- 1Department of Earth Sciences, University of Geneva, Geneva, Switzerland
- 2IDEAN (UBA-CONICET), Buenos Aires, Argentina
- 3Institute of National Agricultural Technology, San Carlos de Bariloche, Argentina
- 4INGEMMET, Observatorio Vulcanológico del INGEMMET, Arequipa, Peru
- 5Met Office, Exeter, United Kingdom
- 6Servicio Geológico Minero Argentino (SEGEMAR), Buenos Aires, Argentina
- 7Desert Research Institute, Reno, NV, United States
- 8Earth and Environmental Sciences, Ludwig-Maximilians-Universität München, Munich, Germany
- 9Department of Physics and Astronomy, Aarhus University, Aarhus, Denmark
- 10Facultad de Ciencias, Universidad de Colima, Colima, Mexico
- 11U.S. Geological Survey, Alaska Volcano Observatory, Volcano Science Center, Anchorage, AK, United States
During explosive volcanic eruptions, large quantities of tephra can be dispersed and deposited over wide areas. Following deposition, subsequent aeolian remobilisation of ash can potentially exacerbate primary impacts on timescales of months to millennia. Recent ash remobilisation events (e.g., following eruptions of Cordón Caulle 2011; Chile, and Eyjafjallajökull 2010, Iceland) have highlighted this to be a recurring phenomenon with consequences for human health, economic sectors, and critical infrastructure. Consequently, scientists from observatories and Volcanic Ash Advisory Centers (VAACs), as well as researchers from fields including volcanology, aeolian processes and soil sciences, convened at the San Carlos de Bariloche headquarters of the Argentinian National Institute of Agricultural Technology to discuss the “state of the art” for field studies of remobilised deposits as well as monitoring, modeling and understanding ash remobilisation. In this article, we identify practices for field characterisation of deposits and active processes, including mapping, particle characterisation and sediment traps. Furthermore, since forecast models currently rely on poorly-constrained dust emission schemes, we call for laboratory and field measurements to better parameterise the flux of volcanic ash as a function of friction velocity. While source area location and extent are currently the primary inputs for dispersion models, once emission schemes become more sophisticated and better constrained, other parameters will also become important (e.g., source material volume and properties, effective precipitation, type and distribution of vegetation cover, friction velocity). Thus, aeolian ash remobilisation hazard and associated impact assessment require systematic monitoring, including the development of a regularly-updated spatial database of resuspension source areas.
Introduction
Explosive volcanic eruptions can disperse significant quantities of ash (diameter <2 mm) over large areas (up to 106 km2; Pyle et al., 2006). Resultant subaerial deposits can consequently be remobilised by different aeolian processes, with larger particles moving by saltation or creep and finer material in suspension (Kok et al., 2012). Regarding ash, remobilisation refers to all aeolian transport mechanisms, whereas resuspension refers only to suspension (Dominguez et al., 2020). Although many sediments undergo aeolian transport, remobilised volcanic ash poses additional hazards to human and animal health and infrastructure (particularly aviation) due to the transient sediment supply, high abrasive potential, low softening temperature and the presence of leachable contaminants.
Impacts from remobilised ash include those shared with, albeit with some differences to, primary ashfall. Airborne particulate matter (PM) threatens human health, contributing to cardiovascular and respiratory diseases (Baxter and Horwell, 2015). Agriculturally, ash can damage crops and contaminate livestock food sources (Craig et al., 2016b; Forte et al., 2018), e.g., fluoride leaching (Cronin et al., 2003). Airborne ash can impact air traffic (Hadley et al., 2004; Elissondo et al., 2016) and also reduce visibility (Weinzierl et al., 2012), threatening transport networks. Remobilisation can extend the temporal and spatial scales of these impacts. Additionally, deposits can form migrating bedforms that further inundate farmland (Wilson et al., 2011). Such structures can inhibit water drainage and become lahar sources. Local meteorology and topography, rather than eruptive style, influence the altitude which resuspended ash reaches. Resuspended ash emitted in September 2013 and April 2017 in Iceland rose up to 2 km due to a temperature inversion (Beckett et al., 2017; Hammond and Beckett, 2019), whereas that from the 2020 Taal eruption, the Philippines (NDRRMC, 2020), and ancient pyroclastic material in the Fiambalá Basin, Argentina (Mingari et al., 2017), have reached 5–6 km. Furthermore, the greater abrasivity and lower softening point of ash (≥700°C) compared to mineral dust (Kueppers et al., 2014) means that ash can potentially damage hot engines more than other remobilised material (Müller et al., 2019; Butwin et al., 2020).
Ash remobilisation was first recorded in 1933, when ash from the 1912 Katmai-Novarupta eruption deposits was observed across northern North America (Alexander, 1934; Miller, 1934). Wider recognition followed the 1980 Mt. St. Helens eruption, USA, where winds around 10 m s−1 remobilised fine ash, significantly reducing visibility (Hobbs et al., 1983). More recently, resuspension following eruptions from Eyjafjallajökull (2010) and Grímsvötn (2011), Iceland (Thorsteinsson et al., 2012; Liu et al., 2014; Butwin et al., 2019), and Hudson (Wilson et al., 2011), Cordón Caulle (Craig et al., 2016a; Forte et al., 2018) and Calbuco (Reckziegel et al., 2016), Chile, have highlighted this secondary hazard. Ash remobilisation occurs over widely-varying timescales, from syn-eruptive to millennia post-eruption (Hadley et al., 2004; Mingari et al., 2017). Furthermore, old deposits exposed by anthropogenic activities, e.g., quarrying and deforestation, can become remobilisable (WMO, 2016; Bonadonna et al., 2020).
The recent and recurrent observations of ash remobilisation events highlight the need for increased monitoring, forecasting and research. Additionally, at the 2016 World Meteorological Organization Volcanic Ash Advisory Center (VAAC) “Best Practice” workshop (Buenos Aires), it was decided that “all VAACs treat re-suspended ash as any other ash cloud and would issue a volcanic ash advisory (VAA) to advise users of it” (WMO, 2016). VAACs therefore rely on monitoring of ash source areas and accurate parameterisations for aeolian processes. In order to identify the required objectives and associated challenges of future work on ash remobilisation, a workshop on wind-remobilisation processes of volcanic ash was held in San Carlos de Bariloche, Argentina (https://www.unige.ch/sciences/terre/CERG-C/international-conferences/ash-remobilisation-2019/presentation/; Bonadonna et al., 2020), involving 47 participants from observatories, VAACs and research institutions, across multiple disciplines (volcanology, aeolian processes, soil science) and expertise (field, experimental, numerical modeling). The workshop identified:
• Parameters controlling duration and intensity of remobilisation events
• Input parameters required for modeling ash remobilisation
• Key issues for monitoring and communicating remobilisation hazard
• Research priorities for understanding and characterising ash remobilisation deposits and processes
The detailed outcomes were reported in a consensual document (Bonadonna et al., 2020) while this paper summarises the key findings and provides perspectives for future work.
Mechanisms of aeolian Remobilisation
Sediment Transport
Grains within a deposit can be remobilised if the friction velocity u∗, a surface shear-stress proxy, exceeds a threshold u∗t. This threshold is determined from a force-balance on a surficial grain; wind-drag and aerodynamic lift, which act to entrain the particle, are resisted by gravity and inter-granular cohesion. While various models describe this balance, it is accepted that
Once mobile, particles can be transported through different modes. Those of diameter ∼70–500 μm saltate, following ballistic trajectories (Bagnold, 1941), while larger particles reptate (jumps <1 cm; Ungar & Haff, 1987) or creep (rolling/sliding; Bagnold, 1941). Finer particles become suspended (Nickling and McKenna Neuman, 2009), with those of diameter ≤20 μm entering long-term (weeks-months) suspension and those in the range 20–70 μm undergoing short-term suspension (≤days).
Controls on Duration and Intensity of Remobilisation Events
The key control on the duration and intensity of a remobilisation event is
Deposit properties also control remobilisation susceptibility. Specifically, the volume of erupted material, deposit thickness and spatial extent directly control the amount of remobilisable material available. For large eruptions in arid landscapes, e.g., the 1912 Novarupta eruption which produced ∼28 km3 of pyroclastic deposit (Fierstein and Hildreth, 1992), remobilisation can continue for centuries post-eruption (Hadley et al., 2004), although re-vegetation in more humid climates can stabilise deposits. Other important deposit characteristics include the surficial grain size distribution (GSD) and particle density, since these largely control
For fine particles,
Finally, the amount and type of vegetation onto which ash deposits are also important. Plants can act as sediment traps, whereby ash (primary or remobilised) deposited within and around a plant can be protected from aeolian forcing (Dominguez et al., 2020). The effectiveness of a particular species as a sediment trap depends on its size and structural porosity (Wolfe and Nickling, 1993; Gillies et al., 2002).
Field Characterisation of Remobilisation Processes and Deposits
Fieldwork characterising remobilisation processes and the resulting deposits is crucial for multiple reasons. Aside from improving our fundamental understanding, measurements pertaining to ash remobilisation allow testing of model parameterisations. Furthermore, field data provide necessary inputs for numerical models, e.g., Fall3D which uses a grainsize-dependent emission scheme (Folch et al., 2014). Thus, to maximise the usefulness of field data for interpretation and use in models, common methodologies are required to allow spatial and temporal comparisons.
Since any exposed sediments are potential sources of transportable material, both primary and remobilised ash deposits require examination. Table 1 summarises important observations and measurements that should be made concerning deposits. Particularly important are the thickness and spatial distribution of the primary deposit, since these constrain the volume of remobilisable material. Ultimately, deposit features can constrain
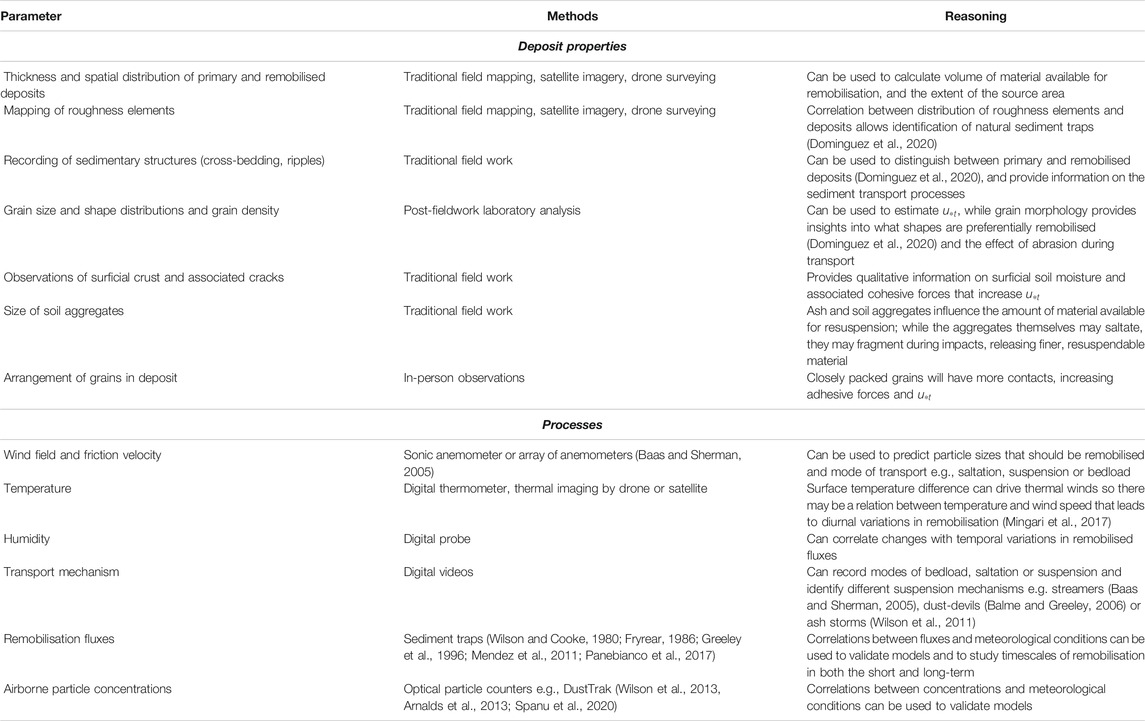
TABLE 1. Key deposit properties and processes to observe and measure in the field in order to characterise ash remobilisation.
An initial challenge is distinguishing between primary and remobilised deposits, particularly when syn-eruptive remobilisation occurs, e.g., Sabancaya volcano, Peru, where multiple Vulcanian explosions currently occur daily, depositing ash that is continuously remobilised. Nonetheless, some general features can differentiate between primary and remobilised deposits (Dominguez et al., 2020). Specifically, primary deposit thicknesses decrease with distance from the vent but are typically uniform at a local scale (assuming deposition on a flat surface). Conversely, surface roughness strongly controls the erosion and re-deposition of ash, generating sub-metre variations in remobilised deposit thicknesses and even unconnected deposits. Cross-bedding, resulting from unsteady wind conditions, can also be indicative in remobilised deposits.
Field measurements characterising remobilisation processes can also be performed. Particularly important is characterising
Modeling and Forecasting of Remobilisation
Modeling remobilisation currently focusses on dispersion modeling of resuspended ash clouds, with an emphasis on operational forecasts (Folch et al., 2014), e.g., the United Kingdom Met Office forecasts resuspension on a daily basis in Iceland using NAME (Hammond and Beckett, 2019). The model source terms come from emission schemes that provide the vertical mass flux of material F(
Dispersion model results for ash resuspension strongly depend on the horizontal and vertical resolution of meteorological and topography data and the dispersion model. In the operational setup of NAME, source regions are represented at a horizontal resolution of 0.01° × 0.01° (Leadbetter et al., 2012) while meteorological and topographical fields have a horizontal resolution of ∼10 km (at mid-latitudes). Sufficiently fine horizontal resolutions are required to capture topographic effects on
Monitoring and Communicating Remobilisation Hazard and Mitigating Impact
Ash remobilisation can impact local and regional stakeholders (e.g., damage to livestock and crops, air quality alteration, air traffic disruption). It is therefore important that observatories can monitor such processes and provide useful information. Locally, communities may observe ash and be concerned that an eruption is occurring, meaning observatories require the ability to identify the source of the ash (eruptive or aeolian) and communicate this to local stakeholders. Promising techniques for distinguishing between primary and remobilised ash clouds include satellite observations (e.g., cloud location, water content estimates; Toyos et al., 2017) in combination with ground-based cameras and geophysical monitors. No single method is likely to be definitive, thus data from different sources requires synthesis, presenting a challenge for poorly-monitored volcanoes.
Regionally, a 2016 VAAC meeting (Buenos Aires) agreed that best practice would be to release a VAA for resuspension clouds if there are supporting observations (WMO, 2016), with low-altitude ash being a hazard near airports. Forecasting by VAACs, therefore, relies on updated monitoring data being provided by observatories. Despite this, monitoring and communication strategies vary globally. Even though the nine observatories involved in the workshop (Supplementary Material) confirmed that ash remobilisation is a common and concerning phenomenon, only four of these routinely monitor and/or report it. This lack of consistency makes providing usable information to VAACs difficult, something that could be alleviated with standardised protocols for data collection and communication. However, timely and precise monitoring of resuspension is challenging.
Table 2 lists parameters relevant to ash remobilisation that need monitoring and is separated into those that require regular recording in preparation for remobilisation episodes, and those to be recorded during events. Those requiring regular recording can evolve periodically (diurnally, seasonally) or monotonically (e.g., source material volume reduces with time). These quantities should ideally be recorded in a continuously-updated database of input parameters to be available for forecast modeling. Ultimately though, this relies on observatories being aware of possible source areas.
During resuspension events, cloud height and extent need to be monitored so that forecast modeling can use assimilated data (Schmehl et al., 2011). A readily-available data source comes from satellite images, which could, for example, be automatically processed in real-time using the VOLCAT program (Pavolonis et al., 2015a; Pavolonis et al., 2015b; Pavolonis et al., 2018). For eruptive clouds, ash detection in satellite images typically uses the brightness temperature difference (BTD) between the 11 and 12 μm channels (Watson et al., 2004), with a negative BTD indicating the presence of ash at high-altitude (Prata, 1989). However, this method is unsuitable for resuspended clouds that remain at altitudes <2 km (Beckett et al., 2017). In these cases, a positive BTD can instead be used to detect ash (Beckett et al., 2017). Satellite imagery is thus an essential resource for forecast modeling, while height estimates are also obtainable by combining satellite observations with radiosonde data (Toyos et al., 2017).
Air quality and visibility present threats during remobilisation events and need to be monitored, but not all observatories can measure these parameters. Variations can also be extremely local and thus go undetected. Furthermore, organisations other than volcano observatories are normally responsible for measuring air quality. Therefore, monitoring strategies need to be tailored to each observatory’s specific needs and capabilities.
Assessing and monitoring ash remobilisation hazards can identify priority mitigation measures for reducing adverse impacts. Following the 2011 Cordón Caulle eruption, short-to medium-term mitigations included road and town clean-ups, livestock refuges, greenhouse cultivation and windbreaks (Wilson et al., 2013). However, the cleaned-up ash is itself remobilisable (Wilson et al., 2011) and longer-term mitigations regarding storage and stabilisation must be considered. The same eruption also demonstrated the importance of prompt livestock relocation to secure refuges or areas. When possible (e.g., in case of detected unrest), this should occur prior to eruption onset.
Concluding Remarks and Research Priorities
Volcanic ash remobilisation threatens human and animal health, various economic sectors, and critical infrastructure. In recent years, progress has been made characterising field deposits, understanding and modeling remobilisation processes, and monitoring remobilisation phenomena. However, progress remains to be made. Further field observations (e.g., spatial distribution and granulometry of ash deposits) are needed to provide valuable input data for remobilisation forecasts while observations of active remobilisation processes, e.g., using sediment traps and PM instruments, can validate models. Operational forecast models of resuspended ash clouds include emission schemes that relate the vertical flux of resuspended material to the wind friction velocity. Such schemes, however, currently neglect deposit properties and further constraints are needed. To address these issues, the workshop participants identified the following research priorities:
• Practices need to be developed for field characterisation. Methodologies should include: mapping of primary and remobilised deposits and roughness elements; textural, GSD and ash morphology measurements; and observations of active remobilisation processes through videos, sediment traps and PM monitors.
• New techniques are needed to better quantify surficial soil moisture, possibly involving ground-penetrating radar or satellite technologies.
• It is necessary to determine controls on
• Laboratory and field measurements are needed to better constrain the relations among
• A regularly updated spatial database of resuspension source areas needs to be available for real-time forecast modeling, including information on areal extents, material properties, effective precipitation and vegetation cover.
• Ad-hoc impact mitigation measures should be identified in preparation for emergencies, including livestock refuges, greenhouse cultivations, clean-up operations and tephra deposit stabilisation.
Data Availability Statement
The original contributions presented in the study are included in the article/Supplementary Material, further inquiries can be directed to the corresponding author.
Author Contributions
All authors attended the 2019 workshop on Wind-remobilisation processes of volcanic ash in San Carlos de Bariloche and Ingeniero Jacobacci, Argentina. CB, LD, CF, PJ, PF, and DB were members of the workshop organizing committee. PJ drafted the manuscript and all authors contributed to the content, style and structure.
Funding
The workshop (Argentina) was funded by the Swiss National Science Foundation (#200021_163152), the University of Geneva and the Argentinian National Institute of Agricultural Technology.
Conflict of Interest
The authors declare that the research was conducted in the absence of any commercial or financial relationships that could be constructed as a potential conflict of interest.
The reviewer (SB) declared a past co-authorship with one of the authors (CB) to the handling editor. The reviewer (TD) declared a past co-authorship with one of the authors (FB) to the handling editor.
Acknowledgments
Workshop participants are thanked for their stimulating discussions and valuable contributions during the workshop. The Argentinian National Institute of Agricultural Technology are thanked for logistical support. Matthew Hort, David Schneider, Hans Schwaiger and Michael Diggles, along with the editor, James White, and reviewers, Sara Barsotti, Tobias Dürig and Valerio Acocella, are thanked for comments that improved the manuscript.
Supplementary Material
The Supplementary Material for this article can be found online at: https://www.frontiersin.org/articles/10.3389/feart.2020.575184/full#supplementary-material
References
Alexander, A. E. (1934). The dustfall of November 13, 1933 at Buffalo, New York. J. Sediment. Petrol. 4 (2), 81–82. doi:10.1175/1520-0493(1934)62<15:POTGDO>2.0.CO;2
Algeo, J., Slater, L., Binley, A., Van Dam, R. L., and Watts, C. (2018). A comparison of ground-penetrating radar early-time signal approaches for mapping changes in shallow soil water content. Vadose Zone J. 17 (1), 1–11. doi:10.2136/vzj2018.01.0001
Arnalds, O., Thorarinsdottir, E. F., Thorsson, J., Waldhauserova, P. D., and Agustsdottir, A. M. (2013). An extreme wind erosion event of the fresh Eyjafjallajökull 2010 volcanic ash. Sci. Rep. 3, 1257. doi:10.1038/srep01257
Baas, A. C. W., and Sherman, D. J. (2005). Formation and behaviour of aeolian streamers. J. Geophys. Res. Earth Surf. 110 (F3), F03011. doi:10.1029/2004JF000270
Balme, M., and Greeley, R. (2006). Dust devils on Earth and Mars. Rev. Geophys. 44 (3), RG3003. doi:10.1029/2005RG000188
Banks, R. F., Tiana-Alsina, J., Baldasano, J. M., Rocadenbosch, F., Papayannis, A., Solomos, S., et al. (2016). Sensitivity of boundary-layer variables to PBL schemes in the WRF model based on surface meteorological observations, lidar and radiosondes during the HygraA-CD campaign. Atmos. Res. 176–177, 185–201. doi:10.1016/j.atmosres.2016.02.024
Bauer, B. O., and Davidson-Arnott, R. G. D. (2014). Aeolian particle flux profiles and transport unsteadiness. J. Geophys. Res. Solid Earth 119, 1542–1563. doi:10.1002/2014JF003128
Baxter, P. J., and Horwell, C. J. (2015). “Impacts of eruptions on human health,” in The encyclopedia of volcanoes. Cambridge, MA: Academic Press, 1035–1046.
Beckett, K., Kylling, A., Sigurðardóttir, G., von Löwis, S., and Witham, C. (2017). Quantifying the mass loading of particles in an ash cloud remobilized from tephra deposits on Iceland. Atmos. Chem. Phys. 17, 4401–4418. doi:10.5194/acp-17-4401-2017
Bonadonna, C., Jarvis, P. A., Dominguez, L., Frischnecht, C., Forte, P., Bran, D., et al. (2020). Workshop on wind-remobilisation processes of volcanic ash, consensual document. Available at: https://vhub.org/resources/4602 (Accessed February 19, 2020).
Butwin, M. K., Pfeffer, M. A., von Löwis, S., Støren, E. W. N., Bali, E., and Thorsteinsson, T. (2020). Properties of dust source material and volcanic ash in Iceland. Sedimentology 67, 3067–3087. doi:10.1111/sed.12734
Butwin, M. K., von Löwis, S., Pfeffer, M. A., and Thorsteinsson, T. (2019). The effects of volcanic eruptions on the frequency of particulate matter suspension events in Iceland. J. Aerosol Sci. 128, 99–113. doi:10.1016/j.jaerosci.2018.12.004
Craig, H., Wilson, T., Stewart, C., Outes, V., Villarosa, G., and Baxter, P. (2016a). Impacts to agriculture and critical infrastructure in Argentina after ashfall from the 2011 eruption of the Cordón Caulle volcanic complex: as assessment of published damage and function thresholds. J. Appl. Volc. 5, 7. doi:10.1186/s13617-016-0046-1
Craig, H., Wilson, T., Stewart, C., Villarosa, G., Outes, V., Cronin, S., et al. (2016b). Agricultural impact assessment and management after three widespread tephra falls in Patagonia, South America. Nat. Hazards 82 (2), 1167–1229. doi:10.1007/s11069-016-2240-1
Cronin, S. J., Beall, V. E., Lecointre, J. A., Headley, M. J., and Loganathan, P. (2003). Environmental hazards of fluoride in volcanic ash: a case study from Ruapehu volcano, New Zealand. J. Volcanol. Geotherm. Res. 121 (3–4), 271–291. doi:10.1016/S0377-0273(02)00465-1
Del Bello, E., Taddeucci, J., Merrison, J. P., Alois, S., Iversen, J. J., and Scarlato, P. (2018). Experimental simulations of volcanic ash resuspension by wind under the effects of atmospheric humidity. Sci. Rep. 8 (1), 14509. doi:10.1038/s41598-018-32807-2
Dominguez, L., Bonadonna, C., Forte, P., Jarvis, P. A., Cioni, R., Mingari, L., et al. (2020). Aeolian remobilisation of the 2011-Cordón Caulle Tephra-fallout deposit: example of an important process in the life cycle of volcanic ash. Front. Earth Sci. 7, 343. doi:10.3389/feart.2019.00343
Douillet, G. A., Rasmussen, K. R., Kueppers, U., Lo Castro, D., Merrison, J. P., Iversen, J. J., and Dingwell, D. B. (2014). Saltation threshold for pyroclasts at various bedslopes. J. Volcanol. Geotherm. Res., 278-279, 14–24. doi:10.1016/j.jvolgeores.2014.03.011
Elissondo, M., Baumann, V., Bonadonna, C., Pistolesi, M., Cioni, R., Bertagnini, A., et al. (2016). Chronology and impact of the 2011 Cordón Caulle eruption, Chile. Nat. Hazards Earth Syst. Sci. 16, 675–704. 10.5194/nhess-16-675-2016
Etyemezian, V., Gillies, J. A., Mastin, L. G., Crawford, A., Hasson, R., Van Eaton, A. R., et al. (2019). Laboratory experiments of volcanic ash resuspension by wind. J. Geophys. Res. 124 (16), 9534–9560. doi:10.1029/2018JD030076
Etyemezian, V., Nikolich, G., Ahonen, S., Pitchford, M., Sweeney, M., Purcell, R., et al. (2007). The portable in situ wind erosion laboratory (PI-SWERL): a new method to measure PM10 windblown dust properties and potential for emissions. Atmos. Environ. 41 (18), 3789–3796. doi:10.1016/j.atmosenv.2007.01.018
Fierstein, J., and Hildreth, W. (1992). The plinian eruptions of 1912 at Novarupta, Katmai national park, Alaska. Bull. Volcanol. 54 (8), 646–684. doi:10.1007/BF00430778
Folch, A., Mingari, L., Osores, M. S., and Colini, E. (2014). Modeling volcanic ash resuspension—application to the 14–18 October 2011 outbreak episode in central Patagonia, Argentina. Nat. Hazards Earth Syst. Sci. 14 (1), 119–133. doi:10.5194/nhess-14-119-2014
Forte, P., Domínguez, L., Bonadonna, C., Gregg, C. E., Bran, D., Bird, D., et al. (2018). Ash resuspension related to the 2011–2012 Cordón Caulle eruption, Chile, in a rural community of Patagonia, Argentina. J. Volcanol. Geotherm. Res. 350, 18–32. doi:10.1016/j.jvolgeores.2017.11.021
Fries, H., and Yadigaroglu, G. (2002). Modelling of the resuspension of particle clusters from multilayer aerosol deposits with variable porosity. J. Aerosol Sci. 33 (6), 883–906. doi:10.1016/S0021-8502(02)00049-6
Gillette, D. A., Blifford, I. H., and Fryrear, D. W. (1974). The influence of wind velocity on the size distributions of aerosols generated by the wind erosion of soils. J. Geophys. Res. 79 (27), 4068–4075. doi:10.1029/JC079i027p04068
Gillies, J. A., Nickling, W. G., and King, J. (2002). Drag coefficient and plant form response to wind speed in three plant species: burning bush (Euonymus alatus), Colorado Blue Spruce (Picea pungens glauca.), and Fountain Grass (Pennisetum setaceum). J. Geophys. Res. Atmos. 107 (D24), 4760. doi:10.1029/2001JD001259
Greeley, R., Blumberg, D. G., and Williams, S. H. (1996). Field measurements of the flux and speed of wind-blown sand. Sedimentology 43 (1), 41–52. doi:10.1111/j.1365-3091.1996.tb01458.x
Greeley, R., and Iversen, J. D. (1985). Wind as a geological process on Earth, Mars, Venus and Titan. New York: Cambridge University Press.
Hadley, D., Hufford, G. L., and Simpson, J. J. (2004). Resuspension of relic volcanic ash and dust from Katmai: still an aviation hazard. Weather Forecast. 19 (5), 829–840. doi:10.1175/1520-0434(2004)019%3C0829:RORVAA%3E2.0.CO;2
Hammond, K., and Beckett, F. (2019). Forecasting resuspended ash clouds in Iceland at the London VAAC. Weather 74 (5), 167–171. doi:10.1002/wea.3398
Hobbs, P. V., Hegg, D. A., and Radke, L. F. (1983). Resuspension of volcanic ash from Mount St. Helens. J. Geophys. Res. Oceans 88 (C6), 3919–3921. doi:10.1029/JC088iC06p03919
Ishizuka, M., Mikami, M., Leys, J. F., Shao, Y., Yamada, Y., and Heidenreich, S. (2014). Power law relation between size-resolved vertical dust flux and friction velocity measured in a fallow wheat field. Aeolian Res. 12, 87–99. doi:10.1016/j.2013.11.002aeolia
Kok, J. F., Parteli, E. J. R., Michaels, T. I., and Karam, D. B. (2012). The physics of wind-blown sand and dust. Rep. Prog. Phys. 75 (10), 106901. doi:10.1088/0034-4885/75/10/106901
Kueppers, U., Cimarelli, C., Hess, K. U., Taddeucci, J., Wadsworth, F. B., and Dingwell, D. B. (2014). The thermal stability of Eyjafjallajökull ash versus turbine engine test sands. J. Appl. Volc. 3, 4. doi:10.1186/2191-5040-3-4
Leadbetter, S. J., Hort, M. C., von Löwis, S., Weber, K., and Witham, C, S. (2012). Modeling the resuspension of ash deposited during the eruption of Eyjafjallajökull in spring 2010. J. Geophys. Res. 117 (D20). doi:10.1029/2011JD016802
Liu, E. J., Cashman, K. V., Beckett, F. M., Witham, C. S., Leadbetter, S. J., Hort, M. C., et al. (2014). Ash mists and brown snow: remobilization of volcanic ash from recent Icelandic eruptions. J. Geophys. Res. Atmos. 119 (15), 9463–9480. doi:10.1002/2014JD021598
Marticorena, B., Bergametti, G., Gillette, D., and Belnap, J. (1997). Factors controlling threshold friction velocity in semiarid and arid areas of the United States. J. Geophys. Res. Atmos. 102 (D19), 23277–23287. doi:10.1029/97JD01303
McKenna Neuman, C., and Sanderson, S. (2008). Humidity control of particle emissions in aeolian systems. J. Geophys. Res. Earth Surf. 113 (F2), F02S14. doi:10.1029/2007JF000780
Mendez, M. J., Funk, R., and Buschiazzo, D. E. (2011). Field wind erosion measurements with Big 424 spring Number Eight (BSNE) and Modified Wilson and Cook (MWAC) samplers. Geomorphology 425 129 (1-2), 43–48. 10.1016/j.geomorph.2011.01.011
Miller, E. R. (1934). Meteorology of the dustfall of November 12–13, 1933. J. Sediment. Petrol. 4, 78–81. 10.1175/1520-0493(1934)62<15:POTGDO>2.0.CO;2
Mingari, L. M., Collini, E. A., Folch, A., Wolter, B., Bustos, E., Osores, M. S., et al. (2017). Numerical simulations of windblown dust over complex terrain: the Fiambalá episode in June 2015. Atmos. Chem. Phys. 17 (11), 6759–6778. doi:10.5194/acp-17-6759-2017
Mingari, L. M., Folch, A., Dominguez, L., and Bonadonna, C. (2020). Volcanic ash resuspension in Patagonia: numerical simulations and observations. Atmosphere 11, 977. doi:10.3390/atmos11090977
Müller, D., Kueppers, U., Hess, K. U., Song, W., and Dingwell, D. B. (2019). Mineralogical and thermal characterisation of a volcanic ash: implications for turbine interactions. J. Volcanol. Geotherm. Res. 377, 43–52. doi:10.1016/j.jvolgeores.2019.04.005
NDRRMC (2020). Situational report No. 33. Re taal volcano eruption. republic of the Philippines national disaster risk reduction and management council. Available at: http://www.ndrrmc.gov.ph/attachments/article/4007/Update_re_Stiuational_Report_No_33_re_Taal_Volcano_Eruption_6PM.pdf (Accessed January 22, 2020).
Nickling, W. G., and McKenna Neuman, C. (2009). “Aeolian sediment transport,” in Geomorphology of desert environments. Dordrecht, Nertherlands: Spring. 517–555.
Panebianco, J. E., Mendez, M. J., Buschiazzo, D. E., Bran, D., and Gaitán, J. J. (2017). Dynamics of volcanic ash remobilisation by wind through the Patagonian steppe after the eruption of Cordón Caulle, 2011. Sci. Rep. 7, 45529. 10.1038/srep45529
Pavolonis, M. J., Sieglaff, J., and Cinteneo, J. (2015a). Spectrally enhanced cloud objects—a generalized framework for automated detection of volcanic ash and dust clouds using passive satellite measurements: 1. Multispectral analysis. J. Geophys. Res. Atmos. 120, 7813–7841. doi:10.1002/2014JD022968
Pavolonis, M. J., Sieglaff, J., and Cinteneo, J. (2015b). Spectrally enhanced cloud objects – a generalized framework for automated detection of volcanic ash and dust clouds using passive satellite measurements: 2. Cloud object analysis and global application. J. Geophys. Res. Atmos. 120, 7842–7870. doi:10.1002/2014JD022969
Pavolonis, M. J., Sieglaff, J., and Cinteneo, J. (2018). Automated detection of explosive volcanic eruptions using satellite-derived cloud vertical growth rates. Earth Space Sci 5, 903–928. doi:10.1029/2018EA000410
Petropoulos, G. P., Ireland, G., and Barrett, B. (2015). Surface soil moisture retrievals from remote sensing: current status, products and future trends. Phys. Chem. Earth 83–84, 36–56. doi:10.1016/j.pce.2015.02.009
Pianosi, F., Bevan, K., Freer, J., Hall, J. W., Rougier, J., Stephenson, D. B., et al. (2016). Sensitivity analysis of environmental models: a systematic review with practical workflow. Environ. Model. Software 79, 214–232. doi:10.1016/j.envsoft.2016.02.008
Prandtl, L. (1935). “The mechanics of viscous fluids,” in Aerodynamic theory. Editor Durand, F. (Berlin: Springer-Verlag), 57–100.
Prata, A. J. (1989). Observations of volcanic ash clouds in the 10–12 μm window using AVHRR/2 data. Int. J. Rem. Sens. 10 (4–5), 751–761. doi:10.1080/01431168908903916
Pyle, D. M., Ricketts, G. D., Margari, V., van Andel, T. H., Sinitsyn, A., Praslov, N. D., et al. (2006). Wide dispersal and deposition of distal tephra during the Pleistocene “Campanian Ignimbrite/Y5” eruption. Italy Quat. Sci. 21–22, 2713–2728. doi:10.1016/j.quascirev.2006.06.008
Reckziegel, F., Bustos, E., Mingari, L., Báez, W., Villarosa, G., Folch, A., et al. (2016). Forecasting volcanic ash dispersal and coeval resuspension during the April–May 2015 Calbuco eruption. J. Volcanol. Geotherm. Res. 321, 44–57. doi:10.1016/j.jvolgeores.2016.04.033
Schmehl, K. J., Haupt, S. E., and Pavolonis, M. J. (2011). A genetic algorithm variation approach to data assimilation and application to volcanic emissions. Pure Appl. Geophys. 169, 519–537. 10.1007/s00024-011-0385-0
Shao, Y., and Lu, H. (2000). A simple expression for wind erosion threshold friction velocity. J. Geophys. Res. Atmos. 105 (D17), 22437–22443. 10.1029/2000JD900304
Shao, Y., Raupach, M. R., and Findlater, P. A. (1993). Effect of saltation bombardment on the entrainment of dust by wind. J. Geophys. Res. Atmos. 98 (D7), 12719–12726. 10.1029/93JD00396
Spanu, A., Dollner, M., Gasteiger, J., Bui, T. P., and Weinzierl, B. (2020). Flow-induced errors in airborne in-situ measurements of aerosols and clouds. Atmos. Meas. Tech. 13 (4), 1963–1987. doi:10.5194/amt-13-1963-2020
Su, S. L., Singh, D. N., and Baghini, M. S. (2014). A critical review of soil moisture measurement. Measurement 54, 92–105. doi:10.1016/j.measurement.2014.04.007
Thorsteinsson, T., Jóhannsson, T., Stohl, A., and Kristiansen, N. I. (2012). High levels of particulate matter in Iceland due to direct ash emissions by the Eyjafjallajökull eruption and resuspension of deposited ash. J. Geophys. Res. Solid Earth 117 (B9), B00C05. doi:10.1029/2011JB008756
Toyos, G., Mingari, L., Pujol, G., and Villarosa, G. (2017). Investigating the nature of an ash cloud event in Southern Chile using remote sensing: volcanic eruption of resuspension. Remote Sens. Lett. 8 (2), 146–155. doi:10.1080/2150704X.2016.1239281
Ungar, J. E., and Haff, P. K. (1987). Steady state saltation in air. Sedimentology 34 (2), 289–299. doi:10.1111/j.1365-3091.1987.tb00778
Watson, I. M., Realmuto, V. J., Rose, W. I., Prata, A. J., Bluth, G. J. S., Bader, C. E., et al. (2004). Thermal infrared remote sensing of volcanic emissions using the moderate resolution imaging spectroradiometer. J. Volcanol. Geotherm. Res. 135 (1–2), 75–89. doi:10.1016/j.jvolgeores.2003.12.017
Weinzierl, B., Sauer, D., Minikin, A., Reitebuch, O., Dahlkötter, F., Mayer, B., et al. (2012). Phys. Chem. Earth 45–46, 87–102. doi:10.1016/j.pce.2012.04.003
Wilson, T., Stewart, C., Bickerton, H., Baxter, P., Outes, V., Villarosa, G., and Rovere, E. (2013). GNS Science Report 2012/20. Impacts of the June 2011 Puyehue-Cordón Caulle volcanic complex eruption on urban infrastructure, agriculture and public health. 88. Available at: http://hdl.handle.net/11336/78121 (Accessed January 1, 2013).
Wilson, T. M., Cole, J. W., Stewart, C., Cronin, S. J., and Johnston, D. M. (2011). Ash storms: impacts of wind-remobilised volcanic ash on rural communities and agriculture following the 1991 Hudson eruption, southern Patagonia, Chile. Bull. Volcanol. 73 (3), 223–239. doi:10.1007/s00445-010-0396-1
WMO (2016). WMO VAAC “best practice” workshop 2016: final report. Buenos Aires, Argentina. Available at: https://www.wmo.int/aemp/sites/default/files/VAAC_BP_2016_Report_v05_final.pdf (Accessed August 23, 2017).
Keywords: aeolian processes, volcanic ash, ash remobilisation, field geology, modeling, hazard monitoring, ash resuspension
Citation: Jarvis PA, Bonadonna C, Dominguez L, Forte P, Frischknecht C, Bran D, Aguilar R, Beckett F, Elissondo M, Gillies J, Kueppers U, Merrison J, Varley N and Wallace KL (2020) Aeolian Remobilisation of Volcanic Ash: Outcomes of a Workshop in the Argentinian Patagonia. Front. Earth Sci. 8:. doi: 10.3389/feart.2020.575184
Received: 22 June 2020; Accepted: 29 October 2020;
Published: 27 November 2020.
Edited by:
James D. L. White, University of Otago, New ZealandReviewed by:
Sara Barsotti, Icelandic Meteorological Office, IcelandTobias Dürig, University of Iceland, Iceland
Valerio Acocella, Roma Tre University, Italy
Copyright © 2020 Jarvis, Bonadonna, Dominguez, Forte, Frischknecht, Bran, Aguilar, Beckett, Elissondo, Gillies, Kueppers, Merrison, Varley and Wallace. This is an open-access article distributed under the terms of the Creative Commons Attribution License (CC BY). The use, distribution or reproduction in other forums is permitted, provided the original author(s) and the copyright owner(s) are credited and that the original publication in this journal is cited, in accordance with accepted academic practice. No use, distribution or reproduction is permitted which does not comply with these terms.
*Correspondence: Paul A. Jarvis, cGF1bC5qYXJ2aXNAdW5pZ2UuY2g=