- 1Biological Station of Hiddensee, University of Greifswald, Greifswald, Germany
- 2Biological Station Zingst, University Rostock, Rostock, Germany
Baltic coastal lagoons are severely threatened by eutrophication. To evaluate the impact of eutrophication on macrophytobenthos, we compared the seasonal development in macrophytobenthic composition, biomass and production, water column parameters (light, nutrients), phytoplankton biomass and production in one mesotrophic and one eutrophic German coastal lagoon. We hypothesized that light availability is the main driver for primary production, and that net primary production is lower at a higher eutrophication level. In the mesotrophic lagoon, macrophytobenthic biomass was much higher with distinct seasonal succession in species composition. Filamentous algae dominated in spring and late summer and probably caused reduced macrophytobenthic biomass and growth during early summer, thus decreasing vegetation stability. Light attenuation was far higher in the eutrophic lagoon, due to high phytoplankton densities, explaining the low macrophytobenthic biomass and species diversity in every season. Areal net primary production was far lower in the eutrophic lagoon. The “paradox of enrichment” hypothesis predicts lower production at higher trophic levels with increased nutrient concentrations. Our results prove for the first time that this hypothesis may be valid already at the primary producer level in coastal lagoons.
Introduction
At the southern Baltic Sea coast, an intricate system of coastal lagoons connects terrestrial ecosystems with the open Baltic Sea, acting as a filter for nutrients and dissolved organic substances originating from their catchment areas. These lagoons differ in their connectivity with the open sea and size of there catchment areas, whichinfluence the lagoons ecosystem response to eutrophication (Cloern, 2001). Lagoons of the Baltic Sea can be classified either as estuarine, with direct river discharge, or marine, without direct river discharge (sensu Tagliapietra et al., 2009). Especially in estuarine lagoons of the southern Baltic Sea, high nutrient supplies from their catchment area have accelerated pelagic primary production and caused high water turbidity and a massive reduction of the macrophytobenthos (Schiewer, 1998; Munkes, 2005). The macrophytobenthos has up to now not recovered (Schiewer, 1998; Munkes, 2005) though nutrient discharges have considerably decreased since the 1980ies in accordance with national and European water framework directives (Berthold et al., 2018a), Macrophytobenthos increases the structural and functional complexity of shallow waters including coastal lagoons (e.g. Duffy et al., 2001) and is therefore a key component of shallow aquatic ecosystems. Macrophytobenthos consists of “rooted canopy forming” tracheophyte species, rooted “bottom-dwellers”, loosely attached macroalgae, and filamentous algae growing as epiphyton. The complex structure of the macrophytobenthos can slow down hydrodynamic movements (Gregg and Rose, 1982), store and immobilize nutrients (Pederson and Borum, 1997), elevate sedimentation rates and, thus, redirect resources from the pelagic to the benthic system (Kennedy et al., 2010). Macrophytobenthos improves habitat diversity and fosters a rich and diverse associated fauna by providing structural support, shelter from predation and food to higher trophic levels (Hansen et al., 2011; Wlodarska-Kowalczuk et al., 2014). The influence of the macrophytobenthos on its environment depends strongly on the seasonal development of the vegetation canopy and the length of the growing period (Sayer et al., 2010), which is determined by the number of seasonal strategies and, thus, the species richness of the community.
Generally, the macrophytobenthic growing period starts in spring with the growth of rooted species, often fueled by storage metabolites (Middelboe and Markager, 1997) resulting in a macrophytobenthic biomass maximum in summer (Jankowska et al., 2014). Macrophytobenthic primary production is limited mainly by light (Pärnoja et al., 2014; Piepho, 2017) and nutrient availability (Granéli and Solander, 1988). New growing leaves are substrate for fast growing filamentous algae. Fast growing filamentous algae have the ability to take up 30% of occurring dissolved inorganic phosphorus pulses (Howard-Williams and Allanson, 1981). It leads to high production and growth of this group of macrophytes under elevated nutrient concentrations. Proliferating filamentous algae growing on macrophytes prevent leaf uptake, shade and outcompete their host for carbon and nutrients, resulting in an overall reduced production of the overgrown macrophytes (Madsen and Brix, 1997; Apostolaki et al., 2011).
During progressive eutrophication, light availability decreases in the water column because of increasing phytoplankton densities, causing a breakdown of the macrophytobenthos at a certain critical turbidity and a sudden shift to an alternate, phytoplankton-dominated turbid state. The existence of such alternative stable states has not only been shown for freshwater lakes (Scheffer et al., 1993), but also been assumed for brackish water ecosystems such as Baltic Sea coastal lagoons. In a eutrophication gradient in coastal waters of the Baltic Sea (Dahlgren and Kautsky, 2004), observed a change from slow-growing rooted macrophytes to filamentous sheet-like, fast growing species to phytoplankton dominance, similar to changes in freshwater ecosystem, where tall macrophytes with a short vegetation period form an instable “crashing” state between an stable clearwater and a stable turbid state (Sayer et al., 2010).
High self-shading within the phytoplankton assemblage decreases the euphotic zone and phytoplankton gross primary production, and can explain why nutrient enrichment does not further increase pelagic NPP in coastal ecosystems, once a certain threshold is exceeded (Oviatt et al., 1986; Schiewer, 1998). In lakes, higher pelagic NPP has consequently been found under lower nutrient concentrations. Higher total system primary production at lower nutrient and higher light conditions is further explained by the fact that submerged vegetation, together with its epiphyton, is highly productive and the main contributor to total production under these conditions (Lopez-Archilla et al., 1992; Blindow et al., 2006). This pattern supports the “paradox of enrichment” hypothesis originally described by Rosenzweig (1971), which predicts lower production with increasing nutrient availability. While Rosenzweig (1971) described this decrease in production for higher trophic levels due to increasingly instable predator-prey relationships, a corresponding decrease has thus been observed in shallow lakes already evident on the primary production level. For coastal lagoons, Schiewer (1998) and Kemp et al. (2001) assumed lower production of higher trophic levels after eutrophication, caused by reduced trophic efficiencies, but empirical evidence is scarce. Also for coastal lagoons, we assume that a paradox of enrichment already may occur at the primary producer level.
Here, we compare the seasonal development in macrophytobenthic species composition, biomass and net primary production (NPP) in two lagoons of the southern Baltic Sea under different eutrophication pressures: the mesotrophic marine Westrügensche lagoon, and the outermost part of the eutrophic estuarine Darß-Zingst lagoon system. We discuss the seasonal variation of the macrophytobenthos in relation to pelagic NPP, underwater light climate, nutrients and lagoon system attributes. We predict that one-the underwater light availability is the main driver of seasonal development of the macrophytobenthic community in the mesotrophic lagoon two-the macrophytobenthic species numbers are lower, the vegetation period is shorter and the NPP reduced in the eutrophic lagoon and three-the total areal NPP is lower in the eutrophic than in the mesotrophic lagoon. Increased knowledge of drivers and mechanisms of the macrophytobenthos and the changes affecting this community during eutrophication is essential to improve management strategies for the recovery the macrophytobenthos in lagoons of the southern Baltic Sea.
Materials and Methods
Investigation Areas
The mesotrophic, marine Westrügensche lagoon is approximately 170 km2 with an average depth of 1.9 m. It is connected to the Baltic Sea in the northeast and in the south, resulting in a strong water exchange. Its catchment area is relatively small, and only a slight increase in nutrient concentrations was detected in the 1980–1990 (Blindow and Meyer, 2015). The extension and cover of the macrophytobenthos did not change over the last 90 years, but changes in macrophytobenthic species composition and vegetation architecture were observed in the last decades (Blindow et al., 2016). In the Westrügensche lagoon, the samples were taken in the Vitter Bodden, between the island of Rügen and Hiddensee (Figure 1). The shallow coast south of Kloster in the north of Hiddensee was chosen as the sampling location at 54°34.810′N and 13°6.913′E. Here, the macrophytobenthos density increases with depth, from 1 m down to 2.8 m water (the maximum depth of the Vitter Bodden), where it reaches up to 70% coverage. At water depths below 1 m, Ruppia spp. dominates the vegetation with patches of Chara spp. Between one and 1.5 m water depths, Stuckenia pectinata is dominating, and Fucus vesiculosus can build extensive patches. Zostera marina dominates below 1.5 m (Blindow et al., 2016; Bühler, 2016).
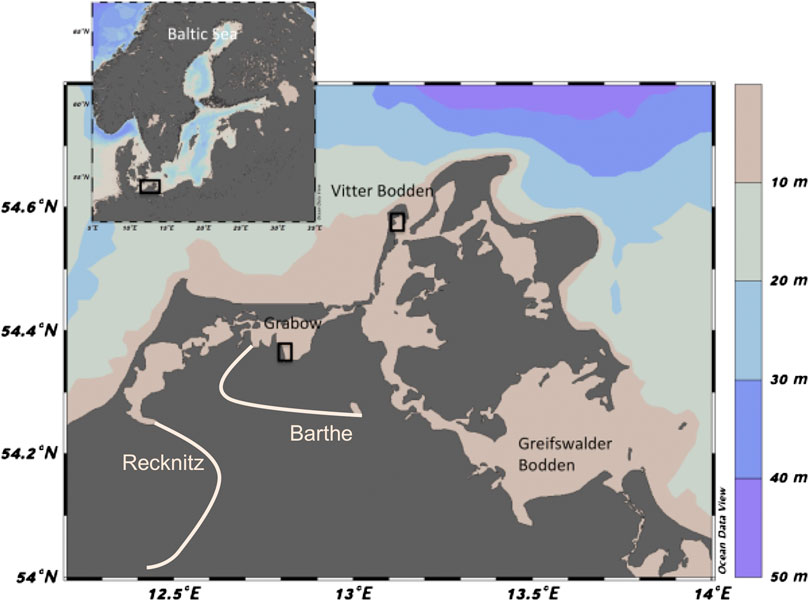
FIGURE 1. Map of sampling sites at Grabow (Darß-Zingst Bodden chain) and Vitter Bodden (Westrügensche Bodden), southern coast of the Baltic Sea. Colors indicate water depths. White lines denote main riverine inflow of the rivers Recknitz and Barthe in this area. Maps were created with Ocean Data View (Schlitzer, 2018).
The eutrophic, estuarine Darß-Zingst Bodden Chain (DZBC) consists of four linked lagoons, which receive different freshwater (and nutrient) influxes, have different nutrient cycling, planktonic community structure and NPP. This lagoon system has been monitored since 1969 with eutrophication documented until the middle of the 1990s (Schiewer, 2007). The DZBC shows almost no recovery from eutrophication over the last 30 years. Freshwater and nutrients are flowing into the DZBC from two rivers, the Recknitz and the Barthe (Figure 1). Here, we focus on the outermost lagoon of the DZBC, the Grabow, which represents 21% of the total area of the four lagoons (Figure 1, Correns, 1976). The Grabow is the only lagoon of the system with a direct connection to the Baltic Sea. The total nitrogen (TN) and total phosphorus (TP) concentrations in the Grabow (100 and 2 mol L−1, respectively) are about 50% lower than the innermost part of the DZBC (Berthold et al., 2018a). The shallow coast east of Dabitz was chosen as the sampling location (54°21.976′N and 12°48.418′E), where the macrophytobenthos reaches high cover down to water depths of 1.2 m and is dominated by S. pectinata (Blindow and Meyer, 2015).
Water Column Parameters
Physical Parameters and Nutrients, Pigment and Seston Concentrations
The Vitter Bodden was monitored biweekly from March 2017 to april 2018. The Grabow was monitored once a month at a central cite (Buoy B53, 54°23.483′N, 12°51.146′E). Temperature and salinity were measured using a WTW Cond 1970i, probe Tetracon 325. Secchi depth was additionally determined. Water samples for determination of nutrients, pigments and seston were taken using a 1.5 m long acrylic glass tube with a diameter of 10 cm to ensure sampling of the entire water column in Vitter Bodden. A Limnos water sampler was used to sample surface water in Grabow. Here, complete mixing of water column can be assumed for most days of the year (Schubert and Foster, 1997).
Seston concentrations were determined after filtering a known volume of water through pre-weighed GF/F (Vitter Bodden, Co. Whatman) and GF6 filters (Grabow, Co. Whatman), which were dried at 60°C until constant weight was achieved. GF6 filters were used to prevent clogging of the filter by a high cyanobacterial biomass with large mucuous envelopes (Schumann et al., 2001). In an investigation performed during 2014, about 1.2 μg L−1 Chl a were lost through GF6-filters (mean value, n = 21), equivalent to 2.6% of the Chl a concentrations obtained after GF/F-filtration (unpublished results).
For pigment analyses, water samples were filtered on same types of filters in triplicates and stored frozen. Pigments were extracted with 96% ethanol and analyzed spectrophotometrically (HELCOM 2014; LUNG SOP-Nr: 640-Chlorophyll-KG). Chlorophyll a values were converted into biomass assuming a C:Chl a−1 weight ratio of 31 (Schumann et al., 2009. Despite the large variation of this ratio (10–130) in coastal waters the annual average was choosen to allow comparison between studied lagoons. Subsamples for nutrient analyses were frozen immediately after sampling for total nutrients and after filtration through cellulose acetate (0.45 µm) for dissolved nutrients. TP, TN, dissolved inorganic phosphorus (DIP) and dissolved inorganic nitrogen (DIN) were analyzed at the Biological Station Zingst (University of Rostock, Germany). TP and TN (10–15 ml per analysis) were digested with an adapted alkaline persulphate procedure (Huang and Zhang, 2009; Berthold et al., 2015) to phosphate and nitrate, respectively. A continuous flow analyser (Alliance Instruments, 5 cm cuvette) was used to determine TP and DIP (Murphy and Riley, 1962; Malcolm-Lawes and Wong, 1990). The determination limit was 0.05 μmol L−1 and the combined standard uncertainty 4.2% for DIP as well as 0.22 μmol L−1 and 8.7% for TP. Nitrate was measured after conversion to nitrite at a cadmium reductor column and nitrite by the same method without the catalyzed reduction step as an azodye (Hansen and Koroleff, 1999). Nitrate was corrected by nitrite. Samples were diluted for nitrate and TN with ultrapure water (Purelab Flex, Elga) by 2–20 times. The samples were measured in a segmented flow analyser (FlowSys, Alliance Instruments) equipped with a 5 cm cuvette (Armstrong et al., 1967). Determination limit for nitrate was 0.32 μmol L−1 and 3 μmol L−1 for TN. Ammonium was measured as an indophenol blue dye photometrically (Hansen and Koroleff, 1999). Samples had to be diluted by up to five times. The samples were measured in a photometer (Hach, 5 cm cuvette). Determination limit is 0.7 μmol L−1 and the combined standard uncertainty 6.3%.
Underwater Light Climate
The solar irradiance data were provided from the German Meteorological Service measured at Arkona station (St.-Nr. 00183) near the two sampling locations. The solar irradiance was recorded as the sum of solar radiation in J cm−2 s−1 in 10 min intervals and transformed into photosynthetic active radiation (PAR) in μmol m−2 s−1 by using a conversion factor of 2.04 (Meek et al., 1984). Underwater irradiance (PAR0) was calculated from solar irradiance, Sun elevation and water attenuation (Walsby, 1997). The light attenuation coefficient (Kd) is the slope of log PAR0 with increasing water depth. The variation of Kd for both lagoons depends on several turbidity parameters and was calculated employing the formula by Xu et al. (2005):
where Chl a is the chlorophyll a concentration in μg L−1, seston the seston concentration in mg L−1, and salinity. The data for the calculation were taken from one biweekly recording of the parameters in Vitter Bodden and from a monthly monitoring of Grabow. At both locations, measurements of light attenuation at the sampling location (Kpar) were additionally recorded with three underwater quantum sensors (LI-192, LICOR Biosciences, Lincoln, United States) attached to a bar at intervals of 50 cm using a LI-1400 (LICOR Biosciences, Lincoln, United States) during the macrophytobenthic biomass determination in the lagoons (see below). Measurements were taken for 5 min every second at mid day during each of the sampling occasions. If available, measured Kpar was used for estimating PARz above the macrophytobenthic canopy.
The underwater irradiance above the macrophytobenthic canopy at 0.75 m water depth (PARz) was derived from the underwater irradiance (PAR0) by applying the Lambert-Beer law:
The self-shading of macrophytobenthos was taken into consideration by estimating the available irradiance within the macrophytobenthic canopy in μmol m−2 s−1 using the equation of Cerco and Moore (2001):
where PARz is the irradiance above the macrophytobenthic canopy at 0.75 m water depth, Kmc the attenuation by the macrophytobenthos (Cerco and Moore, 2001), and MPB the total macrophytobenthic dry mass in mg C m−2.
Biomass and Species Composition of the Macrophytobenthos
In both lagoons, the macrophytobenthos was sampled in 2017 in five replicates in spring (March/April) and winter (November/December) and in 10 replicates in summer (June/July) and late summer (August/September). All replicates were collected randomly at a distance of at least 10 m and within three days during the sampling occasions. The samples were taken at 1 m water depth using a drop trap consisting of a 1.2 m high aluminum frame with a bottom area of 0.25 m2. The trap was lowered from the side of a small boat into the water. The enclosed macrophytobenthos was removed using a metal bow rake. The samples were taken to the laboratory and sorted. The macrophytobenthos was identified to the lowest taxonomical level possible (Table 1). All samples were blotted dry, and wet mass for the entire macrophytobenthos was determined. If filamentous algae were present, a subsample was analyzed for species composition. Afterward, the samples were dried at 60°C until constant weight was reached. Organic carbon content of each sample was determined using an elemental analyser (Flash EA 1112, Thermo Scientific, Milan, Italy) at the LIENSs stable isotope facility of the University of La Rochelle, France (Table 2).
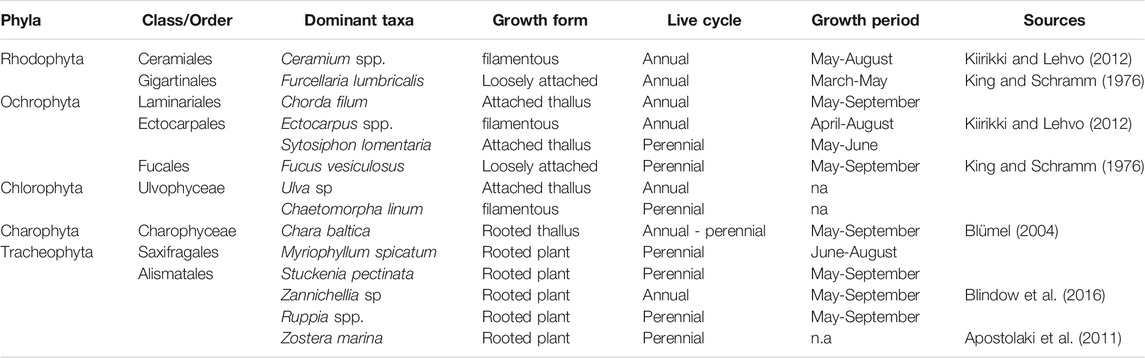
TABLE 1. Complete list of macrophytobenthic species sampled in Vitter Bodden and Grabow from March to December 2017.
Net Primary Production of the Macrophytobenthos
Hourly oxygen evolution rates were calculated of the single macrophytobenthic taxa per gram dry mass (mg O2 g DM−1 h−1) in relation to the available irradiance within the macrophytobenthic canopy. Single species approach was chosen to incorporate seasonal variability in macrophytobenthic species composition, which differ considerably in their photosynthetic parameters. The following steps were repeated for the available irradiance within the macrophytobenthic canopy for each of the seven days during each sampling occasion to access daily variation of hourly oxygen evolution rates. Two different equations were used based on the models given for the single macrophytobenthic taxa in the literature (Table 2). The equation of Walsby (1997) was employed:
where Pmax is the maximum oxygen evolution rate in mg O2 g DM−1 h−1, α the initial slope of the photosynthetic irradiance curve in mg O2 g DM−1 h −1 (µmol photons m−2 s−1)−1, PARmc irradiance within the macrophytobenthic canopy in μmol m−2 s−1, β the factor of photoinhibition and Rd the dark respiration in mg O2 g DM−1 h −1. The second equation employed to calculate hourly oxygen evolution rates of single macrophytobenthic species was taken from Jasby and Platt (1976):
All photosynthetic parameters, either taken from own measurements or from the literature (Table 2), were corrected for temperature. Own photosynthetic parameters were fitted to photosynthesis-irradiance measurements for Ectocarpus sp. and Syctosiphon lomentaria. As described in Piepho (2017), dark adapted apical shoots of plants were incubated in a 2.5 ml cuvette within a photosynthetic light suspension system. Oxygen evolution rates were measured in triplicates at nine light intensities from 13 to 2,000 μmol m−2 s−1 at constant temperature. The seasonal photosynthetic parameters were calculated with the following equation (Prosser, 1961):
where Pmax is the maximum photosynthetic rate in mg O2 g DM−1 h−1 at T1 9°C,
The species-specific hourly productivity (mg C DM−1 h−1) was calculated from positive hourly oxygen evolution rates assuming a photosynthetic quotient of 1.2 during daytime (Oviatt et al., 1986). Negative hourly oxygen evolution rates were transformed into hourly respiration (mg C DM−1 h−1) during night-time assuming a respiration quotient of 1.1 (Oviatt et al., 1986). The species-specific hourly productivity and night-time respiration were multiplied with species-specific DM to calculate the species-specific daytime hourly net primary production (NPP) and respiration (mg C m−2 h−1). Daytime hourly NPP refers to primary production exceeding respiration during hours of daylight. Daily NPP was calculated from the sum of species-specific hourly production and respiration within each sample representing the amount of oxygen produced by each macrophytobenthic species during the hours of sunlight, which is not lost by respiration during the night. The sum of all species-specific daily NPP within a sample was regarded as the total daily macrophytobenthic community NPP. The described steps were repeated for all samples at both locations. For seven days covering each sampling occasion at both lagoons hourly daytime productivity, daytime hourly NPP, daily NPP, and total community daily NPP are reported as sampling occasion averages and were calculated by non-parametric bootstrapping.
Pelagic NPP
The hourly daytime pelagic NPP was measured at both locations in parallel to the quantitative sampling of the macrophytobenthos in 2017. Each of ten bottles was filled with prefiltered ambient water (<55 µm) from the sampling location to remove larger zooplankton. Thus, pelagic NPP consisted of phytoplankton gross primary production and community (bacteria, phytoplankton and microzooplankton) respiration. Four bottles were covered with aluminum foil (dark bottles) and used to determine the respiration. Six bottles (light bottles) were placed pairwise in the water column at the surface, half and total average annual Secchi depth. In Vitter Bodden the light bottles were deployed at 0, 0.8 and 1.6 m water depths, in Grabow at 0, 0.25 and 0.5 m water depths. Oxygen concentrations were measured (HQ40days, LDO, Hach-Lange) before the incubation and in the light bottles after incubation over 4 h (11 am–3 pm). For light bottles, hourly oxygen evolution rates were calculated from concentration differences between before and after incubation divided by the hours of deployment. Pelagic NPP was measured at each location on an average sunny day during the sampling occasion. Significant differences in ambient irradiance between sampling locations were only detected during the spring deployment between lagoons. The dark bottles were kept deployed for 24 h in a closed box within the water before measuring the final oxygen concentration. Percentages of oxygen saturation measured were converted into mg O2 L−1 using solubility values to correct for water salinity and temperature (Benson and Krause, 1984). Water column oxygen evolution rates in light bottles were depth-integrated for a water column of 1 m by extrapolating oxygen evolution rates between deployment depths of light bottles in 1 cm steps. The hourly daytime pelagic NPP (mg C m−2 h−1) was calculated from hourly oxygen evolution rates assuming a photosynthetic quotient of 1.2 (Oviatt et al., 1986).
Statistical Analyses
To assess the uncertainty, error propagation was calculated as the partial derivation of each of the formula used to calculate the underwater light climate and NPP (Supplementary Table S1, Taylor, 1997). Standard errors of solar radiation, attenuation, temperature and biomass were used as input variability in the error propagation.
Water column parameters were compared using Wilcoxon Rank sum test between the lagoons. The same test was used for the comparison between calculated and measured light attenuation. Pearson correlation test was used to analyze the relationship between photosynthetic parameters. Total biomass and production of the macrophytobenthos were compared by a two-way analysis of variance with the independent variables season (March/April, June/July, August/September and November/December) and location (Vitter Bodden, Grabow) followed by a pairwise t-test. To achieve normality and homogeneity of variances, the data were log+1 transformed. Due to their small sample size and lack of normal distribution, the standard error and confidence intervals of the mean values of species-specific biomass and production data were calculated by non-parametric bootstrapping using the R package boot (Davison and Hinkley, 1997; Canty and Ripley, 2017). The non-parametric bootstrapping routine of the confidence intervals of the mean used the biased percentile method (Wang, 2001). We considered the differences significant when 95% confidence intervals of the mean were not overlapping. Seasonal patterns in floral biomass of non-filamentous macroalgal species were examined using canonical correspondence analysis (CCA). Water column parameters included in the CCA were PARmc, the DIN:TP ratio and water column Chl a concentration. Trends in biomass related to environmental gradients were analyzed by canonical permutation tests (number of permutations = 999) using the R package vegan. All statistical analyses were conducted with R version 3.5.3 (R Core Team, 2019).
Results
Water Column Parameters
The water temperature in both lagoons was similar and increased from 4 °C in March to maximum values in July/August (Fig. 2; Supplementary Table S2, S3). The lowest values were recorded in both lagoons in December. The highest water temperature was measured in Vitter Bodden in July reaching 19.8°C, while in Grabow the highest water temperature of 20.0°C was measured in August. The salinity ranged from 8.8 to 9.9 PSU in Vitter Bodden and from 7.8 to 9.5 PSU in Grabow. Seston and Chl a concentration were markedly higher in Grabow. Thus, the average monthly calculated light attenuation (Kd) was significantly lower in Vitter Bodden (n = 24, Z = 3.2, p < 0.01) and showed less variability. The measured attenuation (Kpar) showed the same difference between the lagoons and was in agreement only with the Kd in summer and autumn in both lagoons. Based on measured Kpar, the water depth penetrated by at least 1% of the surface irradiance (i.e. euphotic zone) was on average 60% lower in Grabow than in Vitter Bodden. TP concentrations were about 50% lower in Vitter Bodden than in Grabow in all seasons. Mean TN concentrations were three times higher in Grabow than in Vitter Bodden, while the average DIN:TP ratio was three times higher in Vitter Bodden than in Grabow.
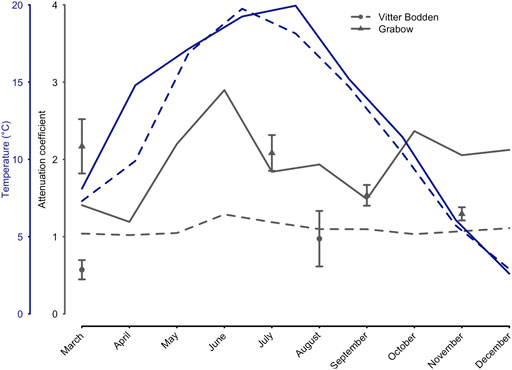
FIGURE 2. Attenuation coefficient and water temperature in 2017. Temperature (°C), salinity, Chlorophyll a (µg L−1) and seston (mg L−1) were monitored biweekly (Vitter Bodden–VB) and monthly (Grabow–GB). Blue dashed (VB), and solid lines (GB) show the temperature development for both sampling locations (March to September). The attenuation coefficient (m−1) was calculated based on Chlorophyll, seston, and salinity (Xu et al., 2005), and additionally measured with Li-Cor sensors simultaneously to macrophyte sampling. Circles (VB) and triangles (GW) show the measured attenuation coefficients during the sampling occasions (mean values + standard deviations).
Seasonal Development of Macrophyte Community Structure and Biomass
Vitter Bodden
The macrophytobenthic total biomass was on average 27 times higher in Vitter Bodden than in Grabow, due to higher biomasses of filamentous algae. Filamentous algae such as Ectocarpus sp., Ceramium sp. and Chaetomorpha linum dominated the vegetation and represented on average 41% of its total biomass throughout the season (Figure 3). The ratio between rooted and non-rooted species was lowest in spring (0.5) and highest in winter (1.0). In spring, Ectocarpus sp. dominated the filamentous biomass representing 78% of its total biomass, while Chaetomorpha linum was the dominant filamentous alga in autumn contributing to 58% to its total biomass. Ceramium spp. represented up to 28% of the filamentous algae biomass in winter. S. pectinata and Ruppia ssp. were the most common tracheophyta and represented together on average 39% of the total floral biomass. Filamentous algae grew mostly as epiphyton on these two tracheophyta.
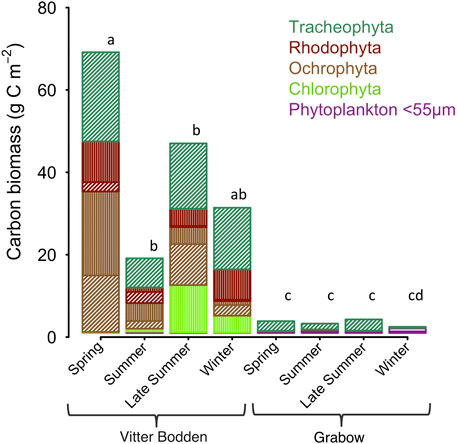
FIGURE 3. Average biomass in carbon (g C m−2) shown for each macrophytobenthic phylum in Vitter Bodden and Grabow. Filamentous macroalgal biomass is highlighted in vertical lines, non-filamentous macrophyte biomass in dashed lines, and phytoplankton in horizontal lines. Letters indicate significant differences between total biomasses (pairwise comparison, F = 3.16, p < 0.05).
Maximum biomasses of filamentous algae, Fucus vesiculosus and S. pectinata were recorded in spring. Biomass composition was highly variable among samples and either dominated by filamentous algae plus tracheophyta or F. vesiculosus plus Furcellaria fastigiata. The macrophytobenthic biomass was lower at the beginning of summer, most pronounced in filamentous algae, which were reduced to 20% of their spring biomass, while tracheophyta were reduced to 33% of their spring biomass (Table 3). Species numbers increased from 10 to 14 from spring to summer. At the end of the summer, macrophyte biomasses recovered, and tracheophyta reached 70% of their spring biomass. The biomass of tracheophyta in November was about the same as in August, while filamentous algae and some phaeophytes had lost biomass.
Grabow
The total macrophytobenthic biomass was significantly lower in Grabow than in Vitter Bodden at each sampling occasion (df = 3, F = 3.16, p < 0.05). The most significant difference of total macrophytobenthic biomass between the lagoons was observed in spring (p < 0.001). Apart from some drifting Zostera marina specimens, only four macrophytobenthic species were found (Table 3). S. pectinata dominated in all seasons representing on average more than 98% of the total biomass (Table 3). As observed in Vitter Bodden, the average total macrophytobenthic biomass in Grabow was reduced to 1.5 g C m−2 at the beginning of summer, and tracheophyta lost 39% of the biomass present in spring. The species number decreased to two from spring to summer. In September, macrophyte biomass recovered and tracheophyta reached their maximum biomass (2.8 g C m−2). In winter, the macrophytobenthos was lowest (0.3 g Cm−2).
Drivers of the Seasonal Development
The seasonal changes in macrophytobenthic community structure in relation to water column parameters were visualized by multivariate correspondence analysis (Figure 4). Along the first axis, only samples from Vitter Bodden were separated. Sample separation reflected the differences in biomass contribution to the total macrophytobenthos community between species with an early and late growth period. In spring, the relative biomass contributions of Myriophyllum spicatum, Enteromorpha sp., and Ectocarpus sp. were the highest. Ruppia spp, C. linum and Chorda filum were observed in the samples from June onwards, corresponding with highest available irradiance within the macrophytobenthic canopy. This seasonal change in species composition was correlated to DIN:TP ratio, which decreased over the season in Vitter Bodden. The second axis refers to the dominance of S. pectinata in the biomass composition of samples. At the positive end of the second axis all samples from Grabow are grouped and clearly separated from all Vitter Bodden samples. The positive end of the second axis was characterized by the lowest available irradiance within the macrophytobenthic canopy but the highest Chl a concentration.
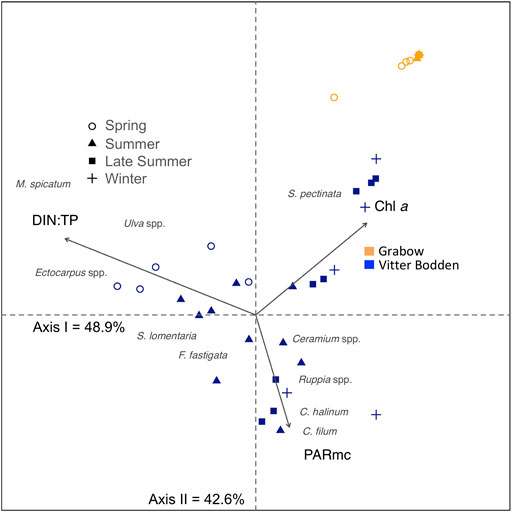
FIGURE 4. Canonical correspondence analysis plot for the macrophytobenthic biomass sampled in spring (March/April, circle), summer (June/July, triangle), late summer (August/September, square), and winter (November/December, cross) in Vitter Bodden (blue) and Grabow (orange). Shown macrophytobenthic species are Ceramium spp, Chaetomorpha linum, Chorda filum, Ectocarpus spp, Furcellaria fastigata, Myriophyllum spicatum, Ruppia spp, Stuckenia pectinata, Sytosiphon lomentaria, Ulva spp.. Environmental factors (arrows) are calculated underwater irradiances within the macrophyte canopy (PARmc), dissolved inorganic nitrogen to total phosphorus ratio (DIN:TP), and Chlorophyll a concentration (Chl a). Positions of macrophytes show their probable occurrence based on the explanatory variables (PARmc, DIN:TP, Chl a). Only environmental factors significantly correlated to the ordination (p < 0.01) are shown.
Photosynthetic Parameters of Dominating Macrophyte Species
Overall there was a strong negative correlation between maximum oxygen evolution and respiration rates (p < 0.01, R2 = 0.87). The highest variability of photosynthetic parameters was found within the attached macroalgae (Table 2), with the highest maximum oxygen evolution and respiration rates in Ulva sp. and the lowest in Fucus vesiculosus. The highest maximum oxygen evolution and respiration rates of filamentous algae were found in Chaetomorpha linum, while the lowest values of these two parameters were found for Ectocarpus sp. Tracheophyta showed the lowest variability in maximum oxygen evolution rates with the lowest values in Zannichellia sp. and the highest in M. spicatum. The initial slope of the photosynthesis-irradiance curve was the highest in Ulva sp. and the lowest in Zannichellia sp. The Q10 coefficient for the maximum oxygen evolution rate was the lowest in Furcellaria fastigata and the highest in Chara baltica. On average, tracheophyta had a considerably higher Q10 than all other macrophytobenthos.
Seasonal Development of Macrophytobenthic Production
The mean macrophytobenthic hourly daytime NPP was 3.5 mg C m−2 h−1 in Grabow compared to 383.3 mg C m−2 h−1 in Vitter Bodden (Figure 5). In Vitter Bodden, the average hourly daytime NPP of filamentous algae was highest in March and lowest in June with highest variability in August (Figure 5). The average daytime hourly NPP of tracheophyta and macroalgae increased between March and August in Vitter Bodden. For each individual species, average hourly daytime productivity was similar in Grabow and Vitter Bodden except for S. pectinata that had significantly lower values in September in Grabow (Table 4).
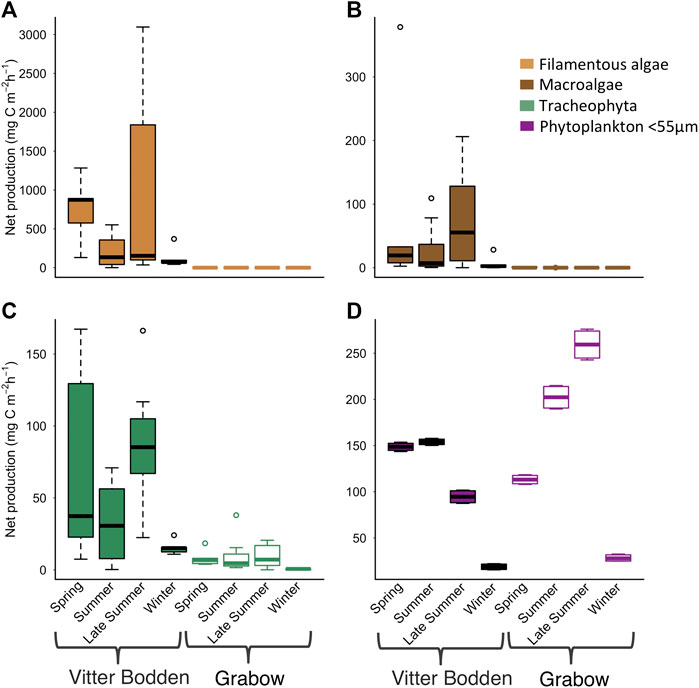
FIGURE 5. Average hourly daytime primary production for (A) filamentous algae (B) macroalgae (C) tracheophyte (D) phytoplankton in Vitter Bodden (filled) and Grabow (open) at sampling occasions. Phytoplankton production was experimentally determined by oxygen evolution in light and dark bottles. The benthic production from filamentous algae, tracheophyte and macrophytes was calculated using species specific photosynthesis parameters from the literature combined with own biomass data. Note the different scales.
In Vitter Bodden, all macrophytobenthos groups had a positive daily NPP from spring to autumn. The mean value of daily macrophytobenthos NPP was about two orders of magnitudes lower in Grabow (39.2 mg C m−2 d−1; range based on error propagation: 25.7 and 52.8 mg C m−2 d−1) than in Vitter Bodden (4.1 g C m−2 d−1; range based on error propagation: 2.9–5.2 g C m−2 d−1; Figure 6; Supplementary Table S3). In Vitter Bodden, filamentous algae were the main primary producers contributing to 83% of the total community daily NPP (mean of all sample occasions), followed by tracheophyta (11%) and macroalgae (6%). In Grabow, S. pectinata was the dominant primary producer representing about 98% of the mean annual daily community NPP. In Grabow, only tracheophyta and macroalgae had a positive daily NPP from april until September.
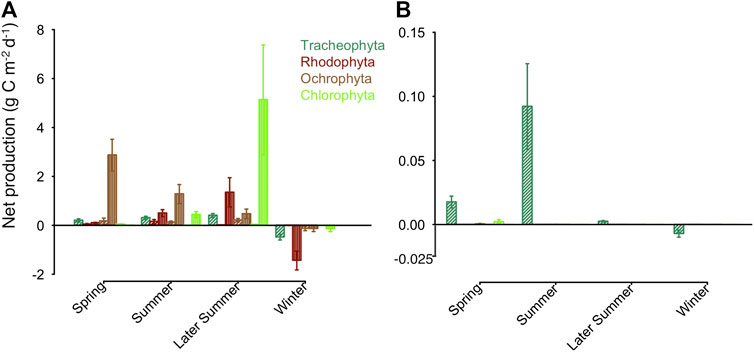
FIGURE 6. Average daily net primary production for each macrophytobenthic phylum in (A) in g Cm−2d−1 for Vitter Bodden and (B) in g Cm−2d−1 for Grabow. Filamentous growth is shown in vertical lines, all other growth forms in dashed lines. Bars are representing standard error of the mean.
Seasonal Comparison of Pelagic Producer Biomass and NPP
The mean biomass of pelagic producers was about one order of magnitude lower in Vitter Bodden (0.1 g C m−2) than in Grabow (0.9 g C m−2) (Figure. 3). In Vitter Bodden, the mean pelagic hourly NPP was 104.0 mg C m−2 h−1 with the highest values in March and June and the lowest values in November (Figure 5). Here, the hourly NPP was significantly higher in spring than in late summer. In Vitter Bodden, the hourly macrophytobenthic NPP was higher than the pelagic NPP in spring, equal in summer and five times higher in autumn. The mean total hourly NPP per square meter of both macrophytobenthos and pelagic producers was three times higher in Vitter Bodden than in Grabow.
In Grabow, pelagic producers represented 50% of macrophytobenthic biomass in summer and had four-times higher biomasses than the macrophytobenthos in winter. Mean pelagic hourly production of phytoplankton was 150.8 mg C m−2 h−1 with the highest values in September and the lowest in December. Phytoplankton contributed 98% of total primary production per square meter in comparison to 2% of the macrophytobenthos with highest contributions in summer and lowest in autumn.
Discussion
Seasonal Development of the Macrophytobenthic Community in Vitter Bodden
Macrophytobenthic species composition in Vitter Bodden was similar to earlier observations (Blindow et al., 2016; Meyer et al., 2019). The main growing period of the macrophyte community occurred between March and September with maximum biomass reached in summer.
Species-specific differences in onset and duration of the growing period could be influenced by the specific needs of individual species for light and nutrients. In spring, high values of TN:TP and DIN:TP suggested P limitation with high availability of N, while low values of these ratios suggested N limitation in summer, which is supported by previous studies in Baltic coastal areas (Kronvang et al., 2005; Schumann et al., 2009; Berthold et al., 2018a). The filamentous alga Ectocarpus sp. which usually starts growing at the beginning of the season (Wennberg, 1992), contributed most to macrophytobenthic biomass and may have benefitted from highly available N in spring. The higher surface to volume ratio of filamentous algae allows higher P and N uptake rates from the water column compared to thick leathery tracheophytes (Raven and Taylor, 2003), which may contribute to the high spring production and biomass accumulation of this filamentous alga in spring. At similar TN and TP concentrations, proliferating filamentous algae were found also in other coastal areas of the Baltic Sea during spring (Dahlgren and Kautsky, 2004). The strong decrease of about 75% of the biomasses of macrophytes and epiphyton in early summer is probably linked to this high spring production of filamentous algae, which is assumed to have resulted in a severe decrease in light availability within the macrophytes canopy. As rooted macrophytes can use sediment nutrients (Granéli and Solander, 1988), any nutrient competition with epiphyton is assumed to be of minor importance. High grazing pressure by macrozoobenthos species may have added up to this reduction of biomass. In fact, high biomasses of macrozoobenthic grazers were observed within the vegetation (personal observation) similarly as in other vegetated Baltic lagoons (Hansen et al., 2011; Wlodarska-Kowalczuk et al., 2014). Our results suggest synergetic effects of epiphyton shading and herbivore grazing triggering macrophytobenthic biomass loss as assumed by (Hidding et al., 2016). A second bloom of the green filamentous alga C. linum was observed in late summer, probably related to the higher availability of P in the water column in this season. Proliferation of C. linum was observed under similar P concentrations (Menendez et al., 2002). The reduction in macrophytes total biomass at the end of the season was most probably caused by autumnal senescence and increasing hydrodynamic disturbances.
Apart from the temporary biomass decrease in early summer, the macrophytobenthic biomass was high throughout the vegetation period in the Vitter Bodden. High species numbers including different life strategies and species-specific growth periods may explain the high level of calculated macrophytobenthic NPP throughout the vegetation season. Spatial (e.g., distribution, growth forms) as well as temporal (e.g., seasonal strategies, length of growing season) variation in the macrophytobenthic community probably had a positive and stabilizing effect on its NPP. Small, low light adapted species such as F. fastigiata and F. vesiculosus grow in patches between and below higher vegetation, mainly consisting of S. pectinata, which creates a dense macrophytobenthic cover during the summer months (personal observations). High NPP was observed in other multi-species macrophytobenthic communities explained by the averaging species-specific photosynthetic performance and exposing constantly new tissue to irradiance in dense canopies (Middelboe and Binzer, 2004). Despite uncertainty in our estimations of available irradiance within the macrophytes canopy and macrophytobenthic NPP, calculated macrophytobenthic NPP in Vitter Bodden was within the range of temperate seagrass beds (Duarte, 1989) and macrophyte assemblages in freshwater lakes (Vadeboncoeur et al., 2001). The seasonal development of the macrophytobenthos in this mesotrophic lagoon indicates that reduced light availability within the macrophytobenthos is the most limiting factor with co-occurring nutrient limitation of epiphyton in spring and late summer. The underwater light climate has been shown to be the most relevant limiting factor for primary production of the macrophytobenthos in coastal waters of the southern Baltic Sea (Pärnoja et al., 2014; Piepho 2017).
Low Light Availability in Grabow Efficiently Suppresses Seasonal Development in the Macrophytobenthos
During 2017, high concentrations of seston and pigments caused a constantly low light availability in Grabow with a decreased euphotic zone of 60% in comparison to Vitter Bodden. The phytoplankton in the whole lagoon system of the DZBC is dominated by small-celled cyanobacteria of the Cyanobium clade (Albrecht et al., 2017), which are able to take up available nutrients very efficiently in this system (Berthold and Schumann, 2020), due to their high surface to volume ratio (Friebele and Fasut, 1978; Grillo and Gibson, 1979) resulting in high biomasses and low underwater light availability. Only S. pectinata persisted throughout the year. High abundances in clear water have been observed at somewhat elevated nutrient conditions and are explained by a competitive advantage of this fast-growing species under such conditions (Hilt et al., 2013; Blindow et al., 2016).
Biomass and primary production in the Grabow in 2017 were similar to what was described in the mid-1980s after the shift from clearwater to turbid water (Schiewer, 2001). In the early 1980ies, eutrophication caused a collapse of the macrophytobenthos in the entire DZBC which became dominated by the pelagic producers (Schiewer, 1998). Lagoons of the Baltic Sea receive nutrients from diffuse run-offs (Berthold et al., 2018b), atmospheric wet and dry deposition (Berthold et al., 2019) and from sediment (Bitschofsky, 2016; Berthold et al., 2018c). In 1990, new wastewater treatment plants reduced the discharge of P and N in the lagoons of the German Baltic Sea by over 89% (Nausch et al., 2011). Despite an 80% decline in nitrogen and phosphate discharge from the direct river sources, water column nutrient concentrations in the single lagoons of the DZBC have not decreased in the same magnitude (Berthold et al., 2018a), and the macrophytobenthos did not recover in the last 3 decades (Schiewer, 2001; Blindow and Meyer, 2015), which may be explained by long water retention times within this lagoon system and low exchange with the Baltic Sea.
Importance of Macrophytobenthos for the Lagoons’ Ecosystem Functioning
The moderate eutrophication of the Westrügensche lagoon still allows for a dense and species-rich macrophytobenthos, but with seasonal dominance of fast growing epiphyton which causes lower NPP and growth in the overgrown rooted tracheophyta and, combined with probably high grazing pressure, causes temporarily biomass decreases. Such seasonally fluctuating biomass indicates a reduction of vegetation stability, and are interpreted as an early warning signal of eutrophication, similarly to former observations in the Vitter Bodden that “bottom-dwellers” such as charophytes were replaced by taller macrophytes such as S. pectinata (Blindow et al., 2016). In the long-term, these changes may cause a decline of macrophytobenthic species richness and shortening of the vegetation period, described as a “crashing state” of freshwater ecosystems (Sayer et al., 2010). Proliferating filamentous algae have caused a decline of rooted vegetation in response to nutrient enrichment in other lagoons of the Baltic Sea over the last decades (Dahlgren and Kautsky, 2004).
Decreases in macrophytobenthic biomass weaken the habitat-stabilizing effects of the complex vegetation structure by reduction of water movements, sediment stabilization, immobilization of nutrients and accumulation of floral biomass (Gregg and Rose, 1982; Pedersen and Borum, 1997; Middelboe and Binzer, 2004) and has far reaching consequences for a lagoon’s food web. In the Vitter Bodden, the effect of the vegetation on water column parameters such as nutrients, phytoplankton densities and suspended material was found to be negligible, probably due to high water exchange rates with the open Baltic Sea and a low vegetation height. However, a distinct refuge effect of this vegetation was found for zooplankton and assumed to contribute to the temporarily high grazing pressure on phytoplankton (Meyer et al., 2019). In the more eutrophic Grabow, macrophytobenthos had low contributes to total system primary production and a reduced accumulation of biomass, which indicates severe light limitation.
Phytoplankton biomasses were roughly one order of magnitude higher in the Grabow than in the Vitter Bodden, but measured daytime hourly NPP rates were rather similar in both lagoons. These findings suggest that also phytoplankton was severely light-limited in the Grabow. The dominating alpha picocyanobacteria are of low food value for zooplankton, as their mucous envelopes may obstruct the filtering organs or lead to a rejection of the colonies (Schumann et al., 2009), and low phytoplankton mortality may therefore explain the maintenance of high densities in spite of low NPP rates. Earlier investigations from the same lagoon system showed that high water turbidity, caused by high phytoplankton biomass, can cause negative depth-integrated net primary production in winter and spring (Schumann et al., 2005). In addition, high microbial respiration rates may occur in summer months (Schiewer 1998). High self-shading and high community respiration rates can explain why nutrient enrichment does not further increase pelagic NPP in aquatic ecosystems, once a certain threshold is exceeded (Oviatt et al., 1986; Schiewer 1998; Blindow et al., 2006). Despite uncertainty in our estimates, we can show that total community areal NPP was far lower in the eutrophic lagoon than in the mesotrophic lagoon. We suggest that like shallow freshwater ecosystems (Lopez-Archilla et al., 1992; Blindow et al., 2006), also coastal lagoons can show a “paradox of enrichment” phenomenon already on the primary production level. According to our knowledge, this is the first empirical evidence of this phenomenon for coastal lagoons. As primary producers form the basis of the food web, we predict that the same pattern may be reflected by higher trophic levels.
Macrophytobenthos Response to Eutrophication and Management Recommendation
Macrophytobenthos, which is the most important contributor to total ecosystem primary production in the Vitter Bodden, has severely declined in the DZBC. Restoration efforts have to focus on stabilizing or–when lost–trying to restore this community. Thereby, it has to be considered that the steps leading to macrophytobenthic re-colonization may deviate from a simple reversion of the causes, which were responsible for the former loss (Duarte et al., 2008), and that, once lost, considerable efforts are necessary to restore this community (Scheffer et al., 1993). In the two studied coastal lagoons, the macrophytobenthos showed a complex response to different eutrophication pressures. Moderate eutrophication in the marine lagoon still allowed for a typical seasonal succession of the macrophytobenthic community, but the heavy overgrowth of rooted macrophytobenthos by filamentous algae in spring and autumn indicates that the nutrient levels were critical. During 2017, the macrophytobenthos main growth period and species succession was squeezed between the turbid spring and late summer condition, where proliferation of filamentous algae reduced the light available under the macrophytobenthic canopy. Additionally, a species shift from small bottom-dwellers, especially charophytes, to tall canopy-formers has occurred since the 1930s (Blindow et al., 2016). As a restoration measurement, exogenous nutrient flows into the lagoon should be reduced to prevent further decline in species diversity and thereby its habitat and food web stabilising effect. To control the success of this restoration effort, new macrophytobenthic health indicators sensitive to nutrient loads need to be developed, as already proposed for Danish coastal waters (Carstensen et al., 2014). For example, sensitive species with a later growing period such as F. vesiculosus and F. fastigiata need to be included into the macrophytobenthic health assessment and their biomass and cover regularly monitored in coastal lagoons (Carstensen et al., 2014).
High eutrophication pressure in the estuarine lagoon reduced macrophytobenthic species number, lowered biomass and production and shortened its growing period (Piepho, 2017). Only the pondweed S. pectinata was present during the complete observation period of 2017 and could start its growing period before the high phytoplankton biomasses limiting underwater light availability during the rest of the year. Exogenous nutrient load reductions for the DZBC over the last 40 years have not led to the recovery of the macrophytobenthic species diversity and biomasses in Grabow. Strong feedback mechanisms are assumed to stabilise the phytoplankton dominance (Schiewer, 1998). Additional internal restoration measurements have to be considered to reduce the endogenous nutrient burden and phytoplankton biomass and improve the under water light availability, but are hard to apply because of the large catchment area of this lagoon system and its connection to surrounding lagoons. Planting of submerged macrophytes in spring, when light conditions are most favourable for plant growth should be considered, to recreate lost vegetated areas (van Keulen et al., 2003).
Data Availability Statement
The datasets generated for this study are available on request to the corresponding author.
Author Contributions
All authors were involved in the conception and design of the study as well as the provision of study material. MP, MB, and RS analyzed and interpreted the nutrient data. MP and IB analyzed the macrophytobenthos data set. SD monitored water column parameter in Vitter Bodden. MP wrote the manuscript with extensive inputs from MB, RS, SD and IB. All authors read and approved the manuscript.
Funding
This research was funded by the Federal Ministry of Education and Research, Germany, project BACOSA (03F0737C). We acknowledge support for the Article Processing Charge from the DFG (German Research Foundation, 393148499) and the Open Access Publication Fund of the University of Greifswald.
Conflict of Interest
The authors declare that the research was conducted in the absence of any commercial or financial relationships that could be construed as a potential conflict of interest.
Acknowledgments
This work was done with the permission of the State Office for Agriculture, Food Safety and Fisheries Mecklenburg-Vorpommern and the National Park Authority Vorpommern. The authors thank the German Meteorological Service for providing the irradiance and wind data sets. This study would not have been possible without the logistic and analytic support by the employees of the Biologische Station Zingst, namely R. Wulff and V. Reiff, and the Biologische Station of Hiddensee, namely I. Kreuzer, G. Zenke and T. Kreuzer. We like to thank the field assistance by B. Anderson, M. Steinmüller, R. Liebetrau, F. Blaffert, L. Sniehotte, J. Reiche, T. Wagner, A. Brauer, K. Steinfurth, B. Heeren, I. Petersen, and D. Auch. Thanks to H. Radtke for his help with calculating the error propagation. The manuscript improved considerably due to the valid comments of both reviewers.
Supplementary Material
The Supplementary Material for this article can be found online at: https://www.frontiersin.org/articles/10.3389/feart.2020.542391/full#supplementary-material.
References
Albrecht, M., Pröschold, T., and Schumann, R. (2017). Identification of cyanobacteria in a eutrophic coastal lagoon on the southern Baltic coast. Front. Microbiol. 8, 923. doi:10.3389/fmicb.2017.00923
Angradi, T. R. (1993). Chlorophyll a content of seston in a regulated Rocky Mountain river, Idaho, USA. Hydrobiologia. 259, 39–46. doi:10.1007/BF00005963
Apostolaki, E. T., Holmer, M., Marbà, N., and Karakassis, I. (2011). Epiphyte dynamics and carbon metabolism in a nutrient enriched Mediterranean seagrass (Posidonia oceanica) ecosystem. J. Sea Res. 66, 135–142. doi:10.1016/j.seares.2011.05.007
Armstrong, F. A., Stearns, C. R., and Strickland, J. D. (1967). Measurement of upwelling and subsequent biological processes by means of Technicon autoanalyzer and associated equipment. Deep-Sea Res. 14, 381–389. doi:10.1016/0011-7471(67)90082-4
Benson, B. B., and Krause, J. C. (1984). The concentration and isotopic fractionation of oxygen dissolved in freshwater and seawater in equilibrium with the atmosphere. Limnol. Oceanogr. 29, 620–632. doi:10.4319/lo.1984.29.3.0620
Berthold, M., Karsten, U., von Weber, M., Bachor, A., and Schumann, R. (2018a). Phytoplankton can bypass nutrient reductions in eutrophic coastal water bodies. Ambio. 47, 146–158. doi:10.1007/s13280-017-0980-0
Berthold, M., Karstens, S., Buczko, U., and Schumann, R. (2018b). Potential export of soluble reactive phosphorus from a coastal wetland in a cold-temperate lagoon system: buffer capacities of macrophytes and impact on phytoplankton. Sci. Total Environ. 616-617, 46–54. doi:10.1016/j.scitotenv.2017.10.244
Berthold, M., and Schumann, R. (2020). Phosphorus dynamics in a eutrophic lagoon: uptake and Utilization of nutrient pulses by phytoplankton. Front Mar Sci. 7, 281. doi:10.3389/fmars.2020.00281
Berthold, M., Wulff, R., Reiff, V., Karsten, U., Nausch, G., and Schumann, R. (2019). Magnitude and influence of atmospheric phosphorus deposition on the southern Baltic Sea coast over 23 years: implications for coastal waters. Environ. Sci. Eur. 31, 27. doi:10.1186/s12302-019-0208-y
Berthold, M., Zimmer, D., Reiff, V., and Schumann, R. (2018c). Phosphorus contents re-visited after 40 years in muddy and sandy sediments of a temperate lagoon system. Front Mar Sci. 5, 305. doi:10.3389/fmars.2018.00305
Berthold, M., Zimmer, D., and Schumann, R. (2015). A simplified method for total phosphorus digestion with potassium persulphate at sub-boiling temperatures in different environmental samples. Rostocker Meeresbiol Beitr. 25, 7–25.
Bitschofsky, F. (2016). “Phosphorus dynamics in sediments of Darß-Zingst Bodden chain, a eutrophic estuary in the southern Baltic Sea.” Available at: http://rosdok.uni-rostock.de/file/rosdok_disshab_0000001782/rosdok_derivate_0000037768/Dissertation_Bitschofsky_2017.pdf.
Blindow, I., Dahlke, S., Dewart, A., Flügge, S., Hendreschke, M., Kerkow, A., et al. (2016). Long-term and interannual changes of submerged macrophytes and their associated diaspore reservoir in a shallow southern Baltic Sea bay: influence of eutrophication and climate. Hydrobiologia. 778 (1), 121–136. doi:10.1007/s10750-016-2655-4
Blindow, I., Hargeby, A., Meyercordt, J., and Schubert, H. (2006). Primary production in two shallow lakes with contrasting plant form dominance: a paradox of enrichment?. Limnol. Oceanogr. 51, 2711–2721. doi:10.4319/LO.2006.51.6.2711
Blindow, I., and Meyer, J. (2015). Submerse Makrophyten während Eutrophierung und Remesotrophierung – ein Vergleich von inneren und äußeren Boddengewässern. Rostocker Meeresbiol Beitr. 25, 105–118.
Blümel, C. (2004). “Chara baltica,” in Charophytes of the Baltic Sea. Editors H. Schubert and I. Blindow (Ruggell, Liechtenstein: Gantner), 53–56.
Bühler, A. (2016). Distribution of Zostera marina in the coastal waters of the Baltic Sea - a case study at the German island HiddenseeMaster thesis. Germany: University of Rostock.
Canty, A. S., and Ripley, B. (2016). Boot: bootstrap R (S-plus) functions. R package version1., 3–18.
Carstensen, J., Krause-Jensen, D., and Josefson, A. (2014). Development and testing of tools for intercalibration of phytoplankton, macrovegetation and benthic fauna in Danish coastal areas. Aarhus: Aarhus University, DCE – Danish Centre for Environment and Energy, 85. Available at:http://dce2.au.dk/pub/SR93.pdf.
Cerco, C. F., and Moore, K. (2001). System-wide submerged aquatic vegetation model for Chesapeake Bay. Estuar. Coast. 24, 622–634. doi:10.2307/1353254
Cloern, J. E. (2001). Our evolving conceptual model of the coastal eutrophication problem. Mar. Ecol. Prog. Ser. 210, 223–253. doi:10.3354/meps210223
Correns, M. (1979). Der Wasserhaushalt der Bodden- und Haffgewässer der DDR als Grundlage für die weitere Erforschung ihrer Nutzungsfähigkeit zu Trink- und Brauchwasserzwecken. Berlin, Germany: Dissertation, Humboldt-Universität.
Dahlgren, S., and Kautsky, L. (2004). Can different vegetative states in shallow coastal bays of the Baltic Sea be linked to internal nutrient levels and external nutrient load?. Hydrobiologia. 514, 249–258.
Davison, A. C., and Hinkley, D. V. (1997). Bootstrap methods and their applications. Cambridge, UK: Cambridge University Press.
Duarte, C. M., Conley, D. J., Carstensen, J., and Sánchez-Camacho, M. (2008). Return to neverland: shifting baselines affect eutrophication restoration targets. Estuar. Coast. 32, 29–36. doi:10.1007/s12237-008-9111-2
Duarte, C. M. (1989). Temporal biomass variability and production/biomass relationships of seagrass communities. Mar. Ecol. Prog. Ser. 51, 269–276. doi:10.3354/meps051269
Duffy, J. E., Macdonald, K. S., Rhode, J. M., and Parker, J. D. (2001). Grazer diversity, functional, redundancy, and productivity in seagrass beds: an experimental test. Ecology. 82, 2417–2434. doi:10.1890/0012-9658(2001)082[2417:GDFRAP]2.0.CO;2
Evans, A. S., Kenneth, L. W., and Penhale, P. A. (1986). Photosynthetic temperature acclimation in two coexisting seagrasses, Zostera marina L. and Ruppia maritima L. Aquat. Bot. 24, 185–197. doi:10.1016/0304-3770(86)90095-1
Friebele, E. S., and Fasut, M. A. (1978). Relationship between phytoplankton cell size and the rate of orthophosphate uptake: in situ observations of an estuarine population. Mar. Biol. 45, 39–52. doi:10.1007/BF00388976
Granéli, W., and Solander, D. (1988). Influence of aquatic macrophytes on phosphorus cycling in lakes. Hydrobiologia. 170, 245–266. doi:10.1007/BF00024908
Gregg, W. W., and Rose, F. L. (1982). The effects of aquatic macrophytes on the stream microenvironment. Aquat. Bot. 14, 309–324. doi:10.1016/0304-3770(82)90105-X
Grillo, J. F., and Gibson, J. (1979). Regulation of phosphate accumulation in the unicellular cyanobacterium Synechococcus. J. Bacteriol. 140, 508–517.
Hansen, H. P., and Koroleff, F. (1999). “Chapter 10 determination of nutrients,” in Methods of seawater analysis. Editors K. Grasshoff, K. Kremling, and M. Erhardt 3rd ed. (Wiley VCH), 159–228.
Hansen, J. P., Wikström, S. A., Axemar, H., and Kautsky, L. (2011). Distribution differences and active habitate choices of invertebrates between macrophytes of different morphological complexity. Aquat. Ecol. 45, 11–22. doi:10.1007/s10452-010-9319-7
Hidding, B., Bakker, E. S., Hootsmans, M. J. M., and Hilt, S. (2016). Synergy between shading and herbivory triggers macrophytes loss and regime shift in aquatic systems. Oikos. 125, 1489–1495. doi:10.1111/oik.03104
Hilt, S., Köhler, J., Adrian, R., Monaghan, M. T., and Sayer, C. D. (2013). Clear, crashing, turbid and back – long-term changes in macrophyte assemblages in a shallow lake. Freshw. Biol. 58, 2027–2036. doi:10.1111/fwb.12188
Howard-Williams, C., and Allanson, B. R. (1981). Phosphorus cycling in a dense Potamogeton pectinatus L. bed. Oecologia. 49, 56–66. doi:10.1007/BF00376898
Huang, X. L., and Zhang, J. Z. (2009). Neutral persulfate digestion at sub-boiling temperature in an oven for total dissolved phosphorus determination in natural waters. Talanta. 78, 1129–1135. doi:10.1016/j.talanta.2009.01.029
Jankowska, E., Wlodarska-Kowalczuk, M., Kotwicki, L., Balazy, P., and Kuliński, K. (2014). Seasonality in vegetation biometrics and its effects on sediment characteristics and meiofauna in Baltic seagrass meadows. Estuar. Coast Shelf Sci. 1139, 159–170. doi:10.1016/j.ecss.2014.01.003
Jasby, S. W., and Platt, T. (1976). Mathematical formulation of the relationship between photosynthesis and light for phytoplankton. Limnol. Oceanogr. 21, 540–547. doi:10.4319/lo.1976.21.4.0540
Johansson, G., and Snoeijs, P. (2002). Macroalgal photosynthetic responses to light in relation to thallus morphology and depth zonation. Mar. Ecol. Prog. Ser. 244, 63–72. doi:10.3354/meps244063
Kemp, W. M., Brooks, M. T., and Hood, R. R. (2001). Nutrient enrichment, habitat variability and trophic transfer efficiency in simple models of pelagic ecosystems. Mar. Ecol. Prog. Ser. 223, 83–87. doi:10.3354/MEPS223073
Kennedy, H., Beggins, J., Duarte, C. M., Fourqurean, J. W., Holmer, M., Marbà, N., et al. (2010). Seagrass sediments as a global carbon sink: isotopic constraints. Global Biogeochem. Cycles. 24, GB4026. doi:10.1029/2010GB003848
Kiirikki, M., and Lehvo, A. (2012). Life strategies of filamentous algae in the northern Baltic proper. Sarsia. 82, 259–267. doi:10.1080/00364827.1997.10413653
King, R. J., and Schramm, W. (1976). Photosynthetic rates of benthic marine algae in relation to light intensity and seasonal variations. Mar. Biol. 37, 215–222. doi:10.1007/BF00387606
Kronvang, B., Jeppesen, E., Conley, D. J., Søndergaard, M., Larsen, S. E., Ovesen, N. B., et al. (2005). Nutrient pressures and ecological responses to nutrient loading reductions in Danish streams, lakes and coastal waters. J. Hydrol. 304, 274–288. doi:10.1016/j.jhydrol.2004.07.03
Lapointe, B. E., and Tenore, K. R. (1981). Experimental outdoor studies with Ulva fasciata Delile. I. Interaction of light and nitrogen on nutrient uptake, growth, and biochemical composition. J. Exp. Mar. Biol. Ecol. 53, 135–152. doi:10.1016/0022-0981(81)90015-0
Leskinen, E., Miikinen, A., Fortelius, W., Lindström, M., and Salemaa, H. (1992). “Primary production of macroalgae in relation to the spectral range and sublittoral light conditions in the Tvärminne archipelago, northern Baltic Sea,” in Algological studies of nordic coastal waters. (Uppsala, Sweden: Acte Phytogeographica Suecica), 85–94.
López-Archilla, A. I., Mollá, S., Coleto, M. C., Guerrero, M. C., and Montes, C. (1992). Ecosystem metabolism in a mediterranean shallow lake (laguna de Santa olallan, doñana national Park, SW Spain). Wetlands. 24, 848–858. doi:10.1672/0277-5212(2004)024[0848:emiams]2.0.co;2
Madsen, T. V., and Brix, H. (1997). Growth, photosynthesis and acclimation by two submerged macrophytes in relation to temperature. Oecologia. 110, 320–327. doi:10.1007/s004420050165
Malcolm-Lawes, D. J., and Wong, K. H. (1990). Determination of orthophosphate in water and soil using a flow analyzer. Analyst. 15, 65–67. doi:10.1039/AN9901500065
Marcus, B. M. (1980). Relationship between light intensity and chlorophyll content in Myriophyllum spicatum L. in Canadice Lake (New York, U.S.A.). Aquat. Bot. 9, 169–172. doi:10.1016/0304-3770(80)90017-0
Meek, D., Hatfield, J., Howell, T., Idso, S., and Reginato, R. J. (1984). A generalized relationship between photosynthetically active radiation and solar radiation. Argonomy J. 76, 939–945.
Menendez, M., Herrera, J., and Comín, F. A. (2002). Effect of nitrogen and phosphorus supply on growth, chlorophyll content and tissue composition of the macroalga Chaetomorpha linum (O.F. Müll.) Kütz in a Mediterranean coastal lagoon. Sci. Mar. 66, 355–364.
Meyer, J., Dahlke, S., Kafka, M., Kerkow, A., Lindner, C., Kube, S., et al. (2019). Submerged vegetation in a shallow brackish lagoon does not enhance water clarity but offers substantial refuge for zooplankton. Aquat. Bot. 154, 1–10. doi:10.1016/j.aquabot.2018.12.002
Middelboe, A. L., and Blinzer, T. (2004). Importance of canopy structure on photosynthesis in single- and multi-species assemblages of marine macroalgae. Oikos. 107, 422–432. doi:10.1111/j.0030-1299.2004.13345.x
Middelboe, A. L., and Markager, S. (1997). Depth limits and minimum light requirements of freshwater macrophytes. Freshw. Biol. 37, 553–568.
Munkes, B. (2005). Eutrophication, phase shift, the delay and the potential return in the Greifswalder Bodden, Baltic Sea. Aquat. Sci. 67, 372–381. doi:10.1007/s00027-005-0761-x
Murphy, J., and Riley, J. P. (1962). A modified single solution method for the determination of phosphate in natural waters. Anal. Chim. Acta. 27, 31–36. doi:10.1016/S0003-2670(00)88444-5
Nausch, G., Bachor, A., Petenati, T., Voss, J., and Von Weber, M. (2011). Nutrients in the German coastal waters of the Baltic Sea and adjacent areas. Meeresumwelt Aktuell Nord Ostsee. 1, 1–16.
Oviatt, C. A., Rudnick, D. T., Keller, A. A., Sampou, P. A., and Almqvist, G. T. (1986). A comparison of system (O2 and CO2) and C-14 measurements of metabolism in estuarine mesocosms. Mar. Ecol. Prog. Ser. 28, 57–68.
Pärnoja, M., Kotta, J., Orav-Kotta, H., and Paalme, T. (2014). Comparisons of individual and community photosynthetic production indicate light limitation in the shallow water macroalgal communities of the Northern Baltic Sea. Mar. Ecol. 35, 19–27. doi:10.1111/maec.12074
Pedersen, M. F., and Borum, J. (1997). Nutrient control of estuarine macroalgae growth: Growth strategy and balance between nitrogen requirements and uptake. Mar. Ecol. Prog. Ser. 161, 155–163. doi:10.3354/meps161155
Piepho, M. (2017). Assessing maximum depth distribution, vegetated area, and production of submerged macrophytes in shallow, turbid coastal lagoons of the southern Baltic Sea. Hydrobiologia. 788, 1–14. doi:10.1007/s10750-017-3107-5
Plus, M., Auby, I., Verlaque, M., and Levavasseur, G. (2005). Seasonal variations in photosynthetic irradiance response curves of macrophytes from a Mediterranean coastal lagoon. Aquat. Bot. 81, 157–173. doi:10.1016/j.aquabot.2004.10.004
Prosser, C. L. (1961). “Oxygen: respiration and metabolism,” in Comparative anidal physiology. Editors C. L. Prosser, F. A. Brown, and B. Saunders 2nd ed. (Philadelphia, PA: W.B. Saunders), 165–211.
R Core Team (2019). R: a language and environment for statistical computing. Vienna, Austria: R Foundation for Statistical Computing. Available at:https://www.R-project.org/.
Rasmusson, L. (2015). Seagrass respiration. An assessment of oxygen consumption patterns of temperate marine macrophytes. Stockholm, Sweden: Stockholm University.
Raven, J. A., and Taylor, R. (2003). Macroalgae growth in nutrient-enriched estuaries: a biogeochemical and evolutionary approach. Water. Air Soil Pollut. 3, 7–26. doi:10.1023/A:1022167722654
Rosenzweig, M. L. (1971). Paradox of enrichment: destabilization of exploitation ecosystems in ecological time. Science 171, 385–387. doi:10.1126/science.171.3969.385
Russell, G., Ruuskanen, A., Kiirikki, M., and Høisæter, T. (2012). Sunlight, shade and tidal night: Photoadaptation in Fucus vesiculosus L. Sarsia. 83, 381–386. doi:10.1080/00364827.1998.10413697
Sayer, C. D., Burgess, A., Karl, K., Davidson, T. A., Peglar, S., Yang, H., et al. (2010). Long-term dynamics of submerged macrophytes and algae in a small and shallow, eutrophic lake: implications for the stability of macrophyte-dominance. Freshw. Biol. 55, 565–583. doi:10.1111/j.1365-2427.2009.02353.x
Scheffer, M., Hosper, S. H., Meijer, M. L., Moss, B., and Jeppesen, E. (1993). Alternative equilibria in shallow lakes. Trends Ecol. Evol. 8, 275–279. doi:10.1016/0169-5347(93)90254-M
Schiewer, U. (1998). 30 years’ eutrophication in shallow brackish waters – lessons to be learned. Hydrobiologia. 363, 73–79. doi:10.1023/A:1003194226294
Schiewer, U. (2007). “Darß-Zingst Boddens, Northern Rügener Boddens and Schlei,” in Ecology of Baltic coastal waters. Editors U. Schiewer, M. M. Caldwell, G. Heldmaier, R. B. Jackson, O. L. Lange, H. A. Mooneyet al. (Berlin-Heidelberg:Springer), 35–86.
Schiewer, U. (2001). Salzhaff, Greifswalder Bodden, Darß-Zingster Boddenkette: Gewässereutrophierung und Pufferkapazität – ein Vergleich. Rostocker Meeresbiol Beitr. 9, 5–19.
Schlitzer, R. (2018). Ocean Data View. Available at:https://odv.awi.de.
Schubert, H., and Foster, M. (1997). Sources of variability in the factors used for modelling primary productivity in eutrophic waters. Hydrobiologia. 349, 75–85. doi:10.1023/A:1003097512651
Schumann, R., Hammer, A., Görs, S., and Schubert, H. (2005). Winter and spring phytoplankton composition and production in a shallow eutrophic Baltic lagoon. Estuar. Coast Shelf Sci. 62, 169–181. doi:10.1016/j.ecss.2004.08.015
Schumann, R., Rentsch, D., Görs, S., and Schiewer, U. (2001). Seston particles along a eutrophication gradient in coastal waters of the Southern Baltic Sea: Significance of detritus and transparent mucoid material. Mar. Ecol. Prog. Ser. 218, 17–31. doi:10.3354/meps218017
Schumann, R., Schoor, A., and Schubert, H. (2009). Fine resolution of primary production and its limitation in phytoplankton communities of the Darß-Zingst Bodden Chain, a coastal lagoon of the Southern Baltic Sea Baltic. Coast. Zone. 13, 97–125.
Stanley, R. A., and Naylor, A. W. (1972). Photosynthesis in Eurasian Watermilfoil (Myriophyllum spicatum L.). Plant Physiol. 50, 149–151. doi:10.1104/pp.50.1.149
Tagliapietra, D., Sigovini, M., and Chiradini, A. V. (2009). A review of terms and definititons to categorise estuaries, lagoons and associated environments. Mar. Freshw. Res. 60, 497–509. doi:10.1071/MF08088
Vadeboncoeur, Y., Lodge, D. M., and Carpenter, S. R. (2001). Whole lake detilization effects on distribution of primary production between benthic and pelagic habitats. Ecology. 82, 1065–1077. doi:10.1890/0012-9658(2001)082[1065:WLFEOD]2.0.CO;2
van Keulen, M., Paling, E. I., and Walker, C. J. (2003). Effect of planting unit size and sediment stabilization on seagrass transplants in Western Australia. Restor. Ecol. 11, 1–6. doi:10.1046/j.1526-100X.2003.00036.x
Walsby, A. E. (1997). Numerical integration of phytoplankton photosynthesis through time and depth in a water column. New Phytol. 136, 189–209. doi:10.1046/j.1469-8137.1997.00736.x
Wang, F. K. (2001). Confidence interval for the mean of non-normal data. Qual. Reliab. Eng. Int. 17, 257–267. doi:10.1002/qre400
Wennberg, T. (1992). “Colonization and succession of macroalgae on a breakwater in Laholm Bay, a eutrophicated brackish water area (SW Sweden),” in Algological studies of nordic coastal waters. (Uppsala, Sweden: Acte Phytogeographica Suecica), 49–65.
Włodarska-Kowalczuk, M., Jankowska, E., Kotwicki, L., and Balazy, P. (2014). Evidence of season-dependency in vegetation effects on macrofauna in temperate seagrass meadows (Baltic Sea). PloS One. 9, e100788. doi:10.1371/journal.pone.0100788
Keywords: eutrophication, photosynthesis, submerged vegetation, drivers, transitional waters
Citation: Paar M, Berthold M, Schumann R, Dahlke S and Blindow I (2021) Seasonal Variation in Biomass and Production of the Macrophytobenthos in two Lagoons in the Southern Baltic Sea. Front. Earth Sci. 8:542391. doi: 10.3389/feart.2020.542391
Received: 12 March 2020; Accepted: 14 December 2020;
Published: 28 January 2021.
Edited by:
Jianghua Wu, Memorial University of Newfoundland, CanadaReviewed by:
Martin Snickars, Åbo Akademi University, FinlandJan Köhler, Leibniz-Institute of Freshwater Ecology and Inland Fisheries (IGB), Germany
Copyright © 2021 Paar, Berthold, Schumann, Dahlke and Blindow. This is an open-access article distributed under the terms of the Creative Commons Attribution License (CC BY). The use, distribution or reproduction in other forums is permitted, provided the original author(s) and the copyright owner(s) are credited and that the original publication in this journal is cited, in accordance with accepted academic practice. No use, distribution or reproduction is permitted which does not comply with these terms.
*Correspondence: Martin Paar, bWFydGluLnBhYXJAdW5pLXJvc3RvY2suZGU=
†Present address: Martin Paar Aquatic Ecology, Universität Rostock, Rostock, Germany