- 1UMR 6112 LPG-BIAF, Université d'Angers, Université de Nantes, CNRS, Angers, France
- 2Department of Ocean Systems, NIOZ-Royal Netherlands Institute for Sea Research and Utrecht University, Den Burg, Netherlands
- 3Faculty of Geosciences, Utrecht University, Utrecht, Netherlands
Element concentrations of calcite precipitated by foraminifera reflect chemical and physical properties of seawater and can therefore be used to reconstruct (paleo-)environmental conditions. Foraminiferal carbonate associated manganese incorporation (expressed here as Mn/Ca) is a potential proxy for seawater oxygenation, although the impacts of other environmental parameters need to be quantified before Mn/Ca can be robustly applied. Here we report the isolated impact of seawater carbonate chemistry on manganese incorporation in the shells of two large symbiont-bearing benthic foraminiferal species. Moreover, we investigated the role of biomineralization on manganese incorporation by using species with contrasting calcification pathways: the hyaline species Amphistegina gibbosa and the porcelaneous species Sorites marginalis. Furthermore, analyzing shells from a wide range of species grown under identical conditions allowed assessment of species-specific Mn incorporation in other foraminiferal species. Our observations show that species specific differences in biomineralization strategies are the dominant factor determining Mn content. Shells from porcelaneous species, with relatively high Mg contents, are generally also enriched in Mn compared to low-Mg/Ca foraminifera. Superimposed on the effect of biomineralization, chemical speciation of elements in seawater as a function of pCO2 also affects their incorporation. Whereas the impact of the carbonate system is limited, the inter-specific differences call for species specific calibrations in order to use Mn uptake as a (paleo-)oxygenation proxy.
Introduction
The chemical composition of foraminiferal shells is widely used by paleoceanographers to reconstruct ocean conditions, because past fluctuations in physico-chemical conditions of seawater are recorded in the chemistry of the shell. For example, the Mg–Ca ratio (Mg/Ca) of foraminiferal calcite reflects seawater temperature (Nürnberg et al., 1996) and can hence be applied to fossil foraminifera to reconstruct past bottom water (Lear et al., 2000) and sea surface (Barker et al., 2005) temperatures. The toolbox that foraminiferal calcite provides, is ever expanding by addition of new elements of interest, including e.g., the recently proposed Sr/Ca (Keul et al., 2017) and S/Ca (Van Dijk et al., 2017a) as recorders of the seawater carbonate system. In addition, redox sensitive trace metals may serve as proxies to reconstruct (paleo-)oxygenation, a parameter for which accurate reconstruction methods are currently lacking. Manganese (Mn) is a promising candidate for such a proxy, since solubility and/or the oxidation state of Mn is a function of the redox status of the environment (Tribovillard et al., 2006) and pore water carbonate chemistry (Middelburg et al., 1987). Manganese precipitates as a solid phase Mn oxyhydroxide under oxygenated conditions, but at lower oxygen levels, Mn oxyhydroxide is reduced and Mn2+ is released into the surrounding seawater. At high carbonate concentrations in the pore water, Mn2+ is removed by precipitation of Mn carbonate (rhodochrosite). Foraminifera incorporate dissolved Mn2+ (Reichart et al., 2003; Koho et al., 2015; Barras et al., 2018) and therefore the Mn/Ca of their shell is suggested to change with oxygenation conditions (Groeneveld and Filipsson, 2013; Koho et al., 2015; McKay et al., 2015; Ní Fhlaithearta et al., 2018). To further develop this proxy, it is necessary to i) investigate potential other (environmental) parameters influencing Mn incorporation and ii) obtain species-specific calibrations by culture or field studies, and ultimately iii) understand the incorporation pathways of Mn during biomineralization.
The influence of the carbonate system (e.g., pH, alkalinity, dissolved inorganic carbon) on foraminiferal Mn/Ca has, to our knowledge, not yet been studied. In theory, the carbonate system might have an effect on the Mn/Ca of foraminifera, since it is hypothesized that absorption layers might be involved in the incorporation of Mn (Barras et al., 2018) and adsorption on inorganically precipitated calcite depends on pH (Zachara et al., 1991). At higher pH, adsorption of Mn2+ might increase, which would result in overall higher foraminiferal Mn/Ca values. For the small benthic foraminifers Ammonia sp. and Bulimina marginata, thin bands with high Mn/Ca are observed at the start of calcite lamellae (Van Dijk et al., 2019b), which could be due to absorption layers that are exposed to the surrounding seawater between chamber formation events, as hypothesized in Barras et al. (2018). These high Mn/Ca layers are co-located with high Mg/Ca banding and are associated with a carbonate-associated species of Mn (Van Dijk et al., 2019b). Other processes related to seawater carbonate chemistry could also impact element incorporation (Ries et al., 2009). In inorganic calcite, precipitation rate controls incorporation of elements (Mucci, 1987). For Mn, a decreasing precipitation rate increases Mn partitioning (DMn = Mn/CaCALCITE/Mn/CaSEAWATER) and thus favors incorporation of Mn (Lorens, 1981). This is in contrast to Mg and Sr partitioning, which increases with calcite growth rate (Mavromatis et al., 2013). Consequently, when calcite precipitation rates in foraminifera are affected by e.g., ocean acidification or inorganic carbon chemistry in general, the effect on Mn- and Mg-incorporation is expected to be reversed. Unfortunately, so far the response of foraminifera to e.g., ocean acidification seems to be species-specific (Keul et al., 2013; Doo et al., 2014) making it difficult to predict overall effect of acidification on element incorporation.
As with other elements, Mn incorporation may well be species-specific, which would require calibration of this proxy for different species (e.g., see summary in Toyofuku et al., 2011). Barras et al. (2018) found that the two benthic species Ammonia tepida and Bulimina marginata, cultured under the same conditions, have a Mn partitioning of 0.08 and 0.6, respectively, an offset of one order of magnitude. Incorporation of Mn appears to be coupled to that of Mg (Van Dijk et al., 2019b), similar to what is observed for other elements (Van Dijk et al., 2017b), possibly due to crystal lattice strain (Mucci and Morse, 1983; Evans et al., 2015) or simultaneous uptake (Van Dijk et al., 2019a). Even if the relative sensitivity of Mn/Ca to oxygenation is the same for different species, as suggested for certain proxies (e.g., S/Ca; Van Dijk et al., 2017a), the absolute Mn/Ca value is still needed for reconstruction of oxygenation. Therefore, Mn/Ca values of both hyaline and porcelaneous species have to be known before application of this potential oxygenation proxy.
However, there is no consensus on key processes involved in foraminiferal calcification (review in De Nooijer et al., 2014). Uptake of ions could occur for instance through seawater endocytosis (seawater vacuolization model; Erez, 2003; Bentov et al., 2009) or transmembrane transport (Nehrke et al., 2013; Mewes et al., 2015), and is either precipitated from a closed reservoir (Elderfield et al., 1996; Evans et al., 2018) or by a continuous ion supply where precipitation follows a classical or a non-classical pathway (Jacob et al., 2017). These models do not specify how Mn is taken up from seawater by the organism and how it is subsequently incorporated into foraminiferal calcite. According to the seawater vacuolization model model, Mn is transported in a seawater-filled vacuole to the site of calcification (SOC). Whether Mn is actively removed, like is hypothesized for Mg (Bentov and Erez, 2006), remains to be investigated. In the transmembrane transport model, Ca is transported through transmembrane channels, which may occasionally transport other cations with a radius close to the Ca2+, like Zn2+ and Cd2+ (Gonçalves et al., 1999). This would also be the case for Mn2+ ions, since the ionic radius of Mn2+ (0.8 Å) is similar to that of Ca2+(1.0 Å). Based on culture evidence, Barras et al. (2018) hypothesize that the incorporated Mn in the foraminiferal shell might be partly related to an organismal calcification pathway (biological incorporation), as discussed above, in combination with an inorganic pathway (abiological incorporation). This inorganic pathway involves adsorption of Mn ions on specific sites on the exposed outer surface of the calcite shells (Mucci and Morse, 1983) in between chamber formation events. Clearly, further research is needed to understand Mn pathways and create a fundamental basis to explain the empirical relation between foraminifera Mn and the concentration of dissolved oxygen and seawater [Mn].
In this study we address the major issues mentioned above by investigating the effect of seawater carbonate chemistry on shell Mn/Ca values. Observations are used to evaluate the proposed possible pathways of Mn during foraminiferal calcification. We investigated single-chamber Mn/Ca values from a controlled culture experiment with two larger benthic species of foraminifera, Amphistegina gibbosa hosting diatom symbionts (Lee et al., 1995), which precipitates a hyaline shell with intermediate Mg/Ca (∼2% wt Mg) and porcelaneous species Sorites marginalis, which calcifies a shell with high Mg content (>4% wt Mg) and host dinoflagellates (Müller-Merz and Lee, 1976). Specimens were cultured in one of four different conditions, with a pCO2 of 350, 450, 760, or 1,200 ppm, to study the effect of the carbonate system on the incorporation of Mn in foraminiferal calcite. In addition, we analyzed a wide range of species sampled from an Indo-Pacific coral reef aquarium (Ernst et al., 2011) to study species-specific partitioning in hyaline and porcelaneous larger benthic foraminifera from a controlled environment.
Methods
Foraminiferal Samples
In this study we analyzed specimens of two species of foraminifera, A. gibbosa and S. marginalis grown under a range of controlled pCO2, as well as larger benthic foraminifera collected from an Indo-Pacific reef aquarium. The experimental design of the culture experiment under different pCO2 has been described in detail in Van Dijk et al. (2017b). In short, different species of larger benthic foraminifera collected in the Caribbean Sea were incubated under controlled conditions at the Caribbean Netherlands Science Institute (CNSI; St. Eustatius). Foraminifera were cultured in groups in 70 ml Falcon® tissue bottles in four batches of seawater with added Calcein (5 mg/l), which were in equilibrium with four different pCO2 (350, 450, 760, and 1,200 ppm named, respectively, treatment A, B, C, and D) by a pCO2-control system developed in-house (described in detail in Webb et al., 2017). Culture media was replaced every 4 days. At the start and termination of the experiment, 125 ml samples from the stock seawater solutions (one for each experimental treatment) were collected to analyze the concentration of dissolved inorganic carbon and total alkalinity on a Versatile INstrument for the Determination of Titration Alkalinity at the CNSI. Physico-chemical parameters of the culture conditions can be found in Table 1. Foraminifera were cultured at 25 ± 0.2°C for 21 days, during which they build on average 2.4 and 5.0 chambers for A. gibbosa and S. marginalis, respectively. After termination of the experiment, specimens were rinsed three times with de-ionized water and dried at 40°C. Foraminiferal samples were transported in slides to the Royal Netherlands Institute for Sea Research (NIOZ) to investigate the Mn/Ca of the shell and growth parameters. To assess the number of chambers added during the experiment, fluorescent calcein-stained chambers were counted using a ZEISS Axioplan 2 fluorescence microscope equipped with appropriate excitation and emission optics. Pictures were taken using a ZEISS Axiocam MRc 5 camera.
At Burgers’ Zoo in Arnhem, Netherlands, coral debris, rich in foraminifera (Ernst et al., 2011) were collected from the Indo-Pacific coral reef aquarium, one of the largest coral reef aquaria in the world. From these coral debris, hyaline (Amphistegina lessonii and Heterostegina depressa) and porcelaneous (Sorites orbiculus, Spiroculina angulata, Spiroculina communis, Quinqueloculina pseudoreticulata, Quinqueloculina sp.) species of foraminifera were isolated to analyze the shell chemistry. Foraminifera from both the experiment and the aquarium were cleaned following an adapted version of the Barker protocol (Barker et al., 2003), described in Van Dijk et al. (2017a, 2017b). In short, foraminifera were cleaned using an oxidizing step in which organics were removed with a 1% H2O2 solution (buffered with 0.1 M NH4OH), and consequently, after gentle ultrasonication, rinsed with ultrapure water and dried in a laminar flow cabinet.
Foraminiferal Carbonate Analyses
Laser Ablation-Inductively Coupled Plasma Mass Spectrometry
Elemental composition of individual fluorescent chambers of cultured specimens was analyzed by laser ablation-inductively coupled plasma mass spectrometry (ICP-MS) (Reichart et al., 2003; Van Dijk et al., 2017b). In short, the laser system comprising of an ArF Excimer laser (Existar) with deep UV 193 nm wavelength and <4 ns pulse duration (NWR193UC, New Wave Research) was equipped with a Two Volume 2 cell (New Wave Research), characterized by a wash-out time of 1.8 s (1% level). Single chambers were ablated in a helium environment using a circular laser spot with a diameter of 80 μm (S. marginalis) and 60 μm (A. gibbosa). Foraminifera were previously analyzed (Van Dijk et al., 2017b), but at that time Mn could not be included as an analyte due to interferences with ArN due to the addition of N2 to obtain better counting statistics for e.g., Na. Whenever possible, we re-ablated all calcein-stained chambers one to three times. All foraminiferal samples were ablated with an energy density of 1.0 ± 0.1 J/cm2 and a repetition rate of 6 Hz. The resulting aerosol was transported with a helium/argon flow to the quadrupole ICP-MS (iCAP Q, Thermo Scientific). Other monitored masses included 7Li, 23Na, 24Mg, 25Mg, 27Al, 43Ca, 44Ca, 55Mn, 88Sr, and 137Ba.
At the start of each series we analyzed several carbonate standards, including JCt-1 (coral carbonate) and two in-house standards, namely NFHS (NIOZ Foraminifera House Standard; Mezger et al., 2016) as well as an Iceland spar NCHS (NIOZ Calcite House Standard). At the end of each series we analyzed SRM NIST612 and NIST610 glass standard in triplicate (using an energy density of 5.0 ± 0.1 J/cm2). We further analyzed JCp-1 (coral, Porites sp.; Okai et al., 2002) and MACS-3 (synthetic calcium carbonate) at the start of each series, and to monitor drift after every ten samples. All element to calcium ratios were calculated with an adapted version of the MATLAB based program Signal Integration for Laboratory Laser Systems (Guillong et al., 2008). Signal Integration for Laboratory Laser Systems was modified by NIOZ to evaluate LA-ICP-MS measurements on foraminifera, allowing import of Thermo Qtegra software sample list, laser data reduction and laser LOG files (as described in Mezger et al., 2016; Van Dijk et al., 2017b). Some major adaptions include improved automated integration and evaluation of (calibration and monitor) standards, quality control report of the monitor standards and export in element to calcium ratios (mmol/mol). Integration profiles were manually selected for evaluation, by e.g., monitoring decrease in Ca counts and the Al signal, which can be used as a sign of potential contamination or diagenesis of the outer or inner layer of calcite. Calibration was performed against the MACS-3 carbonate standard, with 43Ca as an internal standard and the multiple measurements of MACS-3 were used to apply a linear drift correction. Relative analytical precision, expressed as the relative standard deviation (RSD) of all MACS-3 analyses, is 3% for 7Li/Ca, 3% 23Na/Ca, 3% for 24Mg/Ca, 3% for 25Mg/Ca, 2% for 55Mn/Ca, 3% for 88Sr/Ca, and 3% for 137Ba/Ca. Accuracy, based on values for JCp-1 standardized to MACS-3, was 95 and 93% for Mg/Ca and Mn/Ca, respectively.
In total, 193 and 150 analyses were performed on A. gibbosa and S. marginalis, respectively (see Supplementary Material Data Sheet). For details on the amount of specimens and analysis per species, see Table 2. We removed outliers, 13 in total, from the database based on 1.5 × interquartile range of Mn/Ca. Furthermore, we calculated the average Mn/Ca and Mg/Ca per species per treatment and partition coefficient between seawater and foraminiferal calcite, D, which is expressed as DE = (E/CaCALCITE)/(E/CaSW), in which E is the element of interest. Since these foraminifera were previously analyzed, we also compare our new data with the Mg/Ca data of a previous study (Van Dijk et al., 2017b).
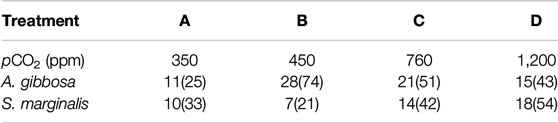
TABLE 2. Summary of the number of specimens and ablations (in brackets) per species per condition (Treatment A, B, C and D) analyzed by LA-Q-ICP-MS.
Sector Field-Inductively Coupled Plasma Mass Spectrometry
Foraminifera from Burgers’ Zoo were grouped per species (A. lessonii, Heterostegina depressa, Sorites orbiculus, Spiroculina angulate, Spiroloculina communis, Quinqueloculina pseudoreticulata, and Quinqueloculina sp.) and dissolved in 0.5 ml 0.1 M HNO3. A 5 s pre-scan for 43Ca was performed on an Element 2 sector field double focusing mass spectrometer (SF-ICP-MS) to determine the [Ca] in the dissolved foraminiferal calcite solutions. According to these results, samples were diluted to 100 ppm Ca. Elemental composition of the foraminifera was measured for a wide range of isotopes, including 24Mg, 55Mn, 43Ca at medium resolution with a 300 ml/min flowrate using a peristaltic pump. Samples were measured against six ratio calibration standards with similar matrix. In addition to the foraminiferal samples, we measured several standards to monitor drift and the quality of the analyses, including NFHS (NIOZ Foraminifera House Standard; for details see Mezger et al., 2016), JCt-1 (Giant Clam, Tridacna gigas) and JCp-1 (coral, Porites sp.; Okai et al., 2002). One of the ratio calibration standards was measured after every fifth sample to monitor drift. Accuracy of Mg/Ca is 105 and 101% for JCt-1 and JCp-1, respectively, with an external precision of 0.4% for both standards. For Mn/Ca, only MACS-3 has a certified value, and accuracy of our measurements is 101% when using this standard.
Seawater Measurements
The sampling strategy and general seawater composition (Ca, Mg, Na, Sr and Ba) of the media used in the pCO2 controlled experiment has been described in Van Dijk et al. (2017b). In short, at the start and end of the experiment and during replacement of the culture media every four days, subsamples were collected and acidified with three times Quartz distilled HCl to pH ∼ 1.8. Additionally, seawater from the aquarium in Burgers’ Zoo was sampled during collection of coral debris at two different occasions. In total, we collected three seawater samples (total n = 6) in 50 ml Falcon tubes, which were returned to the laboratory, acidified and stored at 7°C upon analysis. The seawater composition of the experimental samples as well as the Burgers’ Zoo seawater was analyzed on an Element-2 SF-ICP-MS run in medium resolution mode. International Association for the Physical Sciences of the Ocean Standard Seawater was used as a drift monitor. Analytical precision (RSD) was 3% for Ca, 4% for Mg, 1% Na, 1% for Sr and 5% Ba. For the samples of the culture experiment, we obtained average values of 5.25 ± 0.06 mol/mol for Mg/Ca, 44.6 ± 0.6 mol/mol for Na/Ca, 8.63 ± 0.05 mmol/mol for Sr/Ca, and 9.04 ± 0.47 μmol/mol for Ba/Ca. For Burgers’ Zoo seawater, Mg/Ca was 6.1 ± 0.9 mol/mol.
Due to the low concentration, [Mn] of subsamples from the culture experiment and tropical aquarium had to be analyzed using a commercially available pre-concentration system, SeaFAST S2. With the SeaFAST system elements with low concentrations are pre-concentrated to values above detection limit of the SF-ICP-MS. Accordingly, we measured Cd, Pb, U, B, Ti, Mn, Fe, Co, Ni, Cu, and Zn. In short, 10 ml of sample was mixed with an ammonium acetate buffer to pH 6.2 and loaded on a column containing NOBIAS chelating agent. After rinsing the column with a diluted ammonium acetate buffer the metals were eluted in 750 µL of quartz distilled 1.5 M HNO3 before being quantified on the SF-ICP-MS. Analytical precision (RSD) was 4% for Mn and we obtained an average seawater Mn/Ca value of 5.4 ± 0.3 μmol/mol for the culture experiment, and of 14.8 ± 0.2 μmol/mol for the Burgers’ Zoo samples.
Results
Foraminiferal Mn/Ca and Mg/Ca vs. pCO2 From Controlled Experiments
For the hyaline species A. gibbosa, Mn/Ca is 4.4 ± 2.1 μmol/mol for all measurements on average. When pCO2 increases from 350 to 1,200 ppm, average Mn/Ca increase significantly (p < 0.0025; R2 = 0.95) with pCO2 (Figure 1C). From lowest to highest pCO2 condition, Mn/Ca increases from 3.2 to 5.9 μmol/mol (Figure 1A), an overall increase of factor 1.9, or 22% per 100 ppm CO2. For the correlation between [CO32−] and pH with Mn/Ca, see Supplementary Figure S1. Mn/Ca of the porcelaneous species S. marginalis are ∼11 times higher than the hyaline species A. gibbosa. For this species, Mn/Ca increases significantly with pCO2 as well (p < 0.01; R2 = 0.91; Figure 1D) by a factor of 2.1, or 25% per 100 ppm CO2, from 42.7 to 90.3 μmol/mol. For both species, standard deviation (SD) per treatment increases with increasing pCO2, and hence increasing Mn/Ca. For A. lessonii, the relative SD (RSD) is on average 40% for the different conditions, while for S. marginalis, the RSD increases from 35 to 51% with increasing pCO2.
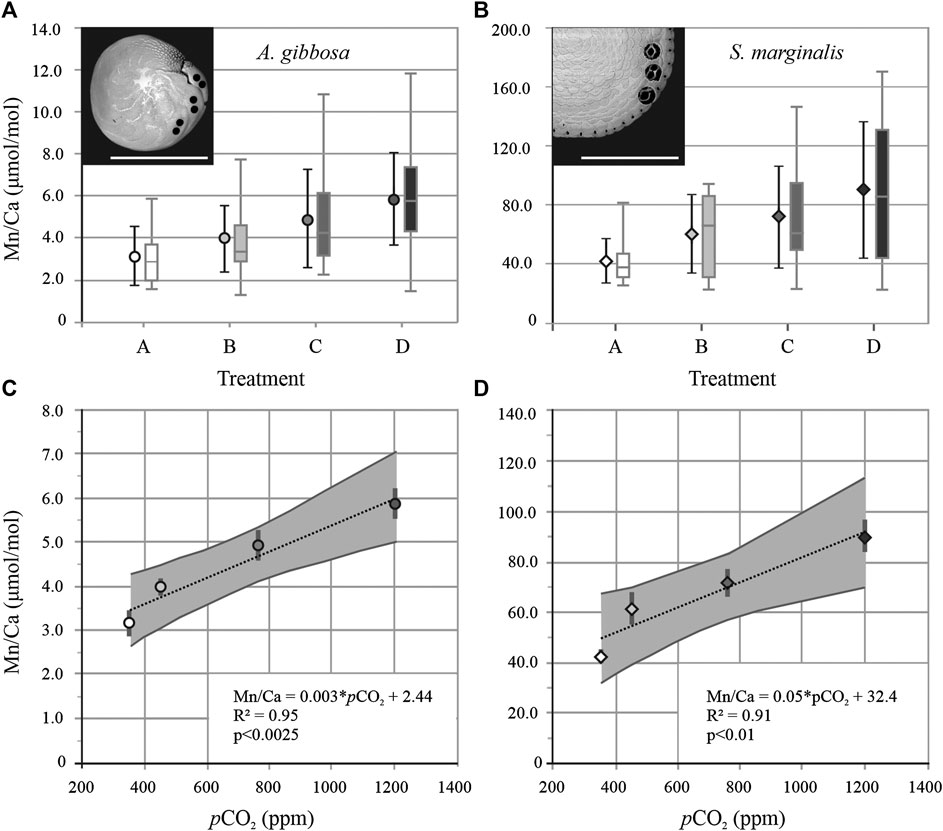
FIGURE 1. Average foraminiferal Mn/Ca with standard deviation and boxplot of all datapoints per treatment A, B, C, and D for A. gibbosa (A; circles) and Sorites marginalis (B; diamonds). Insets show SEM images of both species with laser ablation spots visible. Scale bar = 500 µm. Average Mn/Ca ± standard error (gray bar) vs. pCO2, with regression line and 95% confidence interval (gray area) for A. gibbosa (C; Mn/Ca = 0.003 × pCO2 +2.44 with R2 = 0.95 and p < 0.0025) and S. marginalis (D; Mn/Ca = 0.05 × pCO2 + 32.4 with R2 = 0.91 and p < 0.01).
Individual measurements of Mn/Ca and Mg/Ca for both species are shown in Figure 2. Average Mg/Ca is 27.4 ± 5.3 and 141.9 ± 5.3 mmol/mol for A. gibbosa and S. marginalis, respectively (Table 3), which fits very well with earlier measurements on the same specimens, where the average Mg/Ca values obtained were 27.7 and 145.8 mmol/mol, respectively (Van Dijk et al., 2017b), illustrating the good reproducibility of the LA-ICP-MS analyses. Partitioning of Mg and Mn is, on average respectively 4.7 and 13.5 times higher in S. marginalis compared to A. gibbosa.
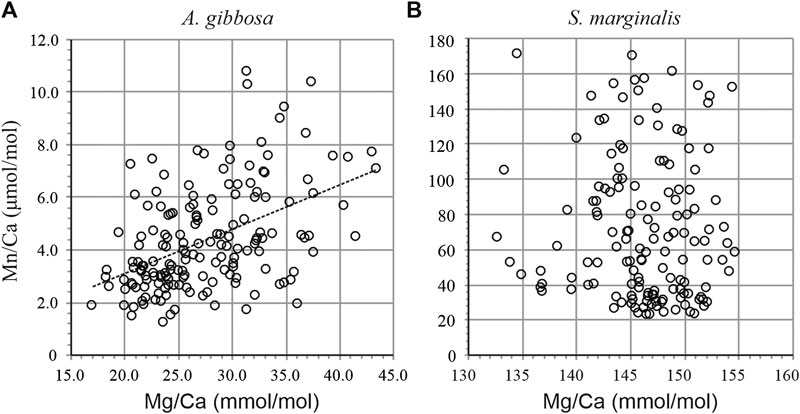
Figure 2. Mn/Ca vs. Mg/Ca of individual laser ablation measurements for A. gibbosa(A) and S. marginalis(B). For A. gibbosa, Mn/Ca increases with Mg/Ca following Mn/Ca = 0.17 × Mg/Ca-0.23.
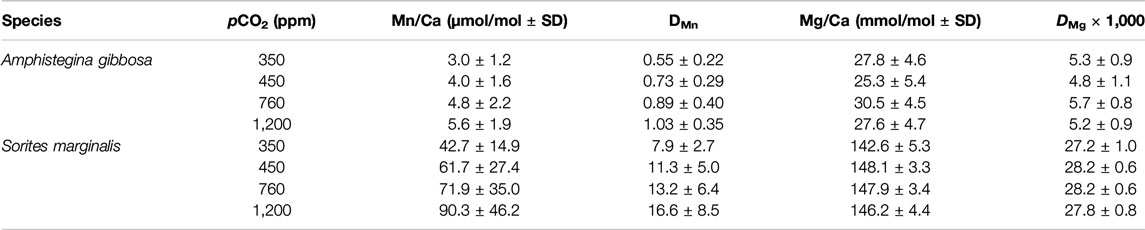
TABLE 3. Mn/Ca and Mg/Ca [±standard deviation (SD)] and element partitioning with propagating SD of hyaline species Amphistegina gibbosa and porcelaneous species Sorites marginalis cultured at different pCO2.
Foraminiferal Growth as a Function of pCO2 From Controlled Experiments
On average for all conditions, ∼80% of the specimens of S. marginalis (for example, see Figure 3B) added new chambers during the experiment, vs. ∼90% of A. gibbosa individuals (for example, see Figure 3C). There is no significant trend of chamber addition rate (number of chambers added per day) with pCO2 for both species studied (Figure 3A). On average, specimens of S. marginalis added more than two times as many chambers during the culture period, 0.28 ± 0.12 chamber per day vs. 0.12 ± 0.05 for specimens of A. gibbosa.
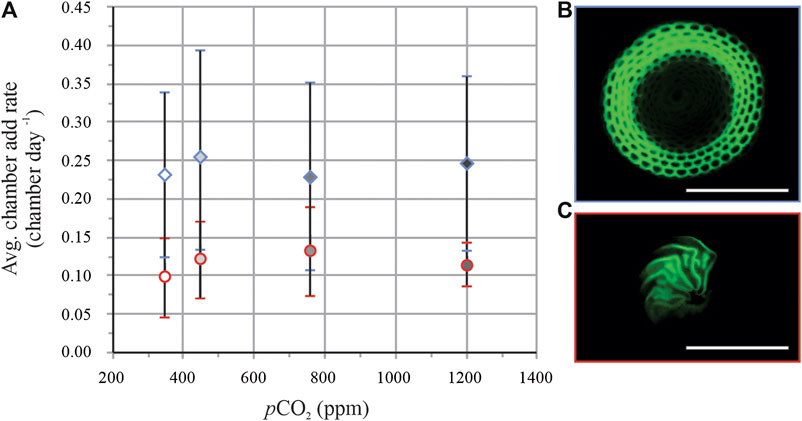
FIGURE 3. Average chamber addition rates (A) per day (±SD) of S. marginalis (blue diamonds) in blue and A. gibbosa (red circles). Right panels show pictures of S. marginalis(B) and A. gibbosa(C).
Mn and Mg Partitioning of Foraminifera From the Indo-Pacific Aquarium
On average partitioning of Mn (DMn) and Mg (DMg × 103) are, respectively: 0.8 ± 0.4 and 5.5 ± 0.1 for A. lessonii, 1.9 ± 0.8 and 26.7 ± 0.3 for H. depressa, which are both hyaline species; and 16.2 ± 3.2 and 26.7 ± 0.4 for S. orbiculus, 11.8 ± 1.6 and 25.3 ± 0.4 for S. angulata, 18.6 ± 1.9 and 22.5 ± 0.4 for S. communis, 23.0 ± 1.8 and 25.9 ± 0.4 for Q. pseudoreticulata and 20.4 ± 2.7 and 23.5 ± 0.4 for Quinqueloculina sp., which are five porcelaneous species (see Table 4). The DMn and DMg values are plotted together to visualize possible trends between the two groups of foraminifera (Figure 4). In general, porcelaneous species incorporate more Mn than hyaline species and the species investigated have similar Mg content (DMg × 103 of 22.5–26.7). In contrast, hyaline species comprise a wider range in DMg compared to the porcelaneous species.
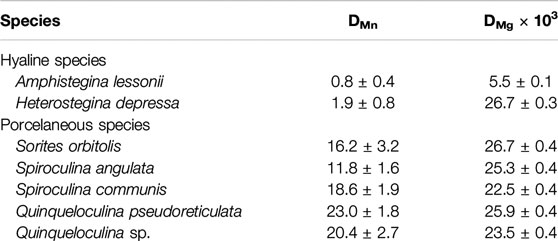
TABLE 4. Mn and Mg partitioning with propagating standard deviation for species collected from Burgers’ Zoo aquarium.
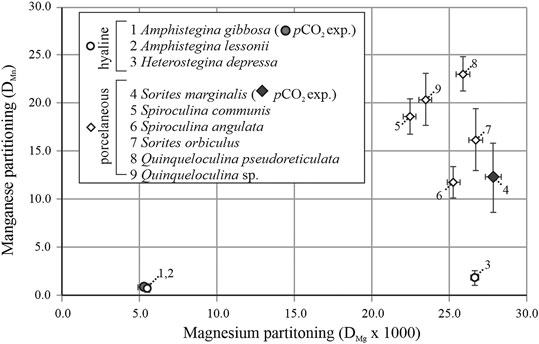
FIGURE 4. Overview of the average partitioning of Mn (DMn) as a function of DMg × 103 for foraminiferal species studied here, from both the controlled pCO2 experiment (average value, closed symbols) and the Indo-Pacific coral reef aquarium (open symbols). Hyaline species and porcelaneous species are indicated with circles and diamond respectively. Error bars indicate SD and numbers indicate species.
Discussion
Mn Incorporation as a Function of pCO2
For both species studied here, the hyaline A. gibbosa and the porcelaneous S. marginalis, we observe that shell Mn/Ca increases with increasing pCO2 (and hence decreasing sea water pH, [CO32−] or Ω). A similar trend was observed for the same species for Zn and Ba, but was not found case for elements such as Mg, Na and Sr (Van Dijk et al., 2017b). We do not suggest that Mn/Ca values can be used as recorders of the carbonate system, since Mn/Ca in foraminiferal shells is hypothesized influenced by seawater [Mn] (Barras et al., 2018), but this could hamper the precision of this proxy (cf. Chapter 4.3). Still, comparing sensitivity of Mn incorporation to pCO2 to that of other elements provides information on the underlying mechanisms involved in organismal trace element uptake. Even though the slope of the Mn/Ca-pCO2 calibrations differs between species (0.003 for A. gibbosa and 0.05 for S. marginalis), the incorporation of Mn as a function of pCO2 is, in terms of relative sensitivity, similar for A. gibbosa (22% increase in Mn/Ca per 100 ppm CO2) and S. marginalis (25% increase in Mn/Ca per 100 ppm increase in CO2). This is surprising since these species are known to have contrasting calcification strategies (e.g., Bé et al., 1979; De Nooijer et al., 2009a; Hemleben et al., 1986). This could imply that Mn incorporation as a function of pCO2 is governed by identical processes in calcification. This might relate to the (active) uptake of trace elements or the subsequent precipitation of carbonate. The first factor inherently includes e.g., symbiont and enzyme activity, speciation of Mn in seawater and pH-dependent absorption of Mn to calcite. The second factor, actual carbonate precipitation, includes both abiotic factors such as precipitation rate and also potentially biological controls during this phase.
Impact of pCO2 on Biological Processes
Growth of marine organisms is, in general, negatively impacted by current changes in oceanic conditions, global warming and ocean acidification (e.g., Orr et al., 2005; Kroeker et al., 2013). For inorganic precipitation experiments, crystal growth rate has a negative effect on incorporation of Mn, resulting in lower Mn/Ca values at higher precipitation rates (Lorens, 1981). Therefore, an increase in Mn incorporation in foraminiferal calcite with pCO2 might be explained by a decrease in the precipitation rate of the foraminiferal shell at higher pCO2, assuming that results from synthetic calcites can be directly translated to biogenic calcite. In our experiments, we measured the average number of newly formed chambers per day for both species and we can conclude that pCO2 concentrations have no impact on their calcification capacity, i.e., chamber addition rates (n chambers day−1) are stable over the range of culture conditions investigated. However, from these data, we cannot evaluate the calcite precipitation rate itself. As shown by meta-studies on the effect of pCO2 on foraminiferal calcification rates, calculated using a variety of methods by Doo et al. (2014) and Keul et al. (2013), response of foraminifera growth is very species-specific, including negative, positive and no effect growth parameters with increasing pCO2 conditions. Studies that investigate calcification rates of similar species as used in this study in more detail, i.e., by calculating changes in surface area and buoyant weight or shell density as a function of pH, observed opposite trends according to the species considered: no decrease in growth rates for dinoflagellate-bearing porcelaneous species like Marginopora vertebralis after extended periods (30–43 days) at a pH 7.6–7.4 (Vogel and Uthicke, 2012; Prazeres et al., 2015), while the diatom-bearing A. lessonii showed a steady reduction of shell density and volume at lower pH (Prazeres et al., 2015). Assuming that the calculated calcification rates in these studies would be correlated to crystal growth rates, there would be no change in precipitation rate in porcelaneous species in the range of pH studied here and therefore the trend in Mn/Ca would not be explained by precipitation rate. However, the link between calcite addition rate and crystal growth rate still needs to be explored. A detailed study on foraminiferal calcification rate, in which chamber addition rates should be clearly decoupled from crystal growth rates, is necessary to evaluate the impact of precipitation rate on foraminiferal Mn/Ca values and other elemental ratios in general. Since we cannot prove that the observed trends in Mn/Ca are (only) driven by carbonate chemistry-drive changes in crystal growth rates, we also explore other possibilities.
Foraminiferal Mn/Ca values may be link to Ca-ATPase, which appears to play an important role in regulating Ca2+ uptake for calcification (Toyofuku et al., 2017). According to Prazeres et al. (2015), upregulation of Ca-ATPase is confirmed at lower pH values for A. lessonii. Their interpretation is that the enzyme is used for removing Ca2+ out of the SOC due to shell dissolution. However, this is not logical due to the large amount of Ca2+ foraminifera need for calcification (De Nooijer et al., 2009a); even if shell dissolution would lead to an increase in Ca2+ in the SOC (and not the surrounding seawater), this resource is much too valuable to transport outwards. We therefore argue that Ca-ATPase is used in foraminiferal calcification to take up Ca2+, as proposed by Toyofuku et al. (2017). Higher activity of this enzyme might also increase the accidental uptake of Mn2+, due to the similarity in ionic radii of Mn2+ and Ca2+. This would in theory lead to higher Mn availability in the SOC and therefore incorporation in the shell at lower pH and higher pCO2. However, this increase in Ca-ATPase activity is not observed for porcelaneous species like S. marginalis, in which we also observe a positive correlation between pCO2 and Mn/Ca values and can thus not explain the increase in Mn/Ca in this species.
Effect of pCO2 on Speciation and Adsorption of Mn
Incorporation of Mn might be governed by the speciation of Mn in seawater with pCO2. Besides Mn, the incorporation of Ba and Zn also increases with higher pCO2, which could be explained by changes in the chemical speciation of these elements, induced by seawater carbonate chemistry (Van Dijk et al., 2017b; Van Dijk et al., 2017c). We have tested this hypothesis for Mn using the software package PHREEQC (v.2; Parkhurst and Appelo, 1999) and the standard PHREEQC llnl database, which allows modeling of bioavailability of different chemical species (eg, Mn2+, MnCO3) in seawater. The model shows a shift in element speciation with pCO2 (Figure 5A). When seawater chemistry changes from low pCO2 (or high pH) to higher pCO2 (or low pH), we observe an increase in the activity of free Mn2+, soluble carbonate-complexes decreases, which is similar to the findings for Zn and Ba speciation with pCO2 (Van Dijk et al., 2017b).
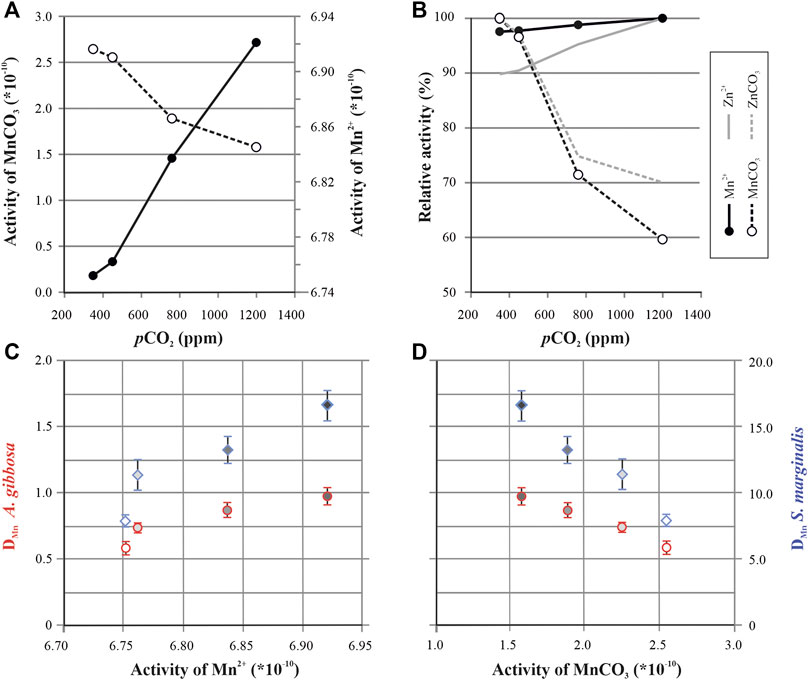
FIGURE 5. Modeled activity of Mn by PHREEQC. Speciation of free (Mn2+; closed symbols) and complexed (MnCO3; open symbols) Mn plotted as a function of pCO2(A). Relative change in activity of free Mn2+ and Mn-CO3 complexes (B) plotted together with relative activity changes of free Zn2+ (solid gray line) and ZnCO3 complexes (dashed gray line) from van Dijk et al. (2017c). Mn partitioning for both species (A. gibbosa = red circles; Sorites marginalis = blue diamonds) as a function of Mn2+ activity (C) and MnCO3 activity (D), with symbol filling corresponding to pCO2 of the treatments, in which darker filling represents higher pCO2 conditions, see also Figure 2.
When considering other pH/CO32− depending proxies, the increase in Mn over the studied range in pCO2 is considerably smaller than for example the increase in Zn incorporation (Van Dijk et al., 2017b). For instance, when comparing the relative change in the activities of Mn and Zn (Figure 5B), the change in activity of free Zn (Zn2+; 10%) is larger than the changes in free Mn (Mn2+; 2%). This could then explain why Zn/CaCALCITE is more sensitive to pCO2 compared to Mn/CaCALCITE. For instance, for A. gibbosa, Mn/Ca increases 22% per 100 ppm CO2 (this study; Figure 1), while Zn/Ca increases 53% (Van Dijk et al., 2017b), which might be due to the higher increase in availability of free ions of Zn compared to Mn (Figure 5B).
Based on the PHREEQC model, increase in Mn incorporation at higher pCO2 could be explained by more (bio)available Mn2+ (Figures 5C,D) which may be transported by Ca-channels during calcification (Nehrke et al., 2013). However, to accurately model chemical speciation during chamber formation and assess its role in element incorporation, it is crucial to know the carbonate chemistry of both the foraminiferal microenvironment and at the SOC. In addition to the pH during calcification (De Nooijer et al., 2009; Toyofuku et al., 2017; Glas et al., 2012a) a second parameter of the inorganic carbon system is necessary to reconstruct the complete carbon system inside and outside the foraminifer. Since there are examples of functioning proxies for seawater carbonate chemistry, one of the most established one being the boron isotopic composition of foraminiferal shells as a proxy for seawater pH (e.g., Sanyal et al., 1996; Pagani et al., 2005; Foster and Rae, 2016), it is likely that the external and internal pH are in some way affected by ocean acidification.
Finally, Barras et al. (2018) suggested that Mn might be (partially) incorporated into the shell by incorporation into adsorption layers. This was hypothesized to explain different ontogenetic trends (differences in chamber to chamber values of Mn/Ca) observed between species of (deep-sea and intertidal) small benthic foraminifera. Sorption of divalent metals, like Mn2+, on the surface of synthetic calcite is pH dependent (Zachara et al., 1991), with lower pH resulting in lower adsorption. If such adsorption process would exist in large tropical species, which has not been proven yet, we would have expected that higher pCO2 would decrease Mn incorporation. However, we observe the exact opposite. Either the hypothesis of adsorption layers playing a role during biocalcification is not correct, at least for both large benthic species studied here, or this effect is much smaller and therefore negligible compared to e.g., changes in Mn speciation.
Manganese Incorporation in Different Species of Foraminifera
Since speciation of Mn is sensitive to carbonate chemistry of the seawater (Figure 5A) and also at the SOC, offsets in internal pH during calcification between species might be responsible for observed difference in Mn incorporation. Since internal (De Nooijer et al., 2009a) and external pH (Glas et al., 2012b) are linked due to proton pumping (Toyofuku et al., 2017), a higher internal pH would correspond to a lower pH in the foraminiferal microenvironment. This will lead in turn to a higher activity of free Mn outside the organism, which could be transported through Ca-channels. Following this hypothesis, foraminifera with higher Mn/Ca, like H. depressa (Figure 4), might have a higher passive transport of Mn and/or a higher internal pH during calcification compared to A. gibbosa. No independent evidence exists, however, for such a difference in internal pH between species or between the groups precipitating a hyaline or porcelaneous shell. For example, for hyaline species, also changes in the regulation of Ca-ATPase (Prazeres et al., 2015) between species might be responsible for the observed differences in Mn incorporation.
When investigating Mn incorporation across a wider range of foraminiferal species, we observe that overall Mn/Ca values seem linked to Mg content which seems to primarily reflect calcification strategy (Figure 4). Porcelaneous species incorporate in general more Mn and Mg, however we observe no correlation between shell Mg/Ca and Mn/Ca within this group of species. In contrast, within the hyaline species, we observe that species that have a higher Mg content also incorporate more Mn. Based on inorganic precipitation experiments, higher precipitation rates would lead to higher Mg partitioning (Mavromatis et al., 2013), but would also lower Mn/CaCALCITE due to a decrease in partitioning (Lorens, 1981). This could potentially explain the observed increase of Mg/CaCALCITE and simultaneous decrease of Mn/CaCALCITE with temperature in cultured A. lessonii (Van Dijk et al., 2019a). However, it does not explain the trends we observe for different hyaline and porcelaneous species (Figure 4), in which both Mg/Ca and Mn/Ca increase in concert. Whatever the cause of the offset in Mg content between species (discussed in more detail in Van Dijk et al., 2019a), difference in Mg/Ca can potentially by itself alter the incorporation of other elements, through crystal lattice distortion. Based on inorganic experiments, Mg incorporation alters the structure of the crystal lattice (Mucci, 1987) and the resulting strain on the destabilized crystal lattice allows for higher incorporation of other trace metals. Lattice distortion has been proposed before to explain an increase in Na with Mg for larger benthic foraminifera (e.g., Evans et al., 2015). Similarly, this might explain the observed trends for hyaline species, for which we observe a co-variation of Mn/Ca and Mg/Ca between individuals (Figure 2) and between species (Figure 4). However, this would also imply that porcelaneous, and high-Mg hyaline species that have the same DMg as high-Mg porcelaneous species (like H. depressa), should have a similar DMn. However, hyaline species have lower calcite Mn/Ca values compared to porcelaneous species, even when looking at species with similar Mg content, for instance H. depressa (DMg × 103 = 26.7, DMn = 1.9) compared to S. marginalis (DMg × 103 = 26.6, DMn = 13.2), which incorporates ∼7 times more Mn while Mg content is similar (Figure 4). This suggests there is an additional process, probably fundamentally related to calcification of either hyaline or porcelaneous shells, which respectively decreases or increases the Mn/Ca of the precipitated calcite.
Potential Constrains for Mn/Ca As a (Paleo-)Oxygenation Proxy
In this study we observed that partitioning of Mn is species-specific and that Mn/Ca is positively correlated with pCO2 (Figure 1) and/or negatively correlated with pH, [CO32−], Ω (Supplementary Figure S1). Although it is complicated to incisively explain this empirical relation with a single process, these observations have to be taken into account when applying Mn/Ca to field or (sub) geological samples as a proxy for oxygenation (Groeneveld and Filipsson, 2013; Ní Fhlaithearta et al., 2018; Petersen et al., 2018; Guo et al., 2019). Calibrations of the Mn/Ca oxygenation proxy for foraminifera require quantifying the direct impact of carbonate parameters on the proxy sensitivity, especially since covariation between pCO2 and oxygenation can occur in benthic environments. However, we expect that for benthic species the impact of differences in carbonate chemistry on foraminiferal Mn/Ca is limited compared to the effect of oxygen-induced changes in Mn2+ and subsequently foraminiferal Mn/Ca, due to the low sensitivity observed in this study (Figure 1). Further studies are needed to decouple the effect of both environmental parameters, like obtaining sensitivity of Mn/Ca of larger benthic species to changes in O2/seawater [Mn2+]. Furthermore, for calibration studies, in the water column, parameters of the carbonate chemistry will behave similar to this culture experiments, but in the sediment, characterized by high alkalinity, changes in carbonate chemistry might be buffered, and fluctuations in e.g., pH could therefore become negligible. More importantly, our observations of major differences in Mn incorporation between hyaline, both low and high Mg species, and porcelaneous species (Figure 4) is crucial to take into account for calibration and application of a potential O2 proxy. Reconstructing oxygen concentration requires species-specific calibrations for Mn/Ca as well as insight into factors potentially affecting Mn speciation.
Conclusions
Manganese incorporation in two larger benthic foraminifera, A. gibbosa and S. marginalis is shown to be affected by seawater carbonate chemistry. Average chamber addition rate in the studied species is not impacted by the range in carbonate chemistry studies in this short-term culture experiment. We show that for both species, grown in controlled conditions, shell Mn/Ca increases with pCO2 and hypothesize that this might not (only) be caused by changes of growth or precipitation rate or adsorption of Mn to the shell surface, but is likely impacted by changes in Mn speciation in seawater. Furthermore, in contrast to porcelaneous species, Mg and Mn incorporation in hyaline species seems to be correlated on specimen and species level, suggesting that the transport of both ions is somehow coupled during foraminiferal calcification. The high species-specific differences in partitioning of Mn calls for species-specific calibrations for Mn/Ca with oxygen content for proper application of the foraminiferal based Mn-oxygen proxy.
Data Availability Statement
The raw data supporting the conclusions of this article will be made available by the authors, without undue reservation. LA-ICP-MS data is provided in the Supplementary Material.
Author Contributions
IvD, LdN, and GJR designed the experiment, IvD performed the experiment and measurements. IvD, LdN, CB, and GJR discussed the data and wrote the manuscript. All authors contributed to data interpretation and writing of the final manuscript.
Funding
Main funding comes from Darwin Center for Biogeosciences (project 3020) and Netherlands Earth System Science Center (NESSC; Grant No. 024.002.001) to GJR with additional funding from University Bretagne Loire and Angers Loire Metropole (France) to CB. Experimental set-up was financed by NWO Grants 858.14.021 and 858.14.022.
Conflict of Interest
The authors declare that the research was conducted in the absence of any commercial or financial relationships that could be construed as a potential conflict of interest.
Acknowledgments
We would like to thank Johan Stapel for hosting the 2015 foraminiferal culture expedition at the CNSI, St. Eustatius, as well as all the participants, especially Esmee Geerken and Alice Webb. The controlled pCO2 set-up used in this study was designed and constructed by Steven van Heuven and Bob Koster (NWO Grants 858.14.021 and 858.14.022). We acknowledge Max Janse and Burgers’ Zoo for the samples from the tropical aquarium. Great thanks to Wim Boer for support with LA-Q-ICP-MS measurements and we would like to thank Kirsten Kooijman (Algae culturing), Patrick Laan (SF-ICP-MS), Karel Bakker (DIC) and Jan-Berend Stuut (SEM) for analysis and lab support.
Supplementary Material
The Supplementary Material for this article can be found online at: https://www.frontiersin.org/articles/10.3389/feart.2020.567701/full#supplementary-material
References
Barker, S., Cacho, I., Benway, H., and Tachikawa, K. (2005). Planktonic foraminiferal Mg/Ca as a proxy for past oceanic temperatures: a methodological overview and data compilation for the Last Glacial Maximum. Quat. Sci. Rev. 24, 821–834. doi:10.1016/j.quascirev.2004.07.016
Barker, S., Greaves, M., and Elderfield, H. (2003). A study of cleaning procedures used for foraminiferal Mg/Ca paleothermometry. Geochem. Geophys. Geosyst. 4, 1–8. doi:10.1029/2003gc000559
Barras, C., Mouret, A., Nardelli, M. P., Metzger, E., Petersen, J., La, C., et al. (2018). Experimental calibration of manganese incorporation in foraminiferal calcite. Geochim. Cosmochim. Acta. 237, 49–64. doi:10.1016/j.gca.2018.06.009
Bé, A. W. H., Hemleben, C., Anderson, O. R., Spindler, M., and Be, A. W. H. (1979). Chamber formation in planktonic foraminifera. Micropaleontology. 25, 294–307. doi:10.2307/1485304
Bentov, S., Brownlee, C., and Erez, J. (2009). The role of seawater endocytosis in the biomineralization process in calcareous foraminifera. Proc. Natl. Acad. Sci. U. S. A. 106, 21500–21504. doi:10.1073/pnas.0906636106
Bentov, S., and Erez, J. (2006). Impact of biomineralization processes on the Mg content of foraminiferal shells: a biological perspective. Geochem. Geophys. Geosyst. 7, 319. doi:10.1029/2005gc001015
de Nooijer, L. J., Spero, H. J., Erez, J., Bijma, J., and Reichart, G. J. (2014). Biomineralization in perforate foraminifera. Earth Sci. Rev. 135, 48–58. doi:10.1016/j.earscirev.2014.03.013
de Nooijer, L. J., Toyofuku, T., and Kitazato, H. (2009a). Foraminifera promote calcification by elevating their intracellular pH. Proc. Natl. Acad. Sci. U.S.A. 106, 15374–15378. doi:10.1073/pnas.0904306106
de Nooijer, L. J., Langer, G., Nehrke, G., and Bijma, J. (2009b). Physiological controls on seawater uptake and calcification in the benthic foraminifer Ammonia tepida. Biogeosciences. 6, 2669–2675.
Doo, S. S., Fujita, K., Byrne, M., and Uthicke, S. (2014). Fate of calcifying tropical symbiont-bearing large benthic foraminifera: living sands in a changing ocean. Biol. Bull. 226, 169–186. doi:10.1086/bblv226n3p169
Elderfield, H., Bertram, C., and Erez, J. (1996). A biomineralization model for the incorporation of trace elements into foraminiferal calcium carbonate. Earth Planet. Sci. Lett. 142, 409–423. doi:10.1016/0012-821x(96)00105-7
Erez, J. (2003). The source of ions for biomineralization in foraminifera and their implications for paleoceanographic proxies. Rev. Mineral. Geochem. 54, 115–150. doi:10.2113/0540115
Ernst, S., Janse, M., Renema, W., Kouwenhoven, T., Goudeau, M.-L., and Reichart, G.-J. (2011). Benthic foraminifera in a large Indo-Pacific coral reef aquarium. J. Foraminifer. Res. 41, 101–113. doi:10.2113/gsjfr.41.2.101
Evans, D., Erez, J., Oron, S., and Müller, W. (2015). Mg/Ca-temperature and seawater-test chemistry relationships in the shallow-dwelling large benthic foraminifera Operculina ammonoides. Geochim. Cosmochim. Acta. 148, 325–342. doi:10.1016/j.gca.2014.09.039
Evans, D., Müller, W., and Erez, J. (2018). Assessing foraminifera biomineralisation models through trace element data of cultures under variable seawater chemistry. Geochim. Cosmochim. Acta. 236, 198–217. doi:10.1016/j.gca.2018.02.048
Foster, G. L., and Rae, J. W. B. (2016). Reconstructing ocean pH with boron isotopes in foraminifera. Ann. Rev. Earth Planet. Sci. 44, 207–237. doi:10.1146/annurev-earth-060115-012226
Glas, M. S., Fabricius, K. E., de Beer, D., and Uthicke, S. (2012a). The O2, pH and Ca2+ microenvironment of benthic foraminifera in a high CO2 world. PLoS ONE. 7, e50010. doi:10.1371/journal.pone.0050010
Glas, M. S., Langer, G., and Keul, N. (2012b). Calcification acidifies the microenvironment of a benthic foraminifer (Ammonia sp.). J. Exp. Mar. Biol. Ecol. 424–425, 53–58. doi:10.1016/j.jembe.2012.05.006
Groeneveld, J., and Filipsson, H. L. (2013). Mg/Ca and Mn/Ca ratios in benthic foraminifera: the potential to reconstruct past variations in temperature and hypoxia in shelf regions. Biogeosciences. 10, 5125–5138. doi:10.5194/bg-10-5125-2013
Gonçalves, P., Meireles, S., Neves, P., and Vale, M. (1999). Ionic selectivity of the Ca2+/H+ antiport in synaptic vesicles of sheep brain cortex. Mol. Brain Res. 67 (2), 283–291. doi:10.1016/S0169-328X(99)00081-9
Guillong, M., Meier, D. L., Allan, M. M., Heinrich, C. A., and Yardley, B. W. (2008). SILLS: a MATLAB-based program for the reduction of laser ablation ICP-MS data of homogeneous materials and inclusions. Miner. Assoc. Canada Short Course Ser. 40, 328–333.
Guo, X., Xu, B., Burnett, W. C., Yu, Z., Yang, S., Huang, X., et al. (2019). A potential proxy for seasonal hypoxia: LA-ICP-MS Mn/Ca ratios in benthic foraminifera from the Yangtze River Estuary. Geochim. Cosmochim. Acta. 245, 290–303. doi:10.1016/j.gca.2018.11.007
Hemleben, C. H., Anderson, O. R., Berthold, W., and Spindler, M. (1986). “Calcification and chamber formation in Foraminifera-a brief overview,” in Biomineralization in lower plants and animals. Editors B. S. Leadbeater and R. Riding (Oxford, UK: Clarendon Press), 237–249.
Jacob, D. E., Wirth, R., Agbaje, O. B. A., Branson, O., and Eggins, S. M. (2017). Planktic foraminifera form their shells via metastable carbonate phases. Nat. Commun. 8, 1265. doi:10.1038/s41467-017-00955-0
Keul, N., Langer, G., de Nooijer, L. J., and Bijma, J. (2013). Effect of ocean acidification on the benthic foraminifera. Biogeosciences 10, 6185–6198. doi:10.5194/bg-10-6185-2013
Keul, N., Langer, G., Thoms, S., de Nooijer, L. J., Reichart, G. J., and Bijma, J. (2017). Exploring foraminiferal Sr/Ca as a new carbonate system proxy. Geochem. Cosmochim. Acta. 202, 374–386. doi:10.1016/j.gca.2016.11.022
Koho, K. A., de Nooijer, L. J., and Reichart, G. J. (2015). Combining benthic foraminiferal ecology and shell Mn/Ca to deconvolve past bottom water oxygenation and paleoproductivity. Geochem. Cosmochim. Acta. 165, 294–306. doi:10.1016/j.gca.2015.06.003
Kroeker, K. J., Kordas, R. L., Crim, R., Hendriks, I. E., Ramajo, L., Singh, G. S., et al. (2013). Impacts of ocean acidification on marine organisms: quantifying sensitivities and interaction with warming. Glob. Chang. Biol. 19, 1884–1896. doi:10.1111/gcb.12179
Lear, C. H., Elderfield, H., and Wilson, P. A. (2000). Cenozoic deep-Sea temperatures and global ice volumes from Mg/Ca in benthic foraminiferal calcite. Science 287, 269–272. doi:10.1126/science.287.5451.269
Lee, J. J., Morales, J., Symons, A., and Hallock, P. (1995). Diatom symbionts in larger foraminifera from Caribbean hosts. Mar. Micropaleontol. 26, 99–105. doi:10.1016/0377-8398(95)00004-6
Lorens, R. B. (1981). Sr, Cd, Mn and Co distribution coefficients in calcite as a function of calcite precipitation rate. Geochem. Cosmochim. Acta. 45, 553–561. doi:10.1016/0016-7037(81)90188-5
Müller-Merz, E., and Lee, J. J. (1976). Symbiosis in the larger foraminiferan Sorites marginalis (with notes on Archaias spp.). J. Protozool. 23, 390–396. doi:10.1111/j.1550-7408.1976.tb03793.x
Mavromatis, V., Gautier, Q., Bosc, O., and Schott, J. (2013). Kinetics of Mg partition and Mg stable isotope fractionation during its incorporation in calcite. Geochem. Cosmochim. Acta. 114, 188–203. doi:10.1016/j.gca.2013.03.024
McKay, C. L., Groeneveld, J., Filipsson, H. L., Gallego-Torres, D., Whitehouse, M. J., Toyofuku, T., et al. (2015). A comparison of benthic foraminiferal Mn/Ca and sedimentary Mn/Al as proxies of relative bottom-water oxygenation in the low-latitude NE Atlantic upwelling system. Biogeosciences 12, 5415–5428. doi:10.5194/bg-12-5415-2015
Mewes, A., Langer, G., Reichart, G. J., de Nooijer, L. J., Nehrke, G., and Bijma, J. (2015). The impact of Mg contents on Sr partitioning in benthic foraminifers. Chem. Geol. 412, 92–98. doi:10.1016/j.chemgeo.2015.06.026
Mezger, E. M., de Nooijer, L. J., Boer, W., Brummer, G. J. A., and Reichart, G. J. (2016). Salinity controls on Na incorporation in Red Sea planktonic foraminifera. Paleoceanography 31, 1562–1582. doi:10.1002/2016pa003052
Middelburg, J. J., De Lange, G. J., and van Der Weijden, C. H. (1987). Manganese solubility control in marine pore waters. Geochem. Cosmochim. Acta. 51, 759–763. doi:10.1016/0016-7037(87)90086-x
Mucci, A. (1987). Influence of temperature on the composition of magnesian calcite overgrowths precipitated from seawater. Geochem. Cosmochim. Acta. 51, 1977–1984. doi:10.1016/0016-7037(87)90186-4
Mucci, A., and Morse, J. W. (1983). The incorporation of Mg2+ and Sr2+ into calcite overgrowths: influences of growth rate and solution composition. Geochem. Cosmochim. Acta. 47, 217–233. doi:10.1016/0016-7037(83)90135-7
Nehrke, G., Keul, N., Langer, G., de Nooijer, L. J., Bijma, J., and Meibom, A. (2013). A new model for biomineralization and trace - element signatures of Foraminifera tests. Biogeosciences 10, 6759–6767. doi:10.5194/bg-10-6759-2013
Ní Fhlaithearta, S., Fontanier, C., Jorissen, F., Mouret, A., Dueñas-Bohórquez, A., and Anschutz, P. (2018). Manganese incorporation in living (stained) benthic foraminiferal shells: a bathymetric and in-sediment study in the Gulf of Lions (NW Mediterranean). Biogeosciences. 15, 6315–6328. doi:10.5194/bg-15-6315-2018
Nürnberg, D., Bijma, J., and Hemleben, C. (1996). Assessing the reliability of magnesium in foraminiferal calcite as a proxy for water mass temperatures. Geochem. Cosmochim. Acta. 60, 803–814. doi:10.1016/0016-7037(95)00446-7
Okai, T., Suzuki, A., Kawahata, H., Terashima, S., and Imai, N. (2002). Preparation of a new geological survey of Japan geochemical reference material: coral JCp‐1. Geostand. Newsl. 26, 95–99. doi:10.1111/j.1751-908x.2002.tb00627.x
Orr, J. C., Fabry, V. J., Aumont, O., Bopp, L., Doney, S. C., Feely, R. A., et al. (2005). Anthropogenic ocean acidification over the twenty-first century and its impact on calcifying organisms. Nature 437, 681–686. doi:10.1038/nature04095
Pagani, M., Lemarchand, D., Spivack, A., and Gaillardet, J. (2005). A critical evaluation of the boron isotope-pH proxy: the accuracy of ancient ocean pH estimates. Geochem. Cosmochim. Acta. 69, 953–961. doi:10.1016/j.gca.2004.07.029
Parkhurst, D. L., and Appelo, C. (1999). User's guide to PHREEQC (version 2): a computer program for speciation, batch-reaction, one-dimensional transport, and inverse geochemical calculations. Denver, CO: US Geological Survey.
Petersen, J., Barras, C., Bézos, A., La, C., de Nooijer, L. J., Meysman, F. J. R., et al. (2018). Mn/Ca intra- and inter-test variability in the benthic foraminifer Ammonia tepida. Biogeosciences 15, 331–348. doi:10.5194/bg-15-331-2018
Prazeres, M., Uthicke, S., and Pandolfi, J. M. (2015). Ocean acidification induces biochemical and morphological changes in the calcification process of large benthic foraminifera. Proc. R. Soc. Lond. B Biol. Sci. 282. doi:10.1098/rspb.2014.2782
Reichart, G.-J., Jorissen, F., Anschutz, P., and Mason, P. R. (2003). Single foraminiferal test chemistry records the marine environment. Geology 31, 355–358. doi:10.1130/0091-7613(2003)031<0355:sftcrt>2.0.co;2
Ries, J. B., Cohen, A. L., and McCorkle, D. C. (2009). Marine calcifiers exhibit mixed responses to CO2-induced ocean acidification. Geology 37, 1131–1134. doi:10.1130/g30210a.1
Sanyal, A., Hemming, N. G., Broecker, W. S., Lea, D. W., Spero, H. J., and Hanson, G. N. (1996). Oceanic pH control on the boron isotopic composition of foraminifera: evidence from culture experiments. Paleoceanography 11, 513–517. doi:10.1029/96pa01858
Toyofuku, T., Matsuo, M. Y., de Nooijer, L. J., Nagai, Y., Kawada, S., Fujita, K., et al. (2017). Proton pumping accompanies calcification in foraminifera. Nat. Commun. 8, 14145. doi:10.1038/ncomms14145
Toyofuku, T., Suzuki, M., Suga, H., Sakai, S., Suzuki, A., Ishikawa, T., et al. (2011). Mg/Ca and δ18O in the brackish shallow-water benthic foraminifer Ammonia ‘beccarii.’ Mar. Micropaleontol. 78, 113–120. doi:10.1016/j.marmicro.2010.11.003
Tribovillard, N., Algeo, T. J., Lyons, T., and Riboulleau, A. (2006). Trace metals as paleoredox and paleoproductivity proxies: an update. Chem. Geol. 232, 12–32. doi:10.1016/j.chemgeo.2006.02.012
Van Dijk, I., Barras, C., de Nooijer, L. J., Mouret, A., Geerken, E., Oron, S., et al. (2019a). Coupled calcium and inorganic carbon uptake suggested by magnesium and sulfur incorporation in foraminiferal calcite. Biogeosciences 16, 2115–2130. doi:10.5194/bg-16-2115-2019
Van Dijk, I., de Nooijer, L. J., Boer, W., and Reichart, G. J. (2017a). Sulfur in foraminiferal calcite as a potential proxy for seawater carbonate ion concentration. Earth Planet Sci. Lett. 470, 64–72. doi:10.1016/j.epsl.2017.04.031
Van Dijk, I., de Nooijer, L. J., and Reichart, G. J. (2017b). Trends in element incorporation in hyaline and porcelaneous foraminifera as a function of pCO2. Biogeosciences 14, 497–510. doi:10.5194/bg-14-497-2017
Van Dijk, I., de Nooijer, L. J., Wolthers, M., and Reichart, G. J. (2017c). Impacts of pH and [CO32−] on the incorporation of Zn in foraminiferal calcite. Geochem. Cosmochim. Acta. 197, 263–277. doi:10.1016/j.gca.2016.10.031
Van Dijk, I., Mouret, A., Cotte, M., Le Houedec, S., Oron, S., Reichart, G.-J., et al. (2019b). Chemical heterogeneity of Mg, Mn, Na, S, and Sr in benthic foraminiferal calcite. Front. Earth Sci. 7, 281. doi:10.3389/feart.2019.00281
Vogel, N., and Uthicke, S. (2012). Calcification and photobiology in symbiont-bearing benthic foraminifera and responses to a high CO2 environment. J. Exp. Mar. Biol. Ecol. 424–425, 15–24. doi:10.1016/j.jembe.2012.05.008
Webb, A. E., van Heuven, S. M. A. C., de Bakker, D. M., van Duyl, F. C., Reichart, G.-J., and de Nooijer, L. J. (2017). Combined effects of experimental acidification and eutrophication on reef sponge bioerosion rates. Front. Mar. Sci.4, 313–315. doi:10.3389/fmars.2017.00311
Keywords: foraminifera, manganese, carbon chemistry, pCO2, proxy development
Citation: van Dijk I, de Nooijer LJ, Barras C, Reichart G-J (2020) Mn Incorporation in Large Benthic Foraminifera: Differences Between Species and the Impact of pCO2. Front. Earth Sci. 8:567701. doi:10.3389/feart.2020.567701
Received: 30 May 2020; Accepted: 10 September 2020;
Published: 05 October 2020.
Edited by:
Oscar Branson, University of Cambridge, United KingdomReviewed by:
David Evans, Goethe University Frankfurt, GermanyCatherine Davis, Yale University, United States
Copyright © 2020 van Dijk, de Nooijer, Barras and Reichart. This is an open-access article distributed under the terms of the Creative Commons Attribution License (CC BY). The use, distribution or reproduction in other forums is permitted, provided the original author(s) and the copyright owner(s) are credited and that the original publication in this journal is cited, in accordance with accepted academic practice. No use, distribution or reproduction is permitted which does not comply with these terms.
*Correspondence: Inge van Dijk, SW5nZS52YW4uRGlqa0BuaW96Lm5s