- 1Department of Geology, Centre of Advanced Study, Kumaun University, Nainital, India
Diorites, granites, and associated magmatic enclaves and dykes constitute the bulk of the Ladakh Batholith, which is an integral part of the Trans-Himalayan magmatic arc system. In this paper geometry of microgranular enclaves hosted in the granites has been examined from the Leh-Sabu-Chang La and surrounding regions of the eastern Ladakh Batholith to infer the mechanism and schedule of mafic to hybrid magma injections into evolving felsic magma chambers and the resultant enclave geometry. Mafic or hybrid magmas inject into felsic magma at low volume fraction (<0.35) of crystals and form the rounded to elongated microgranular enclaves in the Ladakh Batholith. Angular to subangular (brecciated), rounded to elongated pillow-like microgranular enclave swarms can also be documented as disrupted synplutonic mafic to hybrid dykes and sheets, when intruding the felsic magma with high volume fraction (>0.65) of crystals. A large rheological difference between coeval felsic and mafic magmas inhibits much interaction. Mafic magma progressively crystallizes and evolves while minimizing thermal and rheological differences. Consequently, the felsic-mafic magma interaction process gradually becomes more efficient causing the dispersion of enclave magma globules and undercooling into the partly crystalline felsic host magma. Thus the evolution of the Ladakh Batholith should be viewed as multistage interactions of mafic to hybrid magmas coeval with felsic magma pulses in plutonic conditions from its initial to waning stages of evolution.
Introduction
Granites (sensu lato) may contain any type of lithic inclusion or fragment, which is commonly referred to as enclave (a French term, Lacroix, 1890 in Didier, 1973). The diverse nature and origin of enclaves hosted in granites are described in many studies (e.g., Didier, 1984; Vernon, 1984; Furman and Spera, 1985; Castro et al., 1991a; Castro et al., 1991b; Shellnutt et al., 2010; Kumar et al., 2004a; Kumar et al., 2004b; Kumar et al., 2005; Clemens et al., 2016; Kumar et al., 2017), and are considered to represent: 1) xenolith (Sollas, 1894) as solid fragment of country rocks mostly confined to the margins of a pluton or may represent enroute deeper-derived lithology or unmelted source material, 2) surmicaceous enclave (Lacroix, 1933) as segregation of refractory source materials (restite) left after partial melting, 3) cognate or autolith (Pabst, 1928) as early-crystallized cumulus phases or segregation of mafic clots or fragments of chilled border rock series of cogenetic felsic magma, 4) microgranular (Didier and Roques, 1959) or microgranitoid (Vernon, 1983) enclaves representing felsic, mafic, and mafic-felsic (intermediate) hybridized magmas entrained, mingled, and undercooled into relatively cooler partly crystalline felsic host magma at any stage of its evolution. However, when mafic or hybrid magma is injected into a largely crystallized felsic magma chamber, it is commonly distributed as swarms of microgranular enclave together with synplutonic dykes (Barbarin, 1989; Kumar, 2014). In the present paper, the term enclave refers to the mafic and mafic-felsic (hybrid) varieties of microgranular or microgranitoid enclaves.
Enclaves in granites can offer significant insight into the understanding of operative physical and chemical processes during the evolution of a felsic plutonic system. The similar ages, chemical, and isotopic signatures of enclaves and host granites have been interpreted in various ways. Some favor the cogenetic relation between the enclave and host granites (e.g., Pin et al., 1990; Shellnut et al., 2010) whereas some argue their derivation from common crustal sources (e.g., Güraslan and Altunkaynak, 2019; Lu et al., 2020); and some advocate mafic-felsic magma mixing, mingling, chemical modification, and isotopic re-equilibration at varying degrees responsible for their partial to nearly equivocal chemical and isotopic features (e.g., Holden et al., 1987; Eberz and Nicholls, 1990; Elburg, 1996; Kumar and Rino, 2006; Adam et al., 2019). The geochemical and isotopic features do not provide unambiguously a viable model of enclave origin. Therefore in most cases, discussion on the origin of enclaves in granites largely revolves around the interpretations of megascopic and microscopic features (e.g., Didier, 1973; Sparks et al., 1977; Vernon, 1983; Eberz and Nicholls, 1988; Vernon et al., 1988; Barbarin, 1989, Castro, 1990; Didier and Barbarin, 1991; Fernandez and Gasquet, 1994; Kumar, 1995; Kumar et al., 2004a; Kumar et al., 2004b; Kumar, 2010a; Wiebe et al., 2007; Vernon, 2014).
The enclaves are usually ubiquitous in metaluminous (I-type), calc-alkaline granites, but are also found in other granite types such as anorogenic A−, hybrid H−, and shoshonitic SH-types. However, the enclaves are relatively rare in peraluminous (S-type) granites and absent in anatectic or leucogranite. The surmicaceous enclaves are rare in anorogenic (A-type), post-collisional granites (Didier, 1984). The enclaves may also enclose xenoliths and are considered to be double enclaves (Didier, 1987), however, large enclaves can enclose several other smaller enclaves, which can be referred to as composite enclaves (e.g., Kumar, 2010a). Zoned enclaves with different cooling history are also reported in the granites (e.g., Adam et al., 2019).
Felsic and associated magmatic rocks constitute the bulk of the Ladakh Batholith (600 km long, 20–80 km wide) roughly trending NW-SE covering an area of ca 30,000 sq km, which is an integral part of the Trans-Himalayan calc-alkaline magmatic arc system located in the north of Indus Tsangpo Suture Zone (ITSZ) (Figure 1A). The Ladakh Batholith is largely formed by the melting of Neo-Tethyan ocean crust subducted below the Asian plate during early Cretaceous-Lower Eocene (Thakur, 1992), and is characterized by mafic to felsic composite intrusions of variable compositions. The mafic-felsic magmatic suites range from gabbro-diorite-tonalite to granodiorite-granite-leucogranite of magnetite (oxidized) and ilmenite (reduced) series granites corresponding to metaluminous (I-type) and peraluminous (S-type) granites, respectively, which are crosscut by several post-plutonic mafic dykes (Auden, 1935; Wadia, 1937; Frank et al., 1977; Rai, 1980; Honegger et al., 1982; Ahmad et al., 1998, Kumar, 2008, Kumar, 2010b). Felsic magmatic rocks forming the Ladakh Batholith are collectively referred as granites unless stated for a specific rock type. Earlier mafic (diorite) stock-like bodies are also found disrupted by intruding granite magma that is more pronounced in the eastern parts of Ladakh Batholith (e.g., Weinberg, 1997; Kumar, 2010a; Kumar et al., 2016). Enclaves as xenoliths of older mafic volcanics in granites are also reported (Rai, 1980; Ahmad et al., 1998; Jain et al., 2003; Singh et al., 2007). Redox conditions of Ladakh granites and related rocks have been assessed in terms of magnetic susceptibility and phase petrology (Kumar, 2008; Kumar 2010b). The occurrence and origin of mafic to hybrid microgranular enclaves hosted in Ladakh Batholith are reported and discussed (Kumar, 2010a).
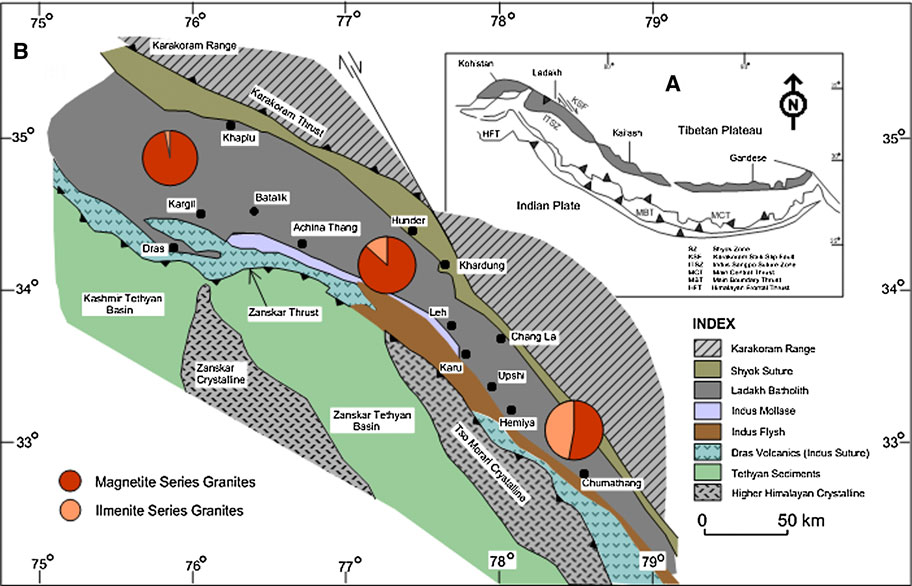
FIGURE 1. (A) Geological structure of Himalaya showing the tectonic elements, Trans-Himalayan magmatic arc and location of the Ladakh Batholith (after Gansser, 1977). (B) Geological map of Ladakh Himalaya (after Sharma and Chaubey, 1983; Thakur, 1987). Relative abundance of magnetite series and ilmenite series granites in western, central and eastern parts of the Ladakh Batholith is shown by wheel diagrams (Kumar, 2008; Kumar, 2010b).
In this paper, new spectacular field and petrographic features of microgranular enclaves, and dyke- and sheet-like enclave swarms, referred as synplutonic dykes, hosted in the granites exposed in and around Leh, Sabu, and Chang La regions of Ladakh Batholith have been documented and examined in order to understand the style and schedule of mafic to hybrid magma injections into felsic magma chambers from its initial to waning stages of evolution (i.e., with increasing crystallinity and rheology).
Geology, Geochemistry, and Geochronology
In the Trans-Himalaya, the Kohistan–Ladakh-Karakoram Batholiths have evolved as a single entity during the late Cretaceous and early Palaeogene (Pundir et al., 2020, and references therein). The Ladakh Batholith represents an integral part of the Trans-Himalayan calc-alkaline magmatic belt extending from east of Nanga Parbat to Lhasa (Figure 1A) and is bounded by Shyok Suture Zone (SSZ) in the north and ITSZ in the south (Figure 1B). The Asian plate in the north and Indian plate in the south are juxtaposed and accreted along the ITSZ with the closure of Neo-Tethyan Ocean. Ahmad et al. (1998) stated that the enclaves in the granites probably represent the initial pulses of magmatism in response to intra-oceanic northward subduction of Neo-Tethyan Ocean beneath an immature arc, and subsequently, a huge amount of felsic magmatism was generated as the arc matured forming the Ladakh Batholith.
The Ladakh Batholith is differentially unroofed in the western (p = 3.35–4.27 kbar), central (p = 2.99 kbar), and eastern (p = 2.17 kbar) parts, as inferred from Al-in-hornblende geobarometer (Kumar, 2010b). The FeOt/MgO ratio of biotites from the granites of western (1.44–2.19), central (1.31–1.90), and eastern (1.71–2.63) parts suggests the contribution of dominant calc-alkaline, metaluminous (I-type), and subordinate amount of peraluminous (S-type) felsic magmas in the evolution of Ladakh Batholith. Ahmad et al. (1998) observed that the enclaves and mafic dykes in granites of the Ladakh Batholith are basaltic-andesite and andesite belonging to subalkaline, calc-alkaline series in terms of major and trace elements. The negative relationship of MgO, Fe2O3t, MnO, TiO2, CaO, Al2O3 against SiO2, and positive trends of TiO2, CaO, CaO/Al2O3 against MgO may indicate fractionation of gabbroic assemblage, however, the absence of negative chondrite normalized Eu and primitive mantle normalized Sr anomalies shows that plagioclase was not an important fractionating phase. Based on geochemical features of enclaves, Ahmad et al. (1998) proposed the cognate or autolith origin for some selected enclaves sampled from western Ladakh, as inferred by Honegger et al. (1982), and others as entrained xenoliths of pre-existing mafic rock into the granites. However, Kumar and Singh (2008) suggest that the enclaves and host granites are largely as calc-alkaline, mildly to strongly metaluminous (molar Al2O3/CaO + Na2O + K2O = 0.9–1.05), which are compositionally different from diorite, gabbro, and mafic dykes. Near linear to curvilinear variation trends observed for TiO2, CaO, Fe2O3t, MgO, MnO, and TiO2 against SiO2 of enclaves and host granites can be attributed to synchronous mafic-felsic magma mixing and fractionation. The large data scatter for Al2O3, Na2O, K2O, P2O5, Rb, Ba, Nb, Yb, and Zr against SiO2 is, however, a combined outcome of elemental diffusion at varying degrees and sorting of minerals hosting these elements during mixing-fractionation events.
The extent and duration of calc-alkaline felsic magmatism constituting the Ladakh Batholith have been constrained based on a variety of chronological database, without taking into account the mafic to hybrid magma contributions. Magmatism within the Ladakh Batholith was most intense during Late Cretaceous to Early Paleogene (67 and 45 Ma) (Singh et al., 2007; Upadhyay et al., 2008; Jain and Singh, 2009; Ravikant et al., 2009; White et al., 2011a; White et al., 2011b; Kumar et al., 2012; Shellnutt et al., 2013; Jain 2014) with early discrete pulses at ca. 102 and 97 Ma identified in the western part (Honegger et al., 1982). Some sporadic geochronological data (Singh et al., 2007) on coarse grained diorite (58.4 ± 1.0 Ma) and granodiorite (60.1 ± 0.5 Ma) from Karu and Chang La regions of the Ladakh Batholith suggest a coeval nature between the diorite and granodiorite. A subvolcanic mafic dyke (45.7 ± 0.8 Ma) and granites (45.27 ± 0.56 Ma) at Daah-Hanu (Weinberg and Dunlop, 2000) provide the same ages of their formation. The likely reasons for synchronous diorite and granodiorite magmatism are not yet described. However, in and around the Leh and Sabu regions, stock-like dioritic bodies are also found as pre-existing older lithological units as compared to the granites (Kumar et al., 2016; Weinberg, 1997).
Materials and Methods
More than 35 outcrops along planned traverses exposed in and around the Leh-Sabu-Chang La regions of eastern Ladakh Batholith have been investigated in order to establish the field relationships between mafic lithological units including the enclaves and host granites. The enclave typology, synplutonic dykes and sheets, and their morphology, size, and contact relations with host granites were recorded and documented. The preferred criteria in classifying the enclave types are their macro‐ and micro‐structures (shapes: spheroidal to ellipsoidal; roundness: as their corners, and edges are rounded, subrounded or angular; contact relationships: sharp, diffused, crenulated to highly convoluted; primary igneous flow-lamination, fine- to coarse-grained phenocryst-bearing or phenocryst-free, cumulates, and solid-state deformation defined by foliation, schistosity, gneissosity, etc.). Representative rock thin sections were prepared for petrographic study and estimating the modal volume percentage of constituting minerals to assign the name of the rocks. The petrographic features relevant to infer the physical properties and processes such as viscosity contrast, magma-mixing, mingling, and undercooling have also been documented.
Megascopic and Microscopic Results
Field Observations
The Ladakh Batholith is mainly composed of diorite, granodiorite (±enclaves), leucogranite, and pegmatite. Granodiorite is the most dominant rock type among all of the observed varieties of granitic rocks. The granite hillocks with melanocratic bodies and patches of diorites are widespread in and around the Leh and Sabu regions. Such diorite outcrops as stock-like bodies are exposed widely at several sq meters to a hundred sq km scale, which can be seen even from far distances. The granite-leucogranite-pegmatite system intrudes the diorites (Figure 2A). Leucogranites and pegmatites are devoid of microgranular enclaves. The intrusive relationships are very well displayed by the presence of partially assimilated xenoliths of diorites and network of leucogranite-pegmatite veins (Figure 2B). At several places, the granite exhibits linear sharp contacts with the diorites. Near Sabu, the granite shows a rare feature of modally graded, crudely layered igneous structures with alternate bands of coarse grained mafic (hornblende, biotite) and felsic (quartz, feldspars) minerals (Figure 2C). At Leh, near the western end of town, undeformed, equigranular medium to coarse grained biotite granites are widely exposed, which occasionally host elongated fine grained mesocratic lithic materials showing diffuse boundaries with the host granites (Figure 2D). At this particular outcrop, amphibole is absent or rare and is devoid of enclaves.
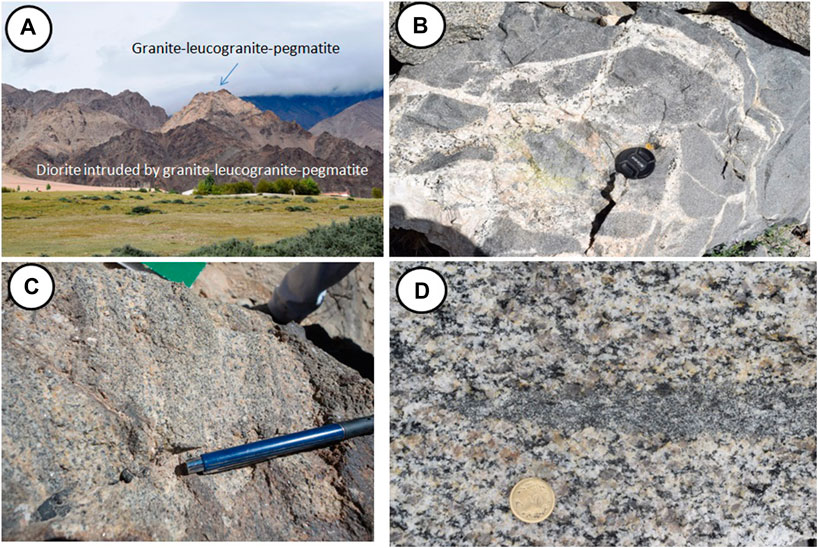
FIGURE 2. (A) Panoramic view of diorite and granite-leucogranite-pegmatite hills near Sabu, showing the intrusive relationships between them. (B) Diorite is dismembered as xenoliths and partially assimilated by the intruding granite-leucogranite-pegmatite system. (C) Modally graded crude igneous layering shown by alternate bands of mafic and felsic minerals in the granite. (D) Biotite granite contains elongated mesocratic fine grained facies with diffused boundaries.
At places, the fine grained mafic rock with devitrified glassy xenocrysts shows jigsaw-like crenulated margins (Figure 3A). A mesocratic porphyritic enclave of nearly circular shape with sharp and slightly diffused margins contains variable proportion of mafic-felsic xenocrysts that appear as phenocrysts (Figure 3B). The felsic xenocrysts, in particular, are rounded to subhedral that indicate their partial resorption in hybrid magma. Fine grained mafic enclaves commonly having subrounded to elongate shapes (up to 15 cm across) are intimately linked with fine mafic schlierens and aggregates. These mafic enclave swarms are oriented in a particular direction of magmatic flowage within the host granite, and they exhibit monadnock like features (Figure 3C). The enclaves showing all kinds of diverse geometry (rounded to subrounded, elliptical, angular to subangular, moderately to highly curved, platy and small spotted) and color index (holomelanocratic, melanocratic, and mesocratic) are randomly distributed and embayed within the host granite without any marked flowage pattern (Figure 3D). Some of them are closely spaced. At some outcrops, a few fine grained mafic enclaves (maximum up to 30 cm across) of variable shapes and sizes with sharp and irregular boundaries follow wavy trails of mafic schlierens that appear to be detached or disaggregated fragments of large enclave magma body (Figure 4A). Some moderately to highly stretched enclaves with or without diffused margins connected with straight and curvilinear mafic schlierens indicate an arrested (frozen) state of magmatic flowage and comingling structures (Figure 4B). These are typical magmatic features resulted from the disaggregation of enclaves during progressive stages from mingling to mixing.
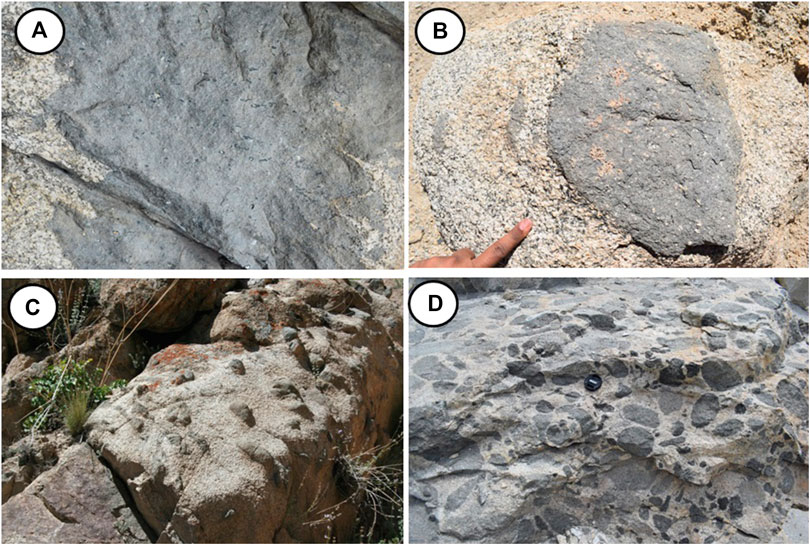
FIGURE 3. (A) Fine grained mafic body contains frequent quartz (glass) xenocrysts and shows serrated contacts with host granite. (B) A large enclave containing mafic-felsic phenocrysts showing sharp contact with host granite. Partial diffuse contact can also be noted. (C) Elongated mafic enclave swarms follow a particular direction in the host granite. Note the enclaves form monadnock ridge. (D) Randomly oriented closely spaced holomelanocratic, melanocratic to mesocratic enclaves with variable shapes and sizes are hosted in granite from northern margin of Ladakh Batholith.
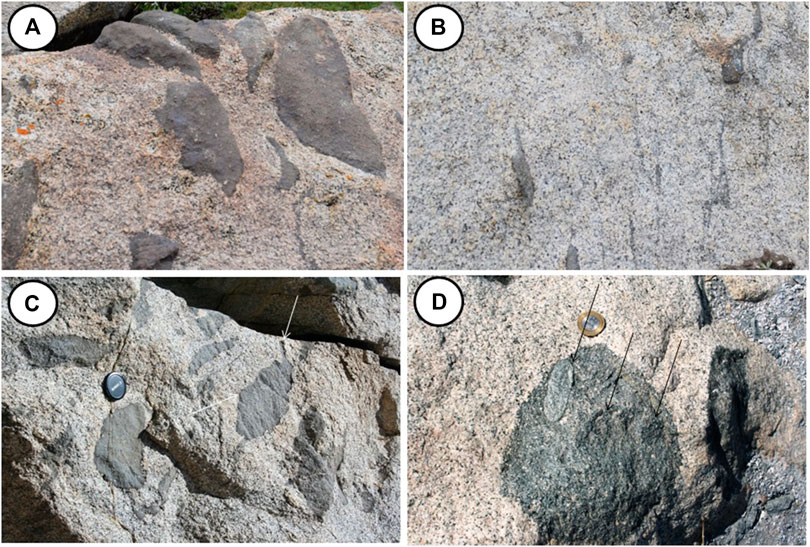
FIGURE 4. (A) Mafic enclaves with variable shapes and sizes showing the common sharp and occasional cuspate margins. Note the presence of minor mafic schlierens. (B) Mafic schlierens correlate well with mostly digested enclaves within the host granite. (C) Mafic enclaves of variable shapes and sizes. Note an enclave shows viscous finger texture and cuspate margin (white arrows). These features of enclaves are from a large part of outcrop as shown in Kumar (2010a). (D) A nearly circular composite enclave encloses circular melanocratic to elongate mesocratic enclaves (shown by black arrows).
An enclave showing viscous fingering texture (Figure 4C) also exhibits a crenulated boundary outline with a concave contact outline toward the felsic host, a kind of pillowing structure that is the result of differences in temperatures and viscosities between the interacting mafic and felsic magmas. A circular, relatively large, mesocratic hybrid (felsic-mafic xenocryst-bearing) enclave has sharp contact with felsic host granite (Figure 4D). A few small-sized fine grained, rounded to elongated, melanocratic to mesocratic enclaves are enclosed within the hybrid enclave (Figure 4D), which together form a composite enclave. The small enclaves yet again show sharp contacts with the enclosing hybrid enclave (Figure 4D). The composite enclaves can be seen at several outcrops in the Ladakh Batholith. Few elongated and stretched enclaves (Figures 5A,B) may give the wrong impression of solid-state deformational objects that are commonly considered suitable for marking the brittle or ductile strain. However, these features were most likely formed during synchronous mafic-felsic magmatic flowage under the influence of a prevailing convection current in the magma chamber.
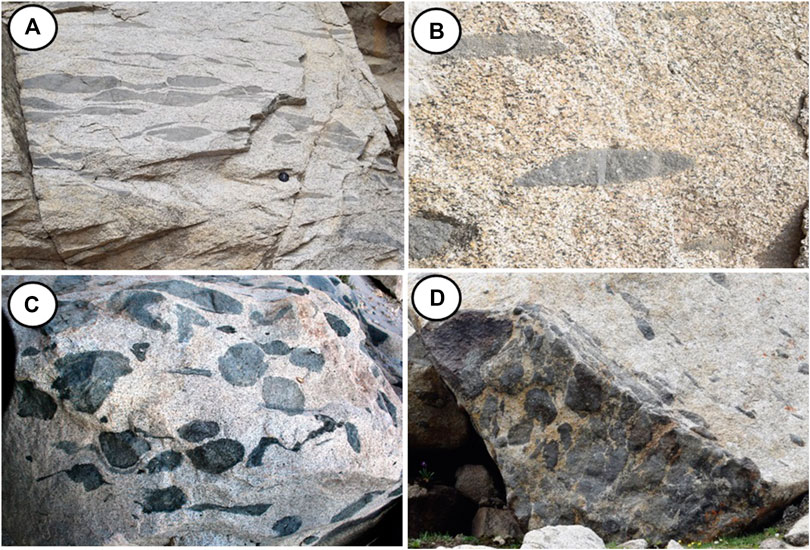
FIGURE 5. (A,B) Elongated and stretched enclaves follow a flow direction of host granite magma. (C, D) Transverse and longitudinal sections of outcrops showing diverse geometry of numerous enclaves that suggest the chaotic nature of some enclaves.
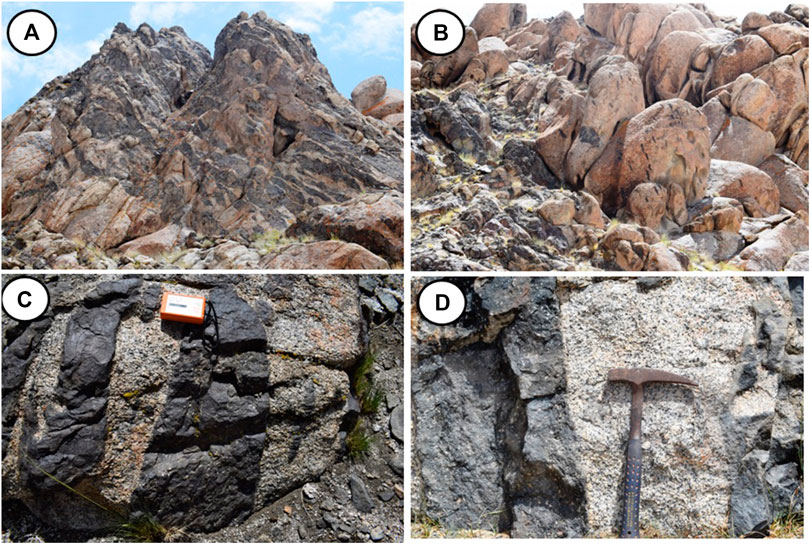
FIGURE 6. (A) Hillock showing disrupted mafic to hybrid synplutonic dykes and sheets injected into most crystallized granite. Note that synplutonic dykes and sheets are discontinuous and have got disrupted appearing pillowed but they broadly follow the same strike direction (NE-SE). (B) There are numerous mafic to hybrid enclave globules that are offset from dykes and sheets. (C) Closer view of part of mafic and hybrid synplutonic dykes. (D) Closer view of parts of hybrid synplutonic dykes. Note the sharp contact and straight contact outline of dyke with host granite, and felsic (quartz and K-feldspar) phenocrysts (xenocrysts) present in the hybrid synplutonic dyke.
A few outcrops of enclaves were preferably chosen, which can exhibit 3D geometry (longitudinal section across the horizontal plane) of the enclaves (Figures 5C,D). On both the surfaces, the enclaves show irregular to chaotic, subrounded, curvilinear, and extreme elongate geometry. Moreover, the enclaves exhibit variable color indices and sharp contacts with host granites (Figure 5C). On the horizontal surface, the enclave swarms display elongate, and spindle shaped geometry whereas on the longitudinal section they manifest closely spaced, platy, and irregular shapes (Figure 5D). Hence, the true geometry of enclaves cannot be easily discerned in the field.
Synplutonic (or synmagmatic) fine grained (mafic) to porphyritic (hybrid) composite dykes intrude the granites at some places. Some of them appear as pillow-like sheets. These are the spectacular field features formed by the injection of mafic or hybrid magmas into the mostly crystallized (>65%) host granite magma (Figures 6A–D). These composite dykes and sheets are emplaced and exposed on both sides of a hillock covering a small area of about 5,000 sq meters. These dykes and sheets are discontinuous and disrupted to form platy and parallel enclave swarms, which are oriented along the dyke strike direction (NE-SW). They commonly have sharp contacts with host granites (Figure 6A). A closer view of the dykes reveals their mafic (Figure 6C) and hybrid (Figures 6C,D) characteristics. Some offset of these injections form rounded to elliptical shaped enclaves (Figure 6B), because of the heterogeneous viscosity of host granite magma. This is primarily governed by differential crystal contents that may allow dispersal of injecting mafic to hybrid magmas as enclave globules. The synplutonic hybrid dykes contain abundant felsic xenocrysts (Figure 6D) that are sourced and injected from mafic-felsic mixed zone hidden at depth below the host felsic magma. The host granite shows medium to coarse grained equigranular textures.
A synplutonic mafic injection into partly crystalline (>65%) granite is moderate to highly disrupted to form clusters of angular (brecciated), subrounded, and elliptical enclave swarms (Figure 7C), which are commonly aligned, disposed, and broadly meander in the strike directions of N-S, E-W, and NE-SW. The disruption of synplutonic mafic dykes (nearly crystallized) into enclave swarms within the granite matrix depends upon the rheology of felsic host magma. This determines the yield strength (crystal-mush plus residual-melt), viscous passages of mafic intrusion, and flow departure of enclave swarms likely under the influence of tensional stress applied to the felsic magma chamber with heterogeneous crystal contents, as demonstrated in the model (Figures 7A,B). It is remarkable that at this outcrop only a mafic synplutonic dyke has intruded the granite whereas in the previously described outcrop both the mafic and hybrid synplutonic dykes have injected into the granite.
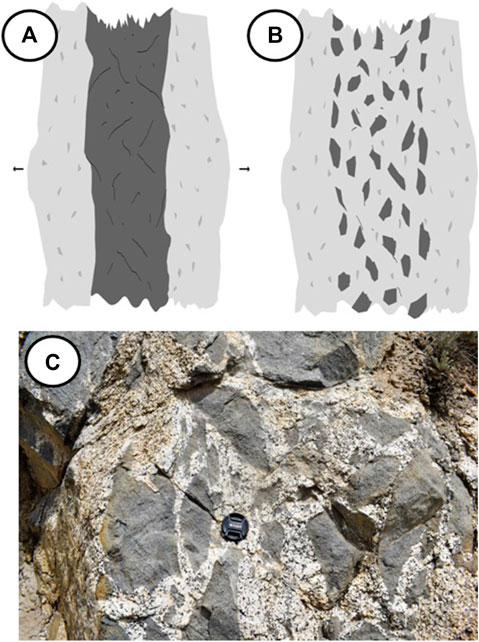
FIGURE 7. Hypothetical model illustrating the likely mechanism of (A) injection of mafic magma into highly crystallized (>65%) felsic granite magma that (B) ruptured and brecciated into angular mafic enclave swarms under the extensional environment of granite magma, which is very well demonstrated (C) at outcrop scale, similar to as shown in Kumar et al. (2016).
Microstructural Features
The modal mineralogy of the studied granites represents quartz diorite, granodiorite, and monzogranite whereas enclaves are micro-monzodiorite, micro-quartz diorite, micro-tonalite, and micro-monzogranite. The granites and enclaves have a common mineral assemblage (hbl-bt-pl-Kf-qtz-ap-zrn-mag ± ilm; mineral symbols are after Kretz, 1983) but they differ in modal proportion, the enclaves being enriched in mafic minerals.
Microscopically, the enclaves exhibit typical magmatic textures such as fine grained, equigranular, hypidiomorphic to porphyritic (Figures 8, 9). The contacts between the enclaves and host granites are sharp and wavy without any reaction signature (Figures 8A,B). In some instances, the contact outline between them is marked by the grain boundaries of either plagioclase or poikilitic amphibole crystallized in the host granite (Figures 8A,B). The grain boundaries of these minerals are devoid of any thermal effect and probably served as surfaces against which the enclave magma globules might have undercooled uniformly, and hence produced fine grained equigranular texture. In some enclaves, biotite dominates over the amphiboles and vice versa (Figures 8A,B). The presence of magnetite both in the enclave and granite indicates oxidizing condition, which is a characteristic of calc-alkaline subduction zone magmatism. The small flaky and platy biotites are commonly aligned along the contact outline (Figure 8A), and hence could not penetrate the semi-solid enclaves during the mingling event.
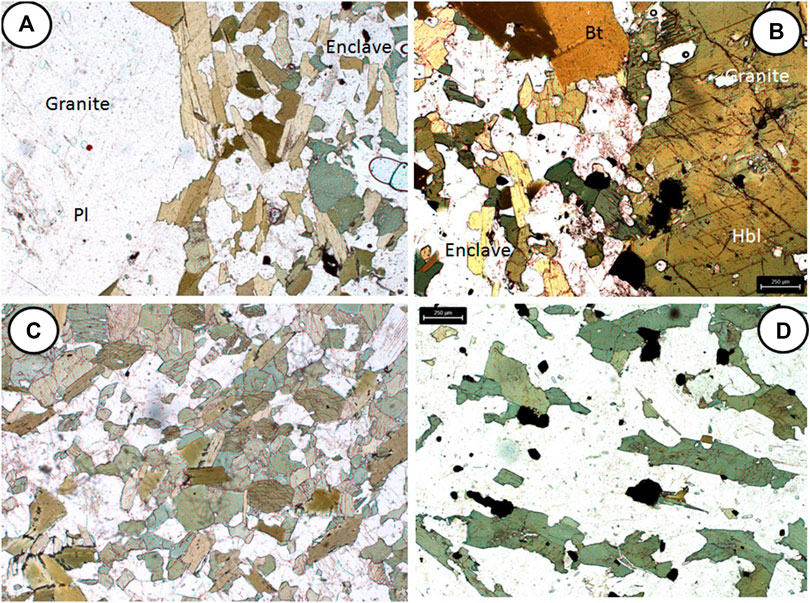
FIGURE 8. (A) Microphotographs showing magmatic textures and contact relationships between the enclave and host granite. Note the sharp contact of enclave against the plagioclase of granite. Plane polarized. (B) Contact between the enclave and host granite is sharp and crenulated without any reaction signature even at the microscopic level. Crossed polar. The base of the photograph equals 2.5 mm. (C) Mafic enclave enriched in amphibole and biotite, which are aggregated at places with interstitial quartz and feldspars. (D) Elongated and anhedral crystals of amphiboles exhibit magmatic flowage. Note the acicular apatite in the interstitial felsic minerals. Plane polarized. The base of the photograph equals to 2.5 mm.
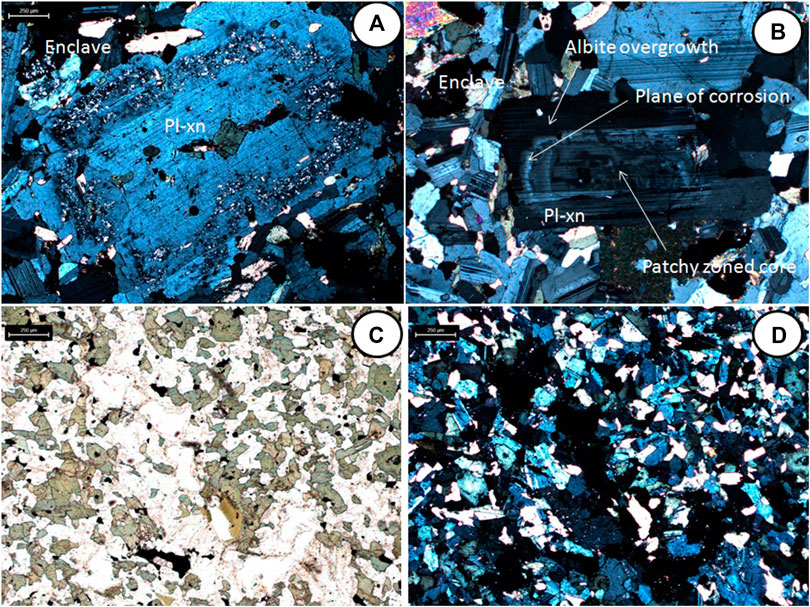
FIGURE 9. (A) Plagioclase xenocryst (Pl‐xn) in the porphyritic (hybrid) enclave. Note the sericitization along the plane of corrosion shown by a white arrow. Crossed polar. (B) Plagioclase xenocryst (Pl‐xn) in porphyritic (hybrid) enclave showing patchy zoned core, corroded margin with the growth of An-rich plagioclase (bright gray) and albite rim. Crossed polar. The base of the photograph equals 2.5 mm. (C) Predominating amphibole and subordinate biotite show subpoikilitic relation with plagioclase laths in mafic synplutonic dyke. Note the quartz rinds in the center surrounded by minute dusty mafic grains. Plane Polarized. (D) Crossed polar view of the same as (C). The base of the photograph equals 2.5 mm.
In a few enclaves, subhedral to euhedral amphiboles along with a minor amount of biotite are clustered and form the mafic aggregates (Figure 8C) that are in close association with tabular plagioclase, interstitial quartz, and K-feldspar. Subhedral to anhedral elongate amphiboles along with magnetite, quartz, and plagioclase show magmatic flowage texture (Figure 8D). Small acicular (needle-shaped) apatite crystals can also be observed poikilitically enclosed within the interstitial felsic minerals, which result from the undercooling of enclave magma globules. Porphyritic enclaves frequently contain plagioclase phenocrysts with normal, patchy, and oscillatory zonings (Figures 9A,B). They exhibit resorption surfaces as a corroded (dissolution) feature over which albitic rims are grown (Figure 9B). In some cases, the albitic rim appears to be replaced by sericite (Figure 9A). Synplutonic mafic dykes and sheets (partly pillow-like) show a typical interlocking subpoikilitic texture where plagioclase laths are partially enclosed by anhedral to subhedral amphiboles and less frequently by biotite, commonly associated with granular magnetite (Figures 9C,D).
Discussion
Diorite Xenoliths, Layered Granite, and Cognate Enclave
Pre-existing stock-like dioritic bodies (Weinberg, 1997) are intruded by the granite-leucogranite-pegmatite system, as evident from the presence of dioritic xenoliths hosted in the intruding lithological units (Figures 2A,B). It is likely that the Neo-Tethyan oceanic subduction-related magmatism may have been initiated with the emplacement of diorite followed by profuse granite magmatism in the Ladakh Himalaya. The rarely occurring layered structure (Figure 2C) in the granite, exposed close to Sabu, is likely due to mafic-felsic mineral sorting under the influence of double diffusion convection and gravity settling operated in a magma chamber (e.g., McBirney, 1985), and not due to the enclave-forming magmatic pulse. The hornblende biotite granite is a major rock constituent of the Ladakh Batholith, which commonly hosts the microgranular enclaves. However, a biotite granite exposure (Figure 2D) contains a fine grained mesocratic enclave with diffused contacts (Figure 2D), which possibly represents cognate enclave of early crystallized fine grained cumulus phases of host magma.
Evidence Against Restite Origin of Enclaves in Ladakh Batholith
The origin of igneous enclaves hosted in the granites is still debated (Clemens et al., 2018, and references therein). The presence of mafic clots, inherited and metamorphic zircons, and Ca-rich patchy zoned (resorbed) plagioclase in enclaves favors the restite origin of enclaves (e.g., Chappell et al., 1987; Chen et al., 1989; Huang et al., 2018). The studied enclaves from Ladakh Batholith, however, lack partial melting textures, deformational, or recrystallization features. In contrast, the enclaves show typical fine grained to porphyritic textures, acicular apatite, some elongated amphiboles (Figures 8C,D, 9A,B), sharp, and lobate enclave-granite contact outlines (Figures 3–5), and patchy zoned core with a corrosive surface of plagioclase phenocrysts (Figures 9A,B), which together oppose the restite origin of enclaves. The observed anorthite rim (bright gray zone) grown over the corroded margin of plagioclase xenocrysts (Figures 9A,B) likely crystallized in a new mafic-felsic magma mixed (high-T) environment (e.g., Hibbard, 1981; Andersson, 1988; Andersson and Eklůnd, 1994; Kumar et al., 2017). The enclave-bearing granite may have, however, experienced later tectono-thermal deformation (e.g., Siebel et al., 2012).
Disproving the Cognate or Cumulate Model of Enclave Origin
The cognate model states that the enclave is a cumulate rock of early crystallized cumulus phases or rapidly cooled (chilled) border rock series of the host granite magma or more mafic cogenetic fractions with trapped interstitial melts (e.g., Phillips et al., 1981; Dodge and Kistler, 1990; Dahlquist, 2002; Esna-Ashari et al., 2011; Xiao et al., 2020). The cumulate hypothesis appears valid where the evidence of excessive nucleation of ferromagnesian minerals by rapid cooling at the margins of a shallow magma chamber and later its fragmentation and dispersal by dynamic arc plutonism is preserved (e.g., Esna-Ashari et al., 2011). Similarly, reheating and remelting of the mafic chilled margin in the ascent conduits can be dislocated by dyke injection and the host rocks can be fragmented to sizes similar to the enclaves (e.g., Fernandez and Castro, 2018). However, in the present case, numerous features such as sharp and crenulate contact outline (Figures 3–5), xenocrystic-bearing (hybrid) enclaves (Figures 9A,B), and large grain size contrast between the enclave and host granite (Figures 8A,B) strongly oppose cognate origin of enclaves. Coarse grained plutonic xenoliths have been reported in the volcanic system (Cooper et al., 2019) but in the felsic plutonic system, they represent early formed crystal mush pooled at the base of the magma chamber (e.g., Wiebe et al., 1997).
Evidence of Mafic-Felsic Magma Interaction, Mingling, and Undercooling
The mafic-felsic magma interacting system truly represents a composite mafic-silicic intrusive system (Wiebe, 1980; Wiebe, 1994) typical to those observed in the present study.
Most magmas experience three rheological states during crystallization, 1) Newtonian state at a low fraction of crystals (F < 0.35), 2) Bingham behavior or stress thinning state with yield stress (F = 0.35–0.65), and 3) solid-like behavior (F > 0.65) (Fernandez and Gasquet, 1994, and references therein). Coeval mafic and felsic magmas with contrasting temperatures and compositions achieve thermal equilibration at which crystallization is more advanced in the mafic magma rather than the felsic magma. At this point, an inversion temperature exists that governs relative viscosities of magmas such as mafic magma becomes less viscous than felsic magma at a temperature higher than the inversion temperature, and vice versa. Thus, the generated inversion of viscosity between the coeval mafic and felsic magmas can explain most of the features described here (e.g., Frost and Mahood, 1987; Fernandez and Gasquet, 1994). However, an open felsic magma chamber intermittently recharged by mafic to hybrid magma pulses may behave in a different and more complex manner. Magma convection ceases once the magma chamber develops a crystallinity of ca 40–50% (Chen et al., 2018).
Enclaves of variable shapes and sizes are formed within the host granite magma with a low crystallinity at an initial stage of its evolution. The enclaves may lack a chilled margin because small size enclaves (ca. 15 cm) are not capable to generate a thermal gradient within it, and hence undercooled at a uniform rate. The large sized enclave globules may, however, generate a thermal gradient forming the chilled margin and coarser core, because they behave as micro-plutons. It is therefore suggested that each enclave partially crystallizes as a discrete body with a unique cooling history (e.g., Rooyakkers et al., 2018). Numerical modeling of deformation of a crystal-rich enclave in a pure shear regime suggests that enclave particles pack early, quickly erasing differences in initial content and building a force chain parallel to the compression axis that transmits stresses to the host granite (Burgisser et al., 2020). It is observed that the enclaves are not deformed under solid state and display similar magnetic fabrics that imply mafic magma globules coexisted and flowed with felsic host magma (Zhu et al., 2018). The mineral constituents of the studied enclaves of the Ladakh Batholith are solely unaffected by deformation, recrystallization, and solid-liquid reaction rims (Figures 8, 9). Vernon (1983) advocated if the magmatic flowage passes through the enclaves, the homogeneity may approach partial to complete disappearance of enclaves, as observed at some places in the present case (Figures 4A,B). This is a process leading to homogenization under a flow-favoured magma mixing environment (e.g., Reid and Hamilton, 1987; Reid et al., 1983; Castro, 1990).
How are the enclaves with diverse geometry and nature brought together at one place (Figures 3D, 5C) in a felsic magma chamber? The most plausible reason is either whole body convection current in the magma chamber or due to gravity settling at an early stage of granite evolution (e.g., Wiebe et al., 2007). However, over the time scale of magma mixing and mingling, convective patterns are difficult to recognize (Montagna et al., 2015). The absence of mafic schlierens among the randomly distributed enclave population indicates the transportation of enclaves in a much weaker medium (low viscosity) of host granite magma. Igneous lamination and schlieren in granites (Figures 3B, 4A,B) are, however, an expression of concurrent magma injection, aggregation with or without mingling/hybridization, hydrodynamic processes coupled with fractional crystallization, and deformation during the growth of plutons (Barbey, 2009). Thus, within a given volume of Ladakh granites and based on the type and behavior of enclaves, one can recognize the comingling and hybrid zones (e.g., Mariano and Sial, 1988; Perugini et al., 2003). Although enclaves do not provide precise information on the depth (pressure) where the felsic-mafic magmas were mixed, the enclaves appear to have ascended from greater depths than their surrounding host granites (Didier et al., 1989).
Schematic Model of Enclave Origin
Water content, crystal size, degree of crystallinity, mass fraction, composition, and temperature determine the rheology of interacting mafic and felsic magmas (Frost and Mahood, 1987). Low viscosity, minimal rheological differences, and thermal equilibrium between the coeval mafic and felsic magmas will produce convective overturn (Huppert et al., 1984; Sparks and Marshall, 1986) forming a hybrid magma zone hidden below the felsic magma at crustal depth. Under the influence of turbulent convection, the hybrid magma may also be injected into the overlying felsic magma, forming either rounded to elongated hybrid enclave globules or disrupted as angular brecciated to dyke-/sheet-like enclave swarms depending upon the schedule of its injection into evolving felsic magma with changing rheology (e.g., Barbarin, 1989; Fernandez and Barbarin, 1991; Barbarin and Didier, 1992; Fernandez and Gasquet, 1994; Kumar, 2014), as shown by a schematic model (Figure 10). A large fine grained circular composite enclave (Figure 4D) lacks zoning. Zoned enclaves are commonly formed either by internal differentiation mechanism or multiple magma mixing and mingling events from magma source to emplacement level (Castro et al., 1990; Zhang et al., 2014; Zhang et al., 2016) or in two different, deep and shallower, magma chambers (Adam et al., 2019). However, the observed composite enclaves (Figure 4D) appear to have formed in an open felsic magma chamber that was essentially recharged intermittently by mafic to hybrid magmas pooled just below the felsic magma chamber (Figure 11), as similarly suggested for the origin of composite and other types of enclaves found in the granites of western Ladakh Batholith (Kumar, 2010a).
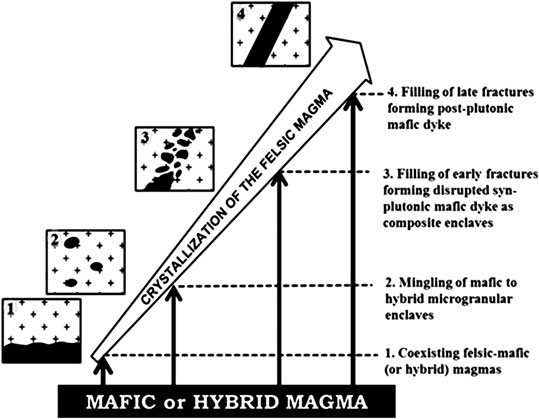
FIGURE 10. Schematic presentation of mafic to hybrid magma injections into the progressively crystallizing felsic magma chamber and the resultant geometry of enclaves (after Barbarin, 1989; Barbarin and Didier, 1992, with the addition of “hybrid” by Kumar, 2014).
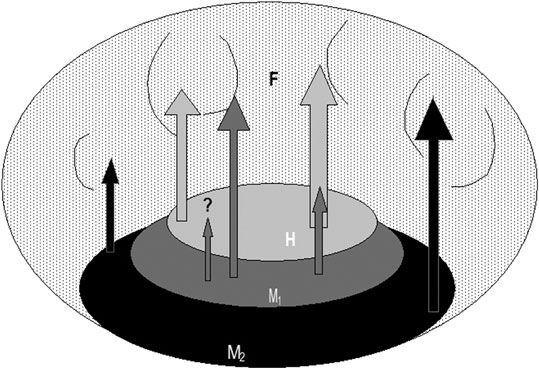
FIGURE 11. A simplified schematic cartoon showing the most viable genetic model of mafic to hybrid and composite enclaves in granites. Mafic magma (M1) pooled below and mixed with the partly crystalline felsic magma chamber to form hybrid magma (H) zone. Both the mafic and felsic magmas were crystal charged. Another pulse of mafic magma (M2) accumulated below the M1. The mafic to hybrid magmas were injected into the overlying felsic magma chamber during its initial to waning stages of crystallization and formed the various enclave types observed in the studied granites of Ladakh Batholith (Based on Kumar, 2010a, and references therein). Post plutonic dyke intrudes after the consolidation of granite magma.
A few sheet-like bodies appear to be pillowed against relatively cooler host granite magma (Figure 6A), similar to as described elsewhere (e.g., Clemens et al., 2016). Mafic synplutonic dykes contain a high volume of crystal content (Figures 9C,D) and hence behaved more like a solid and become brecciated angular enclaves (Figures 7A–C). Depending upon the viscosity of enclave-hosting felsic magma and prevailing local strain, the structures like flowing or static breccias may develop (Fernandez and Gasquet, 1994). Brecciation and disruption (break-up) of mafic to hybrid synplutonic dykes (Figures 6A, 7C) might have occurred within a transition zone between the crystal mush (>65%) and melt condition of host felsic magma (Janoušek et al., 2000; Rooyakkers et al., 2018). However, the hybrid dyke magma might have been sourced from the felsic-mafic hybrid magma zone from where the other hybrid enclave globule types were also derived (e.g., Kumar and Rino, 2006), and hence they could be genetically linked (Zhang et al., 2014). These aspects need to be tested further using geochemical and isotopic investigations.
Conclusions
• Melanocratic to mesocratic, mafic to hybridized, rounded to elliptical microgranular enclaves hosted in the granites of Ladakh Batholith are considered products of mingling and undercooling of enclave magma globules against a relatively cooler, partly crystalline felsic host magma in a plutonic environment.
• It is more likely that felsic magma chambers were fed intermittently by mafic to hybrid magma pulses throughout its crystallization history with progressively changing rheology, and formed the enclave globules, synplutonic mafic dykes, sheets, and disrupted enclave swarms.
• Large sized enclave globules may get disaggregated into several smaller ones or partly to completely homogenize within the host granites depending upon the crystal content (<35%) and intensity of convection current prevailed in the chamber.
• The Ladakh Batholith is formed by composite plutons evolved through multistage interactions of coeval mafic to hybrid and felsic magma pulses.
Data Availability Statement
All datasets presented in this study are included in the article/supplementary material.
Author Contributions
SK is the sole author of the paper and the principal investigator, fund manager, and administrator of the research project on Ladakh Batholith. He is responsible for the conceptualization, data curation, validation, visualization, format analysis, methodology, writing, review, and editing of the article.
Funding
DST‐Science and Engineering Research Board (SERB), New Delhi Grant (EMR/2017/002420).
Conflict of Interest
The authors declare that the research was conducted in the absence of any commercial or financial relationships that could be construed as a potential conflict of interest.
Acknowledgments
The infrastructural facilities developed under UGC-CAS-I & II and DST-FIST-II programmes in the Department of Geology, Kumaun University are gratefully acknowledged. Rajeev K. Mahajan, Rajwant, and Prahlad Ram, SERB, Delhi are thanked for the continuous encouragement. C. P. Dorjey, Karma, and Kapil S. Panwar helped during the geological fieldwork. Manjari Pathak, Saurabh Gupta, and Anjali Solanki helped to document the microstructural features and digitizing the figures. The generous scientific comments from two reviewers helped to improve the earlier versions of the manuscript. J. G. Shellnutt is also thanked for thorough editorial works and correcting the English and grammar of the manuscript. A few remarks from Catherine Annen, Specialty Chief Editor, Petrology Section, also helped improving the manuscript.
References
Adam, M. S. M., Kim, T., Song, Y.-S., and Kim, Y.-S. (2019). Occurrence and origin of the zoned microgranular enclaves (MEs) within the Cretaceous granite in Taejongdae, SE Korea. Lithos 324–325, 537–550. doi:10.1016/j.lithos.2018.11.008
Ahmad, T., Thakur, V. C., Islam, R., Khanna, P. P., and Mukherjee, P. K. (1998). Geochemistry and geodynamic implications of magmatic rocks from the trans-Himalayan arc. Geochem. J. 32, 383–404. doi:10.2343/geochemj.32.383
Andersson, U. B., and Eklund, O. (1994). Cellular plagioclase intergrowths as a result of crystal-magma mixing in the Proterozoic Åland rapakivi batholith, SW Finland. Contrib. Mineral. Petrol. 117, 124–136. doi:10.1007/bf00286837
Andersson, U. B. (1988). Evidence of plutonic magma mixing, Southern Sweden. Rec. Soc. Ital. Mineral. Petrol. 43, 609–618
Barbarin, B. (1989). Importance des différents processus d’hybridation dans les plutons granitiques du batholite de la Sierra Nevada, Californie. Schweiz Mineral Petrogr. Mitt. 69, 303–315.
Barbarin, B., and Didier, J. (1992). Genesis and evolution of mafic microgranular enclaves through various types of interaction between coexisting felsic and mafic magmas. Earth Sci. 83, 145–153. doi:10.1017/S0263593300007835
Barbey, P. (2009). Layering and schlieren in granitoids: a record of interactions between magma emplacement, crystallization and deformation in growing plutons. Geol. Belg. 12, 109–133.
Burgisser, A., Carrara, A., and Annen, C. (2020). Numerical simulations of magmatic enclave deformation. J. Volcanol. Geoth. Res. 392, 106790. doi:10.1016/j.jvolgeores.2020.106790
Castro, A. (1990). Microgranular enclaves of the quintana granodiorite (los pedroches batholith): petrogenetic significance. Rev. Soc. Geol. España 3, 7–21.
Castro, A., de la Rosa, J. D., and Stephens, W. E. (1990). Magma mixing in the subvolcanic environment: petrology of the Gerena interaction zone near Seville, Spain. Contrib. Mineral. Petr. 106, 9–26. doi:10.1007/bf00306405
Castro, A., Moreno-Ventas, I., and de la Rosa, J. D. (1991a). H-type (hybrid) granitoids: a proposed revision of the granite-type classification and nomenclature. Earth Sci. Rev. 31, 237–253. doi:10.1016/0012-8252(91)90020-g
Castro, A., Moreno-Ventas, I., and de la Rosa, J. D. (1991b). Multistage crystallization of tonalitic enclaves in granitoid rocks (Hercynian belt, Spain): implications for magma mixing. Geol. Rundsch. 80, 109–120. doi:10.1007/bf01828770
Chappell, B. W., White, A. J. R., and Wyborn, D. (1987). The importance of residual source material (restite) in granite petrogenesis. J. Petrol. 28, 1111–1138. doi:10.1093/petrology/28.6.1111
Chen, C., Xing, D., Rui, L., Qi, Z. W., Jian, O. D., Lei, Y., et al. (2018). Crystal fractionation of granitic magma during its non-transport processes: a physical-based perspective. Sci. China Earth Sci. 61, 190–204. doi:10.1007/s11430-016-9120-y
Chen, Y. D., Price, R. C., and White, A. J. R. (1989). Inclusions in three S-type granites from southeastern Australia. J. Petrol. 30, 1181–1218. doi:10.1093/petrology/30.5.1181
Clemens, J. D., Elburg, M. A., and Harris, C. (2017). Origins of igneous microgranular enclaves in granites: the example of Central Victoria, Australia. Contrib. Mineral. Petrol. 172, 88. doi:10.1007/s00410-017-1409-2
Clemens, J. D., Regmi, K., Nicholls, I. A., Weinberg, R., and Maas, R. (2016). The Tynong pluton, its mafic synplutonic sheets and igneous microgranular enclaves: the nature of the mantle connection in I-type granitic magmas. Contrib. Mineral. Petrol. 171, 35. doi:10.1007/s00410-016-1251-y
Cooper, F. G., Blundy, J. D., Macpherson, C. G., Humphreys, M. C. S., and Davidson, J. P. (2019). Evidence from plutonic xenoliths for magma differentiation, mixing and storage in a volatile-rich crystal mush beneath St. Eustatius, Lesser Antilles. Contrib. Mineral. Petrol. 174, 39. doi:10.1007/s00410-019-1576-4
Dahlquist, J. A. (2002). Mafic microgranular enclaves: early segregation from metaluminous magma (Sierra de Chepes), Pampean Ranges, NW Argentina. J. S. Am. Earth Sci. 15, 643–655. doi:10.1016/s0895-9811(02)00112-8
Didier, J. (1973). “Granites and their enclaves: the bearing of enclaves on the origin of granites,” in Developments in petrology. Amsterdam: Elsevier, Vol. 3.
Didier, J. (1984). “The problem of enclaves in granitic rocks: a review of recent ideas on their origin,” in Proceedings of international symposium on Geology of granites and their metallogenetic relations. Editors K. Q. Xu and G. C. Tu (Nanjing and Beijing, China: Nanjing University, Science Press), 137–144.
Didier, J. (1987). Contribution of enclave studies to the understanding of origin and evolution of granitic magmas. Geol. Rundsch. 76, 41–50. doi:10.1007/bf01820572
Didier, J., and Barbarin, B. (1991). “Enclaves and granite petrology,” in Developments in petrology. Amsterdam: Elsevier, Vol. 13, 625.
Didier, J., Fernandez, A., and Elmouraouah, A. (1989). A model for the genesis of granitic magmas by crustal melting around mafic inclusions: the Peyron near Burzet (Ardeche, Massif Central, France). Athens: Theophrastus Publication Sa, 163–192.
Didier, J., and Roques, M. (1959). Sur les enclaves des granites du Massif Central Français. Comptes rendus de l’Académie des sciences, Paris [On the enclaves of the granites of the French Massif Central. Proc. Acad. Sci. 228, 1839–1841.
Dodge, F. C. W., and Kistler, R. W. (1990). Some additional observations on inclusions in the granitic rocks of the Sierra Nevada. J. Geophys. Res. 95, 17841–17848. doi:10.1029/jb095ib11p17841
Eberz, G. W., and Nicholls, I. A. (1988). Microgranitoid enclaves from the Swifts Creek Pluton SE-Australia: textural and physical constraints on the nature of magma mingling processes in the plutonic environment. Geol. Rundsch. 77, 713–736. doi:10.1007/bf01830179
Eberz, G. N. W., and Nicholls, I. A. (1990). Chemical modification of enclave magma by post-emplacement crystal fractionation, diffusion and metasomatism. Contrib. Mineral. Petrol. 104, 47–55. doi:10.1007/bf00310645
Elburg, M. A. (1996). Genetic significance of multiple enclave types in a peraluminous ignimbrite suite, Lachlan fold belt, Australia. J. Petrol. 37, 1385–1408. doi:10.1093/petrology/37.6.1385
Esna-Ashari, A., Hassanzadeh, J., and Valizadeh, M.-V. (2011). Geochemistry of microgranular enclaves in Aligoodarz Jurassic arc pluton, western Iran: implications for enclave generation by rapid crystallization of cogenetic granitoid magma. Miner. Petrol. 101, 195. doi:10.1007/s00710-011-0149-7
Fernandez, A. N., and Barbarin, B. (1991). “Relative rheology of coeval mafic and felsic magmas: nature of resulting iteration processes and shape and mineral fabrics of mafic microgranular enclaves,” in Enclaves and granite petrology. Developments in petrology, Editors Didier, J., and Barbarin, B. (Amsterdam: Elsevier), Vol. 13, 263–275.
Fernandez, A. N., and Gasquet, D. R. (1994). Relative rheological evolution of chemically contrasted coeval magmas: example of the Tichka plutonic complex (Morocco). Contrib. Mineral. Petrol. 116, 316–326. doi:10.1007/bf00306500
Fernández, C., and Castro, A. (2018). Mechanical and structural consequences of magma differentiation at ascent conduits: a possible origin for some mafic microgranular enclaves in granites. Lithos 320–321, 49–61. doi:10.1016/j.lithos.2018.09.004
Frank, W., Thoni, M., and Purtscheller, F. (1977). Geology and petrography of Kullu-south Lahul area. Sci. Terre Himal. CNRS 268, 147–160.
Frost, T. P., and Mahood, G. A. (1987). Field, chemical, and physical constraints on mafic-felsic magma interaction in the Lamarck Granodiorite, Sierra Nevada, California. Geol. Soc. Am. Bull. 99, 272–291. doi:10.1130/0016-7606(1987)99<272:fcapco>2.0.co;2
Furman, T., and Spera, F. J. (1985). Co-mingling of acid and basic magma with implications for the origin of mafic I-type xenoliths: Field and petrochemical relations of an unusual dike complex at eagle lake, Sequoia National Park, California, U.S.A. J. Volcanol. Geoth. Res. 24, 151–178. doi:10.1016/0377-0273(85)90031-9
Gansser, A. (1977). The great suture zone between Himalaya and Tibet: a preliminary account. Sci. Terre Himal. CNRS. 268, 181–192.
Güraslan, I. N., and Altunkaynak, Ş. (2019). Role of mantle and lower continental crust in the genesis of Eocene post-collisional granitoids: insights from the Topuk pluton (NW Turkey). J. Asian Earth Sci. 179, 365–384. doi:10.1016/j.jseaes.2019.05.012
Hibbard, M. J. (1981). The magma mixing origin of mantled feldspars. Contrib. Mineral. Petrol. 76, 158–170. doi:10.1007/bf00371956
Holden, P., Halliday, A. N., and Stephens, W. E. (1987). Neodymium and strontium isotope content of microdiorite enclaves points to mantle input to granitoid production. Nature. 330, 53–56. doi:10.1038/330053a0
Honegger, K., Dietrich, V., Frank, W., Gansser, A., Thöni, M., and Trommsdorff, V. (1982). Magmatism and metamorphism in the Ladakh Himalayas (the Indus-Tsangpo suture zone). Earth Planet Sci. Lett. 60, 253–292. doi:10.1016/0012-821x(82)90007-3
Huang, X.-D., Lu, J.-J., Sizaret, S., Wang, R.-C., Wu, J.-W., and Ma, D.-S. (2018). Reworked restite enclave: Petrographic and mineralogical constraints from the Tongshanling intrusion, Nanling Range, South China. J. Asian Earth Sci. 166, 1–18. doi:10.1016/j.jseaes.2018.07.001
Huppert, H. E., Stephen, R., Sparks, J., and Turner, J. S. (1984). Some effects of viscosity on the dynamics of replenished magma chambers. J. Geophys. Res. 89, 6857–6877. doi:10.1029/jb089ib08p06857
Jain, A. K., and Singh, S. (2009). Geology and tectonics of the southeastern Ladakh and karakoram. Bangalore: Geological Society of India.
Jain, A. K., Singh, S., Manickavasagam, R. M., Joshi, M., and Verma, P. K. (2003). Himprobe programme: integrated studies on geology, petrology, geochronology and geophysics of the Trans-Himalaya and Karakoram. Mem. Geol. Soc. India. 53, 1–56.
Janoušek, V., Bowes, D. R., Braithwaite, C. J. R., and Rogers, G. (2000). Microstructural and mineralogical evidence for limited involvement of magma mixing in the petrogenesis of Hercynian high-K calc-alkaline intrusion: the Kozárovice granodiorite, Central Bohemian pluton, Czech Republic. Earth Environ. Sci. Trans. R. Soc. Edinburgh. 91, 15–26. doi:10.1017/S0263593300007264
Kumar, S. (1995). Microstructural evidence of magma quenching inferred from enclaves hosted in the Hodruša Granodiorites, Western Carpathians. Geol. Carpath. 46, 379–382.
Kumar, S. (2008). Magnetic susceptibility mapping of Ladakh granitoids, northwest higher Himalaya: implication to redox series of felsic magmatism in the subduction environments. Mem. Geol. Soc. India. 72, 83–102.
Kumar, S. (2010a). Mafic to hybrid microgranular enclaves in the Ladakh batholith, northwest Himalaya: implications on calc-alkaline magma chamber processes. J. Geol. Soc. India 76, 5–25. doi:10.1007/s12594-010-0080-2
Kumar, S. (2010b). Magnetite and ilmenite series of granitoids of Ladakh Batholith, Northwest Indian Himalaya: implications on redox conditions of calc-alkaline felsic magma chambers. Curr. Sci. 99, 1260–1264. doi:10.2307/24068524
Kumar, S. (2014). “Magmatic processes: review of some concepts and models,” in Modelling of magmatic and allied processes. Editors S Kumar and R. N. Singh (Cham, Switzerland: Springer-Switzerland), 1–22.
Kumar, S., Bora, S., and Sharma, U. K. (2016). Geological appraisal of Ladakh and Tirit granitoids in the Indus-Shyok Suture Zones of Northwest Himalaya, India. J. Geol. Soc. India. 87, 737–746. doi:10.1007/s12594-016-0446-1
Kumar, S., Pieru, T., Rino, V., and Hayasaka, Y. (2017). Geochemistry and U–Pb SHRIMP zircon geochronology of microgranular enclaves and host granitoids from South Khasi Hills of the Meghalaya Plateau, NE India: evidence of synchronous mafic–felsic magma mixing–fractionation and diffusion in a post-collision tectonic environment during the Pan-African orogenic cycle. Geol. Soc. Lond. Spec. Publ. 457, 253–289. doi:10.1144/SP457.10
Kumar, S., Pieru, T., Rino, V., and Lyngdoh, B. C. (2005). Microgranular enclaves in Neoproterozoic granitoids of south Khasi Hills, Meghalaya plateau, Northeast India: field evidences of interacting coeval mafic and felsic magmas. J. Geol. Soc. India. 65, 629–633.
Kumar, S., and Rino, V. (2006). Mineralogy and geochemistry of microgranular enclaves in Palaeoproterozoic Malanjkhand granitoids, central India: evidence of magma mixing, mingling, and chemical equilibration. Contrib. Mineral. Petrol. 152, 591–609. doi:10.1007/s00410-006-0122-3
Kumar, S., Rino, V., and Pal, A. B. (2004a). Field evidence of magma mixing from microgranular enclaves hosted in Palaeoproterozoic Malanjkhand granitoids, central India. Gondwana Res. 7, 539–548. doi:10.1016/s1342-937x(05)70804-2
Kumar, S., Rino, V., and Pal, A. B. (2004b). Typology and geochemistry of microgranular enclaves hosted in Malanjkhand granitoids, Central India. J. Geol. Soc. India. 64, 277–292.
Kumar, S., and Singh, B. (2008). Mineralogy and Geochemistry of mafic to hybrid microgranular enclaves and felsic host of Ladakh batholith, Northwest Himalaya: Evidence of multistage complex magmatic processes. Himal. J. Sci. 5, 130–131. doi:10.3126/hjs.v5i7.1315
Kumar, S., Singh, B., Wu, F. Y., and Ji, W. Q. (2012). Timing of Asia–India collision evident from U–Pb–Lu–Hf isotopes of zircons from granitoids and enclaves of Ladakh Batholiths, NW Himalaya. J. Nepal Geol. Soc. 45, 164–165.
Lacroix, A. (1890). Sur les enclaves du trachyte de Menet (Cantal), sur leurs modifications et leur origin. Paris: C R Academy of Science, 11, 1003–1006.
Le Maitre, R. W. (2002). “A classification and glossary of terms,” in Recommendations of the IUGS Subcommission on the systematics of igneous rocks. 2nd Edn. Cambridge: Cambridge University Press, 236.
Lu, Y. H., Gao, P., Zhao, Z. F., and Zheng, Y. F. (2020). Whole-rock geochemical and zircon Hf–O isotopic constraints on the origin of granitoids and their mafic enclaves from the Triassic Mishuling pluton in West Qinling, central China. J. Asian Earth Sci. 189, 104136. doi:10.1016/j.jseaes.2019.104136
Mariano, G., and Sial, A. C. (1988). Evidence of magma mixing in the Itaporanga Batholith, NE Brazil. Rec. Soc. Ital. Mineral. Petrolo. 43, 555–568.
McBirney, A. R. (1985). Further considerations of double-diffusive stratification and layering in the Skaergaard Intrusion. J. Petrol. 26, 993–1001. doi:10.1093/petrology/26.4.993
Montagna, C. P., Papale, P., and Longo, A. (2015). “Timescales of mingling in shallow magmatic reservoirs,” in Chemical, physical and temporal evolution of magmatic systems. Editors L Caricchi and J. D. Blundy (London, UK: Geological Society, London, Special Publications), Vol. 422.
Pabst, A. (1928). Observation on inclusions in the granitic rocks of the Sierra Nevada. California, United States: University of California Geological Sciences, 17, 325–388.
Perugini, D., Poli, G., Christofides, G., and Eleftheriadis, G. (2003). Magma mixing in the Sithonia Plutonic Complex, Greece: evidence from mafic microgranular enclaves. Mineral. Petrol. 78, 173–200. doi:10.1007/s00710-002-0225-0
Phillips, G. N., Wall, V. J., and Clemens, J. D. (1981). Petrology of the Strathbogie Batholith: a cordierite-bearing granite. Can. Mineral. 19, 47–63.
Pin, C., Binon, M., Belin, J. M., Barbarin, B., and Clemens, J. D. (1990). Origin of microgranular enclaves in granitoids: equivocal Sr‐Nd evidence from Hercynian rocks in the Massif Central (France). J. Geophys. Res. 95, 17821–17828. doi:10.1029/JB095iB11p17821
Pundir, S., Adlakha, V., Kumar, S., and Singhal, S. (2020). Closure of India–Asia collision margin along the Shyok suture zone in the eastern Karakoram: new geochemical and zircon U–Pb geochronological observations. Geol. Mag. 157, 1451–1472. doi:10.1017/s0016756819001547.
Rai, H. (1980). Origin and emplacement of Ladakh Batholith: present day knowledge. Himal. Geol. 10, 78–93.
Ravikant, V., Wu, F. Y., and Ji, W. Q. (2009). Zircon U–Pb and Hf isotopic constraints on petrogenesis of the Cretaceous–Tertiary granites in eastern Karakoram and Ladakh, India. Lithos. 110, 153–166. doi:10.1016/j.lithos.2008.12.013
Reid, J. B., Jr., Evans, O. C., and Fates, D. G. (1983). Magma-mixing in granitic rocks of the Central Sierra Nevada, California. Earth Planet Sci. Lett. 66, 243–261. doi:10.1016/0012-821X(83)90139-5
Reid, J. B., Jr., and Hamilton, M. A. (1987). Origin of Sierra Nevada granite: evidence from small scale composite dikes. Contrib. Mineral. Petrol. 96, 441–454.
Rooyakkers, S. M., Wilson, C. J. N., Schipper, C. I., Barker, S. J., and Allan, A. S. R. (2018). Textural and micro-analytical insight into mafic-felsic interactions during the Oruanui eruption, Contrib. Mineral. Petrol. 173, 35. doi: 10.1007/s00410-018-1461-6.
Sharma, K. K., and Choubey, V. M. (1983). “Petrology, geochemistry, and geochronology of the southern margin of the Ladakh Batholith between Upshi and Chumathang,” in Geology of Indus suture zone of Ladakh. Editors V. C. Thakur and K. K. Sharma (Dehradun: Wadia Institute of Himalayan Geology), Vol. 240, 41–60.
Shellnutt, J. G., Jahn, B.-M., and Dostal, J. (2010). Elemental and Sr-Nd isotopes geochemistry of microgranular enclaves from peralkaline A-type granite plutons of the Emeishan large igneous province, SW China. Lithos. 119, 34–46. doi:10.1016/j.lithos.2010.07.011
Shellnutt, J. G., Lee, T.-Y., Brookfield, M. E., and Chung, S.-L. (2013). Correlation between magmatism of the Ladakh Batholith and plate convergence rates during the India–Eurasia collision. Gondwana Res. 26, 1051–1059. doi:10.1016/j.gr.2013.09.006
Singh, S., Kumar, R., Barley, M. E., and Jain, A. K. (2007). SHRIMP U-Pb ages and depth of emplacement of Ladakh Batholith, Eastern Ladakh, India. J. Asian Earth Sci. 30, 490–503. doi:10.1016/j.jseaes.2006.12.003
Sollas, J. W. (1894). On the volcanic district of Calingford and Slieve Gullion. I: On the relation of the granite to the gabbro of Barnavarve. Trans. R. Irish Acad. 30, 477–512.
Siebel, W., Shang, C. K., Thern, E., Danišík, M., and Rohrmüller, J. (2012). Zircon response to high-grade metamorphism as revealed by U–Pb and cathode-luminescence studies. Int. J. Earth Sci. 101, 2105–2123. doi:10.1007/s00531-012-0772-5
Sparks, R. J. S., and Marshall, L. A. (1986). Thermal and mechanical constraints on mixing between mafic and silicic magmas. J. Volcanol. Geoth. Res. 29, 99–124. doi:10.1016/0377-0273(86)90041-7
Sparks, R. J. S., Sigurdson, H., and Wilson, L. (1977). Magma mixing: a mechanism for triggering acid explosive eruptions. Nature. 267, 6337–6344. doi:10.1038/267315a0
Thakur, V. C. (1987). Plate tectonic interpretation of the western Himalaya. Tectonophysics. 134, 91–102. doi:10.1016/0040-1951(87)90251-4
Upadhyay, R., Frisch, W., and Siebel, W. (2008). Tectonic implication of U-Pb zircon ages of the Ladakh Batholith, Indus suture zone, northwest Himalaya, India. Terra. Nova. 20, 309–317. doi:10.1111%2Fj.1365-3121.2008.00822.x
Vernon, R. H. (1983). “Restite, xenoliths and microgranitoid enclaves in granites,” in Clarke memorial lecture. NSW: Journal Proceeding Royal Society, Vol. 116, 77–103.
Vernon, R. H. (1984). Microgranitoid enclaves in granites-globules of hybrid magma quenched in a plutonic environment. Nature. 309 (5967), 438–439. doi:10.1038/309438a0
Vernon, R. H. (2014). Microstructures of microgranitoid enclaves and the origin of S-type granitoids. Aust. J. Earth Sci. 61, 227–239. doi:10.1080/08120099.2014.886623
Vernon, R. H., Etheridge, M. A., and Wall, V. J. (1988). Shape and microstructures of microgranitoid enclaves: indicators of magma mingling and flow. Lithos. 22, 1–11. doi:10.1016/0024-4937(88)90024-2
Wadia, D. N. (1937). The Cretaceous volcanic series of Astor-Deosai Kashmir and is intrusion. Record Geol. Surv. India. 72, 151–161.
Weinberg, R. F. (1997). The disruption of a diorite magma pool by intruding granite: the Sobu body, Ladakh Batholith, Indian Himalayas. J. Geol. 105, 87–98. doi:10.1086/606149
Weinberg, R. F., and Dunlop, W. J. (2000). Growth and deformation of the Ladakh Batholith, northwest Himalayas: implications for timing of continental collision and origin of calc-alkaline Batholith. J. Geol. 108, 303–320. doi:10.1086/314405
White, L. T., Ahmad, T., Ireland, T. R., Lister, G. S., and Forster, M. S. (2011a). Deconvolving episodic age spectra from zircons of the Ladakh Batholith, northwest Indian Himalaya. Chem. Geol. 289, 179–196. doi:10.1016/j.chemgeo.2011.07.024
White, L. T., Ahmad, T., Lister, G. S., and Ireland, T. R. (2011b). Where does Indian plate and the Eurasian plate begin? Geol. Geochem. Geophys. 12 (Q10013), 6–201. doi:10.1029/2011GC003726
Wiebe, R. A. (1980). Comingling of contrasted magmas in the plutonic environment: examples from the Nain anorthositic complex. J. Geol. 88, 197–209. doi:10.1086/628491
Wiebe, R. A. (1994). Silicic magma chambers a trap for basaltic magmas: The Cadillac mountain intrusive complex, Mount Desert island, Maine. J. Geol. 102, 423–427. doi:10.1086/629684
Wiebe, R. A., Jellinek, M., Markley, M. J., Hawkins, D. P., and Snyder, D. (2007). Steep schlieren and associated enclaves in the Vinalhaven granite, Maine: possible indicators for granite rheology. Contrib. Mineral. Petrol. 153, 121–138. doi:10.1007/S00410-006-0142-Z
Wiebe, R. A., Manon, M. R., Hawkins, D. P., and McDonough, W. F. (2004). Late-stage mafic injection and thermal rejuvenation of the Vinalhaven granite, Coastal Maine. J. Petrol. 45, 2133–2153. doi:10.1093/petrology/egh050
Wiebe, R. A., Smith, D., Sturn, M., King, E. M., and Seckler, M. S. (1997). Enclaves in the Cadillac mountain granite (Coastal Maine): samples of hybrid magma from the base of the chamber. J. Petrol. 38, 393–426. doi:10.1093/petroj/38.3.393
Xiao, Y., Chen, S., Niu, Y., Wang, X., Xue, Q., Wang, G., et al. (Forthcoming 2020). Mineral compositions of syn-collisional granitoids and their implications for formation of juvenile continental crust and adakitic magmatism. J. Petrol.
Zhang, Y-J., Ma, C-Q., Zhang, C., and Li, J.-W. (2014). Fractional crystallization and magma mixing: evidence from porphyritic diorite-granodiorite dyke and mafic microgranular enclaves within the Zhoukoudian pluton, Beijing. Mineral. Petrol. 108, 777–800. doi:10.1007/s00710-014-0336-4.
Zhang, J., Wang, T., Castro, A., Zhang, L., Shi, X., Tong, Y., et al. (2016). Multiple mixing and hybridization from magma source to final emplacement in the Permian Yamatu pluton, the Northern Alexa Block. China. J. Petrol. 57, 933–980. doi:10.1093/PETROLOGY/EGW028
Keywords: enclaves, synplutonic dykes, granites, Ladakh Batholith, Trans-Himalaya, India
Citation: Kumar S (2020) Schedule of Mafic to Hybrid Magma Injections Into Crystallizing Felsic Magma Chambers and Resultant Geometry of Enclaves in Granites: New Field and Petrographic Observations From Ladakh Batholith, Trans-Himalaya, India. Front. Earth Sci. 8:551097. doi:10.3389/feart.2020.551097
Received: 11 April 2020; Accepted: 01 September 2020;
Published: 01 October 2020.
Edited by:
J. Gregory Shellnutt, National Taiwan Normal University, TaiwanReviewed by:
Koushik Sen, Wadia Institute of Himalayan Geology, IndiaFatemeh Sarjoughian, University of Kurdistan, Iran
Copyright © 2020 Kumar. This is an open-access article distributed under the terms of the Creative Commons Attribution License (CC BY). The use, distribution or reproduction in other forums is permitted, provided the original author(s) and the copyright owner(s) are credited and that the original publication in this journal is cited, in accordance with accepted academic practice. No use, distribution or reproduction is permitted which does not comply with these terms.
*Correspondence: Santosh Kumar, c2t5YWRhdmFuQHlhaG9vLmNvbQ==