1156–1169. doi:10.1007/s11430-006-1156-0
Wang, G. X., Lin, S., Hu, Z. Y., Lu, Y. Q., Sun, X. Y., and Huang, K. W. (2020). Improving actual evapotranspiration estimation integrating energy consumption for ice phase change across the Tibetan plateau. J. Geophys. Res. Atmos. 125. doi:10.1029/2019JD031799
Wang, G. X., Liu, L. A., Liu, G. S., Hu, H. C., and Li, T. B. (2010). Impacts of grassland vegetation cover on the active-layer thermal regime, Northeast Qinghai-Tibet Plateau, China. Permafr. Periglac. Process. 21, 335–344. doi:10.1002/ppp.699
Wang, G. X., Mao, T. X., Chang, J., Song, C. L., and Huang, K. W. (2017). Processes of runoff generation operating during the spring and autumn seasons in a permafrost catchment on semi-arid plateaus. J. Hydrol. 550, 307–317. doi:10.1016/j.jhydrol.2017.05.020
Wang, N. L., Zhang, S. B., He, J. Q., Pu, J. C., Wu, X. B., and Jiang, X. (2009). Tracing the major source area of the mountainous runoff generation of the Heihe River in northwest China using stable isotope technique. Chin. Sci. Bull. 54, 2751–2757. doi:10.1007/s11434-009-0505-8
Wang, Y. H., Yang, H. B., Gao, B., Wang, T. H., Qin, Y., and Yang, D. W. (2018). Frozen ground degradation may reduce future runoff in the headwaters of an inland river on the northeastern Tibetan Plateau. J. Hydrol. 564, 1153–1164. doi:10.1016/j.jhydrol.2018.07.078
Woo, M. K., Kane, D. L., Carey, S. K., and Yang, D. Q. (2008). Progress in permafrost hydrology in the new millennium. Permafr. Periglac. Process. 19, 237–254.
Wu, Y. H., and Zhu, L. P. (2008). The response of lake-glacier variations to climate change in Nam Co Catchment, central Tibetan Plateau, during 1970-2000. J. Geogr. Sci. 18, 177–189. doi:10.1007/s11442-008-0177-3
Wu, P., Liang, S. H., Wang, X. S., Mckenzie, J. M., and Feng, Y. Q. (2020a). Climate change impacts on cold season runoff in the headwaters of the Yellow River considering frozen ground degradation. Water. 12, 602. doi:10.3390/w12020602
Wu, Q. B., Li, Z. J., and Shen, Y. P. (2020b). Cryosphere engineering science supporting interactivity infrastructures construction. Bull. Chin. Acad. Sci. 35, 443–449. doi:10.16418/j.issn.1000-3045.20200301001
Wu, S. H., Jansson, P. E., and Zhang, X. Y. (2011). Modelling temperature, moisture and surface heat balance in bare soil under seasonal frost conditions in China. Eur. J. Soil Sci. 62, 780–796. doi:10.1111/j.1365-2389.2011.01397.x
Wu, T. H., Li, S. X., Cheng, G. D., and Nan, Z. T. (2005). Using ground-penetrating radar to detect permafrost degradation in the northern limit of permafrost on the Tibetan Plateau. Cold Reg. Sci. Technol. 41, 211–219. doi:10.1016/j.coldregions.2004.10.006
Xiang, L. W., Wang, H. S., Steffen, H., Wu, P., Jia, L. L., Jiang, L. M., et al. (2016). Groundwater storage changes in the Tibetan Plateau and adjacent areas revealed from GRACE satellite gravity data. Earth Planet Sci. Lett. 449, 228–239. doi:10.1016/j.epsl.2016.06.002
Xiao, Y., Zhao, L., Dai, Y. J., Li, R., Pang, Q. Q., and Yao, J. M. (2013). Representing permafrost properties in CoLM for the Qinghai-Xizang (Tibetan) plateau. Cold Reg. Sci. Technol. 87, 68–77. doi:10.1016/j.coldregions.2012.12.004
Xie, C. W., and Gough, W. A. (2013). A simple thaw-freeze algorithm for a multi-layered soil using the stefan equation. Permafr. Periglac. Process. 24, 252–260. doi:10.1002/ppp.1770
Yang, D., Herath, S., and Musiake, K. (1998). Development of a geomorphology-based hydrological model for large catchments. Ann. J. Hyd. Eng. JSCE 42, 169–174.
Yang, K., Qin, J., Zhao, L., Chen, Y. Y., Tang, W. J., Han, M. L., Lazhu Chen, Z. Q., Lv, N., Ding, B. H., Wu, H., and Lin, C. G. (2013). A multiscale soil moisture and freeze-thaw monitoring network ON the third POLE. Bull. Am. Meteorol. Soc. 94, 1907–1916. doi:10.1175/BAMS-D-12-00203.1
Yang, K., Wu, H., Qin, J., Lin, C. G., Tang, W. J., and Chen, Y. Y. (2014). Recent climate changes over the Tibetan Plateau and their impacts on energy and water cycle: a review. Global Planet. Change. 112, 79–91. doi:10.1016/j.gloplacha.2013.12.001
Yang, M. X., Nelson, F. E., Shiklomanov, N. I., Guo, D. L., and Wan, G. N. (2010). Permafrost degradation and its environmental effects on the Tibetan Plateau: a review of recent research. Earth Sci. Rev. 103, 31–44. doi:10.1016/j.earscirev.2010.07.002
Yang, M. X., Yao, T. D., Gou, X. H., Koike, T., and He, Y. Q. (2003). The soil moisture distribution, thawing-freezing processes and their effects on the seasonal transition on the Qinghai-Xizang (Tibetan) plateau. J. Asian Earth Sci. 21, 457–465. doi:10.1016/S1367-9120(02)00069-X
Yang, Y., Chen, R. S., Ye, B. S., Song, Y. X., Liu, J. F., Han, C. T., et al. (2013). Heat and water transfer processes on the Typical underlying surfacesof frozen soil in cold regions (Ⅰ): model comparison. J. Glaciol. Geocryol. 35, 1545–1554.
Yang, Y. Z., Wu, Q. B., Jin, H. J., Wang, Q. F., Huang, Y. D., Luo, D. L., et al. (2019). Delineating the hydrological processes and hydraulic connectivities under permafrost degradation on Northeastern Qinghai-Tibet Plateau, China. J. Hydrol. 569, 359–372. doi:10.1016/j.jhydrol.2018.11.068
Ye, B. S., Yang, D. Q., Zhang, Z. L., and Kane, D. L. (2009). Variation of hydrological regime with permafrost coverage over Lena Basin in Siberia. J. Geophys. Res. Atmos. 114. doi:10.1029/2008JD010537
Yi, S. H., Woo, M. K., and Arain, M. A. (2007). Impacts of peat and vegetation on permafrost degradation under climate warming. Geophys. Res. Lett. 34. doi:10.1029/2007GL030550
You, Q. L., Wu, T., Shen, L. C., Pepin, N., Zhang, L., Jiang, Z. H., et al. (2020). Review of snow cover variation over the Tibetan Plateau and its influence on the broad climate system. Earth Sci. Rev. 201. doi:10.1016/j.earscirev.2019.103043
Yu, L. Y., Zeng, Y. J., Wen, J., and Su, Z. B. (2018). Liquid-vapor-air flow in the frozen soil. J. Geophys. Res. Atmos. 123, 7393–7415. doi:10.1029/2018JD028502
Yu, Q. H., Pan, X. C., Cheng, G. D., and He, N. W. (2008). An experimental study on the cooling mechanism of a shading board in permafrost engineering. Cold Reg. Sci. Technol. 53, 298–304. doi:10.1016/j.coldregions.2007.07.003
Zeng, Y. J., Su, Z. B., Van Der Velde, R., Wang, L. C., Xu, K., Wang, X., et al. (2016). Blending satellite observed, model simulated, and in situ measured soil moisture over Tibetan plateau. Rem. Sens. 8, 268. doi:10.3390/rs8030268
Zhang, G. Q., Yao, T. D., Piao, S. L., Bolch, T., Xie, H. J., Chen, D. L., et al. (2017). Extensive and drastically different alpine lake changes on Asia's high plateaus during the past four decades. Geophys. Res. Lett. 44, 252–260. doi:10.1002/2016GL072033
Zhang, T. (2005). Influence of the seasonal snow cover on the ground thermal regime: an overview, Rev. Geophys. 43, RG4002. doi:10.1029/2004RG000157
Zhang, T. J., Jin, R., and Gao, F. (2009). Overview of the satellite remote sensing of frozen ground: visible-thermal infrared and radar sensor. Adv. Earth Sci. 24, 963–972. doi:10.1080/789610186
Zhang, Y. L., Cheng, G. D., Li, X., Han, X. J., Wang, L., Li, H. Y., et al. (2013). Coupling of a simultaneous heat and water model with a distributed hydrological model and evaluation of the combined model in a cold region watershed. Hydrol. Process. 27, 3762–3776. doi:10.1002/hyp.9514
Zhang, Y. S., and Guo, Y. (2011). “Water cycle changes during the past 50 years over the Tibetan Plateau: review and synthesis,” in Cold regions hydrology in a changing climate. Editors D. Yang, P. Marsh, and A. Gelfan, 130–135.
Zhang, Y. S., Ohata, T., and Kadota, T. (2003). Land-surface hydrological processes in the permafrost region of the eastern Tibetan Plateau. J. Hydrol. 283, 41–56. doi:10.1016/S0022-1694(03)00240-3
Zhang, Z., and Wu, Q. (2012). Predicting changes of active layer thickness on the Qinghai-Tibet Plateau as climate change. J. Glaciol. Geocryol. 34 (3), 506–511
Zhao, J. Y., Chen, J., Wu, Q. B., and Hou, X. (2018). Snow cover influences the thermal regime of active layer in Urumqi River Source, Tianshan Mountains, China. J. Mountain Sci. 15 (12). doi:10.1007/s11629-018-4856-y
Zhao, L., Zou, D., Hu, G., Du, E., Pang, Q., Xiao, Y., et al. (2020). Changing climate and the permafrost environment on the Qinghai–Tibet (Xizang) plateau. Permafr. Periglac. Process. 31, 396–405. doi:10.1002/ppp.2056
Zhao, L., Hu, G. J., Zou, D. F., Wu, X. D., Ma, L., Sun, Z., et al. (2019). Permafrost changes and its effects on hydrological processes on Qinghai-Tibet Plateau. Bull. Chin. Acad. Sci. 34, 1233–1246. doi:10.16418/j.issn.1000-3045.2019.11.006
Zheng, D., Wang, X., Velde, R. V. D., Zeng, Y., Wen, J., Wang, Z., et al. (2017). L-band microwave emission of soil freeze–thaw process in the third pole environment. Remote Sens. Environ. 55, 5324–5338. doi:10.1109/TGRS.2017.2705248
Zhou, J., Kinzelbach, W., Cheng, G., Zhang, W., He, X., and Ye, B. (2013). Monitoring and modeling the influence of snow pack and organic soil on a permafrost active layer, Qinghai–Tibetan Plateau of China. Cold Reg. Sci. Technol. 90-91, 38–52. doi:10.1016/j.coldregions.2013.03.003
Zhou, J., Pomeroy, J. W., Zhang, W., Cheng, G. D., Wang, G. X., and Chen, C. (2014). Simulating cold regions hydrological processes using a modular model in the west of China. J. Hydrol. 509, 13–24. doi:10.1016/j.jhydrol.2013.11.013
Zhou, J. X., Wu, J. K., Liu, S. W., Zeng, G. X., Qin, J., Wang, X. N., et al. (2015). Hydrograph separation in the headwaters of the shule river basin: combining water chemistry and stable isotopes. Adv. Meteorol. 2015, 1–10. doi:10.1155/2015/830306
Zhou, J., Zhang, W., Pomeroy, J., Cheng, G. D., Wang, G. X., Chen, C., et al. (2013). Simulating the cold regions hydrological processes in Northwest China with modular modeling method. J. Glaciol. Geocryol. 35, 389–400. doi:10.1016/j.jhydrol.2013.11.013
Zhou, X. H., Zhou, J., Kinzelbach, W., and Stauffer, F. (2014). Simultaneous measurement of unfrozen water content and ice content in frozen soil using gamma ray attenuation and TDR. Water Resour. Res. 50, 9630–9655. doi:10.1002/2014WR015640
Keywords: permafrost, frozen soil, Qinghai-Tibet Plateau, freeze–thaw process, permafrost hydrological model
Citation: Gao H, Wang J, Yang Y, Pan X, Ding Y and Duan Z (2021) Permafrost Hydrology of the Qinghai-Tibet Plateau: A Review of Processes and Modeling. Front. Earth Sci. 8:576838. doi: 10.3389/feart.2020.576838
Received: 27 June 2020; Accepted: 19 October 2020;
Published: 12 January 2021.
Edited by:
Xiuping Li, Institute of Tibetan Plateau Research (CAS), ChinaCopyright © 2021 Gao, Wang, Yang, Pan, Ding and Duan. This is an open-access article distributed under the terms of the Creative Commons Attribution License (CC BY). The use, distribution or reproduction in other forums is permitted, provided the original author(s) and the copyright owner(s) are credited and that the original publication in this journal is cited, in accordance with accepted academic practice. No use, distribution or reproduction is permitted which does not comply with these terms.
*Correspondence: Hongkai Gao, Z2FvaG9uZ2thaTIwMDVAMTI2LmNvbQ==; Yongjian Ding, ZHlqQGx6Yi5hYy5jbg==