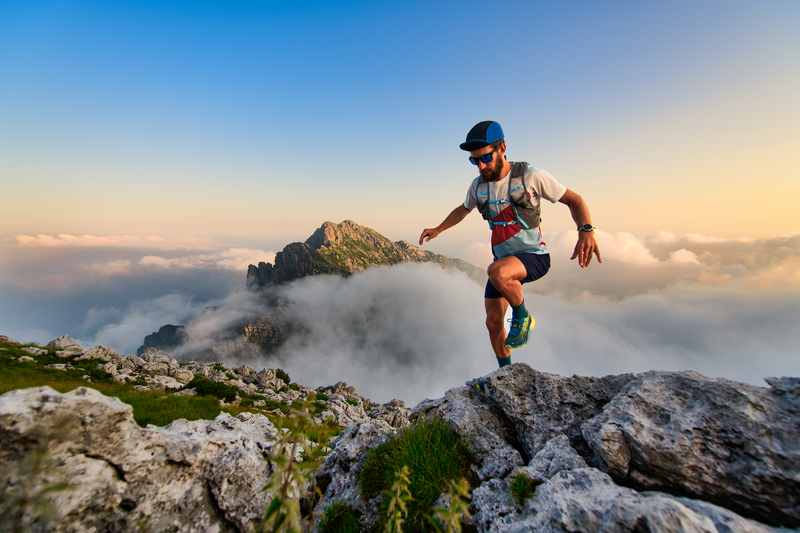
94% of researchers rate our articles as excellent or good
Learn more about the work of our research integrity team to safeguard the quality of each article we publish.
Find out more
REVIEW article
Front. Earth Sci. , 18 December 2020
Sec. Petrology
Volume 8 - 2020 | https://doi.org/10.3389/feart.2020.541094
This article is part of the Research Topic The Early Earth Crust and its Formation View all 7 articles
The size of continents is an essential parameter to understand the growth of the continental crust and the evolution of the solid Earth because it is subject to tectonism and mantle convection and affects the preservation of the crust. This article reviews the secular change in the size of continents on the early Earth, focusing on terrigenous clastic rocks, especially quartzose sandstones occurring on relatively large continents. The earliest continental crust in the Hadean or early Archean was produced with a width of ∼200–500 km, similar to modern oceanic island arcs along subduction zones or oceanic islands in hot spot regions by mantle plume heating. Through the collision and amalgamation of such primitive continental crusts, continental blocks over 500 km in width and length evolved and appeared by ca. 3.5 Ga. Through further amalgamation, during ca. 3.3–2.5 Ga, the Archean continents emerged with widths and lengths greater than 1,000 km, which were still smaller than those of modern continents. Continents with widths and lengths of nearly 10,000 km have existed since ca. 2.4 Ga (early Proterozoic). Further analyses of the composition and formation mechanism of clastic rocks will help reveal more quantitative secular changes in the sizes of continents.
The bimodal topography, land masses consisting of several major continents with islands of various sizes and an ocean with an average depth of nearly 4,000 m, is unique to the Earth among other planets of our Solar System. Since the establishment of plate tectonics (Wilson, 1965; Morgan, 1968), the subduction zones of oceanic plates are characterized by the production of granitic continental crust through arc magmatism and its subsequent reworking by sedimentary and metamorphic processes (Dewey and Bird, 1970; Matsuda and Uyeda, 1971). Recycling of the continental crust into the mantle is also significant in the subduction zones through tectonic erosion (subduction erosion), sedimentary subduction, and subduction of the island arc crust (e.g., von Huene and Lallemand, 1990; Scholl and von Huene, 2007, 2009; Clift et al., 2009; Yamamoto et al., 2009; Stern and Scholl, 2010). The growth history of the continental crust is a cumulative result of the production, recycling, and reworking processes; in addition, it has been debated for a long time.
By compiling the worldwide whole-rock geochronological data available before the 1960s, Hurley and Rand (1969) demonstrated the age structure of modern continents. They clarified that Archean crusts occupy only 20% of the total continental volume. Considering the influence of crustal reworking and recycling on the age structure of the modern continents, many studies aiming to reconstruct the growth history of the continental crust appeared (e.g., Fyfe, 1978; Armstrong, 1981; O’Nions et al., 1979; Dewey and Windley, 1981; Allègre and Luck, 1980; McLennan and Taylor, 1982; Reymer and Schubert, 1984; Figure 1). After the technical development and popularization of in-situ zircon U–Pb dating using SHRIMP (Froude et al., 1983; Stern et al., 2016) or LA-ICPMS (Hirata and Nesbitt, 1995) and zircon Lu-Hf isotopic analysis (Thirlwall and Walder, 1995; Vervoort et al., 1996; Knudsen et al., 2001; Griffin et al., 2004), many studies have contributed to the elucidation of continental growth history based on zircon analyses and data compilation (Rino et al., 2004; Rino et al., 2008; Condie et al., 2009; Hawkesworth et al., 2009; Belousova et al., 2010; Voice et al., 2011; Dhuime et al., 2012). By comparing the age structure given by Rino et al. (2004; 2008), older crust (before ca. 2 Ga) is known to be rare in modern continents, even after the exclusion effect of the intra-crustal sedimentary processes, as previously shown by Hurley and Rand (1969). Furthermore, based on compilations of the zircon U–Pb age and Lu–Hf isotopic analyses, several studies considered the crustal reworking in continental growth models (Belousova et al., 2010.; Komiya, 2011; Dhuime et al., 2012; Roberts and Spencer, 2015; Cawood and Hawkesworth, 2019). These studies can possibly evaluate the relative degree of crustal reworking and the fraction of new crust from the depleted mantle at each period. However, they cannot directly determine the volumes of crustal production and destruction because some assumptions for geotectonic processes are required. Subsequently, advanced statistical techniques or box model calculations have been adapted to interpret the zircon data compilation (Cheng, 2017; Puetz et al., 2017; Dhuime et al., 2018; Korenaga, 2018; Puetz and Condie, 2019), but the estimation of crustal production and destruction through time are still unclear.
FIGURE 1. (A) Previous growth models of the continental crust (F1978: Fyfe, s1978; A1981: Armstrong, 1981; MandT 1982: McLennan and Taylor, 1982; MandB 1994: McCulloch and Bennett, 1994; B+2010: Belousova et al., 2010; D+2012: Dhuime et al., 2012). Black solid and dashed lines show the age structure of the modern continents based on geochronological data (Hurley and Rand, 1969; Rino et al., 2008) which commonly indicate the rareness of the ancient crust in modern continents. The growth curves of the continental crust differs largely between studies, considering the great effects of crustal recycling/reworking (red lines) and the limited effects of crustal recycling/reworking (green lines). (B) Geotectonic map of the modern continents with localities of Hadean zircon grains modified after Tsutsumi et al. (2018) (GS, Greenstone). The abundant occurrence of Hadean detrital zircon is limited in ca. 3.0–3.3 Ga sandstone and quartzose clastic rocks (Froude et al., 1983; Compston and Pidgeon, 1986; Wilde et al., 2001; Cavosie et al., 2007; Nebel et al., 2014; Valley et al., 2014; Nelson, 2002; Wyche et al., 2004; GSWA; 2005; Wyche, 2007; Paquette et al., 2015; Byerly et al., 2018), even though many odd localities have been reported (Mojzsis and Harrison, 2002; Iizuka et al., 2006; Martel et al., 2008; Diwu et al., 2013; Nadeau et al., 2013; Cui et al., 2013; Xing et al., 2014; Li et al., 2016).
To improve the understanding of the growth history of continental crusts, this study focuses on the secular change in the size of continental blocks as a major factor in determining the preservation and destruction of continental crust and as a physical parameter to constrain the evolution of the solid Earth. Previous studies on continental growth simplified the crustal differentiation and did not focus on a concrete geological entity of the continental crust. When the total amount of continental crust in the early Earth was not large, continental crusts should have existed as small continental blocks. With the increase in the total continental crust amount, the size of continental blocks should have increased through repeated amalgamations of smaller continental blocks. The size of continents affects the preservation of old crusts and the growth rate of the total amount because the production and destruction of new and preexisting continental crusts have occurred along the plate subduction zones, respectively. In the modern continental crust, the preservation of old crust occurs in the interior of continents, several hundred kilometers away from subduction zones (Figure 1). In contrast, if the size of continental blocks on the early Earth was substantially smaller than that of modern ones, the ratio of plate subduction margins to the total mass of the continental crust should have been larger than the present ratio. Additionally, small continental blocks are easily recycled into the mantle through continental subduction. As a result, the old crust was rarely preserved on the surface of the early Earth (Figures 2A,B). In the early Earth, plate subduction could have been limited, and the production of felsic rocks was mainly caused by a mantle plume (Figure 2D: e.g., Sizova et al., 2010; discussed in the next chapter). Before the start of plate subduction, the production and destruction of continental crust were inactive and would have occurred regardless of the size and other topographic features of the continental blocks. Nonetheless, the size of continents would significantly contribute to the preservation of the crust immediately after the plate subduction had begun. Furthermore, the secular change in the size of continents possibly reflects the evolutionary history of the planetary interior because amalgamation and rifting of continental blocks are controlled by plate motion and mantle convection. To determine the growth history of the continental crust, numerical calculations involving mantle convection and tectonic activities are ultimately necessary. Geological observations and petrological-geochemical signatures can work as constraints in the numerical simulation. Among them, the size of continents can be used more directly for such geotectonic simulations, as a special physical parameter. This study aims to examine the existing knowledge regarding the size of continents on the early Earth. Prior to this, the tectonic regime on the early Earth, especially with regard to the time of initialization of plate subduction and the generation of the granitic continental crust, is reviewed in the next section.
FIGURE 2. Difference in the preservation of older continental crust from subduction orogen depending on the size of continental blocks (A) In a large continent, there are many areas of continental interior far from the subduction orogen and the older crust can be preserved (B) In a small continental block like the oceanic island arc, most of it is covered with subduction orogenic belt and there is only limited preservation of relatively older crust (C) Archean subduction model similar to modern oceanic island arcs (D) Archean pre-subduction model.
There are many theories on the initiation of plate tectonics or plate subduction. Hereafter, this paper uses the term “plate subduction” to indicate the sheet-shaped continuous dropping of the lithosphere composed of the mafic oceanic crust and ultramafic mantle rocks into the deeper portion of the mantle. This definition offers a clear distinction between the Archean plate subduction and modern-style plate tectonics, which implies a continuous subduction of large, cooled, and rigid oceanic plates (Figures 2A,B). Archean oceanic plates and their subduction would have been different from their modern counterparts. Due to the hotter Archean mantle (Herzberg et al., 2010), more extensive partial melting occurred at the mid-oceanic spreading center to form an Archean oceanic crust that was less depleted and over 4–5 times thicker than at present (∼7 km) (Sleep and Windley 1982; Abbott et al., 1994; Hastie et al., 2016). The Archean oceanic plates are considered to have been subjected to shallow dip angles underneath the other oceanic plates (Figure 2C; de Wit and Hart, 1993; de Wit, 1998; Komiya et al., 2002; Smithies et al., 2018; Martin et al., 2005; Ernst, 2009; Hastie et al., 2016; Ernst, 2017).
Clear geological records of plate subduction are as old as ca. 3.0 Ga (late Archean). Through geological observations and seismic profiling, the internal structures of cratons have been investigated. Many cratons preserve the crustal structure of terranes arranged in parallel and bounded by low-angle faults, suggesting the downward stacking of crustal blocks by repeated subduction and accretion processes; e.g., the Superior (Ludden and Hynes 2000; White et al., 2003; Angus et al., 2009), Yilgarn (Blewett et al., 2010; Czarnota et al., 2010; Goscombe et al., 2019), and Dharwar cratons (Mandal et al., 2017). A recent paleomagnetism study of the Honeyeater Basalt in the East Pilbara Craton suggests a modern plate motion velocity of ≥2.5 cm/year between ca. 3.4–3.2 Ga (Brenner et al., 2020). Conversely, plate subduction before ca. 3.0 Ga is controversial, as units >3.0 Ga are much rarer and their original geological structures are not well preserved. Several studies have identified ca. 4.0–3.8 Ga accretionary complexes with duplex structures and oceanic plate stratigraphy from the Isua Greenstone belt and Nulliak supracrustal rocks (Komiya et al., 2002, Komiya et al., 2015; Shimojo et al., 2016; Komiya et al., 2017). These could provide an important view of the early Earth subduction tectonics, but these interpretations still do not reach a consensus. Recent studies on the petrology and geochemistry consider that the start of plate subduction was between 3.5 and 3.0 Ga (e.g., Dhuime et al., 2012; Naeraa et al., 2012; Griffin et al., 2014; Dhuime et al., 2015; Tang et al., 2016; Brown and Johnson, 2018; Smithies et al., 2018; Smit et al., 2019), even though other studies argue that subduction was initiated in the early Archean or Hadean eras (e.g., Harrison, 2009; Hoffman et al., 2011; Polat et al., 2011; Furnes et al., 2014; Komiya et al., 2015; Ernst et al., 2016; Koshida et al., 2016; Greber et al., 2017; Maruyama et al., 2018; Sawada et al., 2018; Liu et al., 2020; Nutman et al., 2020). However, most of them have implications on the basis of some geochemical signatures. In these studies, a high occupancy of mafic rocks on the early Archean crust has often been regarded as an indication of no plate subduction. Instead, plume-related crustal production processes, such as oceanic islands or oceanic plateaus, have been envisaged for the ancient period. Even if plate subduction had started, a small amount of felsic crust would have occurred in the initial period because the first plate subduction should have started between two oceanic plates. For example, in oceanic island arcs like the Izu-Bonin-Mariana (IBM) arc occurring on a subduction zone between the Pacific Plate and Philippine Sea Plate, geophysical observations have detected primitive granitic continental crust with a thickness of over 20 km, in contrast to the basaltic or andesitic islands above sea level (Suyehiro et al., 1996). Most of the geochemical studies listed above mainly reflect the composition of materials in limited parts of the crust or mantle where the initiation of plate subduction is difficult to judge. Therefore, the discussion of subduction initiation is still inconclusive.
Limited plate subduction in the early Archean era has been suggested by estimating the buoyant oceanic plates because of the thick basaltic crust, approximately five times thicker than the present crust (Davies, 1992; Davies et al., 1995), or the highly depleted and mechanically strong peridotitic lithosphere (Davaille and Jaupart, 1993; Solomatov, 1995). Under a tectonic regime without plate subduction, a single plate referred to as the “stagnant-lid” covered the surface of the Earth, and crust formation was caused only by mantle plume upwelling (Solomatov, 1995). Recent numerical simulations of mantle convection combined with crustal production processes suggested a slightly more complex model of “lid-plume tectonics,” wherein the intermittent sagging and dropping of the mafic crust into the mantle is assumed, known as sagduction (Sizova et al., 2010; Thébaut and Rey, 2013). They considered that Archean felsic continental crusts were generated through the melting of the mafic crust sagging into the mantle. Sagduction was originally proposed by the geological observation of Archean granite-greenstone terranes (Gorman et al., 1978; Goodwin and Smith, 1980). The similarity between the sagduction model for the Archean granite-greenstone terranes and geosyncline model for Pacific-type orogenic belts should be noted, which was predominant as a pre-plate tectonics idea (Dewey and Bird, 1970; Isozaki, 1996).
However, the claims against the Archean plate subduction can be refuted by several factors. Firstly, recent modeling of the MOR melting and thermal structure of the lithosphere based on the assumed Archean geotherm suggests that the Archean oceanic lithosphere was dense enough to subduct into the mantle (Weller et al., 2019). Furthermore, the thick mafic crust on the oceanic lithosphere can promote plate subduction because of the mineral phase-change to eclogite, which is denser than the mantle peridotite (Komiya et al., 2002; Komiya et al., 2004). Secondly, most numerical simulations of mantle convection have a serious problem in explaining plate subduction because they adopt a yielding model to express the behavior of the lithosphere (Tackley, 1998; Tackley, 2000). As observed in many geological records of orogenic belts and rifted basins, a fractured lithosphere does not adhere again, and convergence boundaries continue to exist at almost the same position; however, numerical simulations with a yielding model cannot reproduce such features (Ogawa, 2014). Additionally, the Peierls mechanism in rock rheological strength, which is exponentially dependent on stress, enhances the deformation at a significantly lower stress than the rheology with diffusion creep and power-law creep (Tsenn and Carter, 1987; Katayama and Karato, 2008; Demouchy et al., 2013; Azuma et al., 2017). This is presumed to have contributed to promoting plate subduction in the early Earth. Recently, several researchers have pointed out that the first fracture of the stagnant-lid lithosphere, produced by the solidification of the magma ocean, would have been shredded by meteorite impact events and allowed the initiation of subduction (Maruyama et al., 2018; O’Neill et al., 2020).
The petrology of the Archean tonalite-trondhjemite-granodiorite (TTG) series has been discussed with strong relevance to the initiation of plate subduction. The trace element composition of the Archean TTG is depleted of heavy rare earth elements and lacks Eu and Sr anomalies, which reflect the presence of garnet and the lack of plagioclase in the residual material (Barker and Arth, 1976; Smithies et al., 2003; Rapp et al., 2003; Martin et al., 2008). Two types of environments for the Archean TTG magma genesis have been advocated by many researchers: the hot plate subduction zone (Figure 2C; Martin et al., 2008; Hoffman et al., 2011; Nagel et al., 2012; Laurie et al., 2013; Martin et al., 2014; Roman and Arndt, 2020), and mantle plume heating of preexisting thick mafic crust (Figure 2D; Van Karnendonk et al., 2007; Kamber, 2015; Palin et al., 2016; Johnsson et al., 1991). The plume heating model is apparently supported by the computer simulation of the early crust and upper mantle evolution. This model showed a similar mechanism to produce felsic magma during the transition from the stagnant-lid regime to the plate subduction regime (Sizova et al., 2010). The computer simulation is based on the stagnant-lid model for the initial stage of the early Earth and possesses the problems discussed above. Conversely, the plate subduction model for the Archean TTG genesis has the strength to supply hydrated metabasite into the deeper regions where garnet is stable. Presently, it is difficult to definitively reject either model. Both plate subduction and plume heating processes for the TTG genesis may have occurred once in the Archean crust.
To date, there is no consensus on the timing of the start of plate subduction in the Archean or Hadean eras. Ancient plate subduction was probably intertwined with the mantle plume activity (Wyman et al., 2002), which would have been similar to the back-arc spreading activity in the Phanerozoic Earth. Based on the two models of the early Earth tectonic regime, the next chapter discusses how the primitive continental crust, such as the oceanic island arc or hot spot oceanic islands, developed into massive continental blocks.
It has long been postulated that continents in moderate size formed through collision and amalgamation of small continental blocks (de Wit and Hart, 1993; Hoffman, 1988; Hoffman, 1989), but the actual size of these small blocks has not been well understood yet. Based on modern continental blocks and plate margins (Inman and Nordstrom, 1971), this study classifies continental blocks as follows. Class 1 includes ca. 200 km wide continental crusts without large exposure above sea level like the IBM island arc along the Pacific subduction zone or the Hawaiian islands on a hot spot. Class 2 includes ca. 500 km wide continental blocks like the Japanese islands and Greater Sunda islands along the subduction orogenic belts or Iceland on a hot spot. Class 3 includes continental blocks greater than ca. 1,000–3,000 km in width and length like Greenland and Australia. Class 4 continental blocks are above ca. 5,000–10,000 km in width and length like North America. Considering this classification of modern geographic features as a guideline, we discuss the secular change in the size of continents over time.
The modern extant pre-Cambrian terranes can constrain the minimum size of continents at that time, but we need to investigate the actual size of the continents from geological records taking into account later continental break-up. To estimate the land surface area of continental blocks indirectly, terrigenous clastic rocks are useful because their composition and stratigraphy reflect the size of the provenances. Small continental blocks with lengths and widths less than several tens of kilometers yield only immature clastic rocks with compositions close to rocks in the provenance and various grain sizes. Larger continental blocks with lengths and widths of several hundred kilometers can produce clastic rocks comprised of detrital grains sorted by mineral species and grain size through prolonged weathering. On even larger stable continental areas, highly quartzose sandstones are characteristic of the cratonic cover (Dickinson et al., 1983). Subsequent studies, especially those of the Orinoco River in South America, have indicated that tropical weather and low relief topography play major roles in the formation of quartzose sandstone and the size of continents do not relate to its formation directly (Johnsson et al., 1991). Nonetheless, it is important to note that many quartzose sandstones have actually been observed as cratonic cover sequences on large continents. This geological observation is likely related to preservation potential of sedimentary basins. Additionally, the thickness of such quartzose sandstone strata is mainly controlled by tectonic setting. In Proterozoic and Phanerozoic continents of class 3 or 4, several kilometers thick quartzose sandstone strata deposited on continental rift basins and passive continental margins over nearly 100 million years as the continents were large enough to exist stably for a long period of time. Based on these considerations, here we focus on the quartzite sandstone for the estimation of the size of continents in conjunction with lithology of the sedimentary sequences.
Detrital zircon age patterns from sandstones also have the potential to estimate the size of the provenance area. It is well known that the complexity of a detrital zircon age pattern is largely controlled by the topographic features and tectonic setting (Cawood et al., 2012; Aoki et al., 2014). In sedimentary basins on small continental blocks, such as island arcs and oceanic islands, most detrital zircons are derived from young igneous rocks related to arc magmatism, and zircons far older than the depositional age are not found. In contrast, sedimentary basins in rift settings or passive margins on large continents have a supply of detrital zircon from multiple aged crusts in their provenance.
The Rodinia Supercontinent, formed ca. 1.2–0.8 Ga and rifted ca. 0.75–0.6 Ga, is the oldest supercontinent whose paleogeography is well constrained by paleomagnetic analysis and the succession of geological units (Moores, 1991; Karlstrom et al., 2001; Li et al., 2008; Evans, 2009). Although several Proterozoic supercontinents before 1.3 Ga have been proposed such as Columbia (Rogers and Santosh, 2003), Sclavia, Superia and Vaalbara (Bleeker, 2003), the paleogeography and size of the continents are still under debate (e.g., Bradley, 2011; Nance and Murphy, 2013; Piper, 2018). Nonetheless, the 2.0–1.8 Ga shield of the “Nuna continent” is preserved in modern North America and is one of the most substantial records for the existence of a continent above 5,000 km in width and length at least. Moreover, it also provides evidence for the successive growth of the continent size during the Proterozoic era (Hoffman, 1988; Whitemeyer and Karlstrom, 2007). The ∼1.8 Ga middle Proterozoic quartzose sandstone stratum is over 1,000 m thick that occurs worldwide; for example, the ca. 1.7–1.6 Ga Sioux and Barron quartzites in the southern Lake Superior region of North America (Southwick and Mossler, 1984; Holm et al., 1998), ca. 1.8–1.6 Ga Waterberg Supergroup in southern Africa (Meinster and Tickell, 1975; Tankard et al., 1982), the ca. 1.7–1.6 Ga McArthur Group in Australia (Rawlings, 1999; Rawlings et al., 2004; Jackson et al., 2007), and ca. 1.8–1.3 Ga Cuddapah, Kaladgi, and Pranhita-Godavari basins in the Indian Peninsula (Saha et al., 2016). Deposition of these thick sedimentary units is considered to be due to stable large continents during the Proterozoic era.
During the early Proterozoic era, between ca. 2.4 and 1.8 Ga, large-scale sedimentary sequences had formed on many continental rift basins and passive continental margins (Martin et al., 2013). The increased sedimentary sequences caused the great oxygenation of the surface environment of the Earth (Rye and Holland, 1998; Bleeker et al., 1999), and possibly the emergence of eukaryotes (Han and Runnegar, 1992; El Albani et al., 2010; Edou-Minko et al., 2017). The thickness of the quartzose sandstone strata in the continental rift basins and passive continental margins are over 1,000 m and are comparable to those after 1.8 Ga. For example, the 2.4–2.2 Ga Huron Supergroup on the Superior Craton and the Snowy Pass Supergroup on the Wyoming Craton deposited in a broad basin on the passive continental margin contain quartzose sandstone strata that are over several thousand meters thick (Roscoe and Card, 1993). The size of continents during the early Proterozoic period should have been similar to that after 1.8 Ga, i.e., continents of class 4. The continents may correspond to the previously named Sclavia and Superia (Bleeker, 2003) based on age dating and the paleomagnetic analysis of radial dyke swarms. Although the paleogeography of these continents is not well constrained, it is certain that continents as large as those after 1.8 Ga have existed since ca. 2.4 Ga.
Before the ca. 2.5 Ga Archean-Proterozoic boundary, direct geological records for the size of continents are rarely preserved. Some continents larger than the modern-extant Superior Craton, over 1,000 km in width and length, must have existed at ca. 2.7 Ga (late Archean). Other, mostly Archean, cratons have a width and length of 500–1,000 km or less (Figure 1). The presence of Archean quartzose sandstone has been interpreted as an important indicator of cratonic interior on relatively stable large continents at the depositional age (Rogers, 1996; Eriksson et al., 2013; Cawood et al., 2018), even though the origin of Archean quartzose sandstone is subject to debate because the Archean surface environment and climate is different from modern ones (Condie, 1981). The absence of vegetation on the Precambrian land possibly hindered chemical weathering by accelerating the transport of clastic particles (Dott, 2003). On the other hand, a Precambrian CO2-rich atmosphere could have promoted chemical weathering (Sleep and Hessler, 2006). Due to these differences in the continental surface, the conditions for the formation of quartzose sandstone would have been different between the modern and Precambrian environments. In the Archean supracrustal units, we can find both immature non-quartzose sandstones such as graywacke and mature quartzose sandstones as a cratonic cover for the Archean terranes (Condie, 1993). This indicates that the formation of quartzose sandstone requires flat topographic features even under the Archean atmospheric conditions. We proceed on the assumption that the conditions for quartzose sandstone formation were not extremely different from those at present.
Archean quartzose sandstone on a granitic basement with a clear unconformity can be traced back to 3.0 Ga; for example, the ca. 2.6 Ga Moeda Formation of the Minas Supergroup in the Sao Francisco Craton (Minter et al., 1990; Alkmim and Marshak, 1998; Alkmim and Martins-Neto, 2012; Koglin et al., 2014), ca. 2.7 Ga Hardey Formation of the lowermost Fortescue Group in the Pilbara Craton (Thorne and Trendall, 2001; Hall, 2005), ca. 2.8–2.7 Ga Central Slave Cover Group in the Slave Craton (Bleeker et al., 1999), ca. 2.9 Ga Steep Rock, Lumby Lake, Savant Lake, and other greenstone belts of the Superior Craton (Wilks and Nisbet, 1988; Davis and Jackson, 1988; Davis et al., 1988; Donaldson and de Kemp, 1988), ca. 3.0–2.8 Ga Lower and Upper Burawayan greenstone units on the Zimbabwe Craton (Wilson et al., 1995; Fedo et al., 1996; Hunter et al., 1998), ca. 3.0–2.9 Ga Witwatersrand and Pongola groups in the Kaapvaal Craton (Beukes and Cairncross, 1991; Robb and Meyer, 1995; Kositcin and Krapez, 2004), and so on. These quartzose sandstone strata mostly occupy the lowermost part of sedimentary sequences and are shallow marine deposits overlain by pelitic rocks, basaltic-komatiitic lava flow including pillow structure, and banded iron formation (Bleeker, 2003). This characteristic lithology indicates that the sedimentary sequences deposited on the continental rifting basin or passive continental margin. Quartzose sandstones within a similar lithological combination are also found in highly metamorphosed Archean terranes; for example, ca. 3.1 Ga Beit Bridge Group of the Limpopo belt (Eriksson et al., 1988), ca. 3.2 Ga Mt. Narryer complex, Jack Hills belt and Reynard Hills belts on the Yilgarn Craton (Myers, 1988; Spaggiari et al., 2007), ca. 3.2 Ga Beartooth Mountain on the Wyoming Craton (Maier et al., 2012), ca. 3.2–3.0 Ga Bababudan Group in the Dharwar Craton (Srinivasan and Ojakangas, 1986), ca. 3.0 Ga Keonjhar Quartzite in the Singhbhum Craton (Ghosh et al., 2016), ca. 3.0 Ga Naharmagra quartzite of the North Delhi belt in the Aravalli Craton (Raza et al., 2010), and ca. 3.3 Ga Sebakwian quartzite on the Zimbabwe Craton (Bolhar et al., 2017). Older Archean siliceous sandstones also exist, for example, the ca. 3.4 Ga Noisy Formation in the Kaapvaal Craton (de Wit et al., 2011), and ca. 3.5 Ga Mt. Goldworthy quartzite in the Pilbara Craton (Sugitani et al., 2003); however, these quartzose sandstone strata do not have the lithology of ∼ca. 3.3 Ga continental rift basins or passive continental margins. Local hydrothermal silicification is considered to be a major factor for the origin of the ∼ca. 3.3 Ga quartzose sandstone shown above.
It is notable that most of the Archean quartzose sandstone layers are less than a hundred meters thick, in contrast to Proterozoic ones (Figure 3). For example, the basal quartzite of the 2.8 Ga Central Slave Cover Group on the Slave Craton is a typical example, which is less than ∼100 m thick (Bleeker et al., 1999; Figure 3). The Moeda Formation in the lowermost Minas Supergroup is less than 350 m thick (Minter et al., 1990). Most of the other Archean quartzose sandstone strata are similar or thinner than the Moeda Formation (Bleeker, 2001). The change in thickness of quartzose sandstone on continental rift basins or passive continental margins suggests that such basins with continental or shallow marine environments for deposition in the Archean era did not remain for long periods. The short life span of such basins can be explained by the smaller size of continents or thinner continental crust than those after the Proterozoic period. The model of thin continental crust is denied by seismic data of Archean terranes, which indicates that the Archean cratons were nearly 30 km thick since ca. 3 Ga (Drummond, 1988). The smaller size of continents is more plausible for the reason of short life span of the depositional basins because the model can explain the short-lived continental break-up and subsidence. Therefore, the relatively thin ca. 3.3–2.5 Ga quartzose sandstones in the continental rift basin and passive continental margin imply the emergence of relatively stable continental interior environments, but the size of them was still not as large as that of the continents after the Proterozoic era, probably ∼1,000 km in width and length, similar to class 3 continents. The exceptionally thick the ca. 3.0 Ga West Rand Group of the Witwatersrand Supergroup in the Kaapvaal Craton may indicate a slightly larger continent at that time than in the previous and subsequent ages, or that a local continental collision affected to increased sedimentary supply (Burke et al., 1986; Catuneanu, 2001).
FIGURE 3. Stratigraphic columns of representative quartzose sandstone layer from ca. 3.0 Ga to ca. 2.3 Ga. (A) ca. 2.3 Ga Huron Supergroup on the Superior Craton (Roscoe and Card, 1993). (B) ca. 2.6 Ga Minas Supergroup in the Sao Francisco Craton (Minter et al., 1990; Koglin et al., 2014); (C) ca. 2.8 Ga Central Slave Cover Group in the Slave Craton (Bleeker et al., 1999); (D) ca. 3.0 Ga West Rand Group of the Witwatersrand Supergroup in the Kaapvaal Craton (Frimmel, 2010).
Some of the older Archean supracrustal units contain well-classified clastic rocks, for example, the conglomerate in the ca. 3.5 Ga Warawoona Group on the Pilbara Craton (Buick et al., 1995). The well-sorted clastic rock indicates that the size of the continental block at that time was similar to that of class 2 continents. Before ca. 3.4 Ga, the size of the continents might have been less than several hundred-kilometers in width and length, similar to that of classes 1 and 2.
Additionally, detrital zircon age patterns in Archean quartzose sandstone and other clastic rocks are also important geological records for the estimation of the size of continents. The ca. 3.3–2.5 Ga quartzose sandstone and quartzite in the continental rift basin or passive margin show complex detrital zircon age patterns and often contain Eoarhcean and Hadean ones (e.g., Sircombe et al., 2001; Maier et al., 2012; Zeh et al., 2014; Bolhar et al., 2017), indicating that their detrital grains are widely provided from relatively large areas. Furthermore, it is noteworthy that Hadean detrital zircon grains occur in ca. 3.3–3.1 Ga sandstones. Ancient detrital zircon U–Pb ages are extremely rare in clastic rocks with depositional ages younger or even older than ca. 3.3–3.1 Ga (Figure 1). A lesser occurrence of Hadean zircon in clastic rocks younger than 3.0 Ga (late Archean) simply indicates that the Hadean geological units had been destroyed by crustal reworking and recycling by 3.0 Ga. Conversely, no occurrence of Hadean zircon in >3.3 Ga clastic rocks suggests that only small provenances existed because continental blocks were fragmented through rifting and sedimentary basins did not receive detrital grains from Hadean units. The detrital zircon age pattern in Archean rocks would also reflect the secular change in the size of continents.
The secular change in the size of continents estimated above is summarized as follows: 1) mature clastic rocks older than ca. 3.4 Ga have not been found, but ca. 3.5 Ga well-sorted clastic rocks occur in several cratons. This indicates that ca. 3.5–3.4 Ga continental blocks were in scales of several hundred kilometers or less. 2) During ca. 3.3–2.5 Ga (late half of Archean), quartzose sandstone of the continental rift basin or passive margin thinner than a hundred meters occurred on many cratons, indicating that the continents were nearly 1,000 km in width and length or more. 3) After ca. 2.4 Ga, quartzose sandstone strata thicker than 1,000 m are widely deposited worldwide. Since this period, the width or length of continents has been nearly 10,000 km until the present. Figures 4A and B are schematics of the above-described secular change in the occurrence of quartzose sandstone and the size of continents.
FIGURE 4. (A) Schematic diagram of the maximum scale of continental blocks through the history of Earth in Supercontinental periods (Bradley, 2011). (B) Secular trend in the mantle potential temperature estimated through petrological methods (Komiya, 2004; Herzberg et al., 2010).
The secular change in the size of continents is shown in Figure 5 as a global map. Figures 5A and B show the model of the early initiation of plate subduction. After the start of plate subduction in the Hadean and early Archean era, a narrow and thin continental crust formed like that in modern oceanic island arcs (Figure 5A). These primitive continental crusts had amalgamated to form slightly larger continental blocks over 500 km in width by ca. 3.5 Ga at the latest (Figure 5B). In contrast, Figures 5A′ and B′ show the model of limited plate subduction on the early Earth. Early felsic crust was formed in hot spot regions by a mantle plume, dominated by a mafic crust and ∼500 km in diameter (Figure 5A′). After ca. 3.5 Ga initiation of the plate subduction, the plume crusts had amalgamated to form felsic continental blocks (Figure 5B′). In any case, the primitive continental crust had further amalgamated and the first continents with a width greater than 1,000 km, larger than modern Greenland or Australia, appeared around ca. 3.3 Ga. The Archean continents repeatedly rifted and collided and had been smaller in size than those after the Proterozoic. A small peak in the size of continents might have occurred at ca. 3.2–3.0 Ga, as suggested by thick quartzose sandstone strata with eolian structures. The continents were likely rifted into smaller blocks after 3.0 Ga, which was related to the late Archean peak in the mantle potential temperature (Komiya, 2004; Herzberg et al., 2010; Figure 4C). By ca. 2.4 Ga, the continental blocks further amalgamated to form larger continents greater than 5,000 km, like modern North America. The stabilization of large continents during the early Proterozoic era was also possibly related to the decreased mantle temperature (Höning and Spohn, 2016).
FIGURE 5. Map view of secular change in the size of continents modified after Sawada et al. (2018). (A) After the start of the plate subduction, many narrow and thin oceanic island arc-like continental crust were produced. (B) Through the amalgamation of the narrow, thin continental crust, evolved continental blocks with a width of over 500 km. Old Hadean and early Archean crusts had been preserved in the interior of the continental blocks. (A′) Plate subduction was still limited and the thin felsic crust was conceived in plume-related mafic crust. (B′) After the start of plate subduction, the plume crust gradually amalgamated and larger continental blocks emerged. Production of arc crust likely started. (C) Larger continents with over a 1,000 km width appeared around 3.3 Ga. Most of the continents during this period were not as large as the modern ones, although some exceptions with nearly a 5,000 km width likely existed during 3.2–3.0 Ga. (D) The Archean continents finally amalgamated to form large continents similar to modern ones.
This study attempts to estimate the secular change in the size of continents by using limited information, mainly from the occurrence of quartzose sandstones. However, this estimation is still crude, and the size of continents before ca. 1.8 Ga is not well constrained. Unfortunately, most parameters in the petrology and geochemistry of igneous and metamorphic rocks and their minerals do not contain any information on the length, area, or volume, but only on the temperature, pressure, time, and the relative amount of fractionation. To obtain physical parameters related to the size of continents, more investigation on the Archean clastic sedimentary rocks is required.
Further information about the size of continents can be obtained from the U-Pb age pattern of detrital mineral grains because it can reflect the age structure of the provenance area on a continent. A compilation of detrital zircon ages and isotopic data were undertaken by combining large datasets and investigating the peaks in the compiled datasets (e.g., Condie et al., 2009; Belousova et al., 2010; Roberts and Spencer, 2015). However, such data compilations, without checking the depositional ages of the host clastic rocks, would obtain superimposed signals of the production and destruction of the continental crust over time, resulting in a misunderstanding of the true evolution of continents. To disclose the secular change in the age distribution pattern of detrital zircon grains, the age structure of continents, evolution of continents, and time-lapse analysis of the detrital zircon age pattern are important (Parman, 2015; Spencer et al., 2017; Sawada et al., 2018; Puetz and Condie, 2019). The differences in detrital zircon age patterns have the potential to determine the size of continents, especially during the early period in which geological units and structures are poorly preserved. Although a perfect correspondence between the size of continents and detrital zircon age patterns is not always achieved, it is possible to detect the general trend in the size of continents by using a large dataset. Detrital zircon Lu-Hf isotopic studies to estimate crustal reworking through time should be reexamined because Hf isotopic values are likely affected by the mineral composition of residual rocks during the partial melting and crustal assimilation processes according to recent detailed zircon analysis combined with geological and petrological observations (Chen et al., 2015; Petersson et al., 2019). Trace element analyses of zircon combined with Lu-Hf isotopic analysis could help in solving this problem as they reflect the parental magmatic composition and residual mineral assemblage well (Grimes et al., 2015). The detrital monazite U-Pb age also has the potential to reveal the crustal evolution, especially by metamorphism during the orogeny (Itano et al., 2016; 2018). Other minerals, such as apatite, titanite, allanites, and garnet also have the potential to contribute to our understanding of the early Earth. However, they are challenging to use for Archean detrital studies because of the high content of initial Pb and weaknesses for alteration (e.g., Chen et al., 2015; Seman et al., 2017). Presently, it is not easy to prelaunch the success of the massive chronological data by minerals other than zircon.
This study used the quartzose sandstone strata for the estimation of the size of continents, but the correlation between the occurrences and size of continents was not always perfect because of the intense chemical weathering caused by the Precambrian atmosphere. The sedimentary structures of the Archean sandstone strata depend on the preservation of the sedimentary basin in the Archean terranes. In contrast, the geochemistry of clastic sedimentary rocks is worth exploring. For example, by using whole-rock Zr/Sc vs. Th/Sc plots for modern turbidites, McLennan et al. (1990) pointed out that relatively hard zircon is more concentrated in sediments along passive continental margins than those along active margins. This is because the narrow and limited provenance along active margins allow the composition of clastic rocks to be close to igneous rocks, whereas clastic rocks on wide and large provenances along passive margins are strongly affected by sedimentary sorting. Likewise, it is possible to detect the size of the provenance from a whole-rock composition of clastic rocks, especially from relatively coarse-grained ones such as sandstones. Based on the sedimentary structure and mineralogical-chemical composition of relatively young, well-preserved Phanerozoic and Proterozoic quartzose sandstone and quartzite, chemical proxies need to be developed and applied to Archean ones. These expected chemical proxies should be applicable for metamorphosed and fragmented Archean quartzite, and more information for older continents can be obtained.
In the above estimation of the size of continents, some significant difficulties still exist in obtaining the actual evolutionary history of the solid Earth. Constraints on the amount of continental crust produced through magmatism and recycled into the mantle has been a major issue for solid Earth studies. Due to the small and thin continental crust in the initial stage of the plate subduction, like that of modern oceanic island arcs or hot spot islands, most of the primitive continental crust should have been subducted to the mantle without any detectable geological records (Santosh et al., 2009; Yamamoto et al., 2009; Spencer et al., 2017; Sawada et al., 2018). Although some geochemical signatures of subducted continental crust have been found from modern hot spot lavas (Jackson et al., 2007; Workman et al., 2008; Willbold and Stracke, 2010), it seems almost impossible to estimate the total amount and influx of the subducted continental crust during the Hadean and Archean periods through conventional, petrological, or geochemical methods for geological samples. To understand the surficial environments and the interior of the early Earth, more realistic computer simulations of the crust–mantle evolution are required. Modeling of the mantle convection in a 3D spherical shell is necessary, which is free from significant approximation and simplification, and is able to reproduce plate subduction based on the significant contrast of viscosity. Through these simulations, many parameters can be calculated, such as the speed of plate subduction, size and life span of plates, production and destruction rates of new continental crust, and movement and amalgamation of the generated continental crust. Geochemical and petrological studies can determine some of these parameters and evaluate the results of computer simulations. Combined with numerous petrological and geochemical data reported previously, estimating the size of continents must be relevant in the near future, and research on coarse-grained clastic sedimentary rocks can offer a solution for this purpose.
This paper reviewed the crustal development in the early Earth and the current understanding of the size of continents over time. The size of continents is emphasized as an essential parameter to determine the geotectonic evolution of the continental crust. Clastic sedimentary rocks are possibly the key for estimating the size of continents because their composition reflects the physical processes occurring on the continental surface, such as transportation to the depositional basin. The present paper summarizes the tentatively estimated secular changes in the size of continents in the Archean and Proterozoic eras based on the occurrence of quartzose sandstones, which indicate a high degree of mineral sorting. We can trace back the existence of massive continents with widths and lengths of nearly 10,000 km like modern North America to ca. 2.4–2.3 Ga. The first continents, which were over 1,000 km in width and length, appeared at ca. 3.3 Ga. Most of the Archean continents were still not as large as those after ca. 2.4 Ga. Further analyses of clastic rocks can enable a more quantitative estimation of the size of continents, which can contribute to understanding the geotectonic history of the early Earth.
The first author, HS, constructed the discussion and interpretation of the data and participated in the preparation of the manuscript.
This work was supported by JSPS Grants-in-Aid for Scientific Research (Numbers 17J07214, and 19K23458) from the Japanese Ministry of Education, Science, Sports, Technology, and Culture.
The author declares that the research was conducted in the absence of any commercial or financial relationships that could be construed as a potential conflict of interest.
Constructive discussion on this paper by Prof. Yukio Isozaki and Prof. Shigenori Maruyama was greatly appreciated. Comments from the editor and reviewers were helpful into improving this study. Many thanks to Editage (www.editage.com) for English language editing.
Abbott, D., Drury, R., and Smith, W. H. (1994). Flat to steep transition in subduction style. Geology 22, 937–940. doi:10.1130/0091-7613(1994)022
Alkmim, F. F., and Marshak, S. (1998). Transamazonian orogeny in the southern SãoFrancisco craton region, Minas Gerais, Brazil: evidence for paleoproterozoiccollision and collapse in the quadrilátero Ferrífero. Precambrian Res. 90, 29–58. doi:10.1016/S0301-9268(98)00032-1
Alkmim, F. F., and Martins-Neto, M. A. (2012). Proterozoic first-order sedimentary sequences of the São Francisco craton, eastern Brazil. Mar. Petrol. Geol. 33, 127–139. doi:10.1016/j.marpetgeo.2011.08.011
Allègre, C. J., and Luck, J. M. (1980). Osmium isotopes as petrogenetic and geological tracers. Earth Planet Sci. Lett. 48, 148–154. doi:10.1016/0012-821X(80)90177-6
Angus, D. A., Kendall, J. M., Wilson, D. C., White, D. J., Sol, S., and Thomson, C. J. (2009). Stratigraphy of the Archean western Superior Province from P-and S-wave receiver functions: further evidence for tectonic accretion? Phys. Earth Planet. In. 177, 206–216. | doi:10.1016/j.pepi.2009.09.002
Aoki, K., Isozaki, Y., Kofukuda, D., Sato, T., Yamamoto, A., Maki, K., et al. (2014). Provenance diversification within an arc‐trench system induced by batholith development: the Cretaceous Japan case. Terra Nova 262, 139–149. doi:10.1111/ter.12080
Armstrong, R. (1981). Radiogenic isotopes: the case for crustal recycling on a near-steady-state no-continental-growth Earth. Phil. Trans. Roy. Soc. Lond. A:301, 443–472. doi:10.1098/rsta.1981.0122
Azuma, S., Yamamoto, S., Ichikawa, H., and Maruyama, S. (2017). Why primordial continents were recycled to the deep: role of subduction erosion. Geosci. Front. 8, 337–346. doi:10.1016/j.gsf.2016.08.001
Barker, F., and Arth, J. G. (1976). Generation of trondhjemitic-tonalitic liquids and Archean bimodal trondhjemite-basalt suites. Geology 4, 596–600.: doi:10.1130/0091-7613(1976)4<596:GOTLAA>2.0.CO;2
Belousova, E. A., Kostitsyn, Y. A., Griffin, W. L., Begg, G. C., O'Reilly, S. Y., and Pearson, N. J. (2010). The growth of the continental crust: constraints from zircon Hf-isotope data. Lithos 1193, 457–466. doi:10.1016/j.lithos.2010.07.024
Beukes, B., and Cairncross, B. (1991). A lithostratigraphic-sedimentological reference profile for the late Archaean Mozaan group, Pongola sequence: application to sequence stratigraphy and correlation with the Witwatersrand Supergroup. S. Afr. J. Geol. 94, 44–69.
Bleeker, W., Ketchum, J. W., Jackson, V. A., and Villeneuve, M. E. (1999). The Central Slave Basement Complex, Part I: its structural topology and autochthonous cover. Can. J. Earth Sci. 36, 1083–1109. doi:10.1139/e98-102
Bleeker, W. (2003). The late Archean record: a puzzle in ca. 35 pieces. Lithos 71, 99–134. doi:10.1016/j.lithos.2003.07.003
Blewett, R. S., Czarnota, K., and Henson, P. A. (2010). Structural-event framework for the eastern Yilgarn Craton, Western Australia, and its implications for orogenic gold. Precambrian Res. 183, 203–229. doi:10.1016/j.precamres.2010.04.004
Bolhar, R., Hofmann, A., Kemp, A. I., Whitehouse, M. J., Wind, S., and Kamber, B. S. (2017). Juvenile crust formation in the Zimbabwe Craton deduced from the O-Hf isotopic record of 3.8–3.1 Ga detrital zircons. Geochim. Cosmochim. Acta 215, 432–446. doi:10.1016/j.gca.2017.07.008
Bradley, D. C. (2011). Secular trends in the geologic record and the supercontinent cycle. Earth Sci. Rev. 108, 16–33. doi:10.1016/j.earscirev.2011.05.003
Brenner, A. R., Fu, R. R., Evans, D. A., Smirnov, A. V., Trubko, R., and Rose, I. R. (2020). Paleomagnetic evidence for modern-like plate motion velocities at 3.2 Ga. Sci. Adv. 6, eaaz8670. doi:10.1126/sciadv.aaz8670
Brown, M., and Johnson, T. (2018). Secular change in metamorphism and the onset of global plate tectonics. Am. Mineral. 103, 181–196. doi:10.2138/am-2018-6166
Buick, R., Thornett, J. J., McNaughton, N. J., Smith, J. B., Barley, M. E., and Savage, M. (1995). Record of emergent continental crust ∼3.5 billion years ago in the Pilbara craton of Australia. Nature 375, 574‒577. doi:10.1038/375574a0
Burke, K., Kidd, W. S. F., and Kusky, T. M. (1986). Archean foreland basin tectonics in the Witwatersrand, South Africa. Tectonics 5, 439–456. doi:10.1029/TC005i003p00439
Byerly, B. L., Lowe, D. R., Drabon, N., Coble, M. A., Burns, D. H., and Byerly, G. R. (2018). Hadean zircon from a 3.3 Ga sandstone, Barberton greenstone belt, South Africa. Geolog. 46, 967–970. doi:10.1130/G45276.1
Catuneanu, O. (2001). Flexural partitioning of the late Archaean Witwatersrand foreland system, South Africa. Sediment. Geol. 141, 95–112. doi:10.1016/S0037-0738(01)00070-7
Cavosie, A. J., Valley, J. W., and Wilde, S. A. (2007). The oldest terrestrial mineral record: a review of 4400 to 4000 Ma detrital zircons from Jack Hills, Western Australia. Develop. Precambrian Geol. 15, 91–111. doi:10.1016/S0166-2635(07)15025-8
Cawood, P. A., and Hawkesworth, C. J. (2019). Continental crustal volume, thickness and area, and their geodynamic implications. Gondwana Res. 66, 116–125. | doi:10.1016/j.gr.2018.11.001
Cawood, P. A., Hawkesworth, C. J., and Dhuime, B. (2012). Detrital zircon record and tectonic setting. Geology 40, 875–878. doi:10.1130/G32945.1
Cawood, P. A., Hawkesworth, C. J., Pisarevsky, S. A., Dhuime, B., Capitanio, F. A., and Nebel, O. (2018). Geological archive of the onset of plate tectonics. Phil. Trans. Math. Phys. Eng. Sci. 376, 20170405. | doi:10.1098/rsta.2017.0405
Chen, Y. X., Gao, P., and Zheng, Y. F. (2015). The anatectic effect on the zircon Hf isotope composition of migmatites and associated granites. Lithos 238, 174–184. doi:10.1016/j.lithos.2015.09.026
Cheng, Q. (2017). Singularity analysis of global zircon U-Pb age series and implication of continental crust evolution. Gondwana Res. 51, 51–63. doi:10.1016/j.gr.2017.07.011
Clift, P. D., Vannucchi, P., and Morgan, J. P. (2009). Crustal redistribution, crust–mantle recycling and Phanerozoic evolution of the continental crust. Earth Sci. Rev. 971, 80–104. doi:10.1016/j.earscirev.2009.10.003
Compston, W., and Pidgeon, R. T. (1986). Jack Hills, evidence of more very old detrital zircons in Western Australia. Nature 321, 766–769. doi:10.1038/321766a0
Condie, K. C., Belousova, E., Griffin, W. L., and Sircombe, K. N. (2009). Granitoid events in space and time: constraints from igneous and detrital zircon age spectra. Gondwana Res. 153, 228–242. doi:10.1016/j.gr.2008.06.001
Condie, K. C. (1993). Chemical composition and evolution of the upper continental crust: contrasting results from surface samples and shales. Chem. Geol. 104, 1–37. doi:10.1016/0009-2541(93)90140-E
Cui, P. L., Sun, J. G., Sha, D. M., Wang, X. J., Zhang, P., Gu, A. L., et al. (2013). Oldest zircon xenocryst (4.17 Ga) from the north China craton. Int. Geol. Rev. 55, 1902–1908. doi:10.1080/00206814.2013.805925
Czarnota, K., Champion, D. C., Goscombe, B., Blewett, R. S., Cassidy, K. F., Henson, P. A., et al. (2010). Geodynamics of the eastern Yilgarn craton. Precambrian Res. 183, 175–202. doi:10.1016/j.precamres.2010.08.004
Davaille, A., and Jaupart, C. (1993). Transient high-Rayleigh-number thermal convection with large viscosity variations. J. Fluid Mech. 253, 141–166. doi:10.1017/S0022112093001740
Davies, G. F. (1992). On the emergence of plate tectonics. Geology 20, 963–966. doi:10.1130/0091-7613(1992)020<0963:OTEOPT>2.3.CO;2
Davies, G. F. (1995). Punctuated tectonic evolution of the earth. Earth Planet Sci. Lett. 136, 363–379. doi:10.1016/0012-821x(95)00167-b
Davis, D. W., and Jackson, M. C. (1988). Geochronology of the Lumby Lake greenstone belt: a 3 Ga complex within the Wabigoon Subprovince, northwest Ontario. Geol. Soc. Am. Bull. 100, 818–824. doi:10.1130/0016-7606(1988)100<0818:GOTLLG>2.3.CO;2
Davis, D. W., Sutcliffe, R. H., and Trowell, N. F. (1988). Geochronological constraints on the tectonic evolution of a late Archaean greenstone belt, Wabigoon Subprovince, Northwest Ontario, Canada. Precambrian Res. 39, 171–191. doi:10.1016/0301-9268(88)90041-1
de Wit, M. J. (1998). On Archean granites, greenstones, cratons and tectonics: does the evidence demand a verdict? Precambrian Res. 91, 181–226. doi:10.1016/S0301-9268(98)00043-6
de Wit, M. J., Furnes, H., and Robins, B. (2011). Geology and tectonostratigraphy of the Onverwacht suite, Barberton greenstone belt, South Africa. Precambrian Res. 186, 1–27. doi:10.1016/j.precamres.2010.12.007
de Wit, M. J., and Hart, R. A. (1993). Earth's earliest continental lithosphere, hydrothermal flux and crustal recycling. Lithos 303, 309–335. doi:10.1016/0024-4937(93)90043-c
Demouchy, S., Tommasi, A., Ballaran, T. B., and Cordier, P. (2013). Low strength of Earth’s uppermost mantle inferred from tri-axial deformation experiments on dry olivine crystals. Phys. Earth Planet. In. 220, 37–49. doi:10.1016/j.pepi.2013.04.008
Dewey, J. F., and Bird, J. M. (1970). Plate tectonics and geosynclines. Tectonophysics 10, 625–638. doi:10.1016/0040-1951(70)90050-8
Dewey, J. F., and Windley, B. F. (1981). Growth and differentiation of the continental crust. Phil. Trans. Roy. Soc. Lond. 301, 189–206.
Dhuime, B., Hawkesworth, C. J., Cawood, P. A., and Storey, C. D. (2012). A change in the geodynamics of continental growth 3 billion years ago. Science 335, 1334–1336. doi:10.1126/science.1216066
Dhuime, B., Hawkesworth, C. J., Delavault, H., and Cawood, P. A. (2018). Rates of generation and destruction of the continental crust: implications for continental growth. Phil. Trans. Math. Phys. Eng. Sci. 376, 20170403. doi:10.1098/rsta.2017.0403
Dhuime, B., Wuestefeld, A., and Hawkesworth, C. J. (2015). Emergence of modern continental crust about 3 billion years ago. Nat. Geosci. 8, 552–555. doi:10.1038/ngeo2466
Dickinson, W. R., Beard, L. S., Brakenridge, G. R., Erjavec, J. L., Ferguson, R. C., Inman, K. F., et al. (1983). Provenance of North American Phanerozoic sandstones in relation to tectonic setting. Geol. Soc. Am. Bull. 94, 222–235. doi:10.1130/0016-7606(1983)94<222:PONAPS>2.0.CO;2
Diwu, C., Sun, Y., Wilde, S. A., Wang, H., Dong, Z., Zhang, H., and Wang, Q. (2013). New evidence for∼ 4.45 Ga terrestrial crust from zircon xenocrysts in Ordovician ignimbrite in the North Qinling Orogenic Belt, China. Gondwana Res. 23, 1484–1490. doi:10.1016/j.gr.2013.01.001
Donaldson, J. A., and de Kemp, E. A. (1998). Archaean quartz arenites in the Canadian shield: examples from the superior and Churchill provinces. Sediment. Geol. 120, 153–176. doi:10.1016/S0037-0738(98)00031-1
Dorr, J. V. N. (1969). Physiographic, stratigraphic and structural development of the Quadrilátero Ferrífero, Minas Gerais, Brazil, U.S. Geological Survey Professional Paper, 641-A, 110.
Dott, R. H. (2003). The importance of eolian abrasion in supermature quartz sandstones and the paradox of weathering on vegetation-free landscapes. J. Geol. 111, 387–405. doi:10.1086/375286
Drummond, B. J. (1988). A review of crust/upper mantle structure in the Precambrian areas of Australia and implications for Precambrian crustal evolution. Precambrian Res. 40, 101–116. doi:10.1016/0301-9268(88)90063-0
Edou-Minko, A., Moussavou, M., Sato, T., Sawaki, Y., Ondo, S. N., Maire, R., et al. (2017). Growth, duplication and lateral mutual compressive deformation of Akouemma hemisphaeria on the Seafloor of Okondja Basin at 2.2 Ga (Gabon). Int. J. Geosci. 8, 1172. doi:10.4236/ijg.2017.89067
El Albani, A., Bengtson, S., Canfield, D. E., Bekker, A., Macchiarelli, R., Mazurier, A., et al. (2010). Large colonial organisms with coordinated growth in oxygenated environments 2.1 Gyr ago. Nature 466, 100. doi:10.1038/nature09166
Eriksson, K. A., Kidd, W. S., and Krapez, B. (1988). “Basin analysis in regionally metamorphosed and deformed early Archean terrains: examples from southern Africa and Western Australia,” in New perspectives in basin analysis. Berlin: Springer, 371–404.
Eriksson, P. G., Banerjee, S., Catuneanu, O., Corcoran, P. L., Eriksson, K. A., Hiatt, E. E., et al. (2013). Secular changes in sedimentation systems and sequence stratigraphy. Gondwana Res. 24, 468–489. doi:10.1016/j.gr.2012.09.008
Ernst, W. G. (2009). Archean plate tectonics, rise of Proterozoic supercontinentality and onset of regional, episodic stagnant-lid behavior. Gondwana Res. 15, 243–253. doi:10.1016/j.gr.2008.06.010
Ernst, W. G. (2017). Earth’s thermal evolution, mantle convection, and Hadean onset of plate tectonics. J. Asian Earth Sci. 145, 334–348. doi:10.1016/j.jseaes.2017.05.037
Ernst, W. G., Sleep, N. H., and Tsujimori, T. (2016). Plate-tectonic evolution of the Earth: bottom-up and top-down mantle circulation. Can. J. Earth Sci. 53, 1103–1120. doi:10.1139/cjes-2015-0126
Evans, D. A. (2009). The palaeomagnetically viable, long-lived and all-inclusive Rodinia supercontinent reconstruction. Geol. Soc., Lond., Special Publications 327, 371–404. doi:10.1144/sp327.16
Fedo, C. M., Eriksson, K. A., and Krogstad, E. J. (1996). Geochemistry of shales from the Archean (∼ 3.0 Ga) Buhwa Greenstone Belt, Zimbabwe: implications for provenance and source-area weathering. Geochim. Cosmochim. Acta 60, 1751–1763. doi:10.1016/0016-7037(96)00058-0
Frimmel, H. (2010). “The Witwatersrand basin and its gold deposits,” in The Archaean geology of the Kaapvaal craton, southern Africa. Berlin: Springer, 255–275.
Froude, D. O., Ireland, T. R., Kinny, P. D., Williams, I. S., Compston, W., Williams, I. T., et al. (1983). Ion microprobe identification of 4,100–4,200 Myr-old terrestrial zircons. Nature 304, 616. doi:10.1038/304616a0
Furnes, H., De Wit, M., and Dilek, Y. (2014). Four billion years of ophiolites reveal secular trends in oceanic crust formation. Geosci. Front. 5, 571–603. doi:10.1016/j.gsf.2014.02.002
Fyfe, W. S. (1978). The evolution of the Earth's crust: modern plate tectonics to ancient hot spot tectonics? Chem. Geol. 23, 89–114. doi:10.1016/0009-2541(78)90068-2
Ghosh, S., De, S., and Mukhopadhyay, J. (2016). Provenance of> 2.8 Ga Keonjhar quartzite, Singhbhum craton, eastern India: implications for the nature of Mesoarchean upper crust and geodynamics. J. Geol. 124, 331–351. doi:10.1086/685862
Goodwin, A. M., and Smith, I. E. M. (1980). Chemical discontinuities in Archean metavolcanic terrains and the development of Archean crust. Precambrian Res. 10, 301–311. doi:10.1016/0301-9268(80)90016-9
Gorman, B. E., Pearce, T. H., and Birkett, T. C. (1978). On the structure of Archean greenstone belts. Precambrian Res. 6, 23–41. doi:10.1016/0301-9268(78)90053-0
Goscombe, B., Foster, D. A., Blewett, R., Czarnota, K., Wade, B., Groenewald, B., et al. (2019). Neoarchaean metamorphic evolution of the Yilgarn Craton: a record of subduction, accretion, extension and lithospheric delamination. Precambrian Res., 105441. doi:10.1016/j.precamres.2019.105441
Greber, N. D., Dauphas, N., Bekker, A., Ptáček, M. P., Bindeman, I. N., and Hofmann, A. (2017). Titanium isotopic evidence for felsic crust and plate tectonics 3.5 billion years ago. Science 357, 1271–1274. doi:10.1126/science.aan8086
Griffin, W. L., Belousova, E. A., O'Neill, C., O'Reilly, S. Y., Malkovets, V., Pearson, N. J., et al. (2014). The world turns over: Hadean–Archean crust–mantle evolution. Lithos 189, 2–15. doi:10.1016/j.lithos.2013.08.018
Griffin, W. L., Belousova, E. A., Shee, S. R., Pearson, N. J., and O’reilly, S. Y. (2004). Archean crustal evolution in the northern Yilgarn Craton: U–Pb and Hf-isotope evidence from detrital zircons. Precambrian Res. 131, 231–282. doi:10.1016/j.precamres.2003.12.011
Grimes, C. B., Wooden, J. L., Cheadle, M. J., and John, B. E. (2015). “Fingerprinting” tectono-magmatic provenance using trace elements in igneous zircon. Contrib. Mineral. Petrol. 170, 46. doi:10.1007/s00410-015-1199-3
Hall, C. E. (2005). SHRIMP U–Pb depositional age for the lower Hardey Formation: evidence for diachronous deposition of the lower Fortescue Group in the southern Pilbara region. Western Australia 52, 403–410. doi:10.1080/08120090500134506
Han, T. M., and Runnegar, B. (1992). Megascopic eukaryotic algae from the 2.1-billion-year-old Negaunee iron-formation, Michigan. Science 257, 232–235. doi:10.1126/science.1631544
Harrison, T. M. (2009). The Hadean crust: evidence from> 4 Ga zircons. Annu. Rev. Earth Planet Sci. 37, 479–505. doi:10.1146/annurev.earth.031208.100151
Hastie, A. R., Fitton, J. G., Bromiley, G. D., Butler, I. B., and Odling, N. W. (2016). The origin of Earth’s first continents and the onset of plate tectonics. Geology 44, 855–858. doi:10.1130/G38226.1
Hawkesworth, C., Cawood, P., Kemp, T., Storey, C., and Dhuime, B. (2009). A matter of preservation. Science 323, 49–50. doi:10.1126/science.1168549
Herzberg, C., Condie, K., and Korenaga, J. (2010). Thermal history of the Earth and its petrological expression. Earth Planet. Sci. Lett. 292, 79–88. doi:10.1016/j.epsl.2010.01.022
Hirata, T., and Nesbitt, R. W. (1995). U-Pb isotope geochronology of zircon: Evaluation of the laser probe-inductively coupled plasma mass spectrometry technique. Geochem. Cosmochim. Acta 59, 2491–2500. doi:10.1016/0016-7037(95)00144-1
Hoffman, P. F. (1989). “Precambrian geology and tectonic history of North America,” in The geology of North America—an overview (Boulder: Geological Society of America), 447–512.
Hoffman, P. F. (1988). United Plates of America, the birth of a craton-early Proterozoic assembly and growth of Laurentia. Annu. Rev. Earth Planet Sci. 16, 543–603. doi:10.1146/annurev.ea.16.050188.002551
Hoffmann, J. E., Münker, C., Næraa, T., Minik, T. R., Herwartz, D., Garbe-Schönberg, D., et al. (2011). Mechanisms of Archean crust formation inferred from high-precision HFSE systematics in TTGs. Geochim. Cosmochim. Acta 75, 4157–4178. doi:10.1016/j.gca.2011.04.027
Holm, D., Schneider, D., and Coath, C. D. (1998). Age and deformation of Early Proterozoic quartzites in the southern Lake Superior region: implications for extent of foreland deformation during final assembly of Laurentia. Geology 26, 907–910. doi:10.1130/0091-7613(1998)026<0907:AADOEP>2.3.CO;2
Höning, D., and Spohn, T. (2016). Continental growth and mantle hydration as intertwined feedback cycles in the thermal evolution of Earth. Phys. Earth Planet. In. 255, 27–49. doi:10.1016/j.pepi.2016.03.010
Hunter, M. A., Bickle, M. J., Nisbet, E. G., Martin, A., and Chapman, H. J. (1998). Continental extensional setting for the Archean Belingwe greenstone belt, Zimbabwe. Geology 26, 883–886. doi:10.1130/0091-7613(1998)026<0883:CESFTA>2.3.CO;2
Hurley, P. M., and Rand, J. R. (1969). Pre-drift continental nuclei. Science 164, 1229–1242. doi:10.1126/science.164.3885.1229
Iizuka, T., Horie, K., Komiya, T., Maruyama, S., Hirata, T., Hidaka, H., et al. (2006). 4.2 Ga zircon xenocryst in an Acasta gneiss from northwestern Canada: evidence for early continental crust. Geology 34, 245–248. doi:10.1130/G22124.1
Inman, D. L., and Nordstrom, C. E. (1971). On the tectonic and morphologic classification of coasts. J. Geol. 79, 1–21. doi:10.1086/627583
Isozaki, Y. (1996). Anatomy and genesis of a subduction‐related orogen: a new view of geotectonic subdivision and evolution of the Japanese Islands. Isl. Arc 5, 289–320. doi:10.1111/j.1440-1738.1996.tb00033.x
Itano, K., Iizuka, T., Chang, Q., Kimura, J. I., and Maruyama, S. (2016). U–Pb chronology and geochemistry of detrital monazites from major African rivers: constraints on the timing and nature of the Pan-African Orogeny. Precambrian Res. 282, 139–156. doi:10.1016/j.precamres.2016.07.008
Itano, K., Iizuka, T., and Hoshino, M. (2018). REE-Th-U and Nd isotope systematics of monazites in magnetite-and ilmenite-series granitic rocks of the Japan arc: implications for its use as a tracer of magma evolution and detrital provenance. Chem. Geol. 484, 69–80. doi:10.1016/j.chemgeo.2017.11.033
Jackson, M. G., Hart, S. R., Koppers, A. A., Staudigel, H., Konter, J., Blusztajn, J., Kurl, M., et al. (2007). The return of subducted continental crust in Samoan lavas. Nature 448 (7154), 684–687. doi:10.1038/nature06048
Johnsson, M. J., Stallard, R. F., and Lundberg, N. (1991). Controls on the composition of fluvial sands from a tropical weathering environment: sands of the Orinoco River drainage basin, Venezuela and Colombia. Geol. Soc. Am. Bull. 103, 1622–1647. doi:10.1130/0016-7606(1991)103<1622:COTCOF>2.3.CO;2
Kamber, B. S. (2015). The evolving nature of terrestrial crust from the Hadean, through the Archaean, into the Proterozoic. Precambrian Res. 258, 48–82. doi:10.1016/j.precamres.2014.12.007
Karlstrom, K. E., Åhäll, K. I., Harlan, S. S., Williams, M. L., McLelland, J., and Geissman, J. W. (2001). Long-lived 1.8–1.0 Ga convergent orogen in southern Laurentia, its extensions to Australia and Baltica, and implications for refining Rodinia. Precambrian Res. 1111, 5–30. doi:10.1016/S0301-9268(01)00154-1
Katayama, I., and Karato, S. I. (2008). Low-temperature, high-stress deformation of olivine under water-saturated conditions. Phys. Earth Planet. In. 168, 125–133. doi:10.1016/j.pepi.2008.05.019
Knudsen, T. L., Griffin, W., Hartz, E., Andresen, A., and Jackson, S. (2001). In-situ hafnium and lead isotope analyses of detrital zircons from the Devonian sedimentary basin of NE Greenland: a record of repeated crustal reworking. Contrib. Mineral. Petrol. 141, 83–94. doi:10.1007/s004100000220
Koglin, N., Zeh, A., Cabral, A. R., Gomes, A. A. S., Neto, A. V. C., Brunetto, W. J., et al. (2014). Depositional age and sediment source of the auriferous Moeda Formation, Quadrilátero Ferrífero of Minas Gerais, Brazil: new constraints from U–Pb–Hf isotopes in zircon and xenotime. Precambrian Res. 255, 96–108. doi:10.1016/j.precamres.2014.09.010
Komiya, T. (2011). Continental recycling and true continental growth. Russ. Geol. Geophys. 52 (12), 1516–1529. doi:10.1016/j.rgg.2011.11.001
Komiya, T. (2004). Material circulation model including chemical differentiation within the mantle and secular variation of temperature and composition of the mantle. Phys. Earth Planet. Int. 146, 333–367. doi:10.1016/j.pepi.2003.03.001
Komiya, T., Hayashi, M., Maruyama, S., and Yurimoto, H. (2002). Intermediate-P/T type Archean metamorphism of the Isua supracrustal belt: implications for secular change of geothermal gradients at subduction zones and for Archean plate tectonics. Am. J. Sci. 302, 806–826. doi:10.2475/ajs.302.9.806
Komiya, T., Maruyama, S., Hirata, T., Yurimoto, H., and Nohda, S. (2004). Geochemistry of the oldest MORB and OIB in the Isua Supracrustal Belt, southern West Greenland: implications for the composition and temperature of early Archean upper mantle. Isl. Arc 13, 47–72. doi:10.1111/j.1440-1738.2003.00416.x
Komiya, T., Yamamoto, S., Aoki, S., Koshida, K., Shimojo, M., Sawaki, Y., et al. (2017). A prolonged granitoid formation in Saglek Block, Labrador: zonal growth and crustal reworking of continental crust in the Eoarchean. Geosci. Front. 8, 355–385. doi:10.1016/j.gsf.2016.06.013
Komiya, T., Yamamoto, S., Aoki, S., Sawaki, Y., Ishikawa, A., Tashiro, T., et al. (2015). Geology of the Eoarchean, > 3.95 Ga, Nulliak supracrustal rocks in the Saglek Block, northern Labrador, Canada: the oldest geological evidence for plate tectonics. Tectonophysics 662, 40–66. doi:10.1016/j.tecto.2015.05.003
Korenaga, J. (2018). Estimating the formation age distribution of continental crust by unmixing zircon ages. Earth Planet Sci. Lett. 482, 388–395. doi:10.1016/j.epsl.2017.11.039
Koshida, K., Ishikawa, A., Iwamori, H., and Komiya, T. (2016). Petrology and geochemistry of mafic rocks in the Acasta Gneiss Complex: implications for the oldest mafic rocks and their origin. Precambrian Res. 283, 190–207. doi:10.1016/j.precamres.2016.07.004
Kositcin, N., and Krapez, B. (2004). Relationship between detrital zircon age-spectra and the tectonic evolution of the Late Archaean Witwatersrand Basin, South Africa. Precambrian Res. 129, 141–168. doi:10.1016/j.precamres.2003.10.011
Laurie, A., Stevens, G., and van Hunen, J. (2013). The end of continental growth by TTG magmatism. Terra Nova 25, 130–136. doi:10.1111/ter.12015
Li, Z., Chen, B., and Wei, C. (2016). Hadean detrital zircon in the North China Craton. J. Mineral. Petrol. Sci. 11, 283–291. doi:10.2465/jmps.150929
Li, Z. X., Bogdanova, S. V., Collins, A. S., Davidson, A., De Waele, B., Ernst, R. E., et al. (2008). Assembly, configuration, and break-up history of Rodinia: a synthesis. Precambrian Res. 1601, 179–210. doi:10.1016/j.precamres.2007.04.021
Liu, H., Sun, W. D., and Deng, J. (2020). Statistical analysis on secular records of igneous geochemistry: implication for the early Archean plate tectonics. Geol. J. 55, 994–1002. doi:10.1002/gj.3484
Ludden, J., and Hynes, A. (2000). The Lithoprobe Abitibi-Grenville transect: two billion years of crust formation and recycling in the Precambrian Shield of Canada. Can. J. Earth Sci. 37, 459–476. doi:10.1139/e99-120
Maier, A. C., Cates, N. L., Trail, D., and Mojzsis, S. J. (2012). Geology, age and field relations of Hadean zircon-bearing supracrustal rocks from Quad Creek, eastern Beartooth Mountains (Montana and Wyoming). Chem. Geol. 312, 47–51. doi:10.1016/j.chemgeo.2012.04.005
Mandal, B., Rao, V. V., Sarkar, D., Bhaskar Rao, Y. J., Raju, S., Karuppannan, P., et al. (2017). Deep crustal seismic reflection images from the Dharwar craton, Southern India—evidence for the Neoarchean subduction. Geophys. J. Int. 212, 777–794. doi:10.1093/gji/ggx427
Martel, E., van Breemen, O., Berman, R. G., and Pehrsson, S. (2008). Geochronology and tectonometamorphic history of the Snowbird Lake area, Northwest Territories, Canada: New insights into the architecture and significance of the Snowbird tectonic zone. Precambrian Res. 161, 201–230. doi:10.1016/j.precamres.2007.07.007
Martin, A. P., Condon, D. J., Prave, A. R., and Lepland, A. (2013). A review of temporal constraints for the Palaeoproterozoic large, positive carbonate carbon isotope excursion (the Lomagundi-Jatuli Event). Earth-Sci. Rev. 127, 242–261. doi:10.1016/j.earscirev.2013.10.006
Martin, E., Martin, H., and Sigmarsson, O. (2008). Could Iceland be a modern analogue for the Earth’s early continental crust? Terra Nova 20, 463–468. doi:10.1111/j.1365-3121.2008.00839.x
Martin, H., Moyen, J. F., Guitreau, M., Blichert-Toft, J., and Le Pennec, J. L. (2014). Why Archaean TTG cannot be generated by MORB melting in subduction zones. Lithos 198, 1–13. doi:10.1016/j.lithos.2014.02.017
Martin, H., Smithies, R. H., Rapp, R., Moyen, J. F., and Champion, D. (2005). An overview of adakite, tonalite–trondhjemite–granodiorite (TTG), and sanukitoid: relationships and some implications for crustal evolution. Lithos 79, 1–24. doi:10.1016/j.lithos.2004.04.048
Maruyama, S., Santosh, M., and Azuma, S. (2018). Initiation of plate tectonics in the Hadean: eclogitization triggered by the ABEL Bombardment. Geosci. Front. 9, 1033–1048. doi:10.1016/j.gsf.2016.11.009
Matsuda, T., and Uyeda, S. (1971). On the Pacific-type orogeny and its model—extension of the paired belts concept and possible origin of marginal seas. Tectonophysics 111, 5–27. doi:10.1016/0040-1951(71)90076-X
McCulloch, M. T., and Bennett, V. C. (1994). Progressive growth of the Earth’s continental crust and depleted mantle: geochemical constraints. Geochim. Cosmochim. Acta 58, 4717–4738. doi:10.1016/0016-7037(94)90203-8
McLennan, S. M., and Taylor, S. R. (1982). Geochemical constraints on the growth of the continental crust. J. Geol., 347–361. doi:10.1086/628690
McLennan, S. M., Taylor, S. R., McCulloch, M. T., and Maynard, J. B. (1990). Geochemical and Nd-Sr isotopic composition of deep-sea turbidites: crustal evolution and plate tectonic associations. Geochim. Cosmochim. Acta 54, 2015–2050. doi:10.1016/0016-7037(90)90269-Q
Meinster, B., and Tickell, S. J. (1975). Precambrian aeolian deposits in the Waterberg Supergroup. South Afric. J. Geol. 78, 191–199.
Minter, W. E. L., Renger, F. E., and Siegers, A. (1990). Early proterozoic gold placers of the Moeda Formation within the gandarela syncline, Minas gerais, Brazil. Econ. Geol. 85, 943–951. doi:10.2113/gsecongeo.85.5.943
Mojzsis, S. J., and Harrison, T. M. (2002). Establishment of a 3.83-Ga magmatic age for the Akilia tonalite (southern West Greenland). Earth Planet. Sci. Lett. 202, 563–576. doi:10.1016/S0012-821X(02)00825-7
Moores, E. M. (1991). Southwest US-East Antarctic SWEAT connection: a hypothesis. Geology 195, 425–428. doi:10.1130/0091-7613(1991)019<0425:SUSEAS>2.3.CO;2
Morgan, W. J. (1968). Rises, trenches, great faults, and crustal blocks. J. Geophys. Res. 73, 1959–1982. doi:10.1029/jb073i006p01959
Myers, J. S. (1988). Early Archaean Narryer gneiss complex, Yilgarn craton, western Australia. Precambrian Res. 38, 297–307. doi:10.1016/0301-9268(88)90029-0
Nadeau, S., Chen, W., Reece, J., Lachhman, D., Ault, R., Faraco, M. T. L., et al. (2013). Guyana: the lost Hadean crust of South America? Brazilian J. Geol. 43, 601–606. doi:10.5327/Z2317-48892013000400002
Naeraa, T., Scherstén, A., Rosing, M. T., Kemp, A. I. S., Hoffmann, J. E., Kokfelt, T. F., et al. (2012). Hafnium isotope evidence for a transition in the dynamics of continental growth 3.2 Gyr ago. Nature 485, 627–630. doi:10.1038/nature11140
Nagel, T. J., Hoffmann, J. E., and Münker, C. (2012). Generation of Eoarchean tonalite-trondhjemite-granodiorite series from thickened mafic arc crust. Geology 40, 375–378. doi:10.1130/G32729.1
Nance, R. D., and Murphy, J. B. (2013). Origins of the supercontinent cycle. Geosci. Front. 4, 439–448. doi:10.1016/j.gsf.2012.12.007
Nutman, A. P., Bennett, V. C., Friend, C. R., and Yi, K. (2020). Eoarchean contrasting ultra-high-pressure to low-pressure metamorphisms (< 250 to >1000 °C/GPa) explained by tectonic plate convergence in deep time. Precambrian Res., 105770. doi:10.1016/j.precamres.2020.105770
O'nions, R. K., Evensen, N. M., and Hamilton, P. J. (1979). Geochemical modeling of mantle differentiation and crustal growth. J. Geophys. Res.: Solid Earth 84, 6091–6101. doi:10.1029/jb084ib11p06091
Ogawa, M. (2014). Two‐stage evolution of the Earth's mantle inferred from numerical simulation of coupled magmatism‐mantle convection system with tectonic plates. J. Geophys. Res.: Solid Earth 1193, 2462–2486. doi:10.1002/2013JB010315
O’Neill, C., Marchi, S., Bottke, W., and Fu, R. (2020). The role of impacts on Archaean tectonics. Geology 48, 174–178. doi:10.1130/G46533.1
Palin, R. M., White, R. W., and Green, E. C. (2016). Partial melting of metabasic rocks and the generation of tonalitic–trondhjemitic–granodioritic (TTG) crust in the Archaean: constraints from phase equilibrium modelling. Precambrian Res. 287, 73–90. doi:10.1016/j.precamres.2016.11.001
Parman, S. W. (2015). Time-lapse zirconography: imaging punctuated continental evolution. Geochem.Perspect. Lett. 1, 43–52. doi:10.7185/geochemlet.1505
Petersson, A., Kemp, A. I., Hickman, A. H., Whitehouse, M. J., Martin, L., and Gray, C. M. (2019). A new 3.59 Ga magmatic suite and a chondritic source to the east Pilbara Craton. Chem. Geol. 511, 51–70. doi:10.1016/j.chemgeo.2019.01.021
Piper, J. D. A. (2018). Dominant Lid Tectonics behaviour of continental lithosphere in Precambrian times: palaeomagnetism confirms prolonged quasi-integrity and absence of supercontinent cycles. Geosci. Front. 9, 61–89. doi:10.1016/j.gsf.2017.07.009
Polat, A., Appel, P. W., and Fryer, B. J. (2011). An overview of the geochemistry of Eoarchean to Mesoarchean ultramafic to mafic volcanic rocks, SW Greenland: implications for mantle depletion and petrogenetic processes at subduction zones in the early Earth. Gondwana Res. 20, 255–283. doi:10.1016/j.gr.2011.01.007
Puetz, S. J., Condie, K. C., Pisarevsky, S., Davaille, A., Schwarz, C. J., and Ganade, C. E. (2017). Quantifying the evolution of the continental and oceanic crust. Earth Sci. Rev. 164, 63–83. doi:10.1016/j.earscirev.2016.10.011
Puetz, S. J., and Condie, K. C. (2019). Time series analysis of mantle cycles part I: periodicities and correlations among seven global isotopic databases. Geosci. Front. 10, 1305–1326. doi:10.1016/j.gsf.2019.04.002
Rapp, R. P., Shimizu, N., and Norman, M. D. (2003). Growth of early continental crust by partial melting of eclogite. Nature 425, 605. doi:10.1038/nature02031
Rawlings, D. J. (1999). Stratigraphic resolution of a multiphase intracratonic basin system: the McArthur Basin, northern Australia. Austral. J. Earth Sci. 46, 703–723. doi:10.1046/j.1440-0952.1999.00739.x
Rawlings, D. J., Korsch, R. J., Goleby, B. R., Gibson, G. M., Johnstone, D. W., and Barlow, M. (2004). The 2002 southern McArthur Basin seismic reflection survey. Geosci. Australia, Record 17, 78.
Raza, M., Bhardwaj, V. R., Ahmad, A. H. M., Mondal, M. E. A., Khan, A., and Khan, M. S. (2010). Provenance and weathering history of Archaean Naharmagra quartzite of Aravalli craton, NW Indian shield: petrographic and geochemical evidence. Geochem. J. 44, 331–345. doi:10.2343/geochemj.1.0075
Reymer, A., and Schubert, G. (1984). Phanerozoic addition rates to the continental crust and crustal growth. Tectonics 31, 63–77. doi:10.1029/TC003i001p00063
Rino, S., Komiya, T., Windley, B. F., Katayama, I., Motoki, A., and Hirata, T. (2004). Major episodic increases of continental crustal growth determined from zircon ages of river sands; implications for mantle overturns in the Early Precambrian. Phys. Earth Planet. In. 146, 369–394. doi:10.1016/j.pepi.2003.09.024
Rino, S., Kon, Y., Sato, W., Maruyama, S., Santosh, M., and Zhao, D. (2008). The Grenvillian and Pan-African orogens: world's largest orogenies through geologic time, and their implications on the origin of superplume. Gondwana Res. 14, 51–72. doi:10.1016/j.gr.2008.01.001
Robb, L. J., and Meyer, F. M. (1995). The Witwatersrand Basin, South Africa: geological framework and mineralization processes. Ore Geol. Rev. 10, 67–94. doi:10.1016/0169-1368(95)00011-9
Roberts, N. M., and Spencer, C. J. (2015). The zircon archive of continent formation through time. Geological Society, London, Special Publications 3891, 197–225. doi:10.1144/SP389.14
Rodríguez‐López, J. P., Clemmensen, L. B., Lancaster, N., Mountney, N. P., and Veiga, G. D. (2014). Archean to Recent aeolian sand systems and their sedimentary record: current understanding and future prospects. Sedimentology 61, 1487–1534. doi:10.1111/sed.12123
Rogers, J. J. (1996). A history of continents in the past three billion years. J. Geol. 104, 91–107. doi:10.1086/629803
Rogers, J. J., and Santosh, M. (2003). Supercontinents in earth history. Gondwana Res. 63, 357–368. doi:10.1016/S1342-937X(05)70993-X
Roman, A., and Arndt, N. (2020). Differentiated Archean oceanic crust: its thermal structure, mechanical stability and a test of the sagduction hypothesis. Geochim. Cosmochi. Acta 278, 65–77. doi:10.1016/j.gca.2019.07.009 in press
Roscoe, S. M., and Card, K. D. (1993). The reappearance of the Huronian in Wyoming: rifting and drifting of ancient continents. Can. J. Earth Sci. 30, 2475–2480. doi:10.1139/e93-214
Rye, R., and Holland, H. D. (1998). Paleosols and the evolution of atmospheric oxygen: a critical review. American J. Sci. 298, 621. doi:10.2475/ajs.298.8.621
Saha, D., Patranabis-Deb, S., and Collins, A. S. (2016). “Proterozoic stratigraphy of southern Indian cratons and global context”. in Stratigraphy and timescales. (Amsterdam: Elsevier), Vol. 1, 1–59. doi:10.1016/bs.sats.2016.10.003
Santosh, M., Maruyama, S., and Yamamoto, S. (2009). The making and breaking of supercontinents: some speculations based on superplumes, super downwelling and the role of tectosphere. Gondwana Res. 153, 324–341. doi:10.1016/j.gr.2008.11.004
Sawada, H., Isozaki, Y., Sakata, S., Hirata, T., and Maruyama, S. (2018). Secular change in lifetime of granitic crust and the continental growth: a new view from detrital zircon ages of sandstones. Geosci. Front. 9, 1099–1115. doi:10.1016/j.gsf.2016.11.010
Scholl, D. W., and von Huene, R. (2007). Crustal recycling at modern subduction zones applied to the past—Issues of growth and preservation of continental basement crust, mantle geochemistry, and supercontinent reconstruction. Geol. Soc. Am. Mem. 200, 9-32.
Scholl, D. W., and von Huene, R. (2009). Implications of estimated magmatic additions and recycling losses at the subduction zones of accretionary non-collisional and collisional suturing orogens. Geol. Soc., Lond., Special Publications 3181, 105–125. doi:10.1144/SP318.4
Seman, S., Stockli, D. F., and McLean, N. M. (2017). U-Pb geochronology of grossular-andradite garnet. Chem. Geol. 460, 106–116. doi:10.1016/j.chemgeo.2017.04.020
Shimojo, M., Yamamoto, S., Sakata, S., Yokoyama, T. D., Maki, K., Sawaki, Y., et al. (2016). Occurrence and geochronology of the Eoarchean, ∼ 3.9 Ga, Iqaluk gneiss in the Saglek block, northern Labrador, Canada: evidence for the oldest supracrustal rocks in the world. Precambrian Res. 278, 218–243. doi:10.1016/j.precamres.2016.03.018
Sircombe, K. N., Bleeker, W., and Stern, R. A. (2001). Detrital zircon geochronology and grain-size analysis of a ∼ 2800 Ma Mesoarchean proto-cratonic cover succession, Slave Province, Canada. Earth Planet. Sci. Lett. 189, 207–220. doi:10.1016/S0012-821X(01)00363-6
Sizova, E., Gerya, T., Brown, M., and Perchuk, L. L. (2010). Subduction styles in the Precambrian: insight from numerical experiments. Lithos 1163, 209–229. doi:10.1016/j.lithos.2009.05.028
Sleep, N. H., and Hessler, A. M. (2006). Weathering of quartz as an Archean climatic indicator. Earth Planet Sci. Lett. 241, 594–602. doi:10.1016/j.epsl.2005.11.020
Sleep, N. H., and Windley, B. F. (1982). Archean plate tectonics: constraints and inferences. J. Geol. 90, 363–379. doi:10.1086/628691
Smit, K. V., Shirey, S. B., Hauri, E. H., and Stern, R. A. (2019). Sulfur isotopes in diamonds reveal differences in continent construction. Science 364, 383–385. doi:10.1126/science.aaw9548
Smithies, R. H., Champion, D. C., and Cassidy, K. F. (2003). Formation of Earth's early Archaean continental crust. Precambrian Res. 127, 89–101. doi:10.1016/S0301-9268(03)00182-7
Smithies, R. H., Ivanic, T. J., Lowrey, J. R., Morris, P. A., Barnes, S. J., Wyche, S., et al. (2018). Two distinct origins for Archean greenstone belts. Earth Planet Sci. Lett. 487, 106–116. doi:10.1016/j.epsl.2018.01.034
Solomatov, V. S. (1995). Scaling of temperature‐and stress‐dependent viscosity convection. Phys. Fluids 7, 266–274. doi:10.1063/1.868624
Southwick, D. L., and Mossler, J. H. (1984). “The Sioux quartzite and subjacent regolith in the Cottonwood County basin, Minnesota,” in Shorter contributions to the geology of the Sioux quartzite (early Proterozoic), southwestern Minnesota: Minnesota geological survey report of investigations. Editor D. L. Southwick (Minneapolis: University of Minnesota), Vol. 32, 17–44.
Spaggiari, C. V., Pidgeon, R. T., and Wilde, S. A. (2007). The Jack Hills greenstone belt, Western Australia: part 2: lithological relationships and implications for the deposition of ≥ 4.0 Ga detrital zircons. Precambrian Res. 155, 261–286. doi:10.1016/j.precamres.2007.02.004
Spencer, C. J., Roberts, N. M. W., and Santosh, M. (2017). Growth, destruction, and preservation of Earth’s continental crust. Earth Sci. Rev. 172, 87–106. doi:10.1016/j.earscirev.2017.07.013
Srinivasan, R., and Ojakangas, R. W. (1986). Sedimentology of quartz-pebble conglomerates and quartzites of the Archean Bababudan group, Dharwar craton, South India: evidence for early crustal stability. J. Geol. 94, 199–214. doi:10.1086/629023
Stern, R. J., Leybourne, M. I., and Tsujimori, T. (2016). Kimberlites and the start of plate tectonics. Geology 44, 799–802. doi:10.1130/G38024.1
Stern, R. J., and Scholl, D. W. (2010). Yin and yang of continental crust creation and destruction by plate tectonic processes. Int. Geol. Rev. 52, 1–31. doi:10.1080/00206810903332322
Sugitani, K., Mimura, K., Suzuki, K., Nagamine, K., and Sugisaki, R. (2003). Stratigraphy and sedimentary petrology of an Archean volcanic–sedimentary succession at Mt. Goldsworthy in the Pilbara Block, Western Australia: implications of evaporite (nahcolite) and barite deposition. Precambrian Res. 120, 55–79. doi:10.1016/S0301-9268(02)00145-6
Suyehiro, K., Takahashi, N., Ariie, Y., and Yokoi, Y. (1996). Continental crust, crustal underplating, and low-Q upper mantle beneath an oceanic island arc. Science 272, 390. doi:10.1126/science.272.5260.390
Tackley, P. J. (1998). Self-consistent generation of tectonic plates in three-dimensional mantle convection. Earth Planet Sci. Lett. 157, 9–22. doi:10.1016/S0012-821X(98)00029-6
Tackley, P. J. (2000). Self‐consistent generation of tectonic plates in time‐dependent, three‐dimensional mantle convection simulations. G-cubed 1. doi:10.1029/2000GC000036
Tang, M., Chen, K., and Rudnick, R. L. (2016). Archean upper crust transition from mafic to felsic marks the onset of plate tectonics. Science 351, 372–375. doi:10.1126/science.aad5513
Tankard, A. J., Jackson, M. P. A., Eriksson, K. A., Hobday, D. K., Hunter, D. R., and Minter, W. E. L. (1982). “The earliest red beds”. in crustal evolution of Southern Africa. (New York, NY: Springer), 203–216. doi:10.1007/978-1-4613-8147-1_7
Thébaut, N., and Rey, P. F. (2013). Archean gravity-driven tectonics on hot and flooded continents: controls on long-lived mineralised hydrothermal systems away from continental margins. Precambrian Res. 229, 93–104. doi:10.1016/j.precamres.2012.03.001
Thirlwall, M. F., and Walder, A. J. (1995). In situ hafnium isotope ratio analysis of zircon by inductively coupled plasma multiple collector mass spectrometry. Chem. Geol. 122, 241–247. doi:10.1016/0009-2541(95)00003-5
Thorne, A. M., and Trendall, A. F. (2001). Geology of the Fortescue Group, Pilbara craton, western Australia. Geol. Surv. West. Aust. Bull. 144.
Tsenn, M. C., and Carter, N. L. (1987). Upper limits of power law creep of rocks. Tectonophysics 136, 1–26. doi:10.1016/0040-1951(87)90332-5
Tsutsumi, Y., Sawada, H., and Isozaki, Y. (2018). Search for Hadean zircon: decreasing the time required for pre-analyzing processes and age analyses. J. Geogr. 127, 723–734 (in Japanese with English abstract). doi:10.5026/jgeography.127.723
Van Kranendonk, M. J., Hugh Smithies, R., Hickman, A. H., and Champion, D. C. (2007). Secular tectonic evolution of Archean continental crust: interplay between horizontal and vertical processes in the formation of the Pilbara Craton, Australia. Terra Nova 19, 1–38. doi:10.1111/j.1365-3121.2006.00723.x
Vervoort, J. D., Patchett, P. J., Gehrels, G. E., and Nutman, A. P. (1996). Constraints on early Earth differentiation from hafnium and neodymium isotopes. Nature 379, 624–627. doi:10.1038/379624a0
Voice, P. J., Kowalewski, M., and Eriksson, K. A. (2011). Quantifying the timing and rate of crustal evolution: global compilation of radiometrically dated detrital zircon grains. J. Geol. 1192, 109–126. doi:10.1086/658295
von Huene, R., and Lallemand, S. (1990). Tectonic erosion along the Japan and Peru convergent margins. Geol. Soc. Am. Bull. 1026, 704–720. doi:10.1130/0016-7606(1990)102<0704:TEATJA>2.3.CO;2
Weller, O. M., Copley, A., Miller, W. G. R., Palin, R. M., and Dyck, B. (2019). The relationship between mantle potential temperature and oceanic lithosphere buoyancy. Earth Planet Sci. Lett. 518, 86–99. doi:10.1016/j.epsl.2019.05.005
White, D. J., Musacchio, G., Helmstaedt, H. H., Harrap, R. M., Thurston, P. C., Van der Velden, A., et al. (2003). Images of a lower-crustal oceanic slab: direct evidence for tectonic accretion in the Archean western Superior province. Geology 31, 997–1000. doi:10.1130/G20014.1
Whitmeyer, S. J., and Karlstrom, K. E. (2007). Tectonic model for the proterozoic growth of North America. Geosphere 3, 220–259. doi:10.1130/ges00055.1
Wilde, S. A., Valley, J. W., Peck, W. H., and Graham, C. M. (2001). Evidence from detrital zircons for the existence of continental crust and oceans on the Earth 4.4 Gyr ago. Nature409, 175–178. doi:10.1038/35051550
Wilks, M. E., and Nisbet, E. G. (1988). Stratigraphy of the Steep rock group, northwest Ontario: a major Archaean unconformity and Archaean stromatolites. Can. J. Earth Sci. 25 (3), 370–391. doi:10.1139/e88-040
Willbold, M., and Stracke, A. (2010). Formation of enriched mantle components by recycling of upper and lower continental crust. Chem. Geol. 276, 188–197. doi:10.1016/j.chemgeo.2010.06.005
Wilson, J. F., Nesbitt, R. W., and Fanning, C. M. (1995). Zircon geochronology of Archaean felsic sequences in the Zimbabwe craton: a revision of greenstone stratigraphy and a model for crustal growth. Geological Soc., London, Special Publ. 95, 109–126. doi:10.1144/GSL.SP.1995.095.01.07
Wilson, J. T. (1965). A new class of faults and their bearing on continental drift. Nature 207 (4995), 343. doi:10.1038/207343a0
Workman, R. K., Eiler, J. M., Hart, S. R., and Jackson, M. G. (2008). Oxygen isotopes in Samoan lavas: Confirmation of continent recycling. Geology 36 (7), 551–554. doi:10.1130/G24558A.1
Wyman, D. A., Kerrich, R., and Polat, A. (2002). Assembly of Archean cratonic mantle lithosphere and crust: plume‒arc interaction in the Abitibi-Wawa subduction‒accretion complex. Precambrian Res. 115, 37‒62. doi:10.1016/S0301-9268(02)00005-0
Xing, G. F., Wang, X. L., Wan, Y., Chen, Z. H., Jiang, Y., Kitajima, K., et al. (2014). Diversity in early crustal evolution: 4100 Ma zircons in the Cathaysia Block of southern China. Sci. Rep. 4, 5143. doi:10.1038/srep05143
Yamamoto, S., Nakajima, J., Hasegawa, A., and Maruyama, S. (2009). Izu-Bonin arc subduction under the Honshu island, Japan: evidence from geological and seismological aspect. Gondwana Res. 16, 572–580. doi:10.1016/j.gr.2009.05.014
Keywords: continental crust, size of continent, sandstone, Archean, early Earth
Citation: Sawada H (2020) Estimation of Secular Change in the Size of Continents for Understanding Early Crustal Development. Front. Earth Sci. 8:541094. doi: 10.3389/feart.2020.541094
Received: 07 March 2020; Accepted: 06 October 2020;
Published: 18 December 2020.
Edited by:
Kristoffer Szilas, University of Copenhagen, GermanyReviewed by:
Kenshi Suga, National Taiwan Normal University, TaiwanCopyright © 2020 Sawada. This is an open-access article distributed under the terms of the Creative Commons Attribution License (CC BY). The use, distribution or reproduction in other forums is permitted, provided the original author(s) and the copyright owner(s) are credited and that the original publication in this journal is cited, in accordance with accepted academic practice. No use, distribution or reproduction is permitted which does not comply with these terms.
*Correspondence: Hikaru Sawada, aHNhd2FkYUBqYW1zdGVjLmdvLmpw
Disclaimer: All claims expressed in this article are solely those of the authors and do not necessarily represent those of their affiliated organizations, or those of the publisher, the editors and the reviewers. Any product that may be evaluated in this article or claim that may be made by its manufacturer is not guaranteed or endorsed by the publisher.
Research integrity at Frontiers
Learn more about the work of our research integrity team to safeguard the quality of each article we publish.