- 1Shell Exploration and Production Company, New Orleans, LA, United States
- 2Department of Geology and Geophysics, University of Utah, Salt Lake City, UT, United States
- 3Geological Sciences, School of Agriculture, Earth and Environmental Sciences, University of KwaZulu-Natal, Durban, South Africa
- 4School of Geography and Environmental Science, Ulster University, Coleraine, United Kingdom
Existing barrier island facies models are largely based on modern observations. This approach highlights the heterogeneous and dynamic nature of barrier island systems, but it overlooks processes tied to geologic time scales, such as multi-directional motion, erosion, and reworking, and their expressions as preserved strata. Accordingly, this study uses characteristic outcrop expressions from paralic strata of the Upper Cretaceous Straight Cliffs Formation in southern Utah to update models for barrier island motion and preservation to include geologic time-scale processes. Results indicate that the key distinguishing facies and architectural elements of preserved barrier island systems have very little to do with “island” morphology as observed in modern systems. Four facies associations are used to describe and characterize these barrier island architectural elements. Barrier islands occur in association with backbarrier fill (FA1) and internally contain lower and upper shoreface (FA2), proximal upper shoreface (FA3), and tidal channel facies (FA4). Three main architectural elements (barrier island shorefaces, shoreface-dominated inlet fill, and channel-dominated inlet fill) occur independently or in combination to create stacked barrier island deposits. Barrier island shorefaces record progradation, while shoreface-dominated inlet fill records lateral migration, and channel-dominated inlet fill records aggradation within the tidal inlet. Barrier islands are bound by lagoons or estuaries and are distinguished from other shoreface deposits by their internal facies and outcrop geometry, association with backbarrier facies, and position within transgressive successions. Tidal processes, in particular, tidal inlet migration and reworking of the upper shoreface, also distinguish barrier island successions. In sum, this study expands barrier island facies models and provides new recognition criteria to account for the complex geometries of time-transgressive, preserved barrier island deposits.
Introduction
Barrier islands comprise 10% of modern coastlines (Stutz and Pilkey, 2011), making them prominent coastal features. They are home to growing coastal populations and expanding infrastructure (Zhang and Leatherman, 2011), but are threatened by increasing storm prevalence and magnitude, and rising sea-levels driven by global warming (Leatherman, 1983; Zhang et al., 2002; Moore et al., 2010; Masselink and van Heteren, 2014; Moore and Murray, 2018). Research on modern barrier islands focuses on island motion, dynamics, and sediment budgets, to help better understand how barrier islands move and respond to anthropogenic alteration (Fisher and Dolan, 1977; Short, 1999; Dronkers, 2005; Stutz and Pilkey, 2005; Dyke, 2007; Anthony, 2009; Kana et al., 2011; Lentz and Hapke, 2011; Davis, 2013; Hein et al., 2019).
There is also an increasing drive to understand how barriers and barrier islands respond to forcing agents (e.g., sea-level rise and storms) in the context of local scale controls such as antecedent topography and geology (Cooper et al., 2018; Raff et al., 2018). Preserved barrier islands record transgression, and as such provide valuable tools to document how coastlines evolve through time and space and within the complex interplay between accommodation and sediment supply. Despite the strong emphasis on understanding modern barrier island stratigraphy and dynamics, little attention has been paid to barrier island preservation and dynamics over the longer-term geologic scale.
Barrier islands are elongate, shore-parallel bodies of unconsolidated sediment separated from an adjacent landmass by a body of water (Figure 1; Davis, 1994; Oertel, 1985; Otvos, 2012). The island is a distinct feature within the broader, complex, barrier island system, which includes sub-environments such as the backbarrier, flood- and ebb-tidal deltas, and washover fans (Figure 1), the morphodynamics of which have been studied in detail (cf. McBride et al., 2013). Stratigraphic sections through modern examples of barrier islands (Shepard and Moore, 1955; Hoyt and Henry, 1965; Kumar and Sanders, 1974; Thom, 1984; Moslow and Tye, 1985; Schwab et al., 2014), particularly an early study of Galveston Island (Bernard et al., 1962), are used as predictive models for interpreting preserved strata. Barrier islands are ephemeral features, subject to complicated, multi-directional, and multi-phase shoreline motion (Niedoroda et al., 1985; Swift et al., 1985; Hapke et al., 2010; Schwab et al., 2014) and other processes which were under-appreciated when facies models were first developed.
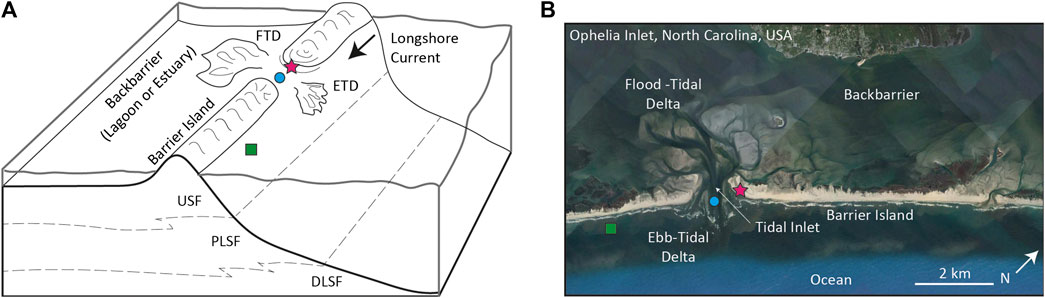
FIGURE 1. (A) Schematic, vertically-exaggerated block diagram and (B) Google Earth image showing the parts of a simple modern barrier island depositional system at a single snapshot in time. Markers indicate the three architectural elements that comprise the barrier island: barrier island shoreface (green square), shoreface-dominated inlet fill (pink star), and channel-dominated inlet fill (blue circle). Note that the shoreface extends across the tidal inlet and is reworked by the ebb-tidal delta. FTD, flood-tidal delta; ETD, ebb-tidal delta; DLSF, distal lower shoreface; PLSF, proximal lower shoreface; USF, upper shoreface.
Barrier islands are not commonly recognized in the rock record [e.g., Johannessen and Nielsen 2010; Mulhern et al., 2019 (references therein); Sixsmith et al., 2008; Painter et al., 2013] and facies models are notably lacking in comparison to other sedimentary environments, particularly with regard to recognition criteria (Boyd, 2010). Research on modern barrier islands forms the basis of existing facies models and recognition criteria for preserved barrier islands (Figure 1B), but the last major conceptual models for interpretation are outdated, not incorporating sedimentological progress made in the last three decades (Dickinson et al., 1972; Reinson, 1979, Reinson, 1984; Reinson, 1979; Schatzinger et al., 1989). Furthermore, the tie to the modern is precarious in that it introduces an inherent bias; modern system observation is limited to short time scales and Pleistocene glacial sea-level fluctuations, whereas ancient systems time-average geologic processes (Storms et al., 2002). Models developed for the 10- to 1000-years timescale and 1–100 km lateral scales are insufficient when upscaled to millions of years and hundreds of kilometers. As a result, many interpretations group barrier islands with shoreface environments, potentially missing important clues to depositional history, sand-body distribution, large-scale sedimentary drivers etc. (Donselaar and Nio, 1982; Olsen et al., 1999; Mellere et al., 2005; Allen and Johnson, 2011; Antia et al., 2011; Kieft et al., 2011). Some barrier islands are identified in the rock record (compiled list in Mulhern et al., 2019) usually based on a stratigraphic transgressive position, but there remains the opportunity to expand on distinguishing sedimentological characteristics to enable proper classification moving forward. Significant progress has been made to expand modern understanding to the millennial-scale, for example preserved barrier island and barrier features on the shoreface (Green et al., 2014, Green et al., 2018). In contrast, links to geologic time scales have only been explored preliminarily (Cooper et al., 2018; Mulhern et al., 2019).
Here we examine a series of preserved barrier island systems from southern Utah. Outcrops of the Upper Cretaceous Straight Cliffs Formation, with lateral exposures that are kilometers long and 10–100s of meters thick, provide an excellent opportunity to study ancient barrier island successions in detail. Measured sections of three successions, interpreted as barrier islands, show the internal facies and geometry of barrier islands in outcrop. In examining the lateral and vertical arrangement of these architectural elements, we provide novel insight into barrier island dynamics and preservation at geologic timescales. By juxtaposing these observations with modern and relict barrier islands preserved on the continental shelves, we show that definitions and recognition criteria for modern islands cannot be used to effectively interpret preserved outcrop facies and geometries. Instead, a new set of architectural elements is proposed to provide a more useful framework to guide the interpretation of ancient barrier island deposits, and to help improve our understanding of barrier island preservation.
Geologic Background and Methods
Outcrop observations from the Cretaceous Straight Cliffs Formation in the Kaiparowits Plateau of southern Utah document the facies of barrier island deposits (Figure 2). Located in the foreland basin of the Cordilleran Sevier fold-and-thrust belt, the northern Kaiparowits Plateau experienced unusually high rates of sediment supply and accommodation during deposition of the Coniacian–early Campanian John Henry Member of the Straight Cliffs Formation. These conditions led to the preservation of stacked, fourth-order, regressive-transgressive, shallow marine successions (Allen and Johnson, 2011). This study focuses on outcrops of the John Henry Member at Buck Hollow and Alvey Wash near Escalante, Utah, where stacked barrier island successions are preserved over a ∼150–250 m thick section of about four million years of deposition (Figure 2; Mulhern and Johnson, 2016).
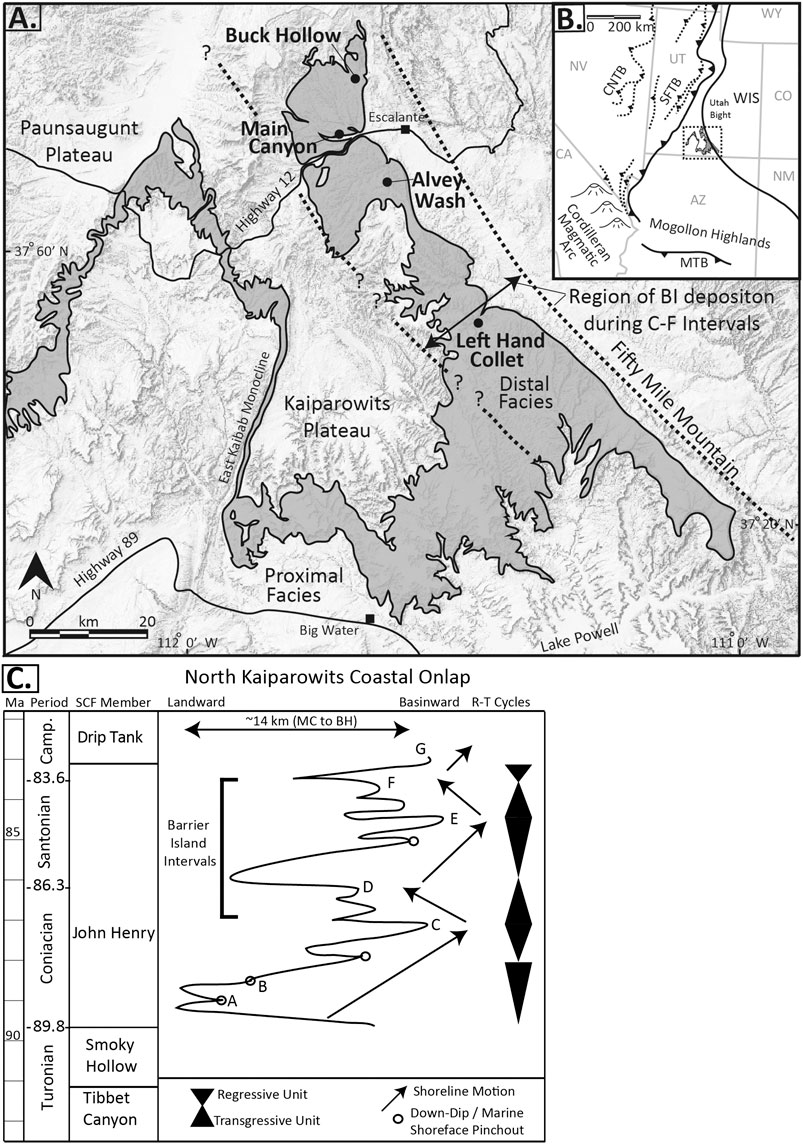
FIGURE 2. (A) Regional map of the Kaiparowits Plateau of southern Utah. Outcrops of the Straight Cliffs Formation are shown in grey and the locations of previous studies focused on the John Henry Member are labelled. General proximal to distal facies relationships in the John Henry Member range from fluvial on the western margin to marine on the eastern margin, with tidal and paralic facies in between. Dashed lines show the region of barrier island (BI) deposition during the C‒F intervals of the John Henry Member. (B) Location of the northern Kaiparowits Plateau geologic map. This regional map shows the shoreline of the Western Interior Seaway (WIS) as well as where deformation was taking place in the region as indicated by the thrust belts. (C) Stratigraphic column showing the age of the four members of the Straight Cliffs Formation. Coastal onlap curve for the northern Kaiparowits Plateau estimates the relative shifts in the shoreline position based on the stratigraphy at Buck Hollow and Main Canyon and down-dip shoreface pinch-outs (into offshore marine) where identified. The letters marking the sub intervals of the John Henry Member are placed at the top of each succession. Modified from Chentnik et al. (2015) and Mulhern and Johnson (2016). CNTB, Central Nevada Thrust Belt; SFTB, Sevier fold-thrust belt; WIS, Western Interior Seaway; MTB, Maria Thrust Belt; Camp., Campanian; SCF, Straight Cliffs Formation; MC, Main Canyon; BH, Buck Hollow; R-T, regressive-transgressive; BI, barrier island.
Previous studies from the John Henry Member provide a sedimentological and sequence stratigraphic framework of the Straight Cliffs Formation in the northern Kaiparowits Plateau (Peterson, 1969; Hettinger et al., 1993; Shanley and McCabe, 1995; Chentnik et al., 2015). The paleoshoreline trend ran roughly north-northwest to south-southeast, therefore depositional strike runs parallel to the eastern edge of the plateau (Figure 2A). The thickest member of the Straight Cliffs Formation, the John Henry Member is broken into eight intervals lettered A through G, which define the main shoreline successions exposed along Fifty Mile Mountain (Figure 2C; Peterson, 1969). The John Henry Member was the focus of early sequence stratigraphic studies, which linked terrestrial and marine depositional environments (Shanley and McCabe, 1995). Detailed stratigraphic analysis recognizes four regressive-transgressive cycles in the John Henry Member, particularly, a regional transgression, which favored deposition and preservation of the barrier island systems documented here (C-D and E-F intervals; Figure 2C; Allen and Johnson, 2011; Chentnik et al., 2015; Dooling, 2013; Mulhern and Johnson, 2016).
Measured sections and aerial photography data from Buck Hollow and Alvey Wash (Figure 2A) were used to document and interpret barrier island facies from within the John Henry Member. The outcrop exposure at Buck Hollow (Figure 2A) consists of a ∼7 km long along-strike outcrop exposure, with ∼456 m of total John Henry Member section (Mulhern and Johnson, 2016). Alvey Wash is located along Smoky Mountain Road, 5 km south of Escalante, Utah (Figure 2A) and the outcrops at Alvey Wash are exposed in a ∼6 km along-strike canyon with parallel cliff-faces on both sides. In addition, east-west oriented side canyons provide dip exposures of ∼330 m of John Henry Member strata.
Results
John Henry Member barrier island deposits are characterized using twelve lithofacies (Table 1) which combine to create four facies associations (Table 2), which are described and interpreted below. Typical stacking patterns of these facies associations define three main barrier island architectural elements, which can occur independently or in combination. A variety of architectural geometries and stratigraphic relationships are documented, to illustrate geologic-scale barrier island dynamics and the ultimate preservation of the resulting sedimentological features.
Facies Associations
Facies Association 1
Description
Carbonaceous Mudstones, Fine-Grained Sandstones, and Coals
This facies is composed of brown and gray carbonaceous mudstones that grade vertically into coals (Figures 3D,H,J). The mudstones are wavy-bedded and contain abundant plant fossils (Figure 3F), terrigenous material, coal fragments, and oyster shell fragments (Figures 3C,E). The mudstone intervals are dissected by lens-shaped, fine- and medium-grained sandstones (0.02–0.30 m thick; Figure 3I), which pinch out laterally over 0.5–2.0 m (Figure 3A). Shell fragments are distributed sporadically throughout the mudstones or gathered into layers and clusters (Figure 3C). Coals are 0.05–2.0 m thick. The upper surfaces of the coal beds have locally abundant Thalassinoides burrows (Figures 3G). Coals and mudstones locally contain flaser to lenticular bedding (Figure 3B). Mudstones contain palynoflora Quadripollis krempii) and dinoflagellates (Spiniferites ramosus and Palaeohystrichophora infusoridides; Mulhern and Johnson, 2016; Pocknall et al., 2016).
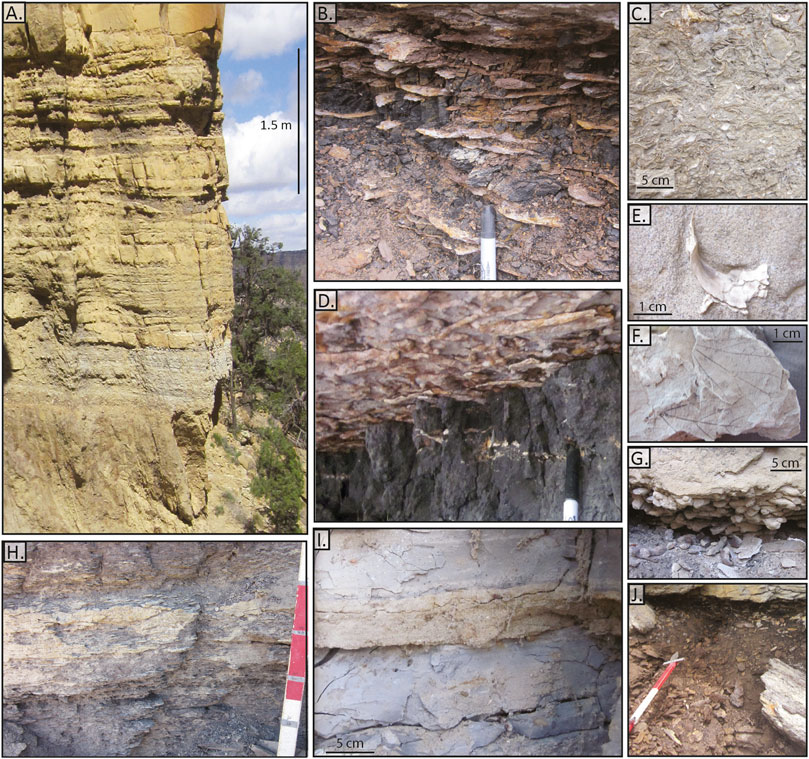
FIGURE 3. Facies association 1: back-barrier fill. (A) Outcrop photo of backbarrier fill with interbedded sandstone lenses. (B) Coal reworked with flaser-to lenticular-bedded fine-grained sand lenses. (C) Oyster shell fragments in a sand matrix. (D)Thalassinoides burrows on the base of a fine-grained sandstone bed overlying coal deposits. (E) Oyster shell fragment. (F) Leaf impressions. (G)Thalassinoides within coal at the base of a medium-grained sandstone. (H) Carbonaceous mudstone with fine- to medium-grained sandstone lenses. Red and white paint on Jacob staff 25 cm while marks are 10 cm apart. (I) Medium-grained sandstone bed within gray mudstone with abundant terrigenous material and leaf impressions. (J) Interval of brown carbonaceous mudstone grading vertically into coal.
Interpretation.
Backbarrier
Despite being a distinct depositional environment separate from the barrier island itself, the backbarrier facies are included in the facies descriptions outlined here because they are commonly recognized in association with barrier islands in contemporary models. Barrier islands can impound both lagoons and wave-dominated estuaries, therefore the term backbarrier is used henceforth to describe both settings.
The fine-grained mudstones and coals suggest deposition within a protected and low energy backbarrier setting. Coals and abundant terrigenous material (Figure 3F) along with oyster shell fragments (Figures 3C,E) suggest both terrestrial and marine influence (Kieft et al., 2011; Painter et al., 2013; Chentnik et al., 2015). Flaser to lenticular bedding suggests tidal reworking and the development of tidalites within the sandstone/mudstone alternations (Figure 3B; Reineck and Singh, 1980; Dalrymple, 2010). Medium-grained, wavy, lens-shape, sandstone beds which interfinger with the mudstones (Figures 3A,I) are interpreted as the distal expression of storm-driven washover fans (cf. Sedgwick and Davis, 2003). Vertical gradation from carbonaceous mudstone to coal (Figure 3J) suggests cyclic wetting upwards (Wadsworth, 2010). Thalassinoides burrows typically found at the top of the coals indicate marine influence and thus a relative deepening or transgression (Figures 3D,G; Savrda, 1991; Carvalho et al., 2007). The thin-walled nature and low abundance of peridinioid dinoflagellates suggests brackish water conditions (Pocknall et al., 2016).
Facies Association 2
Description
Fine- to Medium-Grained, Tabular Sandstones
This facies is composed of fine-grained, 0.1–1 m thick, tabular sandstone beds containing hummocky and swaley cross-stratification (Figure 4A). Beds have sharp bases and grade vertically into finer grained muddy to silty interbeds. These beds continuously grade vertically into medium-grained, 0.5–1.5 m thick, tabular sandstone beds containing low-angle trough cross-stratification, and planar bedding (Figure 4D). Trough packages in these beds range from 5 to 50 cm in height and are bound by indistinct sand on sand surfaces without a noticeable change in grain-size. These notably “blocky” sandstone beds (Figure 4D) stack vertically to form tabular, laterally continuous outcrops (>30 m wide; Figure 4B). In some areas, the internal character of these beds alternates from laminated and/or homogenous to completely bioturbated (Figure 4G). Bioturbated layers contain abundant and pervasive Ophiomorpha nodosa sharp bases and transitional tops (Figures 4E‒G).
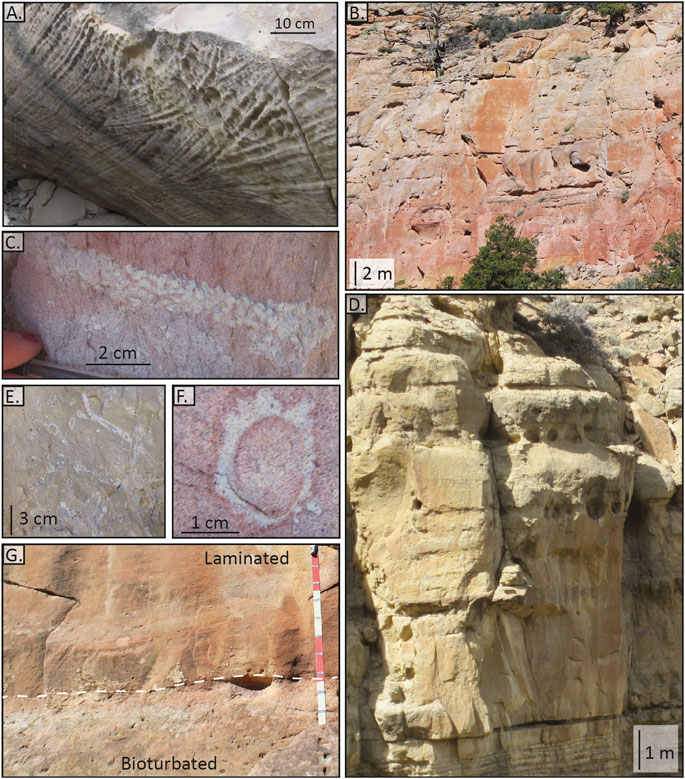
FIGURE 4. Facies association 2: lower to upper shoreface. (A) Hummocky and swaley cross-stratification in a down-dropped block. (B) Blocky outcrops with alternating layers of lamination and bioturbation. (C)Ophiomorpha nodosa. (D) Blocky, fine-grained sandstone with tabular laterally continuous beds. (E) Biotubated bed showing Ophiomorpha nodosa. (F) Cross-section through Ophiomorpha nodosa.(G) Contact between heavily bioturbated bed and overlying laminated bed. Red and white paint on Jacob staff 25 cm while marks are 10 cm apart.
Interpretation
Lower to Upper Shoreface
These sandstones are interpreted as proximal lower and distal upper shoreface deposits. Lower shoreface strata are recognized by the presence of hummocky and swaley cross-stratification (Figure 4A; Dumas, 2005; Dumas and Arnott, 2006), confirming storm-influenced deposition below fair-weather wave base. These storm-dominated facies grade vertically into fair weather wave-dominated facies, evidenced by the gradual transition to medium-grained, trough cross-stratification and planar laminated sandstones (Figure 4D). These wave energy indicators suggest deposition above fair-weather wave base in an upper shoreface environment (Reading and Collinson, 1996; Plint, 2010). Ophiomorpha nodosa (Figures 4C,E,F) is common in shoreface settings (Frey and Howard, 1985; Droser and Bottjer, 1989). Alternating laminated and heavily bioturbated bedding (Figures 4B,G), also called “lam-scram” (MacEachern and Pemberton, 1992; Bann and Fielding, 2004; Pemberton et al., 2012), indicate periods of rapid deposition, creating laminated and homogenous layers, followed by periods of quiescence during which the sands are bioturbated (Figure 4G). The presence of both storm and fair-weather wave influence makes this facies association distinctive.
Facies Association 3
Description
Medium-Grained, Cross-Stratified Sandstone
This facies is composed of medium-grained sandstone beds with abundant trough cross-stratification, accretion sets (Figures 5A,D), ripple laminations (Figure 5E), and bidirectional paleocurrent indicators (trough cross-stratification, accretion sets, and ripple laminations; Table 2). Accretion sets and ripple laminations range from 2 to 10 cm thick while trough cross-stratification ranges from 5 to 50 cm thick. Medium-sized sand grains concentrate along stratification laminations, adding to the laminated texture of this facies and defining cosets. Trough cross-stratification is orientated west to east while accretion sets are oriented south (Table 2). The beds are 0.1–0.5 m thick and generally lack bioturbation, but occasionally overlie bioturbated layers (Figure 5B). Beds contain sporadic abraded shell fragments <1 cm in diameter. These beds stack to form blocky outcrops (Figure 5C). These deposits do not show any evidence of internal dissection or lens-shaped sandstone bodies, beds do not have sharp or distinct surfaces, instead are marked by sand on sand boundaries defined by a change in lamination orientation (Figure 5C).
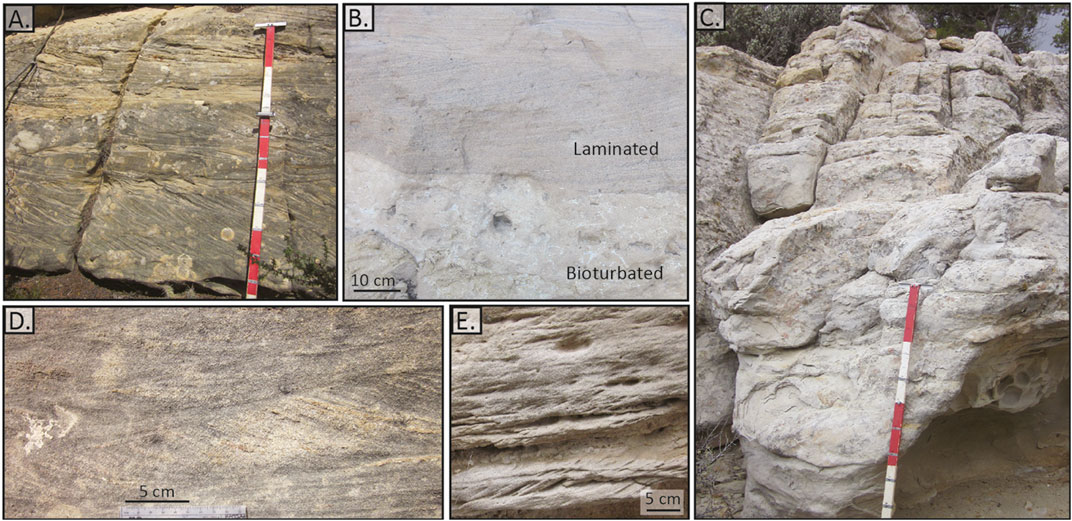
FIGURE 5. Facies association 3: proximal upper shoreface. (A) Sandstone beds showing stacked accretion sets. Red and white paint on Jacob staff 25 cm while marks are 10 cm. (B) Medium-grained sandstone with clear accretion sets cutting into heavily bioturbated strata with clear Ophiomorpha. (C) Outcrop photo showing the blocky character. (D) Sandstone with uni-directional accretion sets. (E) Ripple cross-laminated sandstone.
Interpretation
Proximal Upper Shoreface
The medium-grain size and abundance of accretion sets (Figures 5A,D) and ripples suggest deposition in a high-energy setting, dominated by tide and shore-parallel current energy (Figure 5E; Allen, 1982; Boothroyd, 1985; Longhitano et al., 2012). Accretion sets have a dominant shore parallel orientation (north to south) in some areas, suggesting current influence was dominant. In other areas bed-scale bidirectional paleocurrent indicators (grainsize laminations and bidirectional accretion sets) suggest either tidal influence or longshore transport reversals (Table 2; Dooling, 2013; Chentnik et al., 2015). The alternation of medium and fine grains along laminations also suggests rhythmic tidal influence. These beds lack bioturbation, further indicating high-energy deposition (Hubbard et al., 2002; Dashtgard et al., 2009; Steel et al., 2012). The abundance of high-angle trough cross-stratification and accretion sets (Figure 5D) is indicative of a barred beach system (Isla et al., 2020) and distinguishes this proximal, higher-energy upper shoreface from the upper shoreface of facies association 2, which is dominated by wide (>0.15 m), more sporadic, trough cross-stratification (Figures 4A,D). These proximal upper shoreface deposits lack hummocky and swaley cross-stratification indicative of lower shoreface settings.
Facies Association 4
Description
Lens-Forming, Fine-Grained Sandstone
This interval is composed of laterally discontinuous, lens-shaped bedforms (0.5–2.0 m thick; Figure 6A) of fine- and medium-grained sandstone with erosive bases, cross-cutting each other over 1–10 m laterally (Figure 6H). These sand bodies are highly variable, forming both tabular and lens-shaped beds with a variety of internal bedforms and patterns including abundant trough cross-stratification (Figure 6A), ripple laminations (Figure 6F), accretion set packages (1–5 cm thick; Figure 6I), bi-directional cross-stratification (Table 2; Figure 6J), and convolute bedding (Figure 6E). Mudstone clasts (2–15 mm long) and shell fragments line the basal surfaces and are arranged along bedding planes within sand-bodies. Shell impressions (Figure 6B), shell fragments, double mud drapes (Figures 6I,J), and bioturbation are present (Figures 6C,D,G). The basal surface of some sandstone bodies is heavily bioturbated with Gastrocheanolites (Figures 6D,G) and Teredolites (Figure 6C).
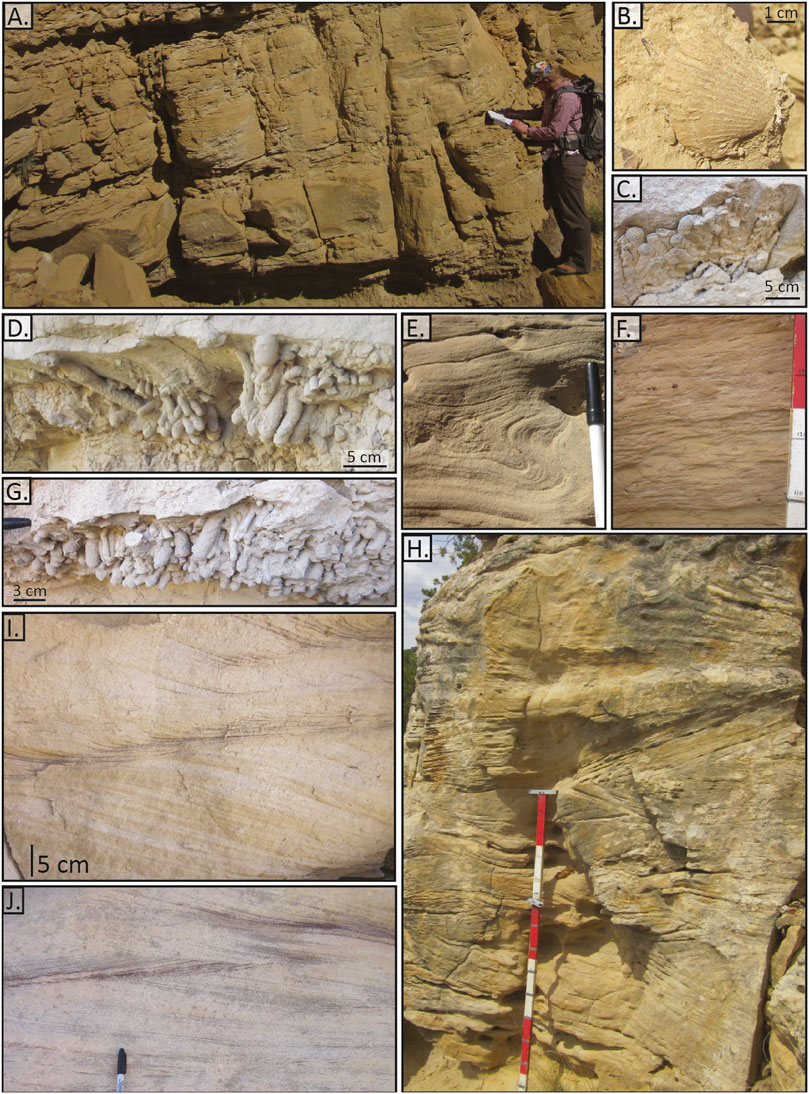
FIGURE 6. Facies association 4: tidal channels. (A) Outcrop with an incisional base and internal trough cross-stratification and variable bed thicknesses. (B) Bivalve shell impression. (C)Teredolites trace fossils preserving borings into wood. (D)Gastrocheanolites. (E) Convolute bedding. (F) Ripple laminated bed. Red and white paint on Jacob staff 25 cm while marks are 10 cm apart. (G)Gastrocheanolites. (H) Outcrop photo showing the cross-cutting and convolute bedding. (I) Double mud drapes and accretion sets indicated by changes in sand color. (J) Bi-directional accretion sets with double mud drapes.
Interpretation
Tidal Channels
These variable sandstones are interpreted as tidal channels. Erosive basal surfaces (Figure 6A), internal cross-cutting (Figure 6H), and high-angle trough cross-stratification suggests channelized flow. Bi-directional accretion sets and double mud drapes (Figures 6I,J) are indicative of tidal processes (Barwis and Hayes, 1979; Hayes, 1980; Nio and Yang, 1991; Longhitano et al., 2012). Brackish trace fossils Teredolites (Figure 6C) and Gastrocheanolites (Figures 6D,G) and shell impressions (Figure 6B) indicate deposition in an area with both marine and freshwater input. Mudstone rip-up clasts suggest erosion and reworking of underlying or adjacent material. Convolute bedding (Figure 6E) suggests dewatering and rapid deposition (Dzuynski and Smith, 1963).
Internal Geometry of Architectural Elements
The four facies associations (Table 2) stack in distinct ways to create three barrier island architectural elements, which we document using measured sections (Figure 7) and outcrop photos (Figure 8), summarized in Figure 9. The outcrop geometries and lateral stacking patterns are discussed in the following section.
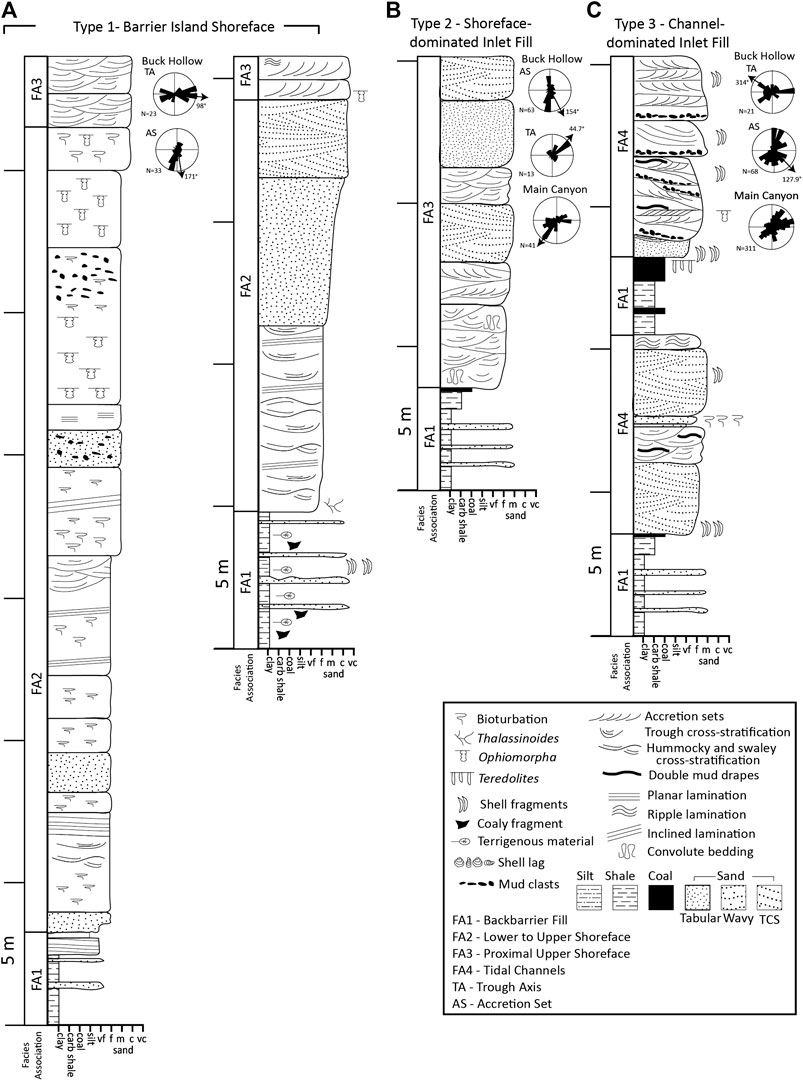
FIGURE 7. Measured sections of the (A) barrier island shoreface, (B) shoreface-dominated inlet fill, and (C) channel-dominated inlet fill architectural elements, with representative paleocurrent measurement data from Buck Hollow and Main Canyon.
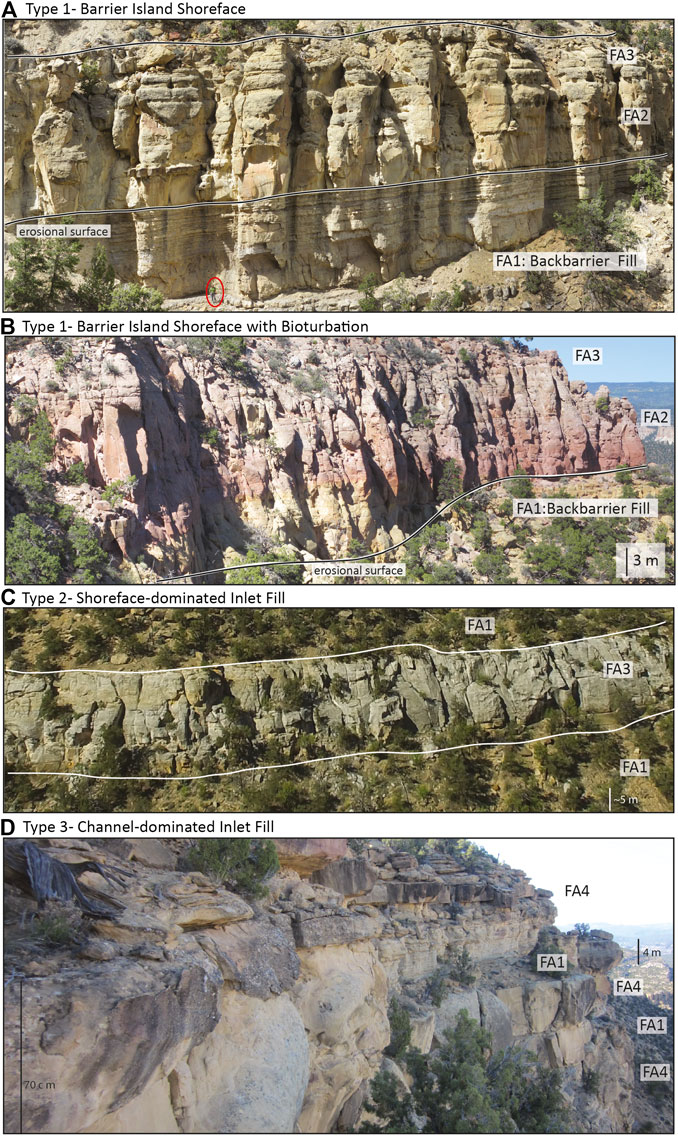
FIGURE 8. Outcrop photos showing (A) barrier island shoreface, (B) barrier island shoreface with alternating bioturbated and laminated beds, (C) shoreface-dominated inlet fill, and (D) channel-dominated inlet fill. Facies associations (Table 2) are labeled in each photo include backbarrier fill (FA1), shoreface (FA2), proximal upper shoreface (FA3), and tidal channels (FA4). Note the two scale bars showing the perspective in the barrier island tidal channel photo (D).
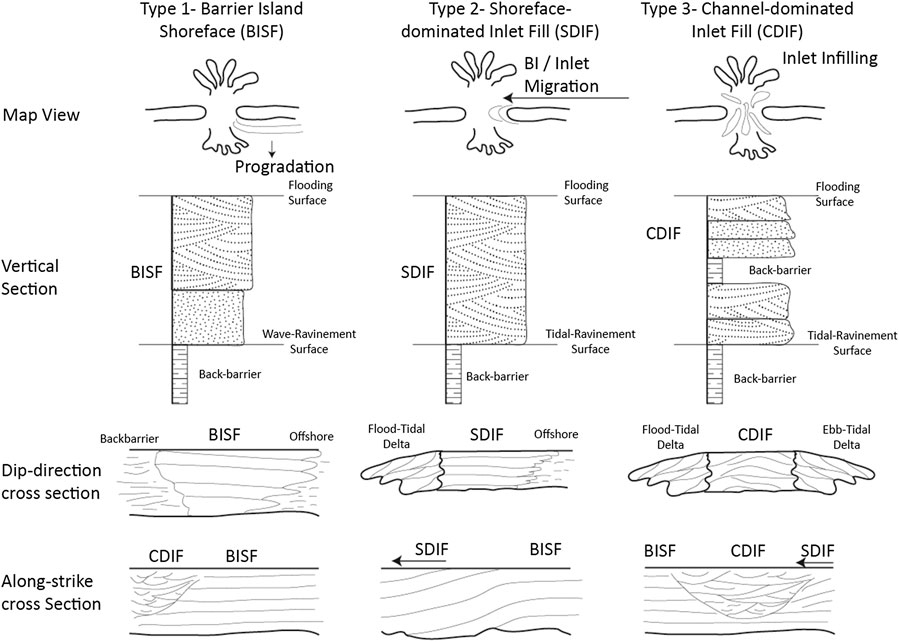
FIGURE 9. Summary of the three barrier island architectural elements showing the facies variation and one set of possible along-strike and dip-direction configurations. BISF, barrier island shoreface; SDIF, shoreface-dominated inlet fill; CDIF, channel-dominated inlet fill.
Type 1: Barrier Island Shoreface
Description
Barrier island shoreface (BISF) elements are composed of 1 to >10 m of carbonaceous mudstone of backbarrier fill (FA1), overlain by a sharp-based, cliff-forming sandstone interval of lower to upper shoreface followed by a relatively thin (2–5 m thick) proximal upper shoreface (FA2 and FA3; Figure 7A). These sand dominated intervals fill voids that were variably eroded into the underlying backbarrier (Figures 8A,B), in some locations sands interbedded with the underlying mudstones (Figures 3A,I), which grade vertically into coals in places (Figure 3J). A sharp contact separates these finer-grained facies from an overlying, blocky, cliff-forming, fine-to medium-grained, lower shoreface sandstone unit (>5 m thick; FA2; Figures 8A,B), which is typically laterally continuous over >2 km. The uppermost portion of the cliff-forming interval contains beds 0.2–1 m thick with high-angle trough cross-stratification with bidirectional paleocurrent indicators (FA3; Figure 7A).
Interpretation
Barrier island shoreface elements (Figures 7A, 8A,B) encompass the lower to upper shoreface (FA2) and proximal upper shoreface (FA3), which rest directly atop backbarrier facies (FA1). In these examples, proximal lower shoreface deposits directly overlie carbonaceous backbarrier fill and coals (Figures 8A,B), offshore and distal lower shoreface deposits are not observed. This suggests non deposition of these more distal facies and/or the preferential preservation of the higher-energy facies. The sharp base and alternating bioturbated and laminated intervals suggest rapid and episodic deposition (MacEachern and Pemberton, 1992; Bann and Fielding, 2004; Pemberton et al., 2012). Bidirectional paleocurrent indicators in the uppermost portion of the sequence suggest tidal reworking (Figure 7A).
Type 2: Shoreface-Dominated Inlet Fill
Description
These elements (Figure 7B) are composed of carbonaceous mudstones (FA1) overlain by erosive-based, cliff-forming sandstone units (>5 m thick) composed of trough cross-stratified medium- to coarse-grained sandstone beds (0.20–0.50 m thick) lacking bioturbation (FA3). The sandstone dominated intervals are blocky and tabular (Figure 8C), with internal beds (0.5–1 m thick) composed of trough cross-stratification (north-east dipping; Figure 7B), mainly unidirectional accretion sets, convolute bedding, ripple laminations, homogenous bedding, and clear bidirectional paleocurrent indicators (north-south dipping; Figures 5, 7B).
Interpretation
Shoreface-dominated inlet fill elements (Figure 7B) are composed of proximal upper shoreface sands (FA3) that stack vertically to create sandstone sheets which are continuous in the along-strike direction >4 km laterally (Figure 8C). Where these overlie the backbarrier deposits (FA1), the inlet fill records the preservation of a barrier island migrating laterally (shore-parallel) into the empty space of a tidal inlet (Anthony et al., 1996; Reddering, 1983). In contrast to the models of inlet fill where the supratidal barrier and washover elements fill the accommodation provided by inlets (Buynevich, 2019; Green et al., 2019), here the proximal barrier island shoreface has filled the inlets preferentially. This has led to the preservation of upper shoreface sandstones reworked by high-energy tidal and current processes (Figure 7B). The blocky, large (>5 m thick) outcrops (Figure 8C) formed by these deposits suggest they are not channelized or fan-shaped. Rather these deposits are notably laterally extensive (>4 km), similar to tidal inlet outcrops described previously (Uhlir et al., 1988). Outcrops with unidirectional accretion sets (Figure 5A) and ripples (Figure 5E) support a dominant direction of lateral (N-S) accretion driven by longshore sediment transport, while bidirectional flow indicators suggest tidal reworking (Figure 7B; Dooling, 2013; Chentnik et al., 2015). Trough cross-stratification is preserved within as nearshore coast migrating bedforms (0.5–1 m thick; Plint, 2010), oriented oblique to the interpreted shoreline direction. These deposits may have low angle (<3°), bed-scale, lateral accretion clinoform surfaces (e.g., Siringan and Anderson, 1993) recognizable in outcrop exposures of <1 km lateral distance. The blocky, amalgamated character of these outcrops (Figures 5, 8C) likely came from the stacking and migration of multiple tidal inlet depositional events (Heron et al., 1984). These deposits also preserve the shoreface just distal of the inlet for a prolonged time period as the island feature migrated, merging this facies type with barrier island shoreface deposits laterally (Figure 9).
Type 3: Channel-Dominated Inlet Fill
Description
These elements are composed of inter-fingered intervals of carbonaceous mudstones and coals (FA1) that are locally scoured by lens-shaped sandstone outcrops (>5 m tall, >10 m wide; Figure 8D). These finer-grained intervals have abundant carbonaceous and terrigenous material (FA1). The thickness of these mudstone-dominated intervals varies as they are eroded by the sandstone-dominated intervals. Internally, the sand dominated intervals are composed of lens-shaped, fine-to medium-grained sandstone beds (FA4; Figure 7C) which locally fine upwards. These beds are 0.5–2.0 m thick and contain trough cross-stratification, double mud drapes, ripple laminations, brackish trace fossils, and shell impressions (FA4; Figure 6).
Interpretation
These lens-shaped sand-dominated outcrops are interpreted as channel-dominated inlet fill elements (Figure 7C). They are composed of vertically and laterally stacked channelized tidal sandstones (FA4; Figure 8D) sometimes interbedded with backbarrier fill (FA1; Figure 7C; Tye and Moslow, 1993). Neither dunes nor bars (Olariu et al., 2012), these outcrops are interpreted as channels based on the lens-shape and erosive characteristics of the internal bed forms (Figures 6A,H), as well as abundant trough cross-stratification. The combination of channel features and tidal indicators (bi-directional accretion sets and mud drapes, both single and double; Figure 7C) make these deposits similar to other tidal inlet channel fill successions (Willis and Moslow, 1994a; Kieft et al., 2011) and modern channelized inlet fills (Kumar and Sanders, 1974; Israel et al., 1987). These plug-like lenses, laterally and vertically stacked and offset to cut in to one another, create a three-dimensional geometry similar to the architecture of tidal inlets observed in seismic section (Ronchi et al., 2019) and in ground penetrating radar from modern barrier islands (FitzGerald et al., 2012). These channel-dominated deposits can be distinguished from shoreface-dominated deposits by their internal geometry and mud content. Shoreface-dominated inlet fill deposits (described above; Figure 8C) are blockier and more uniform, lacking the channel-form geometries and backbarrier interbeds seen in channel-dominated inlet fill deposits (Figure 8D). Furthermore, channel-dominated inlet fill deposits likely record deposition in the more central or more proximal portion of the inlet, rather than the margin or a more distal portion (Heron et al., 1984).
Channel-dominated inlet fill records vertical aggradation during periods of localized high accommodation and inlet deepening. These deposits vary from shoreface-dominated inlet fill architectural elements, which record the lateral extension of the barrier island shoreface into the tidal inlet. Modern analogs often have tidal channels located close to, or contiguous with, barrier island shoreface or tidal delta deposits (FitzGerald et al., 2012). This position, near the open ocean, makes them distinct from deltaic tidal channels and backbarrier tidal channels, which are located in more proximal areas of the backbarrier (Flores, 1978). Channel-dominated inlet fill elements are distinct from deltaic tidal channels because they are not associated with prodelta or delta front facies (Rahmani, 1988). They are distinct from tidal channels within the backbarrier (e.g., open estuarine or lagoonal tidal channels) because they do not show the systematic shallowing-upward patterns characteristic of backbarrier tidal channels (Hughes, 2012), and are not associated with more proximal tidal facies such as tidal flats, bars, or tidally-influenced fluvial deposits (Dalrymple and Choi, 2007).
Outcrop Geometries and Stacking Patterns of Architectural Elements
The three barrier island architectural elements are summarized in Figure 9. Outcrop examples of the Straight Cliffs Formation from Alvey Wash and Buck Hollow show the lateral and vertical variability within barrier island strata (Figures 10–12) deposited during a relatively short (∼4 my) time period (C-F intervals of John Henry Member; Figure 2C), and within a small region (∼20 km2, Escalante area combined; Figure 2). The variability of these barrier island facies highlights the range of ways these deposits can be preserved and creates an initial foundation for the description and interpretation of barrier islands.
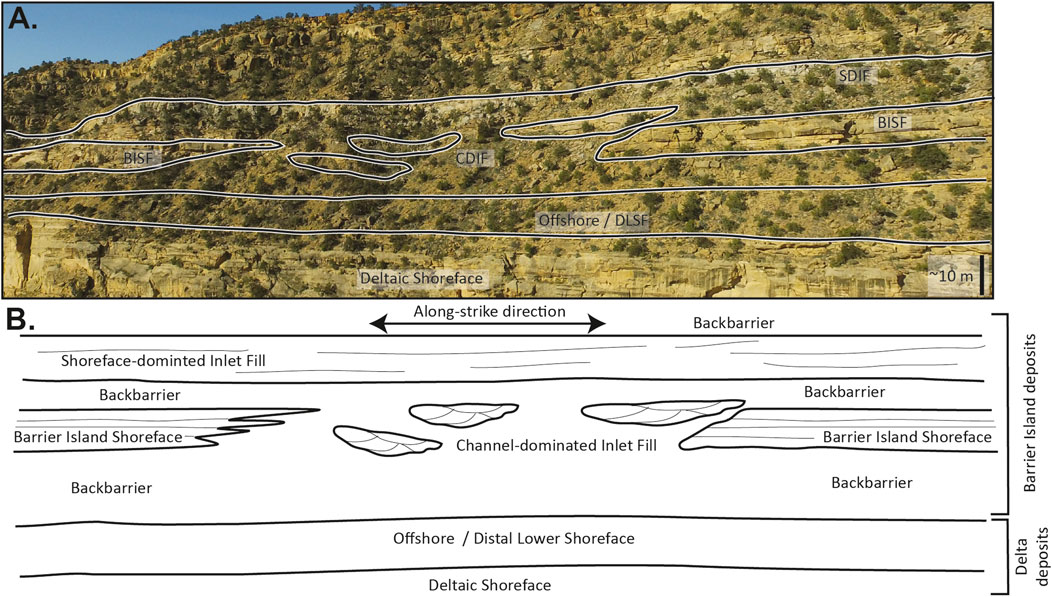
FIGURE 10. (A) Depositional strike orientated outcrop photo from Alvey Wash and schematic (B) interpretation showing a barrier island shoreface (BISF) grading laterally into channel-dominated deposits (CDIF). This composite barrier island, containing both barrier island shoreface and channel-dominated inlet fill elements, is overlain by an interval of backbarrier fill followed by a shoreface-dominated inlet fill (SDIF), which extends across the entire outcrop. DLS, distal lower shoreface.
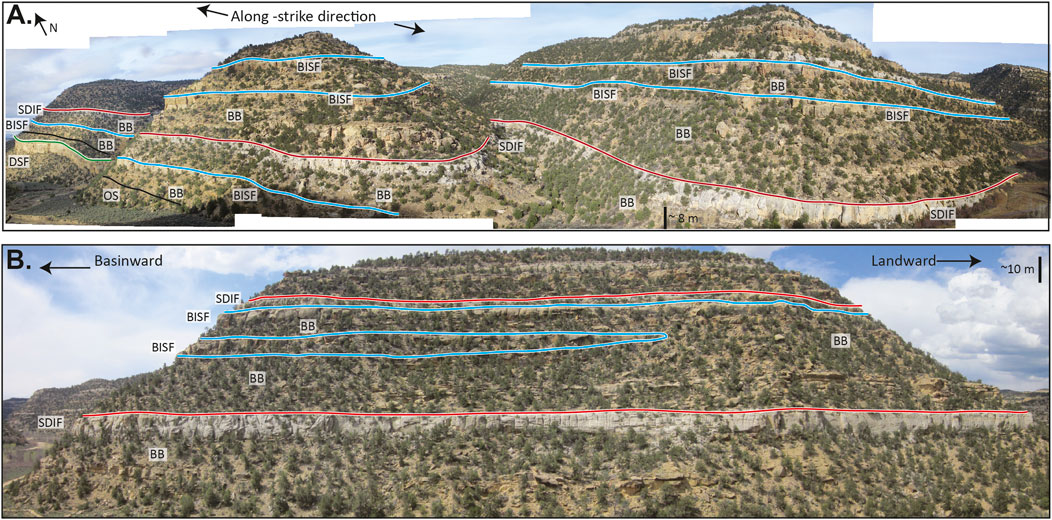
FIGURE 11. (A) Outcrop panel from Alvey Wash oriented oblique to depositional strike showing the lateral extent of a shoreface-dominated inlet fill (SDIF; red). Barrier island shoreface deposits (BISF; blue), channel-dominated inlet fill (CDIF), backbarrier fill (BB), deltaic shoreface (DSF; green), and offshore (OS; black) deposits are also marked. Note scale bar changes with perspective as the photos were taken obliquely. The entire exposure is roughly 1.8 km long. The vertical relief in the foreground is 190 m the vertical relief of the far left (north) side is 250 m. (B) Dip oriented outcrop panel from Alvey Wash showing a shoreface-dominated inlet fill (SDIF; red) with sharp upper and lower contacts. A barrier island shoreface (BISF; blue) pinches out in the middle of the outcrop. Towards the top there is a laterally continuous barrier island shoreface (BISF; blue) overlain by another shoreface-dominated inlet fill (SDIF; red). Backbarriers (BB) and with outcropping tidal channels are also marked. The exposure shown has ∼200 m elevation change and is 0.6 km across.
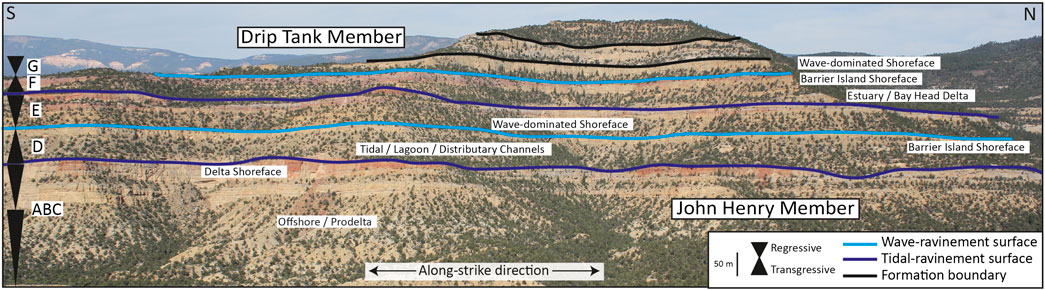
FIGURE 12. Interpreted aerial photograph of an exposure parallel to depositional strike from Buck Hollow showing the relationship of barrier island shoreface deposits relative to other paralic environments. Barrier islands cap the D and F transgressive intervals. Outcrop panel is roughly 1.5 km wide in the strike direction (south to north), has 0.7 km of depth perspective from the foreground to the peak of the mountain (east to west direction), and shows ∼400 m of elevation change. Letters on the side (A–G) refer to the different intervals of the John Henry Member.
Lateral Facies Patterns
The three barrier island architectural elements can occur in a variety of lateral (along-strike; Figures 10, 11A, 12) and shore-perpendicular (dip-direction; Figure 11B) arrangements. Barrier island shoreface elements grade laterally into correlative channel-dominated inlet fill elements in Alvey Wash (Figure 10), similar relationships have been recognized in other examples (Davies and Ethridge, 1971; Davies, 1978; Flores, 1978; Self et al., 1986). The preservation of channel-dominated inlet fill, rather than laterally migrating shoreface-dominated facies, suggests that the inlet was either stationary or relatively short-lived and could be filled efficiently. Green et al. (2019) provide modern analogues for the generation and sealing of stationary and ephemeral inlets and show how these may fill in a matter of days due to overwash.
Shoreface-dominated inlet fill deposits are consistent and horizontally continuous along strike in Alvey Wash (>4 km; Figure 11A), extending the length of the outcrop exposure (>1.82 km). This suggests the migration of a barrier island through lateral infilling and across the length of the Alvey Wash field area (∼5 km by ∼1 km). In Buck Hollow, barrier island shoreface deposits are laterally continuous along strike to the south (>5 km; Figure 12) but grade into shoreface-dominated inlet fill deposits along strike to the north (∼1 km north of Figure 12 location). This lateral facies change is similar to subsurface examples which also show lateral transition between shoreface and inlet fill deposits (Galloway, 1986; Hubbard et al., 2002). As these deposits form continuous cliff bands we suggest they are indicative of inlet migration over long timescales; the gradation indicating the closing of a tidal inlet through lateral migration and infilling. This geometry is not surprising as the shoreface extends across the tidal inlet opening in the modern, outward channel and lobes of the ebb-tidal delta (Figure 1).
Shoreface-dominated inlet fill deposits also show distinct shore-perpendicular continuity (>1 km; Figure 11B) which could result from compound barrier island motion, as shore-perpendicular and lateral motion occur simultaneously (Heron et al., 1984). During oblique motion, sediment temporarily located within the inlet as bars and flood- and ebb-tidal deltas is eroded and sequestered into a combination of shoreface-dominated inlet fill and barrier island shoreface deposits. Barrier island shoreface examples are laterally continuous in the dip direction across the outcrop exposure (Figure 11B), indicating shore-perpendicular progradation of the island system. Other barrier island shoreface deposits are seen pinching out in the updip-direction (Figure 11B) where they interfinger with backbarrier fill facies, suggesting preservation of the proximal part of the island, perhaps withstanding erosion due to cohesive mud interbeds.
Vertical Stacking Patterns
Barrier island deposits also show a variety of vertical stacking patterns. The outcrops at Buck Hollow preserve barrier islands during the transgressive portion of two regressive-transgressive cycles, which are likely are relatively short about ∼1 my each (based on four main regressive-transgressive cycles over ∼ 4 my deposition), and dominated by backbarrier fill deposition (Figure 12; Mulhern and Johnson, 2016). Barrier island shoreface deposits probably record relatively rapid deposition at the end of periods of backbarrier infilling. Both examples of barrier island shorefaces in Buck Hollow are sharp-based cliffs directly overlying backbarrier deposits (Figure 12). Regionally significant flooding surfaces truncate the upper portion of the barrier islands shorefaces, separating them from overlying offshore marine facies. These Buck Hollow barrier island shoreface deposits formed during regional transgression and correlate to an incised valley fill succession ∼15 km up-dip (Chentnik et al., 2015).
The John Henry Member at Alvey Wash provides a more complicated stacking pattern. Here, some barrier island shoreface deposits are overlain by carbonaceous backbarrier fill followed by a shoreface-dominated inlet fill succession (Figure 10). Other barrier island shorefaces are stacked vertically, separated by backbarrier fill (Figure 11B). Higher in the section, a barrier island shoreface is capped with shoreface-dominated inlet fill deposits (Figure 11B). Literature examples also show other barrier island stacking patterns such as barrier island shoreface deposits directly overlying a deltaic sequence (McCubbin, 1982) and strandplain shoreface deposits overlain by shoreface-dominated inlet fill deposits (Cheel and Leckie, 1990). Thus, we consider the John Henry Member at Alvey Wash to preserve a relatively stationary, aggradationally stacked, barrier island and backbarrier system deposited during roughly the same time period as at Buck Hollow. This portion of the basin underwent smaller scale shifts in the relative position of the shoreline, resulting in a long-standing backbarrier system with more complex and variable stacking patterns (Figures 10, 11). Combined, these examples show that the three barrier island architectural elements defined here can be found in a variety of lateral and vertical patterns, underscoring the dynamic nature of barrier island systems as seen in modern examples (McBride et al., 2013; Moore and Murray, 2018).
Discussion
Summary of Barrier Island Recognition Criteria
The barrier island facies, architectural elements, and deposits documented here can be distinguished from shorefaces of other coastal setting and paralic systems, as well as other transgressive sub-environments. We base this on three main criteria: 1) proximity to backbarrier deposits, 2) internal facies and outcrop geometry, and 3) stratigraphic position. These criteria are focused on observations that can be made in outcrop, and expand the sequences proposed for early interpretations to a more comprehensive three-dimensional model (Berg and Davies, 1968; Berg, 1970). These criteria allow barrier islands to be identified in the rock record despite key departures from the examples of modern islands upon which previous models were based, including true island morphology (Schatzinger et al., 1989; Reinson, 1992).
Proximity to Backbarrier Deposits
The barrier island deposits of the Straight Cliffs Formation are found overlying or between backbarrier intervals, within broadly transgressive successions (Figure 12). Although backbarrier deposits are not actually part of the barrier island itself, they are included in the descriptions herein because they generally occur in strong association with barrier islands and thus help recognize and differentiate barrier island deposits from other preserved depositional environments, especially the shoreface. In modern systems, barrier islands are sand bodies that bound lagoons and/or estuaries. In some high accommodation settings, like Alvey Wash, these sub-environments are preserved as shoreface, either directly overlying or time-correlative to, backbarrier deposits. However, this criterion needs to be interpreted in conjunction with other indicators to rule out other possible options. While the examples from Alvey Wash and Buck Hollow directly overlie back barrier deposits (Figures 7, 8), barrier islands can also be time-correlative with backbarrier facies (Boyd and Dyer, 1964; Bibler and Schmitt, 1986; Davies et al., 2006). Similar facies associations develop in systems where there is high littoral sediment supply (e.g., mainland-attached baymouth bar and spit systems), or where large transgressive barrier dunes front coastal plains backed by coastal waterbodies. In many instances, these facies associations evolve from barrier islands either through spit growth and eventual closure of embayments, or they amalgamate to form a contiguous mainland attached sand body (e.g., the coastal waterbodies of SE Africa; Wright et al., 2000; Benallack et al., 2016; Dladla et al., 2019). In contrast, strandplains lack backbarriers (Galloway, 1986), and instead, fluvial and coastal plain facies are the correlative proximal time-equivalent facies to strandplain shoreface deposits (Clifton, 2006). Similarly, deltas are associated with delta plains, coastal plain, and fluvial systems, rather than backbarriers (Cotter, 1975; Bhattacharya and Giosan, 2003; Hampson and Howell, 2005).
Backbarrier deposits can be difficult to interpret from outcrop, and with only cursory investigation, can be confused with both offshore or shelfal marine mudstone deposits and coastal plain deposits. Backbarrier facies can be distinguished from coaly coastal plain deposits by the presence of shell fragments (Figure 3C), particularly oyster shells (Figure 3E), and by marine trace fossils, such as Thalassinoides (Figures 3D,G), which can burrow into the upper layer of backbarrier fill following a marine incursion (Donselaar and Nio, 1982; Savrda, 1991; Carvalho et al., 2007). Overwash, or encapsulation of flood-tidal deltaic sand bodies is expected (Reinson, 1992). Backbarrier deposits can show gradationally increasing carbonaceous material vertically (Figure 3J), and are capped with coals (Figure 3D), suggesting wetting-upward cycles (Wadsworth, 2010). In contrast, coastal plain coal cycles are linked to floodplain dynamics such as avulsion and channel abandonment, and more likely to preserve root traces (McCabe, 1987; Kieft et al., 2011). Offshore and shelfal marine mudstones tend to be gray to black, clay rich, mm-scale planar to wavy laminated mudstones (Macquaker et al., 2007) which can contain marine shell fragments such as Inoceramids, depending on depositional age. They typically lack abundant carbonaceous or terrigenous material, coals, and oyster shells. Biostratigraphy (e.g., pollen, spores, nannofossils, dinoflagellates, and foraminifera) can be used to identify open-marine, brackish, and fresh water conditions based on the type and abundance of fossils preserved (Leckie, 1987; Eaton, 1991; Tibert and Leckie, 2004).
Geometry and Internal Facies
Barrier islands have more variable internal facies and outcrop geometries than shorefaces preserved from other examples because of their multi-dimensional motion through time. The barrier island deposits described here have sharper bases than classic wave-dominated shorefaces (Boyd, 2010; Plint, 2010), missing the offshore and distal lower shoreface components. They also have the added complexity of evidence for lateral motion evidenced by shore-parallel paleocurrent indicators and significant tide and wave reworking. Similar to normal shorefaces, barrier island shoreface deposits also have storm indicators (hummocky and swaley cross-stratification; Dumas and Arnott, 2006) as well as evidence of rapid deposition (homogenous beds, alternating bioturbated and laminated beds). Deltaic and strandplain shorefaces typically preserve offshore and distal lower shoreface deposits (Reading and Collinson, 1996). Our data indicate that these facies may not be preserved in barrier island settings (Figure 7) and this relationship may be used as an indicator. At the outcrop scale, barrier island shoreface deposits can be less laterally continuous and more stratigraphically heterolithic than strandplain shorefaces (Wilkinson et al., 1975; Heward, 1981; Reading and Collinson, 1996). Along-strike, the different barrier island architectural elements (shoreface, shoreface-dominated inlet fill, channel-dominated inlet fill) can grade into one another (Figures 9, 10). The degree of reworking and variability is probably a function of the available accommodation, rate of relative sea-level change, and the local hydrodynamic regime (wave, tidal, storm, and current energy). The variability possible in the outcrops also makes it difficult to correlate and identify genetically related and time contemporaneous deposits from one location to the next.
A sharp-based shoreface geometry is indicative of, but not exclusive to, barrier islands: it also occurs in deltaic deposits as a result of high sediment supply and rapid progradation (Pattison, 1995; Willis and Gabel, 2001; Bhattacharya and Giosan, 2003). Delta-influenced shorefaces show clear upward coarsening, may show a greater amount of terrigenous material, and may also be associated with episodic waning flow events (e.g., hyperpycnites and Bouma sequences) and clinoform geometries (Bhattacharya, 2010; Olariu et al., 2010; Feldman et al., 2014). Forced regression also can place sharp-based shoreface successions directly over more distal marine facies (Posamentier et al., 1992; Plint, 2010). As a result, sharp-based barrier island deposits should be considered in the context of other recognition criteria and indicators.
High-energy, proximal upper shoreface deposits (FA3) at the top of barrier island shoreface successions (Figure 7A) may resemble tidal bars or tidal channels, which can erode into shoreface deposits via tidal ravinement (Figure 13). Proximal upper shoreface deposits associated with barrier islands are vertically continuous, grading upward from the underlying shoreface (Figure 13A). These beds are part of the blocky sandstone outcrop expression and are laterally continuous. They contain high-energy bedforms including distinct, high-angle accretion sets, and small scale, high-angle trough cross-stratification (0.05 m tall). In contrast, tidal channels and tidal bars tend to have erosive bases, cutting down into underlying deposits and creating a tidal ravinement surface, which forms a distinct break in the outcrop (Figure 13B). These deposits can be part of the same cliff band as the shoreface below, but more often they are recessed back, creating a discontinuous layer. Internally, tidal channels and bars contain stacked accretion sets with bi-directional paleocurrent indicators, in addition to multidirectional trough cross-stratification (Chentnik et al., 2015; Mulhern and Johnson, 2016). Proximal upper shoreface deposits show a gradational increase in signals of tidal energy in the upper portion of the shoreface (Dashtgard et al., 2010), but lack evidence of basal tidal ravinement (Figure 7).
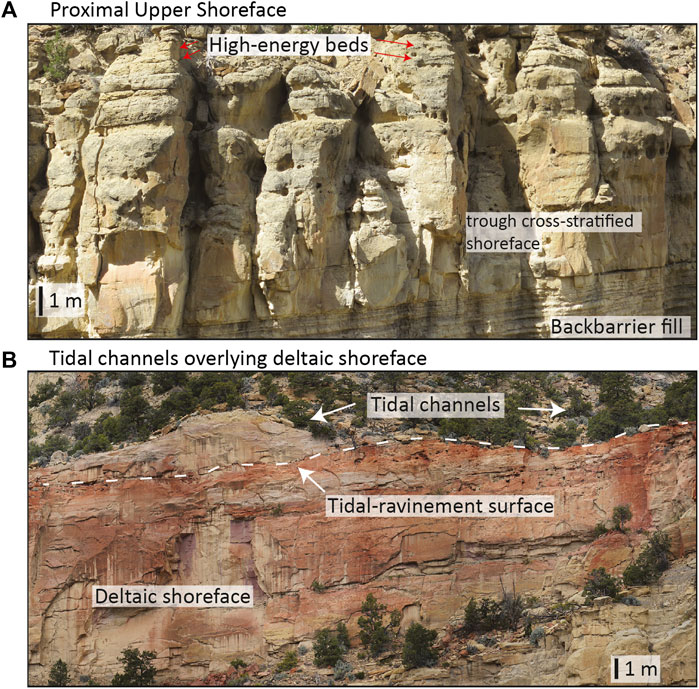
FIGURE 13. Comparison of (A) proximal upper shoreface interpreted as part of a barrier island and (B) backbarrier tidal channels truncating an upper shoreface separated by a tidal-ravinement surface showing the difference in facies and outcrop character.
By considering the barrier island system holistically, the architectural elements described and elaborated on in the previous section imply that deposition that occurred in the tidal inlet (e.g., flood- or ebb-tidal deltas or an aggrading inlet throat) becomes part of the barrier island deposit as either channel-dominated inlet fill or shoreface-dominated inlet fill. This is partly why ancient barrier island outcrops do not necessarily have an isolated, or island morphology (Figure 11).
In modern systems, the tidal inlet is the channel, or void, filled with water, separating two barrier islands or spits (Figure 1). Tidal inlets can be sediment conduits, sources, or sinks, depending on the dynamics of the individual barrier island system and the inherent tidal prisms (Oertel, 1988; Mallinson et al., 2018). Modern tidal inlets are filled laterally with littoral sands welded onto the island during down-drift migration, closing and opening as islands shift, particularly during storms (Hoyt and Henry, 1965; Susman and Heron, 1979; Hayes and FitzGerald, 2013; Seminack and Buynevich, 2013). Inlet closure is especially sensitive to changes in the back-barrier basin as reductions in water volume exchange can result in inlet aggradation, and closure. The opposite is an increase in tidal prism, causing multiple inlet openings and barrier island segmentation (Mallinson et al., 2018). Inlet filling and migration are mostly preserved as laterally building and vertically aggrading deposits, which are interpreted as shoreface-dominated inlet fill and channel-dominated inlet fill successions in the rock record. Through geologic time, these inlet infilling deposits can connect or juxtapose adjacent barrier islands (Moslow and Tye, 1985; Tye and Moslow, 1993; Seminack and Buynevich, 2013) and therefore we argue that sandstones deposited within the tidal inlet are part of the preserved barrier island complex, rather than as independent geobodies.
Sequence-Stratigraphic Position
Barrier islands are relatively thick (>5 m in the Straight Cliffs Formation) sandstone deposits within regional transgressive successions, and therefore can be identified by their sequence stratigraphic relationships. Barrier islands are associated with preserved lagoon and estuary facies, and therefore can be identified by their position within transgressive cycles, often close to the transgressive–regressive turn around. For example, the barrier island shoreface deposits of Buck Hollow are within transgressive intervals, above regressive-transgressive turn-arounds, and below major wave-ravinement surfaces and/or maximum flooding surfaces (Figure 12; Mulhern and Johnson, 2016).
By definition, modern barrier islands are separated from the shoreline by a backbarrier lagoon or estuary (Oertel, 1985). When extrapolated to the ancient, barrier islands are within “transgressive” packages, in the geologic sense, because lagoons and estuaries are inherently transgressive features (Davies, 1978; Kraft et al., 1987). Lagoons form by flooding of coastal plain or strandplain environments during relative sea-level rise (Barnes, 1980; Martin and Dominguez, 1994). As lagoons are infilled, coals and backbarrier deposits can record the regressive turn around (Sixsmith et al., 2008; Allen and Johnson, 2011). Similarly, estuaries are flooded river valleys (Dalrymple et al., 1992; Dalrymple et al., 2012). Once an estuary is a net-exporter of sediment to the coastline, it infills and records the regressive turn around, becoming a delta (Dalrymple, 2006). Combined, the preservation of lagoon and estuary deposits and bounding surfaces can be used identify barrier island successions and place them in a regional sequence stratigraphic context.
Barrier Island Motion and Preservation
Barrier island motion is highly complex in modern environments, and when extrapolated to geologic timescales, the resultant time-transgressive outcrops have highly complex and variable geometries. Current understanding of barrier island motion and preservation comes mainly from modern studies and observations, of particular importance is the distinction between barrier island motion and preservation, and how these concepts differ from modern to ancient time scales. Over short time scales (generally <10,000 years) modern barrier islands can move in all directions, prograding (Bernard et al., 1962; Oost, 1995; Oost et al., 2012) and aggrading (Simms et al., 2006) through processes similar to regular shoreface development (Clifton, 2006). Contrasting models exist for modern barrier island retrogradation: rollover and back-stepping (Figure 14; Curray, 1964). Rollover occurs via overwash and storm reworking during continuous transgression (Figure 14A), leaving behind only a transgressive lag of winnowed material (Swift, 1968; Dillon, 1970; Belknap and Kraft, 1981; Swift et al., 1991; Timmons et al., 2010). The alternative model suggests that barrier islands move through back-stepping, or in-place drowning, in which the island is rapidly drowned and later relocates in a landward position as sea-level stabilizes and equilibrium is met between sediment supply and energy regime (Figure 14B; Swift, 1975; Rampino and Sanders, 1980; Boyd and Penland, 1984; De Falco et al., 2015). A third option, discontinuous, or sporadic rollover can also occur, where smaller remnants of the barrier are left behind as partially overstepped features, with a net landward shoreline trajectory. This can be the result of punctuated rises in sea-level (Pretorius et al., 2016), or to autogenic feedback between shoreface dynamics and overwash (Ciarletta et al., 2019).
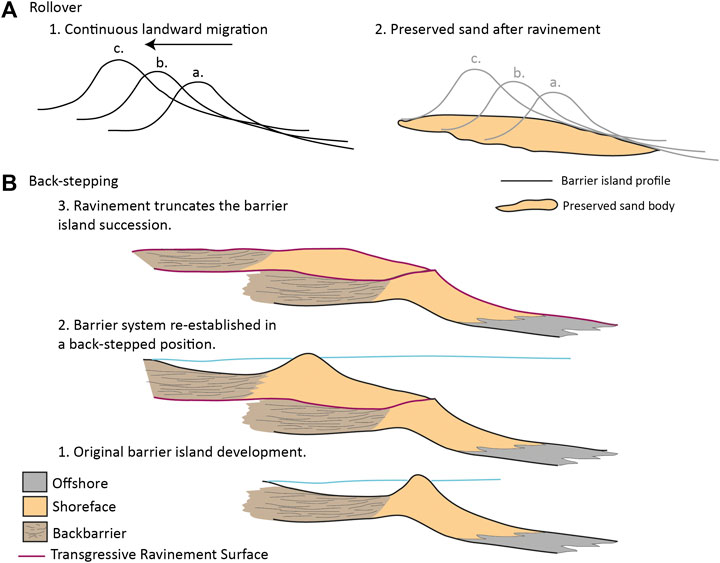
FIGURE 14. Schematic models for modern barrier island motion through rollover (A) as the barrier moves continuous landward and back-stepping (B) which shows the process of a barrier island system back-stepping landward, reestablishing, and then being truncated through ravinement. Based on the outcrop interpretations in this study we argue these modern processes merge together at geologic timescales.
Attempts have been made to extrapolate these models to explain ancient barrier island preservation (Devine, 1991), however the time duration and magnitude of motion described in an aggradation, progradation, or retrogradation used in literature to describe outcrops are orders of magnitude different from the <10,000 years modern timescales. In a sequence stratigraphic sense, barrier islands tend to develop during periods of regional relative sea-level rise, placing them within transgressive successions. Because of this tendency, the conceptual models for modern barrier island retrogradational motion (Figure 14; rollover and back-stepping) have been posed as conflicting models to explain barrier island preservation (Devine, 1991). Current research on recent barrier islands and drowned remnants thereof recognizes the interplay and validity of these processes (Mellett et al., 2012; Green et al., 2014, Green et al., 2018; Cooper et al., 2018). Building on this notion, we argue that, over long timescales (0.1–1 my or greater) and with base-level changes significantly broader than those glacio-eustatic fluctuations of the Pleistocene, the continuum between rollover and back-stepping blurs; these motions merge together and become irresolvable at longer time scales, explaining barrier island preservation and the stacking of multiple individual barrier island deposits.
Periodic geologic scale overstepping and landward reestablishment of barrier islands explains the preservation of thick, stacked, barrier islands which show local, internal progradational or aggradational facies patterns (Figure 14B; Sabins, 1963; Land, 1972; Bridges, 1976; Roehler, 1988; Roy et al., 1994; Sixsmith et al., 2008). Rollover explains the preservation of thin deposits with evidence of washover (Hobday and Orme, 1974; Hobday and Jackson, 1979; Willis and Moslow, 1994b). Yet, when considered at geologic time scales, the two models merge together because they are both dependent on the rate, duration, and magnitude of relative sea-level rise, regardless of the short-term motion of the island system. Rapid transgression limits overwash (Swift, 1968), preventing ravinement (Davis and Clifton, 1987; Pretorius et al., 2016), and ultimately leading to in-place drowning, or partial back-stepping. Consequently, when longer timescales are considered, rollover and overstepping blend together and all three modern island types (aggradational, progradational, retrogradational) can be preserved with sufficient accommodation. Barrier islands can locally move landward through rollover but are preserved through rapid transgression and overstepping. While the disconnect between relative sea-level change and barrier island motions has been recognized and modeled (Storms et al., 2002), this study is the first time they are incorporated into geologic-scale barrier island preservation models.
The preservation of prograding barrier islands through back-stepping implies that barrier island motion can be independent from the relative shoreline motion. Islands can build basinward while the shoreline steps landward, as occurs in both modern and ancient systems. For example, Galveston Island is prograding (Bernard et al., 1962; Morton, 1994) while the Gulf Coast is undergoing transgression (Milliken et al., 2008). Ancient barrier island deposits show progradation within transgressive successions, displaying Waltherian stacking patterns (Middleton, 1973), with more proximal facies (upper shoreface) over more distal facies (lower shoreface). This commonly occurs in the Straight Cliffs examples, where barrier island shoreface examples internally prograde, with shallowing upwards successions from lower to upper shoreface (Figure 7A). This progradation occurs within the regionally transgressive D and F intervals of the John Henry Member (Figure 2B; Chentnik et al., 2015; Mulhern and Johnson, 2016). This concept is not new: local progradation during regional transgression creates retrogradational stacking patterns in sequence stratigraphic models (Posamentier and Allen, 1999). However, applying this concept of disconnect between local deposition and regional stacking patterns to barrier island environments explains the preservation of thick shoreface deposits during transgression, and allows for more plausible and consistent interpretations of ancient successions to be made.
Conclusions
The barrier island deposits presented here are composed of four facies associations (backbarrier fill, lower to upper shoreface, proximal upper shoreface, and tidal channels), which stack to create three barrier island architectural elements (barrier island shorefaces, shoreface-dominated inlet fill, and channel-dominated inlet fill). Barrier island shorefaces preserve proximal lower shoreface and upper shoreface strata over backbarrier fill, recording progradational island motion. Shoreface-dominated inlet fill is composed of high-energy, proximal upper shoreface deposits, recording the lateral migration of a barrier island through time. Channel-dominated inlet fill records vertical aggradation within tidal inlets. The barrier islands described here can be recognized through three key criteria: proximity to backbarrier facies, internal facies patterns, and sequence stratigraphic position.
While these examples from the Straight Cliffs Formation may not encompass all the possible variability, excellent outcrop exposure and preservation provides a new foundation for understanding the most commonly preserved types in barrier island deposits. High accommodation and long-lived paralic environments of the Straight Cliffs Formation created optimal preservation conditions for barrier islands, allowing for facies models to be updated, developed, and described. Barrier islands in other localities may vary in thickness, lateral extent, or the way the architectural elements combine, nevertheless the internal facies and recognition criteria presented here provide a basis for distinguishing barrier islands from other depositional environments. The extension of these identification criteria need to now be tested in other settings where the underpinning variables for preservation may be different. We expect some divergence at the largest scales between other settings, but consider that the smaller scale observations here (e.g., facies associations, architecture) should hold true.
Barrier islands are time-contemporaneous to and associated with backbarriers (lagoons or wave-dominated estuaries) and therefore are inherently preserved within transgressive successions, distinguishing them from regressive strandplain or deltaic shorefaces. The successions documented here depart from existing models by incorporating multidirectional island motion, ravinement, erosion, and reworking and by incorporating deposition within the tidal inlet as part of the barrier island itself, resulting in deposits with variable, non-island like morphologies. The outcrops described here also imply that barrier island motion can be independent of the regional shoreline motion. Pervasive reworking makes outcrop geometries complex and barrier island deposits can record local progradation within transgressive intervals. This study shows that barrier island preservation time-averages the three types of barrier island motion described in the modern (back-stepping, roll over, and sporadic rollover). Barrier island preservation, stacking, and outcrop geometry are dependent on accommodation, sediment supply and geologic time-scale changes in relative sea-level.
Data Availability Statement
The original contributions presented in the study are included in the article/Supplementary Material, further inquiries can be directed to the corresponding author.
Ethics Statement
Written informed consent was obtained from the individual(s) for the publication of any potentially identifiable images or data included in this article.
Author Contributions
JM was the primary author of this paper as it was original research conducted as part of her PhD, advised by CJ. AG contributed detailed understanding of modern barrier island dynamics.
Funding
A variety of sources funded this original research by JM. Her work was supported by the Rocks2Models research consortium with funding from Chevron, ConocoPhillips, Hess ConocoPhillips, Shell, and Statoil. Support was also received from University of Utah Graduate Research Fellowship, ConocoPhillips Graduate Research Fellowship, Rocky Mountain Association of Geologists Foundation Babock Scholarship and SEPM Rocky Mountain Section Donald Smith Research Grant.
Conflict of Interest
This research was completed while JM was at the University of Utah. After completing this work JM became, and is currently, employed by Shell Exploration and Production Company (United States).
The remaining authors declare that the research was conducted in the absence of any commercial or financial relationships that could be construed as a potential conflict of interest.
References
Allen, J. L., and Johnson, C. L. (2011). Architecture and formation of transgressive-regressive cycles in marginal marine strata of the John Henry Member, Straight Cliffs Formation, Upper Cretaceous of southern Utah, USA. Sedimentology 58, 1486–1513. doi:10.1111/j.1365-3091.2010.01223.x
Allen, J. R. L. (1982). Simple models for the shape and symmetry of tidal sand waves: (1) statically stable equilibrium forms. Mar. Geol. 48, 31–49. doi:10.1016/0025-3227(82)90128-1
Anthony, E. J. (2009). Shore Processes and Their Palaeoenvironmental Applications. 1st Edn. Amsterdam, Netherlands: Elsevier.
Anthony, E. J., Lang, J., and Oyede, L. M. (1996). Sedimentation in a tropical, microtidal, wave-dominated coastal-plain estuary. Sedimentology 43, 665–675. doi:10.1111/j.1365-3091.1996.tb02019.x
Antia, J., Fielding, C. R., and Joeckel, R. M. (2011). Multiple cycles of wave-dominated estuarine deposits in low-accommodation settings, Cretaceous J Sandstone, northwestern Nebraska. Am. Assoc. Petrol. Geol. Bull. 95, 1227–1256. doi:10.1306/11051009105
Bann, K. L., and Fielding, C. R. (2004). “An integrated ichnological and sedimentological comparison of non-deltaic shoreface and subaqueous delta deposits in Permian reservoir units of Australia,” in The Application of Ichnology to Palaeoenvironmental and Stratigraphic Analysis. Editor D. M. McIlroy (London, UK: Geological Society, London, Special Publication), 228, 273–310. doi:10.1144/GSL.SP.2004.228.01.13
Barnes, R. S. K. (1980). Coastal Lagoons: The Natural History of a Neglected Habitat. Cambridge, UK: Cambridge University Press.
Barwis, J. H., and Hayes, M. O. (1979). “Regional patterns of modern barrier island and tidal inlet deposits as applied to paleoenvironmental studies,” in Carboniferous Depositional Environments in the Appalachian Region. Editors J. C. Ferm, and J. C. Horne (Columbia, SC: University of South Carolina Geology Department), 472–498.
Belknap, D. F., and Kraft, J. C. (1981). Preservation potential of transgressive coastal lithosomes on the U.S. Atlantic Shelf. Mar. Geol. 42, 429–442. doi:10.1016/0025-3227(81)90173-0
Benallack, K., Green, A. N., Humphries, M. S., Cooper, J. A. G., Dladla, N. N., and Finch, J. M. (2016). The stratigraphic evolution of a large back-barrier lagoon system with a non-migrating barrier. Marine Geology, 379, 64–77.
Berg, R. R. (1970). Identification of sedimentary environments in reservoir sandstones. Trans. Gulf Coast Assoc. Geol. Soc. 20, 137–143.
Berg, R. R., and Davies, D. (1968). Origin of Lower Cretaceous Muddy Sandstone at Bell Creek Field, Montana. Am. Assoc. Petrol. Geol. Bull. 52, 1888–1898. doi:10.1306/5D25C53B-16C1-11D7-8645000102C1865D
Bernard, H. A., LeBlanc, R. J., and Major, C. F. (1962). “Recent and Pleistocene geology of southeast Texas, field excursion no. 3,” in Geology of the Gulf Coast and Central Texas and Guidebook of Excursions. Editors E. H. Rainwater, and R. P. Zingula (Houston, TX: Houston Geological Society), 175–224.
Bhattacharya, J. P. (2010). “Deltas,” in Facies Models 4. Editors N. P. James, and R. W. Dalrymple (St. John’s, Canada: Geolgical Society of Canada), 233–264. doi:10.2110/pec.06.84.0237
Bhattacharya, J. P., and Giosan, L. (2003). Wave-influenced deltas: geomorphological implications for facies reconstruction. Sedimentology 50, 187–210. doi:10.1046/j.1365-3091.2003.00545.x
Bibler, C. J., and Schmitt, J. G. (1986). Barrier-island coastline deposition and paleogeographic implications of the Upper Cretaceous Horsethief Formation, Northern Disturbed Belt, Montana. Mt. Geol. 23, 113–127.
Boothroyd, J. C. (1985). “Tidal inlets and tidal deltas,” in Coastal Sedimentary Environments. Editor R. A. Davis (New York: Springer-Verlag), 445–532.
Boyd, D. R., and Dyer, B. F. (1964). Frio barrier bar system of south Texas. Trans. Gulf Coast Assoc. Geol. Soc. 14, 309–322.
Boyd, R. (2010). “Transgressive wave-dominated coasts,” in Facies Models 4. Editors N. P. James, and R. W. Dalrymple, (St. John’s, Canada: Geological Association of Canada), 265–294.
Boyd, R., and Penland, S. (1984). Shoreface translation and the Holocene stratigraphic record: examples from Nova Scotia, the Mississippi Delta and eastern Australia. Mar. Geol. 60, 391–412. doi:10.1016/0025-3227(84)90159-2
Bridges, P. H. (1976). Lower silurian transgressive barrier islands, southwest wales. Sedimentology 23, 347. doi:10.1111/j.1365-3091.1976.tb00054.x
Buynevich, I. V. (2019). Distribution and morphometry of buried inlet channels along a microtidal paraglacial coast: indispensable role of GPR. J. Appl. Geophys. 162, 58–63. doi:10.1016/j.jappgeo.2019.01.001
Carvalho, C. N. de., Viegas, P. A., and Cachao, M. (2007). Thalassinoides and its producer: populations of Mecochirus buried within their burrow systems, Boca de Chapim Formation (Lower Cretaceous), Portugal. Palaios 22, 104–109. doi:10.2110/palo.2006.p06-011r
Cheel, R. J., and Leckie, D. A. (1990). A tidal-inlet complex in the Cretaceous Epeiric Sea of North America: Virgelle Member, Milk River Formation, southern Alberta, Canada. Sedimentology 37, 67–81. doi:10.1111/j.1365-3091.1990.tb01983.x
Chentnik, B. M., Johnson, C. L., Mulhern, J. S., and Stright, L. E. (2015). Valleys, estuaries, and lagoons: paleoenvironments and regressive-transgressive architecture of the Upper Cretaceous Straight Cliffs Formation. J. Sediment. Res. 85, 1166–1196. doi:10.2110/jsr.2015.70
Ciarletta, D. J., Lorenzo-Trueba, J., and Ashton, A. D. (2019). Mechanism for retreating barriers to autogenically form periodic deposits on continental shelves. Geology 47, 239–242. doi:10.1130/G45519.1
Clifton, H. E. (2006). “A reexamination of facies models for clastic shorelines,” in Facies Models Revisited. Editors H. G. Posamentier, and R. G. Walker (Tulsa, OK: SEPM Special Publication), Vol. 84, 293–337.
Cooper, J. A. G., Green, A. N., and Loureiro, C. (2018). Geological constraints on mesoscale coastal barrier behaviour. Global Planet. Change 168, 15–34. doi:10.1016/J.GLOPLACHA.2018.06.006
Cotter, E. (1975). “Deltaic deposits in the Upper Cretaceous Ferron Sandstone, Utah,” in Deltas: Models for Exploration. Editor M. Lou Broussard (Houston, TX: Houston Geological Society), 471–484.
Curray, J. R. (1964). “Transgressions and regressions,” in Papers in Marine Geology, Shepard Commemorative Volume. Editor R. L. Miller (New York, NY: Macmillan Company), 175–203.
Dalrymple, R. W. (2006). “Incised valleys in time and space: an introduction to the volume and an examination of the controls on valley formation and filling,” in Incised Valleys in Time and Space. Editors R. W. Dalrymple, D. A. Leckie, and R. W. Tillman (Broken Arrow, OK: SEPM Special Publication), Vol. 85, 5–12.
Dalrymple, R. W. (2010). “Tidal depositional systems,” In Facies Models 4. Editors N. P. James, and R. W. Dalrymple, (S. John’s, Canada: Geological Association of Canada), 201–232.
Dalrymple, R. W., and Choi, K. (2007). Morphologic and facies trends through the fluvial–marine transition in tide-dominated depositional systems: a schematic framework for environmental and sequence-stratigraphic interpretation. Earth Sci. Rev. 81, 135–174. doi:10.1016/j.earscirev.2006.10.002
Dalrymple, R. W., Mackay, D. A., Ichaso, A. A., and Choi, K. S. (2012). “Processes, morphodynamics, and facies of tide-dominated estuaries,” in Principles of Tidal Sedimentology. Editors R. A. Davis, and R. W. Dalrymple (Dordrecht, Netherlands: Springer), 79–107.
Dalrymple, R. W., Zaitlin, B. A., and Boyd, R. R. (1992). Estuarine facies models: conceptual basis and stratigraphic implications. J. Sediment. Petrol. 62, 1130–1146. doi:10.1306/D4267A69-2B26-11D7-8648000102C1865D
Dashtgard, S. E., Gingras, M. K., and MacEachern, J. A. (2009). Tidally modulated shorefaces. J. Sediment. Res. 79, 793–807. doi:10.2110/jsr.2009.084
Dashtgard, S. E., MacEachern, J. A., Frey, S. E., and Gingras, M. K. (2010). Tidal effects on the shoreface: towards a conceptual framework. Sediment. Geol. 279, 42–61. doi:10.1016/j.sedgeo.2010.09.006
Davies, D. K. (1978). “Models and concepts for exploration in barrier islands,” in Sedimentary Environments and Hydrocarbons. Editor R. S. Saxena (New Orleans, LA: Gulf Coast Association of Geological Societies), 79–115.
Davies, D. K., and Ethridge, F. G. (1971). The Claiborne Group of central Texas. Trans. Gulf Coast Assoc. Geol. Soc. 21, 115–124.
Davies, R., Howell, J., Boyd, R., Flint, S., and Diessel, C. (2006). High-resolution sequence-stratigraphic correlation between shallow-marine and terrestrial strata: examples from the Sunnyside Member of the Cretaceous Blackhawk Formation, Book Cliffs, eastern Utah, eastern Utah. Am. Assoc. Petrol. Geol. Bull. 90, 1121–1140. doi:10.1306/02210604077
Davis, R. A. (1994). “Barrier island systems- a geologic overview,” in Geology of Holocene Barrier Island Systems. Editor R. A. Davis (Berlin, Germany: Springer-Verlag), 1–46.
Davis, R. A. (2013). A new look at barrier-inlet morphodynamics. J. Coast. Res. Spec. Issue 69, 1–12. doi:10.2112/SI_69_2
Davis, R. A., and Clifton, H. E. (1987). “Sea-level change and the preservation potential of wave-dominated and tide-dominated coastal sequences,” in Sea-Level Fluctuation and Coastal Evolution. Editors D. Nummedal, O. H. Pilkey, and J. D. Howard (Broken Arrow, OK: SEPM Special Publication), 41, 167–178.
De Falco, G., Antonioli, F., Fontolan, G., Lo Presti, V., Simeone, S., Tonielli, R., et al. (2015). Early cementation and accommodation space dictate the evolution of an overstepping barrier system during the Holocene. Mar. Geol. 369, 52–66. doi:10.1016/j.margeo.2015.08.002
Devine, P. E. (1991). Transgressive origin of channeled estuarine deposits in the point lookout sandstone, northwestern New Mexico: a model for Upper Cretaceous, cyclic regressive parasequences of the U.S. Western Interior. Am. Assoc. Petrol. Geol. Bull. 75, 1039–1063. doi:10.1306/0C9B28C1-1710-11D7-8645000102C1865D
Dickinson, K. A., Berryhill, H. L., and Holmes, C. W. (1972). “Criteria for recognizing ancient barrier coastlines,”in Recognition of Ancient Sedimentary Environments. Editors J. K. Rigby, and W. K. Hamblin (Tulsa, OK: SEPM, Special Publication), Vol. 16, 108–145. doi:10.2110/pec.72.02
Dillon, W. P. (1970). Submergence effects on a Rhode Island barrier and lagoon and inferences on migration of barriers. J. Geol. 78, 94–106. doi:10.1086/627490
Dladla, N. N., Green, A. N., Cooper, J. A. G., and Humphries, M. S. (2019). Geological inheritance and its role in the geomorphological and sedimentological evolution of bedrock-hosted incised valleys, lake St Lucia, South Africa. Estuarine, Coastal and Shelf Science, 222, 154–167.
Donselaar, M. E., and Nio, S. (1982). An Eocene tidal inlet/washover type barrier island complex in the South Pyrenean Marginal Basin, Spain. Geol. Mijnbouw 61, 343–353.
Dooling, P. R. (2013). Tidal facies, stratigraphic architecture, and along-strike variability of a high energy, transgressive shoreline, Late Cretaceous, Kaiparowits Plateau, southern Utah. Geol. Geophyics M.S.
Droser, M. L., and Bottjer, D. J. (1989). Ichnofabric of sandstones deposited in high-energy nearshore enviroments measurements and utilization. Palaios 4, 598–604.
Dumas, S. (2005). Experiments on oscillatory-flow and combined-flow bed forms: implications for interpreting parts of the shallow-marine sedimentary record. J. Sediment. Res. 75, 501–513. doi:10.2110/jsr.2005.039
Dumas, S., and Arnott, R. W. C. (2006). Origin of hummocky and swaley cross-stratification - the controlling influence of unidirectional current strength and aggradation rate. Geology 34, 1073–1076. doi:10.1130/G22930A.1
Dzuynski, S., and Smith, A. J. (1963). Convolute lamination, its origin, preservation, and directional significance. J. Sediment. Res. 33, 616–627. doi:10.1306/74D70ED4-2B21-11D7-8648000102C1865D
Eaton, J. G. (1991). “Biostratigraphic framework for the Upper Cretaceous rocks of the Kaiparowits Plateau, southern Utah,” in Stratigraphy, Depositional Environments, and Sedimentary Tectonics of the Western Margin, Cretaceous Western Interior Seaway. Editors J. D. Nations, and J. G. Eaton (Boulder, CO: Geological Society of America), 47–63. doi:10.1130/SPE260-p47
Feldman, H. R., Fabijanic, J. M., Faulkner, B. L., and Rudolph, K. W. (2014). Lithofacies, parasequence stacking, and depositional architecture of wave- to tide-dominated shorelines in the Frontier Formation, Western Wyoming. U.S.A. J. Sediment. Res. 84, 694–717. doi:10.2110/jsr.2014.53
Fisher, J. S., and Dolan, R. (Editors) (1977). in Beach Processes and Coastal Hydrodynamics (Stroudsburg, PA: Dowden, Hutchinson & Ross).
FitzGerald, D. M., Buynevich, I., and Hein, C. (2012). “Morphodynamics and facies architecture of tidal inlets and tidal deltas,” in Principles of Tidal Sedimentology. Editors R. A. Davis, and R. W. Dalrymple (Dordrecht, Netherlands: Springer), 301–333. doi:10.1007/978-94-007-0123-6
Flores, R. M. (1978). Barrier and back-barrier environments of deposition of the Upper Cretaceous Almond Formation, Rock Springs Uplift, Wyoming. Mt. Geol. 15, 57–65.
Frey, R. W., and Howard, J. D. (1985). Trace fossils from the Panther Member, Star Point Formation (Upper Cretaceous), Coal Creek Canyon, Utah. J. Paleontol. 59, 370–404. doi:10.1007/BF02987810
Galloway, W. E. (1986). Reservoir facies architecture of microtidal barrier systems. Am. Assoc. Petrol. Geol. Bull. 70, 787–808. doi:10.1306/9488634E-1704-11D7-8645000102C1865D
Green, A. N., Cooper, J. A. G., and Salzmann, L. (2014). Geomorphic and stratigraphic signals of postglacial meltwater pulses on continental shelves. Geology 42, 151–154. doi:10.1130/G35052.1
Green, A. N., Cooper, J. A. G., and Salzmann, L. (2018). The role of shelf morphology and antecedent setting in the preservation of palaeo-shoreline (beachrock and aeolianite) sequences: the SE African shelf. Geo Mar. Lett. 38, 5–18. doi:10.1007/s00367-017-0512-8
Green, A. N., Pillay, T., Cooper, J. A. G., and Guisado-Pintado, E. (2019). Overwash-dominated stratigraphy of barriers with intermittent inlets. Earth Surf. Process. Landforms 44, 2097–2111. doi:10.1002/esp.4631
Hampson, G. J., and Howell, J. A. (2005). “Sedimentologic and geomorphic characterization of ancient wave-dominated deltaic shorelines: Upper Cretaceous Blackhawk Formation, Book Cliffs, Utah, U.S.A.” in River Deltas; Concepts, Models, and Examples. Editors L. Giosan, and J. P. Bhattacharya (Broken Arrow, OK: SEPM Special Publication), 83, 133–154.
Hapke, C. J., Lentz, E. E., Gayes, P. T., McCoy, C. A., Hehre, R., Schwab, W. C., et al. (2010). A review of sediment budget imbalances along Fire Island, New York: can nearshore geologic framework and patterns of shoreline change explain the deficit? J. Coast Res. 263, 510–522. doi:10.2112/08-1140.1
Hayes, M. O. (1980). General morphology and sediment patterns in tidal inlets. Sediment. Geol. 26, 139–156. doi:10.1016/0037-0738(80)90009-3
Hayes, M. O., and FitzGerald, D. M. (2013). Origin, evolution, and classification of tidal inlets. J. Coast Res. 69, 14–33. doi:10.2112/SI_69_3
Hein, C. J., Fallon, A. R., Rosen, P., Hoagland, P., Georgiou, I. Y., FitzGerald, D. M., et al. (2019). Shoreline dynamics along a developed river mouth barrier island: multi-decadal cycles of erosion and event-driven mitigation. Front. Earth Sci. 7, 103. doi:10.3389/feart.2019.00103
Heron, S. D., Moslow, T. F., Berelson, W. M., Herbert, J. R., Steele, G. A., and Susman, K. R. (1984). Holocene sedimentation of a wave-dominated barrier island shoreline: Cape Lookout, North Carolina. Mar. Geol. 60, 413–434. doi:10.1016/0025-3227(84)90160-9
Hettinger, R. D., McCabe, P. J., and Shanley, K. W. (1993). “Detailed facies anatomy of aransgressive and highstand systems sracts from the Upper Cretaceous of Southern Utah, U.S.A.,” in Siliciclastic Sequence Stratigraphy: Recent Developments and Applications. Editors R. J. Weimer, and H. W. Posamentier (Tulsa, OK: American Association of Petroleum Geologists Memior), Vol. 58, 235–257.
Heward, A. P. (1981). A review of wave-dominated clastic shoreline deposits. Earth Sci. Rev. 17, 223–276. doi:10.1016/0012-8252(81)90022-2
Hobday, D. K., and Jackson, M. P. A. (1979). Transgressive shore zone sedimentation and syndepositional deformation in the Pleistocene of Zululand, South Africa. J. Sediment. Res. 49, 145–158. doi:10.1306/212F76DA-2B24-11D7-8648000102C1865D
Hobday, D. K., and Orme, A. R. (1974). The Port Durnford Formation. Verh. Geol. Ver. Suid Afrika 77, 141–149.
Hoyt, J., and Henry, V. J. (1965). “Significance of inlet sedimentation in the recognition of ancient barrier islands,” in Sedimentation of Late Cretaceous and Tertiary Outcrops, Rock Springs Uplift. Ninteenth Annual Field Conference Guidebook. Editors R. H. DeVoto, and R. K. Bitter (Casper, WY: Wyoming Geological Association), 190–194.
Hubbard, S. M., Gingras, M. K., Pemberton, S. G., and Thomas, M. B. (2002). Variability in wave-dominated estuary sandstones: implications on subsurface reservoir development. Bull. Can. Petrol. Geol. 50, 118–137. doi:10.2113/50.1.118
Hughes, Z. J. (2012). “Tidal channels on tidal flats and marshes,” in Principles of Tidal Sedimentology. Editors R. A. Davis, and R. W. Dalrymple (Dordrecht, Netherlands: Springer), 269–300. doi:10.1007/978-94-007-0123-6_11
Isla, M. F., Schwarz, E., and Veiga, G. D. (2020). Record of a nonbarred clastic shoreline. Geology 48, 338–342. doi:10.1130/G46800.1
Israel, A. M., Ethridge, F. G., and Estes, E. L. (1987). A sedimentologic description of a microtidal, flood-tidal delta, San Luis Pass, Texas. J. Sediment. Res. 57, 288–300. doi:10.1306/212f8b07-2b24-11d7-8648000102c1865d
Johannessen, P. N., and Nielsen, L. H. (2010). Architecture of an Upper Jurassic barrier island sandstone reservoir, Danish Central Graben: implications of a Holocene-Recent analogue from the Wadden Sea. Pet. Geol. Conf. Ser. 7, 145–155. doi:10.1144/0070145
Kana, T. W., Rosati, J. D., and Traynum, S. B. (2011). Lack of evidence for onshore sediment transport from deep water at decadal time scales: Fire Island, New York. J. Coast Res. 59, 61–75. doi:10.2112/SI59-007.1
Kieft, R. L., Hampson, G. J., Jackson, C. A. L., and Larsen, E. (2011). Stratigraphic architecture of a net-transgressive marginal- to shallow-marine succession: Upper Almond Formation, Rock Springs Uplift, Wyoming. U.S.A. J. Sediment. Res. 81, 513–533. doi:10.2110/jsr.2011.44
Kraft, J. C., Chrzastowski, M. J., Belknap, D. F., Toscano, M. A., and Fletcher, C. H. (1987). “The transgressive barrier-lagoon coast of Delaware: morphostratigraphy, sedimentary sequences and responses to relative rise in sea level,” in Sea-Level Fluctuation and Coastal Evolution. Editors D. Nummedal, O. H. Pilkey, and J. D. Howard (Broken Arrow, OK: SEPM Special Publication), 41, 129–143.
Kumar, N., and Sanders, J. E. (1974). Inlet sequence: a vertical succession of sedimentary structures and textures created by the lateral migration of tidal inlets. Sedimentology 21, 491–532. doi:10.1111/j.1365-3091.1974.tb01788.x
Land, C. B. (1972). “Stratigraphy of Fox Hills Sandstone and associated formations, Rock Springs Uplift and Wamsutter Arch Area, Sweetwater County, Wyoming: a shoreline-estuary sandstone model for the Late Cretaceous, in Quarterly of the Colorado School of Mines. (Golden, CO: Colorado School of Mines), Vol. 67, 1–69.
Leatherman, S. P. (1983). Barrier island evolution in response to sea level rise: a discussion. J. Sediment. Petrol. 53, 1026–1033. doi:10.1306/2F212F8314-2B24-11D7-8648000102C1865D
Leckie, R. M. (1987). Paleoecology of Mid-Cretaceous planktonic foraminifera: a comparison of open ocean and epicontinental sea assemblages. Micropaleontology 33, 164–176. doi:10.2307/1485491
Lentz, E. E., and Hapke, C. J. (2011). Geologic framework influences on the geomorphology of an anthropogenically modified barrier island: assessment of dune/beach changes at Fire Island, New York. Geomorphology 126, 82–96. doi:10.1016/j.geomorph.2010.10.032
Longhitano, S. G., Mellere, D., Steel, R. J., and Ainsworth, R. B. (2012). Tidal depositional systems in the rock record: a review and new insights. Sediment. Geol. 279, 2–22. doi:10.1016/j.sedgeo.2012.03.024
MacEachern, J. A., and Pemberton, S. G. (1992). “Ichnological aspects of Cretaceous shoreface successions and shoreface variability in the Western Interior Seaway of North America,” in Applications of Ichnology to Petroleum Exploration. Society of Sedimentary Geology Core Workshop. Editor S. G. Pemberton (Tulsa, OK: SEPM (Society for Sedimentary Geology), Vol. 17, 57–84.
Macquaker, J. H. S., Taylor, K. G., and Gawthorpe, R. L. (2007). High-resolution facies analyses of mudstones: implications for paleoenvironmental and sequence stratigraphic interpretations of offshore ancient mud-dominated successions. J. Sediment. Res. 77, 324–339. doi:10.2110/jsr.2007.029
Mallinson, D., Culver, S., Leorri, E., Mitra, S., Mulligan, R., and Riggs, S. (2018). “Barrier island and estuary co-evolution in response to Holocene Climate and sea-level change: Pamlico Sound and the Outer Banks barrier islands, North Carolina, USA,” in Barrier Dynamics and Response to Changing Climate. Editors L. J. Moore, and A. B. Murray (Cham, Switzerland: Springer International Publishing), 91–120. doi:10.1007/978-3-319-68086-6_3
Martin, L., and Dominguez, J. M. L. (1994). “Chapter 3 geological history of coastal lagoons,” in Coastal Lagoon Processes. Editor B. Kjerfve (Amsterdam, Netherlands: Elsevier), 41–68. doi:10.1016/S0422-9894(08)70008-4
Masselink, G., and van Heteren, S. (2014). Response of wave-dominated and mixed-energy barriers to storms. Mar. Geol. 352, 321–347. doi:10.1016/j.margeo.2013.11.004
McBride, R. A., Anderson, J. B., Buynevich, I. V., Cleary, W., Fenster, M. S., FitzGerald, D. M., et al. (2013). “Morphodynamics of barrier systems: a synthesis,” in Treatise on Geomorphology: Volume 10 Coastal Geomorphology. Editor D. J. Sherman (San Diego, CA: Academic Press), 166–244. doi:10.1016/B978-0-12-374739-6.00279-7
McCabe, P. J. (1987). “Facies studies of coal and coal-bearing strata,” in Coal and Coal-Bearing Strata: Recent Advances. Editor A. C. Scott (London, UK: Geological Society, London, Special Publications), Vol. 32, 51–66. doi:10.1144/GSL.SP.1987.032.01.05
McCubbin, D. G. (1982). “Barrier-island and strand plain facies,” in Sandstone Depositional Environments. Editors P. A. Scholle, and D. Spearing (Tulsa, OK: American Association of Petroleum Geologists Memior), Vol. 31, 247–279.
Mellere, D., Zecchin, M., and Perale, C. (2005). Stratigraphy and sedimentology of fault-controlled backstepping shorefaces, middle Pliocene of Crotone Basin. Southern Italy. Sediment. Geol. 176, 281–303. doi:10.1016/j.sedgeo.2005.01.010
Mellett, C. L., Hodgson, D. M., Lang, A., Mauz, B., Selby, I., and Plater, A. J. (2012). Preservation of a drowned gravel barrier complex: a landscape evolution study from the north-eastern English Channel. Mar. Geol. 315–318, 115–131. doi:10.1016/j.margeo.2012.04.008
Middleton, G. V. (1973). Johannes Walther’s Law of the correlation of facies. Geol. Soc. Am. Bull. 84, 979. doi:10.1130/0016-7606
Milliken, K. T., Anderson, J. B., and Rodriguez, A. B. (2008). “A new composite Holocene sea-level curve for the northern Gulf of Mexico,” in Response of Upper Gulf Coast Estuaries to Holocene Climate Change and Sea-Level Rise. Editors J. B. Anderson, and A. B. Rodriguez (Boulder, CO: Geological Society of America), 1–11. doi:10.1130/2008.2443(01
Moore, L. J., and Murray, A. B. (2018). Barrier dynamics and response to changing climate (Cham, Switzerland: Springer). doi:10.1007/978-3-319-68086-6
Moore, L. J., List, J. H., Williams, S. J., and Stolper, D. (2010). Complexities in barrier island response to sea level rise: Insights from numerical model experiments, North Carolina Outer Banks. J. Geophys. Res. Earth Surf. 115, 1–27. doi:10.1029/2009JF001299
Morton, R. A. (1994). “Texas barriers,” in Geology of Holocene Barrier Island Systems. Editor R. A. Davis (Berlin, Germany: Springer-Verlag), 75–114.
Moslow, T. F., and Tye, R. S. (1985). Recognition and characterization of Holocene tidal inlet sequences. Mar. Geol. 63, 129–151. doi:10.1016/0025-3227(85)90081-7
Mulhern, J. S., Johnson, C. L., and Martin, J. M. (2019). Modern to ancient barrier island dimensional comparisons: implications for analog selection and paleomorphodynamics. Front. Earth Sci. 7, 109. doi:10.3389/feart.2019.00109
Mulhern, J. S., and Johnson, C. L. (2016). “Time–space variability of paralic strata deposited in a high accommodation, high sediment supply setting: example from the Cretaceous of Utah,” in Sedimentology of Paralic Reservoirs. Editors G. J. Hampson, A. D. Reynolds, B. Kostic, and M. R. Wells (London, UK: Geological Society, London, Special Publication), Vol. 444. doi:10.1144/SP444.7
Niedoroda, A. W., Swift, D. J. P., and Hopkins, T. S. (1985). “The shoreface,” in Coastal Sedimentary Environments. Editor R. A. Davis (New York, NY: Springer-Verlag), 533–624.
Nio, S.-D., and Yang, C.-S. (1991). “Diagnostic attributes of clastic tidal deposits,” in Clastic Tidal Sedimentology. Editors D. G. Smith, G. E. Reinson, B. A. Zaitlin, and R. A. Rahmani (Calgary, Canada: Canadian Society of Petroleum Geologists, Memior), 16, 3–28.
Oertel, G. F. (1985). The barrier island system. Mar. Geol. 63, 1–18. doi:10.1016/0025-3227(85)90077-5
Oertel, G. F. (1988). “Processes of sediment exchange between tidal inlets, ebb deltas and barrier islands,” in Hydrodynamics and Sediment Dynamics of Tidal Inlets. Editors D. G. Aubrey, and L. Weishar (New York, NY: Springer-Verlag), 297–318. doi:10.1029/LN029p0297
Olariu, C., Steel, R. J., Dalrymple, R. W., and Gingras, M. K. (2012). Tidal dunes versus tidal bars: the sedimentological and architectural characteristics of compound dunes in a tidal seaway, the lower Baronia Sandstone (lower Eocene), Ager Basin, Spain. Sediment. Geol. 279, 134–155. doi:10.1016/j.sedgeo.2012.07.018
Olariu, C., Steel, R. J., and Petter, A. L. (2010). Delta-front hyperpycnal bed geometry and implications for reservoir modeling: Creatceous Panther Tongue Delta, Book Cliffs, Utah. Am. Assoc. Petrol. Geol. Bull. 94, 819–845. doi:10.1306/11020909072
Olsen, T. R., Mellere, D., and Olsen, T. (1999). Facies architecture and geometry of landward-stepping shoreface tongues: the Upper Cretaceous Cliff House Sandstone (Mancos Canyon, south-west Colorado). Sedimentology 46, 603–625. doi:10.1046/j.1365-3091.1999.00234.x
Oost, A. P. (1995). Geologica Ultraiectina, 126, pp. 1–455. ISSN: 0072-1026 ISBN: 90‐71577‐80‐5 Utrecht University Roehler, 1398, 1–65.
Oost, A. P., Hoekstra, P., Wiersma, A., Flemming, B., Lammerts, E. J., Pejrup, M., et al. (2012). Barrier island management: lessons from the past and directions for the future. Ocean Coast Manag. 68, 18–38. doi:10.1016/j.ocecoaman.2012.07.010
Otvos, E. G. (2012). Coastal barriers—nomenclature, processes, and classification issues. Geomorphology 139–140, 39–52. doi:10.1016/j.geomorph.2011.10.037
Painter, C. S., York-Sowecke, C. C., and Carrapa, B. (2013). Sequence stratigraphy of the Upper Cretaceous Sego Sandstone Member reveals spatio-temporal changes in depositional processes, northwest Colorado. U.S.A. J. Sediment. Res. 83, 323–338. doi:10.2110/jsr.2013.21
Pattison, S. A. J. (1995). Sequence stratigraphic significance of sharp-based lowstand shoreface deposits, Kenilworth Member, Book Cliffs, Utah. Am. Assoc. Petrol. Geol. Bull. 79, 444–462. doi:10.1306/8D2B155C-171E-11D7-8645000102C1865D
Pemberton, S. G., MacEachern, J. A., Dashtgard, S. E., Bann, K. L., Gingras, M. K., and Zonneveld, J.-P. (2012). “19. Shorefaces,” in Trace Fossils as Indicators of Sedimentary Environments. Editors D. Knaust, and R. G. Bromley (Amsterdam, Netherlands: Elsevier), 563–606.
Peterson, F. (1969). Four new members of the Upper Cretaceous Straight Cliffs Formation in the southeastern Kaiparowits Region, Kane County, Utah. Geol. Surv. Bull. 1274‐J, J1–J28. doi:10.3133/b1274J
Plint, A. G. (2010). “Wave-and storm-dominated shoreline and shallow-marine systems,” in Facies Models 4. Editors N. P. James, and R. W. Dalrymple (St. John’s, New Foundland, Canada: Geolgical Society of Canada), 167–199.
Pocknall, D., Johnson, C. L., Mulhern, J. S., and Johnson, C. (2016). “Palynology of non-marine and marine strata of the Straight Cliffs Formation (Coniacian-Campanian), Kaiparowits Plateau, Utah,” in Program and abstracts Joint meeting of the Society of Organic Petrology, International Committee for Coal and Organix Petrology, and AASP—the Palynological Society. Houston, TX, September 2016, 95.
Posamentier, H. W., and Allen, G. P. (1999). Siliciclastic Sequence Stratigraphy: Concepts and Applications. Editor G. P. Allen (Tulsa, OK: SEPM Society for Sedimentary Geology).
Posamentier, H. W., Allen, G. P., James, D. P., and Tesson, M. (1992). Forced regressions in a sequence stratigraphic framework: concepts, examples, and exploration significance. Am. Assoc. Petrol. Geol. Bull. 76, 1687–1709.
Pretorius, L., Green, A., and Cooper, A. (2016). Submerged shoreline preservation and ravinement during rapid postglacial sea-level rise and subsequent “slowstand”. Geol. Soc. Am. Bull. 128, 1059–1069. doi:10.1130/B31381.1
Raff, J. L., Shawler, J. L., Ciarletta, D. J., Hein, E. A., Lorenzo-Trueba, J., and Hein, C. J. (2018). Insights into barrier-island stability derived from transgressive/regressive state changes of Parramore Island, Virginia. Mar. Geol. 403, 1–19. doi:10.1016/j.margeo.2018.04.007
Rahmani, R. A. (1988). “Estuarine tidal channel and nearshore sedimentation of a Late Cretaceous epicontinental sea, Drumheller, Alberta, Canada,” in Tide-Influenced Sedimentary Environments and Facies. Editors P. L. de Boer, A. van Gelder, and S. D. Nio (Dordrecht, Netherlands: Reidel Publishing), 433–474.
Rampino, M. R., and Sanders, J. E. (1980). Evolution of the barrier: islands of southern Long Island, New York. Sedimentology 28, 37–47. doi:10.1111/j.1365-3091.1981.tb01661.x
Reading, H. G., and Collinson, J. D. (1996). “Clastic coasts,” in Sedimentary Environments: Processes, Facies, and Stratigraphy. Editor H. G. Reading (Oxford, UK: Blackwell Science), 154–231.
Reddering, J. S. V. (1983). An inlet sequence produced by migration of a small microtidal inlet against longshore drift: the Keurbooms Inlet, South Africa. Sedimentology 30, 201–218. doi:10.1111/j.1365-3091.1983.tb00665.x
Reinson, G. E. (1979). “Barrier island systems,” in Facies Models. Editor R. G. Walker (Toronto, Canada: Geosciences Canada), 57–74.
Reinson, G. E. (1984). “Barrier-island and associated strand-plain systems,” in Facies Models. Editor R. G. Walker (Toronto, Canada: Geological Association of Canada), 119–140.
Reinson, G. E. (1992). “Transgressive barrier island and estuarine systems,” in Facies Models: Response to Sea-Level Changes. Editors R. G. Walker, and N. P. James (St. John’s, Newfoundland: Geological Association of Canada) 179–194.
Reineck, H.-E., and Singh, I. B. (Editors) (1980). Depositional Sedimentary Environments, with Reference to Terrigenous Clastics. (Berlin, Germany: Springer-Verlag).
Roehler, H. W. (1988). The Pintail coal bed and barrier bar G - a model for coal of barrier bar—lagoon origin, Upper Cretaceous Almond Formation, Rock Springs Coal Field, Wyoming. U.S. Geological Survey Professional Paper 1398, 1—65.
Ronchi, L., Fontana, A., Correggiari, A., and Remia, A. (2019). Anatomy of a transgressive tidal inlet reconstructed through high-resolution seismic profiling. Geomorphology 343, 65–80. doi:10.1016/j.geomorph.2019.06.026
Roy, P. S., Cowell, P. J., Fernland, M. A., and Thom, B. G. (1994). “Wave-dominated coasts,” in Coastal Evolution: Late Quaternary Shoreline Morphodynamics. Editors R. W. G. Carter, and C. D. Woodroffe (Cambridge, UK: Cambridge University Press), 121–186.
Sabins, F. F. (1963). Anatomy of stratigraphic trap, Bisti Field, New Mexico. Am. Assoc. Petrol. Geol. Bull. 47, 193–228. doi:10.1126/science.58.1489.27
Savrda, C. E. (1991). Ichnology in sequence stratigraphic studies: an example from the Lower Paleocene of Alabama. Palaios 6, 39–53. doi:10.2307/2F3514952
Schatzinger, R. A., Szpakiewicz, M., and Sharma, B. (1989). Applicable correlations and overall characteristics of barrier island deposystems. Dep. Energy Rep. 427, 1–39.
Schwab, W. C., Baldwin, W. E., Denny, J. F., Hapke, C. J., Gayes, P. T., List, J. H., et al. (2014). Modification of the Quaternary stratigraphic framework of the inner-continental shelf by Holocene marine transgression: an example offshore of Fire Island. Mar. Geol. 355, 346–360. doi:10.1016/j.margeo.2014.06.011
Sedgwick, P. E., and Davis, R. A. (2003). Stratigraphy of washover deposits in Florida: implications for recognition in the stratigraphic record. Mar. Geol. 200, 31–48. doi:10.1016/S0025-3227(03)00163-4
Self, G. A., Breard, S. Q., Rael, H. P., Stein, J. A., Thayer, P. A., Traugott, M. O., et al. (1986). Lockhart Crossing Field: New Wilcox trend in southeastern Louisiana. Am. Assoc. Petrol. Geol. Bull. 70, 501–515.
Seminack, C. T., and Buynevich, I. (2013). Sedimentological and geophysical signatures of a relict tidal inlet complex along a wave-dominated barrier: assategue Island, Maryland, USA. J. Sediment. Res. 83, 132–144.
Shanley, K. W., and McCabe, P. J. (1995). “Sequence stratigraphy of Turonian-Santonian strata, Kaiparowits Plateau, southern Utah, USA: implications for regional correlation and foreland basin evolution,” in Sequence Stratigraphy of Foreland Basin Deposits. Editors J. C. Van Wagoner, and G. T. Bertram (Tulsa, OK, American Association of Petroleum Geologists Memior), Vol. 64, 103–136.
Shepard, F. P., and Moore, D. G. (1955). Central Texas Coast sedimentation: characteristics of sedimentary environment, recent history, and diagenesis. Am. Assoc. Petrol. Geol. Bull. 39, 1463–1593. doi:10.1306/5CEAE253-16BB-11D7-8645000102C1865D
Short, A. D. (1999). Handbook of Beach and Shoreface Morphodynamics. Chichester, UK: John Wiley & Sons.
Simms, A. R., Anderson, J. B., and Blum, M. (2006). Barrier-island aggradation via inlet migration: Mustang Island, Texas. Sediment. Geol. 187, 105–125. doi:10.1016/j.sedgeo.2005.12.023
Siringan, F. P., and Anderson, J. B. (1993). Seismic facies, architecture, and evolution of the Bolivar Roads tidal inlet/delta complex, East Texas Gulf Coast. J. Sediment. Petrol. 63, 794–808. doi:10.1306/D4267C08-2B26-11D7-8648000102C1865D
Sixsmith, P. J., Hampson, G. J., Gupta, S., Johnson, H. D., and Fofana, J. F. (2008). Facies architecture of a net transgressive sandstone reservoir analog: the Cretaceous Hosta Tongue, New Mexico. Am. Assoc. Petrol. Geol. Bull. 92, 513–547. doi:10.1306/01020807017
Steel, R. J., Plink-Bjorklund, P., and Aschoff, J. (2012). “Tidal deposits of the Campanian Western Interior Seaway, Wyoming, Utah and Colorado, USA,” in Principles of Tidal Sedimentology. Editors R. A. Davis, and R. W. Dalrymple (Dordrecht, Netherlands: Springer), 437–471. doi:10.1007/978-94-007-0123-6
Storms, J. E. A., Weltje, G. J., van Dijke, J. J., Geel, C. R., and Kroonenberg, S. B. (2002). Process-response modeling of wave-dominated coastal systems: simulating evolution and stratigraphy on geological timescales. J. Sediment. Res. 72, 226–239. doi:10.1306/052501720226
Stutz, M. L., and Pilkey, O. H. (2005). The relative influence of humans on barrier islands: humans versus geomorphology. Rev. Eng. Geol. 16, 137–147. doi:10.1130/2005.4016(12
Stutz, M. L., and Pilkey, O. H. (2011). Open-ocean barrier islands: global influence of climatic, oceanographic, and depositional settings. J. Coast Res. 27, 207–222. doi:10.2112/09-1190.1
Susman, K. R., and Heron, S. D. (1979). Evolution of a barrier island, Shackleford Banks, Carteret County, North Carolina. Geol. Soc. Am. Bull. 90, 205–215. doi:10.1130/0016-7606(1979)90<205
Swift, D. J. P. (1968). Coastal erosion and transgressive stratigraphy. J. Geol. 76, 444–456. doi:10.1086/627342
Swift, D. J. P. (1975). Barrier-island genesis: evidence from the central Atlantic Shelf. Sediment. Geol. 14, 1–43. doi:10.1016/0037-0738(75)90015-9
Swift, D. J. P., Niederoda, A. W., Vincent, C. E., and Hopkins, T. S. (1985). Barrier island evolution, middle Atlantic Shelf, U.S.A. Part I: shoreface dynamics. Mar. Geol. 63, 331–361. doi:10.1016/0025-3227(85)90089-1
Swift, D. J. P., Phillips, S., and Thorne, J. A. (1991). “Sedimentation on continental margins, V: parasequences,” in Shelf Sand and Sandstone Bodies: Geometry, Facies and Sequence Stratigraphy. Editors D. J. P. Swift, G. F. Oertel, R. W. Tillman, and J. A. Thorne (Oxford, UK: Special Publication of the International Association of Sedimentologists), Vol. 14, 153–187. doi:10.1002/9781444303933.ch5
Taylor, A. M., and Goldring, R. (1993). Description and analysis of bioturbation and ichnofabric. J. Geol. Soc. London. 150, 141–148. doi:10.1144/gsjgs.150.1.0141
Thom, B. G. (1984). Transgressive and regressive stratigraphies of coastal sand barriers in southeast Australia. Mar. Geol. 56, 137–158. doi:10.1016/0025-3227(84)90010-0
Tibert, N. E., and Leckie, R. M. (2004). High-resolution estuary sea level cycles from the Late Cretacous: amplitude constraints using agglutinated foraminifera. J. Foraminifer. Res. 34, 130–143. doi:10.2113/0340130
Timmons, E. A., Rodriguez, A. B., Mattheus, C. R., and DeWitt, R. (2010). Transition of a regressive to a transgressive barrier island due to back-barrier erosion, increased storminess, and low sediment supply: Bogue Banks, North Carolina, USA. Mar. Geol. 278, 100–114. doi:10.1016/j.margeo.2010.09.006
Tye, R., and Moslow, T. (1993). “Tidal inlet reservoirs: insights from modern examples,” in Marine Clastic Reservoirs: Examples and Analogues. Editors E. G. Rhodes, and T. F. Moslow (New York, NY: Springer-Verlag), 77–100.
Uhlir, D. M., Akers, A., and Vondra, C. F. (1988). Tidal inlet sequence, Sundance Formation (Upper Jurassic), north-central Wyoming. Sedimentology 35, 739. doi:10.1111/j.1365-3091.1988.tb01248.x
Wadsworth, J. A. (2010). Paralic coal as an indicator of accommodation space and correlation tool in terrestrial sediments. Appl. Mod. Stratigr. Tech. Theory Case Hist..
Wilkinson, B. H., McGowen, J. H., and Lewis, C. R. (1975). Ingleside strandplain sand of central Texas Coast. Am. Assoc. Petrol. Geol. Bull. 59, 347–352. doi:10.1306/83D91C95-16C7-11D7-8645000102C1865D
Willis, A. J., and Moslow, T. F. (1994a). Sedimentology and stratigraphy of tidal inlet reservoirs in the Triassic Halfway Formation, Wembley Field, Alberta. Bull. Can. Petrol. Geol. 42, 245–262.
Willis, A. J., and Moslow, T. F. (1994b). Stratigraphic setting of transgressive barrier-island reservoirs with an example from the Triassic Halfway Formation, Wembley Field, Alberta, Canada. Am. Assoc. Petrol. Geol. Bull. 78, 775–791. doi:10.1306/A25FE3B5-171B-11D7-8645000102C1865D
Willis, B. J., and Gabel, S. (2001). Sharp-based, tide-dominated deltas of the Sego Sandstone, Book Cliffs, Utah, USA. Sedimentology 48, 479–506. doi:10.1046/j.1365-3091.2001.00363.x
Wright, C. I., Miller, W. R., and Cooper, J. A. G. (2000). The late Cenozoic evolution of coastal water bodies in Northern Kwazulu-Natal, South Africa. Mar. Geol. 167, 207–229. doi:10.1016/S0025-3227(00)00032-3
Zhang, K., Douglas, B., and Leatherman, S. (2002). Do storms cause long term beach erosion along the U.S. east barrier coast? J. Geol. 110, 493–502. doi:10.1086/340633
Keywords: barrier island, shallow marine, shoreface, facies model, tidal inlet, transgressive deposits, paralic and shallow marine deposits
Citation: Mulhern JS, Johnson CL and Green AN (2021) When Is a Barrier Island Not an Island? When It Is Preserved in the Rock Record. Front. Earth Sci. 8:560437. doi: 10.3389/feart.2020.560437
Received: 08 May 2020; Accepted: 19 November 2020;
Published: 26 February 2021.
Edited by:
Ivar Midtkandal, University of Oslo, NorwayReviewed by:
Allard W. Martinius, Delft University of Technology, NetherlandsStuart Clarke, Keele University, United Kingdom
Copyright © 2021 Mulhern, Johnson and Green. This is an open-access article distributed under the terms of the Creative Commons Attribution License (CC BY). The use, distribution or reproduction in other forums is permitted, provided the original author(s) and the copyright owner(s) are credited and that the original publication in this journal is cited, in accordance with accepted academic practice. No use, distribution or reproduction is permitted which does not comply with these terms.
*Correspondence: Cari L. Johnson, Y2FyaS5qb2huc29uQHV0YWguZWR1