- 1Department of Analytical Chemistry, Faculty of Chemistry, Gdańsk University of Technology, Gdańsk, Poland
- 2Department of Geomorphology, Faculty of Natural Sciences, Institute of Earth Sciences, University of Silesia, Sosnowiec, Poland
- 3Department of Analytical and Environmental Chemistry, Faculty of Chemistry, Adam Mickiewicz University Poznan, Poznań, Poland
Metals and metalloids in snow on glaciers, depending on the season of deposition, may come from various sources: local rock dust (erosion of the geological substratum), marine aerosol, local human activity (e.g., impurities in combusted fuel and waste incineration), and long-range atmospheric transport. Hansbreen, a glacier located close to the Polish Polar Station in southern Svalbard, is a perfect site to study metals and metalloids: it has a complex geological substratum, has a year-round presence of a small group of people, and is near the coast. We analyzed a snapshot of metal and metalloid concentrations in snow samples from shallow cores corresponding to autumn, winter, and spring deposition on Hansbreen. Eighteen cores of snow were collected across the glacier, revealing the influence of potential local sources of metals and metalloids. In these samples, we predominantly found Na, Mg, and K, followed by Zn, Ca, Al, and Fe. Heavy metals, such as Bi or Hg, were also detected. Cluster analysis of the determined elemental concentrations divided them into three distinct groups: Group 1: Ag, As, Bi, Cd, Hg, Mo, Sb, Se, and Zn—the most diverse cluster, representing mostly long-range transported volatile elements, with possible extra local geological sources; Group 2: Al, Fe, Cu, and Mn—elements with crustal sources; and Group 3: Na, Ca, Mg, K, and Sr—with the main source in sea spray aerosol. The latter interpretation was confirmed by the calculation of sea salt contribution based on the composition of mean seawater and the positive significant correlation between their concentrations and the electrical conductivity of snow samples. In the study site, snow was up to six times more efficient in bringing metal pollution into terrestrial environment, when compared to rain.
Introduction
Metal and metalloid impurities in the Arctic snow may come from both local and distant sources, and only some are supplied by human activities (Kozak et al., 2015). Pollutants can be delivered to Svalbard along fast and slow transport routes, and the cold climate favors accumulation of some contaminants (Ruman et al., 2012). Metals and metalloids can occur from background to excessive levels in the Arctic environment and cause harmful effects in people and animals at the latter (AMAP, 2005). Arctic snow captures atmospheric dust efficiently, through dry and wet deposition, and thus forms an archive of the seasonal contamination of the Arctic atmosphere. It transfers the pollutants to glacier surfaces, where they may be incorporated into ice (Lehmann-Konera et al., 2017) and cryoconite matter (Łokas et al., 2016). Snow upon glaciers in Svalbard has only rarely been sampled for metal and metalloid concentrations (beyond the major ion chemistry, as performed by, e.g., Virkkunen et al., 2007; Nawrot et al., 2016) and when it was sampled, the focus was either on one element, usually mercury (Ferrari et al., 2008; Larose et al., 2010), or on one location (Spolaor et al., 2020a). There has also been a study conducted on firn cores across one Svalbard glacier (Singh et al., 2015). Further studies report on the elemental composition of ice samples (Yevseyev and Korzun, 1985; Simoes and Zagorodnov, 2001; Drbal et al., 1992; Lehmann et al., 2016).
The spatial distribution of elemental deposition upon glacier surfaces may be modified by the influence of local sources of metals and metalloids, snow redeposition patterns (e.g., Grabiec et al., 2011), and other postdepositional processes (Larose et al., 2010; Avak et al., 2019). Here, we focus on the spatial distribution of elemental impurities in the snow on one glacier in southern Svalbard, in light with these processes. The site is located in the vicinity of the Polish Polar Station, a well-monitored area which may be considered a natural science laboratory for complex system observations. The area is subject to intensive glaciological research (Błaszczyk et al., 2019), especially Hansbreen (Kosiba, 1963; Pälli et al., 2003; Grabiec et al., 2006; Oerlemans et al., 2011; Petlicki et al., 2015; Laska et al., 2017; Decaux et al., 2019), which forms a perfect basis for the understanding of biogeochemical processes in glacial environments. This can be also enhanced through comparisons with metal and metalloid distribution, as was investigated in various environmental components of the area, such as surface waters (e.g., Kosek et al., 2019), plant tissue (e.g., Wojtuń et al., 2018), or fjord sediments (Frankowski and Zioła-Frankowska, 2014; Zaborska et al., 2017). In the context of glaciers, cryoconite matter has been subject to metal content studies (Łokas et al., 2016), as was the aluminum export from the nearby Werenskiöldbreen (Stachnik et al., 2019). Since the deposition of chemical elements may change seasonally (as was observed, e.g., for Ni and Pb by Berg et al. (2004)), additional temporal information is added in this study through the subdivision of the collected snow cores into layers. These correspond to a snapshot of autumn, winter, and spring deposition of metals and metalloids in the accumulation season of 2017/2018.
Methods
Field Site
Hansbreen is a medium-sized tidewater glacier (56 km2) located in SW Spitsbergen (Wedel Jarlsberg Land). It is approximately 16 km long and 2.5 km wide on average; it terminates into Hansbukta (Hornsund). Bordered by mountains, it is nevertheless linked to other glaciers (Paierlbreen, Vrangpeisbreen, and Werenskiöldbreen) (Szafraniec, 2002; Grabiec et al., 2011). Four tributary glaciers (Staszelisen, Deileggbreen, Tuvbreen, and Fuglebreen) are located in its western bank and one small tributary glacier in its eastern bank (Figure 1). The glacier’s elevation range is ca. 550 m (Grabiec et al., 2012).
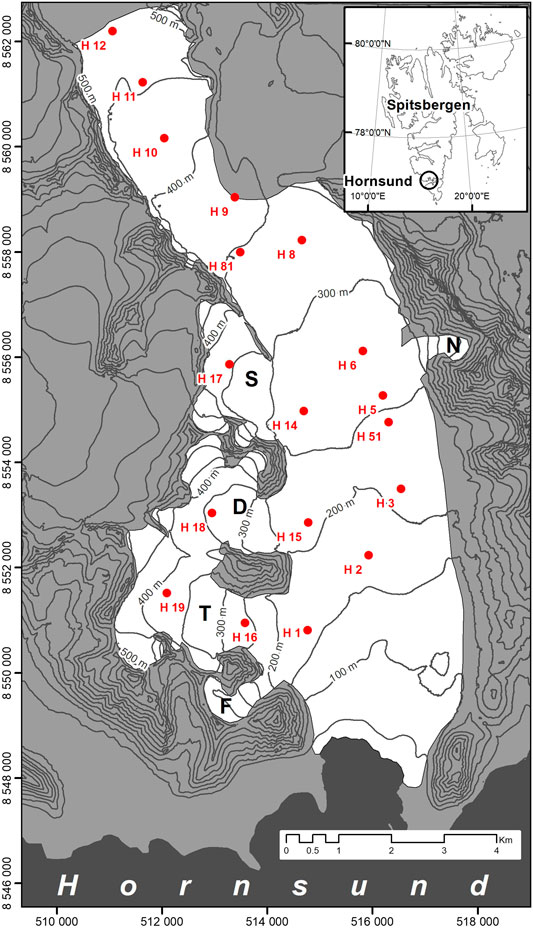
FIGURE 1. Study area: Hansbreen. The snow sampling locations are marked with red dots. Capital letters indicate tributary glaciers: D, Deileggbreen; F, Fuglebreen; N, Nørdstebreen; S, Staszelisen; T, Tuvbreen. The map is plotted in transverse Mercator projection, WGS84 datum, UTM 33X zone, with northing and easting marked on the map frame.
Every year on Hansbreen, new firn is built from the remaining snow cover (Błaszczyk et al., 2019; Uszczyk et al., 2019), yet the entire glacier records a negative mass balance due to the losses from ice melt and calving. The maximum depth of the snow cover at the end of accumulation season (April/May) occurs in the highest parts of the glacier (upglacier from H10, Figure 1). During the last 8 years, it reached 5.99 m in the accumulation season of 2010/2011. At the same point in the season, the minimum snow cover depth of 0.66 m was noted in 2012/2013 in the lower parts of Hansbreen (downglacier from H2, Figure 1). On average, the end-of-accumulation-season snow cover depth was 2.58, 2.01, and 2.22 m (in the 2010/2011, 2012/2013, and 2013/2014, respectively) (Grabiec, 2017). In general, the distribution of snow cover is linked to weather conditions (solid precipitation and temperature). Therefore, the altitude of the glacier determines the snow cover pattern and the snow cover thickness is modified further by the wind. As indicated by Grabiec et al. (2006), the eastern part of the glacier, which has relatively low accumulation, is the place where strong winds occur. Contrastingly, the west side (including tributary glaciers) is the typical place for the higher accumulation of snow cover.
The snow density on Hansbreen depends on snow depth (Uszczyk et al., 2019) and weather conditions (Grabiec et al., 2011; Łupikasza et al., 2019). The highest snowpack density occurred in the higher parts of the glacier, while the low density was nearer its front. A significant factor influencing the snow cover structure and its density is rain-on-snow events (Łupikasza et al., 2019). These events (more frequent than winter thaws) increase the density of snowpack through the formation of hard ice layers and may also leave a chemical footprint (Spolaor et al., 2020b). According to observations in 2010 and 2014 (Laska et al., 2016, 2017; Uszczyk et al., 2019), the snow cover density reached on average 398 kg/m3 at the end of accumulation season and 528 kg/m3 in the ablation season.
Hansbreen terminus lies approximately 3 km from the Polish Polar Station Hornsund, the only human settlement within a radius of 100 km (approximately 10 permanent staff live there). The biggest centre of human activity in Svalbard, Longyearbyen, is located about 140 km north from the study site. Its pollution sources include an airport, a functioning coal mine, local coal-fired power plant, a landfill and untreated sewage outflow (Granberg et al., 2017), a harbor, and local road and snowmobile traffic (e.g., Reimann et al., 2009). Barentsburg, another important settlement in Svalbard, lies over 120 km north from Hansbreen, harboring an active coal mine, a heliport, and a dumpsite where garbage has been burnt repeatedly (Granberg et al., 2017). The local human activity in Hornsund is from the small year-round team and up to 42 people during the peak research season (parts of the summer). It causes the following contamination sources: two arrivals of a supply ship, a diesel power plant, waste incineration, and sewage processing; ship traffic also exists in the nearby Hornsund fjord. In the spring, there is occasional local snowmobile traffic for research purposes.
The glacier is surrounded by diverse geological formations, with ore-bearing veins, which may be a source of metals and metalloids such as Al, As, Bi, Ca, Cd, Co, Cu, Fe, Mg, Mn, Ni, Pb, S, and Zn (Birkenmajer, 1990; Kieres and Piestrzyński, 1992; Czerny et al., 1993). In the wider area (within 15 km radius), there exist geological sources of Al, B, Ba, Be, Ca, Cr, Cs, Cu, Fe, K, Li, Mg, Mn, Na, Nb, Pb, Rb, Sn, Sr, Ta, Th, Ti, U, V, Zn, Zr, and rare-earth elements (cf. Koziol et al., 2020; Spolaor et al., 2020a).
Sampling
Snow samples were collected on 22nd and 25th April 2018. The air temperature during those field trips was approximately −5 and −10°C, respectively; on both days, there was a moderate wind speed of 5 m/s and little-to-no direct solar radiation. A snow core, with 9 cm diameter, was hand-drilled at each point shown on the map in Figure 1. To avoid external contamination entering the snow samples, an outer layer of the collected core was scraped with a precleaned polyethylene scraper. Only selected layers from the core were sampled, representing the bottom, middle, and top of the annual snowpack, which most likely belonged to the seasons autumn 2017, winter 2017/2018, and spring 2018, respectively. In particular, the autumn samples were characterized by an increased density (as compared to the rest of the snow core). The sampled layers spanned between 9 and 32 cm, averaging 17 cm (±4.3, 1 SD), while their density ranged from 0.287 to 0.655 g cm−3 (mean: 0.460 ± 0.0874 g cm−3). Each sampled layer differed from its neighboring layers in terms of hardness and/or grain size. The total thickness of the snow cover has been measured at each sampling site with a graduated avalanche probe, averaging 202 cm (±51.7, 1 SD; full range: 115–337 cm).
Laboratory Analysis
We analyzed potassium, calcium, magnesium, and iron, with inductively coupled plasma optical emission spectrometry (ICP-OES 9820, Shimadzu, Japan). Twenty-one other elements (Ag, Al, As, Ba, Bi, Cd, Co, Cr, Cu, Hg, Li, Mn, Mo, Na, Ni, Pb, Sb, Se, Sr, V, and Zn) were analyzed using inductively coupled plasma mass spectrometry (ICP-MS 2030, Shimadzu, Japan). Before analysis, the samples were acidified with high-purity nitric acid and trace metal basis (Sigma-Aldrich). Analyses were run in triplicate (with a mean CV 3.82% and 6.43% for ICP-OES and ICP-MS measurements, respectively). The specific measurement conditions and parameters are listed in Supplementary Table S1 (Supplementary Information). All measurements were quality-assured. The ICP-MS was calibrated with the ICP IV multielement standard (Merck) and the single-element standards of As, Sb, Se, Mo, and V (Sigma-Aldrich). Solutions of Sc, Rh, Tb, and Ge in suprapure 1% HNO3 (Merck) were applied as internal standards. Samples were diluted (if necessary) using deionized water (from Milli-Q Direct 8 Water Purification System, Merck Millipore). The ICP-OES was calibrated with single-element standards (Sigma-Aldrich) of 1000 mg L−1. Analytical accuracy was verified with two certified reference materials (CRMs): Trace Metals ICP-Sample 1 and Trace Metals ICP-Sample 2 (Sigma-Aldrich). CRM recovery ranged from 96% to 104%. Further analytical parameters, including the detection and quantification limits of the method, are listed in Supplementary Table S2 (Supplementary Information).
Total organic carbon (TOC) was determined through catalytic oxidation with oxygen at 680°C, with a nondispersive infrared spectroscopy detector (Analyser TOC-VCSH/CSN, Shimadzu). The pH and electrical conductivity were measured with a microcomputer pH meter and conductivity meter (inoLab® Multi 9310 IDS pH), fitted with a Tetra-Con® 925 conductivity sensor and a SenTix®940 electrodes. The analytical qualitative parameters of the basic physicochemical characteristics are given in Supplementary Table S3 (Supplementary Information). Metal and metalloid concentrations measured have been corrected by the subtraction of a mean blank value, calculated from five procedural blanks. In the rest of the assays, the blank correction was not required, as background contamination was negligibly low.
Statistical Procedures
Before statistical analyses, results below the limit of detection were assigned 1/3 of its value (to approximate their probable level according to the data distribution curve, approximated best as log-normal for most of the studied variables). All statistical analyses were performed using Statistica, version 13.3 (TIBCO). The first tool used to explore our data was cluster analysis applied to variables. The cluster analysis procedure links elements with concentrations of the most similar variability. In brief, concentrations of elements are standardized (transformed to have a mean of 0 and standard deviation of 1), which means that the concentrations of predominant and minor chemical components of the snow samples can be safely compared. Then, they are plotted upon a dendrogram, and the closer they are on the graph, the more similar is their distribution around their mean. Admittedly, the mean and standard deviation are parameters typical for normally distributed datasets, yet as an exploratory method, cluster analysis can also be used for non-normal data distributions. For the clustering, we used Ward’s method, with squared Euclidean distance. The correlations mentioned further in the text were calculated using two nonparametric indices, Spearman rank ρ, and Kendall τ, due to the non-normal distribution of data.
Results and Discussion
Cluster analysis results (Figure 2) show, at a 33% relative distance level (a typical cutoff point for the most similar items), there are three distinct groups of metals and metalloids: cluster 1: Ag, Bi, Cd, Sb, As, Hg, Zn, Se, and Mo; cluster 2: Al, Cu, Fe, and Mn; cluster 3: Ca, K, Mg, Sr, and Na. Since the snow in the area has been shown to contain a relatively high proportion of sea spray aerosols (Nawrot et al., 2016), cluster 3 is likely connected to this source. Cluster 2 is likely representing local geological substratum variability, with the local metamorphic rocks containing ore-bearing veins with Cu and Fe, while Al and Mn are also generally abundant elements of the Earth’s crust (Birkenmajer, 1990; Kieres and Piestrzyński, 1992; Czerny et al., 1993). Cluster 1 is the most diverse, with elements at very low concentrations, some of which are typically transported to Svalbard from remote anthropogenic sources (e.g., Hg and Cd; Carlsson et al., 2016). Furthermore, the elements in this cluster have volatile forms, which are easily transported over long distances (especially Hg, Zn, As, Cd, Sb, and Se, and likely also Ag, Bi, and Mo; Steinnes and Friedland, 2006). However, in the Hansbreen surroundings, both Hg and Cd, as well as Ag, As, Bi, Sb, and Zn, all occur in ore-bearing veins on the western side of Hansbreen (Kieres and Piestrzyński, 1992), either as their minerals or as admixtures (Cd mainly in sphalerite, Ag in galena, Hg in sphalerite, pyrite, and pyrrhotite). Interestingly, Se occurred as an admixture in rock samples from ore-bearing veins (especially of pyrite, pyrrhotite, and galena), yet at a much lower content level (at least 10 times less than the other elements in the cluster). Furthermore, there was no significant mineral source of Mo found in those rocks, despite this element being included in the geological composition study (Kieres and Piestrzyński, 1992).
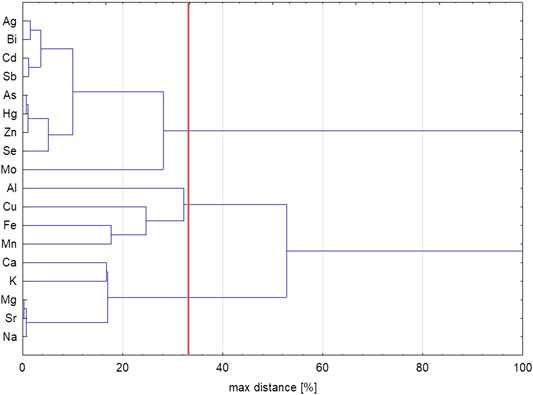
FIGURE 2. Dendrogram resulting from cluster analysis of the metal and metalloid concentrations in the snow cover of the Hansbreen, based on the samples collected in spring 2018. Clustering was performed based on Ward's method, with squared Euclidean distance, on z-transformed (standardized) values.
The spatial and temporal distribution of elements from each cluster is exemplified by one of the cluster members in Figure 3. Additional information on the seasonal distribution of elemental concentrations and on elemental fluxes calculated in this study is also given in Supplementary Tables S4 and S5, respectively (Supplementary Information). The sea spray cluster 3 was characterized by generally lower concentrations of its elements in the winter season layers (Figure 3A), while the highest concentrations of these elements were noted in the spring layers nearest to seawater (Burgerbukta to the east). The concentrations of elements from cluster 3 decreased also up the glacier, which could reflect a decreasing supply of sea spray from the Hornsund fjord. The rock dust in cluster 2 showed a more even distribution of concentrations across the glacier (Figure 3B). Occasionally, elevated concentrations of its elements could be found, especially in the autumn layer. Such concentrations could be interpreted as an effect of rock dust abundance in the period when snow cover only starts forming and does not cover fully the mountain slopes or the moraines. The volatile element cluster 1 (Figure 3C) was patchily distributed, with higher concentrations on the western side of the glacier, where the snow cover was accumulated by wind redeposition (Grabiec et al., 2006), and at higher elevations, perhaps due to the exposure to long-distance-transported air pollution. However, this side of the glacier is also closer to the elemental sources in rock dust, so their concentrations may only be elevated very near to such areas (due to the generally lower concentrations of these elements in the said rocks and their irregular spatial distribution as ore-bearing veins).
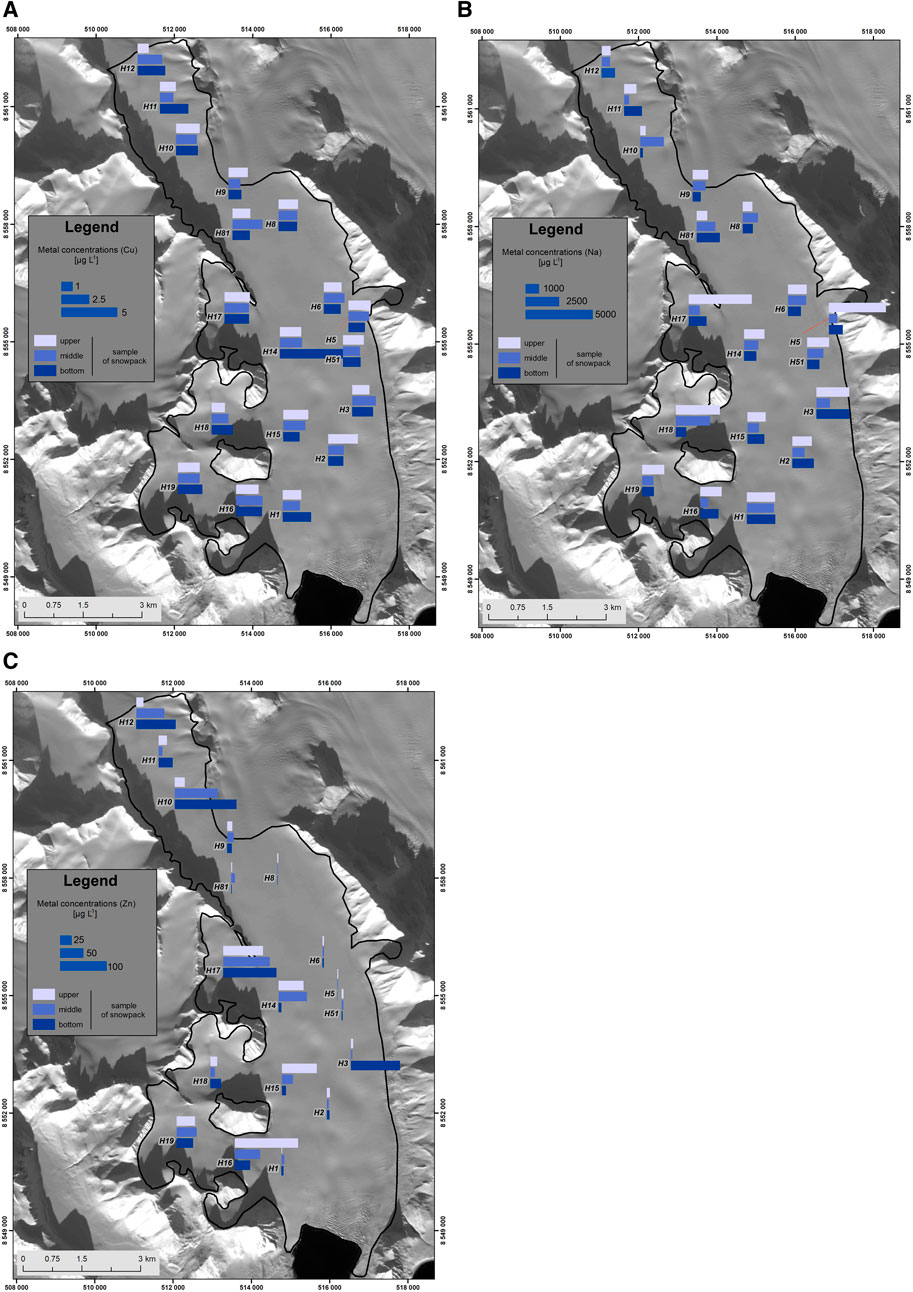
FIGURE 3. Spatial and interlayer distribution of selected metal concentrations [µg L−1] in the snow cover of Hansbreen in the accumulation season of 2017/2018. Each figure concerns a member of a different variable cluster in Figure 2: (A) Na, (B) Cu, and (C) Zn. All maps are plotted in the WGS84 datum, in transverse Mercator projection, UTM 33X zone, with northing and easting marked on the map frame.
To further explore the possible sources of various elements in the snowpack, we calculated correlation coefficients between their concentrations and the pH, electrical conductivity, and total organic carbon (TOC) concentration in the collected samples. Both Spearman ρ and Kendall τ showed the same pairs of variables to correlate significantly at the p level <0.05 (and only the correlations with electrical conductivity were significant at p < 0.01). Electrical conductivity correlated positively and strongly with the concentrations of Ca, K, Mg, Na, and Sr, grouping again the sea salt-related elements in one cluster. Also, the concentration of Fe correlated negatively with pH of the samples, likely indicating the origin of Fe from sulphide minerals, which oxidate producing SO42- ions which acidify the snow (Moses et al., 1987). If we assume that TOC concentration is a sign of likely pollution from snowmobile tracks, then this factor appears as unimportant in shaping the metal and metalloid composition of snow upon the glacier: the highest positive Spearman ρ between any metal concentration and TOC equaled 0.096 for Mo.
Multiple elements seem to be supplied from sea spray on this glacier, which merits the calculation of sea salt contribution (ssC) into the elemental concentrations in the snow cover. This has been performed assuming that all Na was derived from sea salt and that sea spray has the same salt composition as average seawater (Turekian, 1968) (Table 1). In this procedure, all data records with values below the detection limit were omitted. The occurrence of sea salt contributions exceeding 100% indicates either that there were extra sources of Na besides sea salt or that Na may be enriched in sea spray, as compared to mean seawater composition, or else that these elements’ concentrations were depleted postdepositionally. The elements with the highest sea salt contributions were Ca, Mg, Sr, and K (median ssCs >25%), which confirms the origin of the first variable cluster in Figure 2 from sea spray. In isolated cases (maximum ssCs >5%), sea spray seemed to be a significant source of also Mo and Hg.
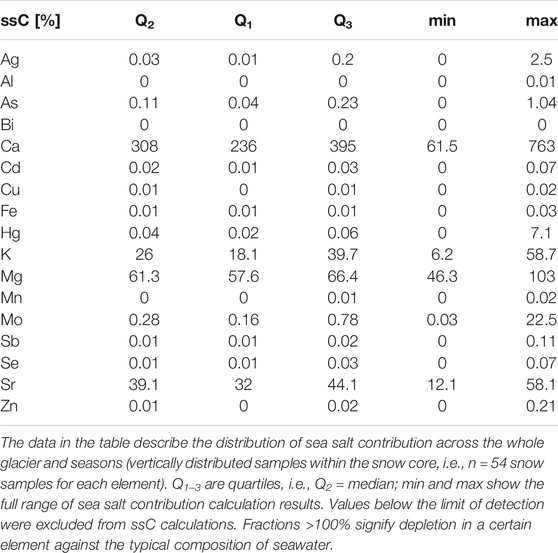
TABLE 1. Sea salt contribution (ssC) to the elemental composition of snow on Hansbreen, calculated from the proportional concentration of each element to sodium.
Crustal fractions of elements were also estimated (Supplementary Table S6, Supplementary Information), assuming all Al was of crustal origin, and using geochemical background composition after the Geochemical Atlas of Svalbard (Ottesen, 2015). Whenever the Geochemical Atlas supplied two different values derived from two different analytical methods, we used the concentrations determined by the analytical method most similar to the one used here. The calculations highlighted the crustal origin of Fe in the collected samples; in certain samples, Mg and As could also be supplied from the general geochemical crustal background (max crustal fractions >5%). However, the very low crustal fractions of other elements calculated in this way do not exclude their origin from local ore-bearing veins. This is because the locally occurring veins would not influence the Svalbard-wide geochemical background concentrations, which were the basis for the calculations. The low crustal fractions of calcium support the interpretation that Ca from rock dust was negligible in the collected snow samples.
Since the deposition of impurities in snow is generally considered very efficient (Błaś et al., 2010), we compare the obtained median concentrations in the snow with the median concentrations measured in the rain at the nearby Polish Polar Station Hornsund (Kozak et al., 2015). Available for comparison were the concentrations of Al, As, Cu, Mn, Se, Sr, and Zn. All these elements were found at higher median concentrations in the snow on Hansbreen, exceeding the concentrations in rain from 1.02 to 5.88 times (for Zn and Al, respectively). The maxima of metal and metalloid concentrations in summer precipitation, published by Koziol et al. (2020), were also mostly lower than maximum concentrations in the snow studied here (for Al, Cu, Sb, and Se). Mn, Sr, and Zn showed the opposite proportion, but the difference between the two maxima was very small for Zn. Overall, the snow cover seems a more important medium than rain for the transfer of metal and metalloid impurities from the atmosphere to the terrestrial system on this glacier. The snow cover also holds such impurities for longer and may release them in different temporal patterns, depending on their abundance in the snowpack (Avak et al., 2019). Thus, snow appears to be a crucial component for the supply of metals and metalloids to the surface waters of the area, which warrants its further investigations to understand the element mobility and long-term concentration changes.
Conclusion
Weinvestigated the concentrations of metal and metalloid impurities in the annual snowpack on a glacier in southern Svalbard, focusing on their spatial variability. We attempted to determine their main sources by the application of statistical analysis and a comparison to seawater and crustal composition. As a result, we found that Na, Mg, Ca, K, and Sr had a significant source in the sea spray aerosol. Al, Fe, Cu, and Mn could be attributed to crustal sources. Multiple elements likely come from long-range transport, with potential secondary sources in the local geological substratum (ore-bearing veins): Ag, As, Bi, Cd, Hg, Sb, Se, and Zn; however, Mo is likely to come from atmospheric transport alone. In comparison to summer rains, snowfall showed higher median concentrations of metals and metalloids (between one and six times higher). The studies on metal and metalloid admixtures in the Arctic snow are important means of measuring the supply of impurities to surface waters and downstream ecosystems, and their long-term changes should be studied in the future.
Data Availability Statement
The datasets generated for this study can be found in the Open Science Framework repository [https://osf.io/ypskz] and in Supplementary Information.
Author Contributions
KK and ŻP developed the concept of the paper. KK wrote the main body of the manuscript and prepared the sampling kit. AU collected the samples, prepared maps, and provided field site description. FP and MF analysed the samples (for basic parameters and metal/metalloid concentrations, respectively) and wrote the description of the analytical methods. All authors participated in the final discussion of the results and checked the final version of the manuscript.
Funding
Internal funding for the statutory activity at the Pedagogical University in Cracow has supported this research (project Spatial Variability of the Concentrations of Macro- and Microelements in the Snow Cover of the Arctic, PI: KK), as did the Leading National Research Centre (KNOW) project received by the Centre for Polar Studies of the University of Silesia, Poland. The article has been written as part of KK’s employment and FP’s scholarship within the National Science Centre of Poland, under research grant no. 2017/26/D/ST10/00630.
Conflict of Interest
The authors declare that the research was conducted in the absence of any commercial or financial relationships that could be construed as a potential conflict of interest.
Acknowledgments
Ł. Małarzewski is thanked for field assistance.
Supplementary Material
The Supplementary Material for this article can be found online at: https://www.frontiersin.org/articles/10.3389/feart.2020.538762/full#supplementary-material.
References
AMAP, (2005). AMAP assessment 2002: heavy metals in the arctic. Oslo, Norway: arctic monitoring and assessment programme (AMAP). Available at: http://www.amap.no/documents/doc/amap-assessment-2002-radioactivity-in-the-arctic/93 (Accessed April 1, 2006).
Avak, S. E., Trachsel, J. C., Edebeli, J., Brütsch, S., Bartels-Rausch, T., Schneebeli, M., et al. (2019). Melt-induced fractionation of major ions and trace elements in an alpine snowpack. J. Geophys. Res. Earth Surf. 124, 1647–1657. doi:10.1029/2019JF005026
Berg, T., Kallenborn, R., and Manø, S. (2004). Temporal trends in atmospheric heavy metal and organochlorine concentrations at zeppelin, Svalbard. Arctic. Antarct. Alp. Res. 36, 284–291. doi:10.1657/1523-0430
Birkenmajer, K. (1990). Geological Map of the Hornsund area 1 : 75 000. Katowice, Poland: University of Silesia.
Błaś, M., Cichała-Kamrowska, K., Sobik, M., Polkowska, Ż., and Namieśnik, J. (2010). Conditions controlling atmospheric pollutant deposition via snowpack. Environ. Rev. 18, 87–114. doi:10.1139/A10-003
Błaszczyk, M., Ignatiuk, D., Uszczyk, A., Cielecka-Nowak, K., Grabiec, M., Jania, J. A., et al. (2019). Freshwater input to the arctic fjord Hornsund (Svalbard). Polar Res. 38, 1–18. doi:10.33265/polar.v38.3506
Carlsson, P., Christensen, J. H., Borgå, K., Kallenborn, R., Aspmo Pfaffhuber, K., Odland, J. Ø., et al. (2016). AMAP 2016. Influence of Climate Change on Transport, Levels, and Effects of Contaminants in Northern Areas-Part 2 (Oslo, Norway: AMAP).
Czerny, J., Kieres, A., Manecki, M., and Rajchel, J. (1993). Geological Map of the SW Part of Wedel-Jarlsberg Land. Spitsbergen.
Decaux, L., Grabiec, M., Ignatiuk, D., and Jania, J. (2019). Role of discrete water recharge from supraglacial drainage systems in modeling patterns of subglacial conduits in Svalbard glaciers. Cryosphere 13, 735–752. doi:10.5194/tc-13-735-2019
Drbal, K., Elster, J., and Komárek, J. (1992). Heavy metals in water, ice and biological material from Spitsbergen. Svalbard. Polar Res. 11, 99–101. doi:10.3402/polar.v11i2.6721
Ferrari, C. P., Padova, C., Faïn, X., Gauchard, P. A., Dommergue, A., Aspmo, K., et al. (2008). Atmospheric mercury depletion event study in Ny-Alesund (Svalbard) in spring 2005. Deposition and transformation of Hg in surface snow during springtime. Sci. Total Environ. 397, 167–177. doi:10.1016/j.scitotenv.2008.01.064
Frankowski, M., and Zioła-Frankowska, A. (2014). Analysis of labile form of aluminum and heavy metals in bottom sediments from Kongsfjord, Isfjord, Hornsund fjords. Environ. Earth Sci. 71, 1147–1158. doi:10.1007/s12665-013-2518-5
Grabiec, M., Jania, J. A., Puczko, D., Kolondra, L., and Budzik, T. (2012). Surface and bed morphology of Hansbreen, a tidewater glacier in Spitsbergen. Pol. Polar Res. 33, 111–138. doi:10.2478/v10183-012-0010-7
Grabiec, M., Leszkiewicz, J., Głowacki, P., and Jania, J. (2006). Distribution of snow accumulation on some glaciers of Spitsbergen. Pol. Polar Res. 27, 309–326
Grabiec, M., Puczko, D., Budzik, T., and Gajek, G. (2011). Snow distribution patterns on Svalbard glaciers derived from radio-echo soundings. Pol. Polar Res. 32, 393–421. doi:10.2478/v10183-011-0026-4
Grabiec, M. (2017). Stan I Współczesne Zmiany Systemów Lodowcowych Południowego Spitsbergen W Świetle Badań Metodami Radarowymi [The State and Contemporary Changes of the Glacial Systems in Southern Spitsbergen in the Light of the Radar Methods]. Katowice, Poland: University of Silesia.
Granberg, M. E., Ask, A., and Gabrielsen, G. W. (2017). Local contamination in Svalbard. Overview and suggestions for remediation actions. Tromsø: Norwegian Polar Institute.
Kieres, A., and Piestrzyński, A. (1992). “Ore-mineralisation of the hecla hoek succession (precambrian) around Werenskioldbreen, south Spitsbergen,” in Studia geologica polonica. Editor K. Birkenmajer (Warsaw: Polish Academy of Sciences, Institute of Geological Sciences), 115–151.
Kosek, K., Kozioł, K., Luczkiewicz, A., Jankowska, K., Chmiel, S., and Polkowska, Ż. (2019). Environmental characteristics of a tundra river system in Svalbard. Part 2: chemical stress factors. Sci. Total Environ. 653, 1585–1596. doi:10.1016/j.scitotenv.2018.11.012
Kosiba, A. (1963). Changes in the Werenskiold glacier and Hans glacier in SW Spitsbergen. Int. Assoc. Sci. Hydrol. Bull. 8, 24–35. doi:10.1080/02626666309493294
Kozak, K., Kozioł, K., Luks, B., Chmiel, S., Ruman, M., Marć, M., et al. (2015). The role of atmospheric precipitation in introducing contaminants to the surface waters of the Fuglebekken catchment, Spitsbergen. Polar Res. 34, 24207. doi:10.3402/polar.v34.24207
Koziol, K., Ruman, M., Pawlak, F., Chmiel, S., and Polkowska, Ż. (2020). Spatial differences in the chemical composition of surface water in the hornsund fjord area: a statistical analysis with a focus on local pollution sources. Water (MDPI). 12, 1–22. doi:10.3390/w12020496
Larose, C., Dommergue, A., De Angelis, M., Cossa, D., Averty, B., Marusczak, N., et al. (2010). Springtime changes in snow chemistry lead to new insights into mercury methylation in the Arctic. Geochem. Cosmochim. Acta. 74, 6263–6275. doi:10.1016/j.gca.2010.08.043
Laska, M., Grabiec, M., Ignatiuk, D., and Budzik, T. (2017). Snow deposition patterns on southern Spitsbergen glaciers, Svalbard, in relation to recent meteorological conditions and local topography. Geogr. Ann. Phys. Geogr. 99, 262–287. doi:10.1080/04353676.2017.1327321
Laska, M., Luks, B., and Budzik, T. (2016). Influence of snowpack internal structure on snow metamorphism and melting intensity on Hansbreen, Svalbard. Polish Polar Res. 37, 193–218. doi:10.1515/popore-2016-0012
Lehmann-Konera, S., Ruman, M., Kozioł, K., Gajek, G., and Polkowska, Ż. (2017). Glaciers as an important element of the world glacier monitoring implemented in Svalbard. Glaciers Evol. A Chang. World., 3–36. doi:10.5772/intechopen.69237
Lehmann, S., Gajek, G., Chmiel, S., and Polkowska, Ż. (2016). Do morphometric parameters and geological conditions determine chemistry of glacier surface ice? Spatial distribution of contaminants present in the surface ice of Spitsbergn glaciers (European Arctic). Environ. Sci. Pollut. Res. Int. 23, 23385–23405. doi:10.1007/s11356-016-7354-1
Łokas, E., Zaborska, A., Kolicka, M., Różycki, M., and Zawierucha, K. (2016). Accumulation of atmospheric radionuclides and heavy metals in cryoconite holes on an Arctic glacier. Chemosphere 160, 162–172. doi:10.1016/j.chemosphere.2016.06.051
Łupikasza, E. B., Ignatiuk, D., Grabiec, M., Cielecka-Nowak, K., Laska, M., Jania, J., et al. (2019). The role of winter rain in the glacial system on Svalbard. Water 11, 334. doi:10.3390/w11020334
Moses, C. O., Kirk Nordstrom, D., Herman, J. S., and Mills, A. L. (1987). Aqueous pyrite oxidation by dissolved oxygen and by ferric iron. Geochem. Cosmochim. Acta. 51, 1561–1571. doi:10.1016/0016-7037(87)90337-1
Nawrot, A. P., Migała, K., Luks, B., Pakszys, P., and Głowacki, P. (2016). Chemistry of snow cover and acidic snowfall during a season with a high level of air pollution on the Hans Glacier, Spitsbergen. Polar Sci. 10, 249–261. doi:10.1016/j.polar.2016.06.003
Oerlemans, J., Jania, J., and Kolondra, L. (2011). Application of a minimal glacier model to Hansbreen. Svalbard. Cryosph. 5, 1–11. doi:10.5194/tc-5-1-2011
Ottesen, R. T. (2015). “Geochemistry of superficial deposits,” in Geoscience atlas of Svalbard. Editor W. K. Dallmann (Tromsø: Norsk Polarinstitutt, 241–248.
Pälli, A., Moore, J. C., Jania, J., Kolondra, L., and Głowacki, P. (2003). The drainage pattern of Hansbreen and Werenskioldbreen, two polythermal glaciers in Svalbard. Polar Res. 22, 355–371. doi:10.3402/polar.v22i2.6465
Petlicki, M., Cieply, M., Jania, J. A., Prominska, A., and Kinnard, C. (2015). Calving of a tidewater glacier driven by melting at the waterline. J. Glaciol. 61, 851–863. doi:10.3189/2015JoG15J062
Reimann, S., Kallenborn, R., and Schmidbauer, N. (2009). Severe aromatic hydrocarbon pollution in the Arctic town of Longyearbyen (Svalbard) caused by snowmobile emissions. Environ. Sci. Technol. 43, 4791–4795. doi:10.1021/es900449x
Ruman, M., Kozak, K., Lehmann, S., Kozioł, K., and Polkowska, Z. (2012). Pollutants present in different components of the Svalbard archipelago environment. Ecol. Chem. Eng. S. 19, 571–584. doi:10.2478/v10216-011-0040-9
Simoes, J. C., and Zagorodnov, V. S. (2001). The record of anthropogenic pollution in snow and ice in Svalbard, Norway. Atmopsheric Environ. 35, 403–413. doi:10.1016/S1352-2310(00)00122-9
Singh, S. M., Gawas-Sakhalkar, P., Naik, S., Ravindra, R., Sharma, J., Upadhyay, A. K., et al. (2015). Elemental composition and bacterial incidence in firn-cores at Midre Lovénbreen glacier, Svalbard. Polar Rec. 51, 39–48. doi:10.1017/S0032247413000533
Spolaor, A., Moroni, B., Luks, B., Nawrot, A., Roman, M., Larose, C., et al. (2020a). Investigation on the sources and impact of trace elements in the annual snowpack and the firn ice in the Hansbreen glacier (southwest Spitsbergen). Front. Earth Sci., doi:10.3389/feart.2020.536036
Spolaor, A., Varin, C., Pedeli, X., Christille, J. M., Kirchgeorg, T., Giardi, F., et al. (2020b). Source, timing and dynamics of ionic species mobility in the Svalbard annual snowpack. Sci. Total Environ. 751, 141640. doi:10.1016/j.scitotenv.2020.141640
Stachnik, L., Yde, J. C., Nawrot, A., Uzarowicz, Ł., Łepkowska (Majchrowska), E., and Kozak, K. (2019). Aluminium in glacial meltwater demonstrates an association with nutrient export (Werenskiöldbreen, Svalbard). Hydrol. Process. doi:10.1002/hyp.13426
Steinnes, E., and Friedland, A. J. (2006). Metal contamination of natural surface soils from long-range atmospheric transport: existing and missing knowledge. Environ. Rev. 14, 169–186. doi:10.1139/A06-002
Szafraniec, J. (2002). Influence of positive degree-days and sunshine duration on the surface ablation of Hansbreen, Spitsbergen glacier. Pol. Polar Res. 23, 227–240.
Uszczyk, A., Grabiec, M., Laska, M., Kuhn, M., and Ignatiuk, D. (2019). Importance of snow as component of surface mass balance of Arctic glacier (Hansbreen, southern Spitsbergen). Pol. Polar Res. 40, 311–338. doi:10.24425/ppr.2019.130901
Virkkunen, K., Moore, J. C., Isaksson, E., Pohjola, V., Perämäki, P., Grinsted, A., et al. (2007). Warm summers and ion concentrations in snow: comparison of present day with Medieval Warm Epoch from snow pits and an ice core from Lomonosovfonna, Svalbard. J. Glaciol. 53, 623–634. doi:10.3189/002214307784409388
Wojtuń, B., Samecka-Cymerman, A., Kolon, K., and Kempers, A. J. (2018). Metals in racomitrium lanuginosum from arctic (SW Spitsbergen, Svalbard archipelago) and alpine (Karkonosze, SW Poland) tundra. Environ. Sci. Pollut. Res. Int. 25, 12444.doi:10.1007/s11356-018-1508-2
Yevseyev, A. V., and Korzun, A. V. (1985). On the chemical composition of ice cover on Nordaustlandet [in Russian]. Mater. Glyatsologicheskikh Isledovaniy. 52, 205–209.
Keywords: Svalbard, Arctic, spatial distribution, snow, heavy metals, trace elements
Citation: Koziol K, Uszczyk A, Pawlak F, Frankowski M and Polkowska Ż (2021) Seasonal and Spatial Differences in Metal and Metalloid Concentrations in the Snow Cover of Hansbreen, Svalbard. Front. Earth Sci. 8:538762. doi: 10.3389/feart.2020.538762
Received: 28 February 2020; Accepted: 10 December 2020;
Published: 14 January 2021.
Edited by:
Feiteng Wang, Chinese Academy of Sciences, ChinaReviewed by:
Janae Csavina, National Ecological Observatory Network, United StatesGiovanni Baccolo, University of Milano-Bicocca, Italy
Copyright © 2021 Koziol, Uszczyk, Pawlak, Frankowski and Polkowska. This is an open-access article distributed under the terms of the Creative Commons Attribution License (CC BY). The use, distribution or reproduction in other forums is permitted, provided the original author(s) and the copyright owner(s) are credited and that the original publication in this journal is cited, in accordance with accepted academic practice. No use, distribution or reproduction is permitted which does not comply with these terms.
*Correspondence: Żaneta Polkowska, emFucG9sa29AcGcuZWR1LnBs